- Cellular and Organismic Networks, Faculty of Biology, Center for Organismic Adaptation, Ludwig-Maximilians-Universität München, Munich, Germany
Question: The large earth bumble bee (Bombus terrestris) maintains a social core gut-microbiota, similar as known from the honey bee, which plays an important role for host health and resistance. Experiments under laboratory conditions with commercial hives are limited to vertically transmitted microbes and neglect influences of environmental factors or external acquisition of microbes. Various environmental and landscape-level factors may have an impact on the gut-microbiota of pollinating insects, with consequences for pollinator health and fitness in agroecosystems. Still, it is not fully clear whether access to different flower diversities will have a significant influence on the bumble bee microbiota. Here, we tested in a semi-field experiment if the bumble bee microbiota changes over time when exposed to different flower diversities within outdoor flight cages. We used commercial hives to distinguish between vertically and horizontally transmitted bacteria, respectively from the nest environment or the exposed outside environment.
Result: The sequential sampling of foraging workers over a period of 35 days indicated a temporal progression of the bumble bee microbiota when placed outside. The microbiota increased in diversity and changed in composition and variability over time. We observed a major increase in relative abundance of the families Lactobacillaceae, Bifidobacteriaceae and Weeksellaceae. In contrast, major core-taxa like Snodgrassella and Gilliamella declined in their relative abundance over time. The genus Lactobacillus showed a high diversity and strain specific turnover, so that only specific ASVs showed an increase over time, while others had a more erratic occurrence pattern. Exposure to different flower diversities had no significant influence on the progression of the bumble bee microbiota.
Conclusion: The bumble bee microbiota showed a dynamic temporal succession with distinct compositional changes and diversification over time when placed outdoor. The exposure of bumble bees to environmental conditions, or environmental microbes, increases dissimilarity and changes the gut-community composition. This shows the importance of environmental influences on the temporal dynamic and progression of the bumble bee microbiota.
1 Introduction
Bumble bees are important for ecosystem service worldwide due to their role as pollinators for a large variety of plants (Klein et al., 2007; Garibaldi et al., 2013). They are of high commercial value, as they can be used for the pollination of various agricultural-grown plants within field environments (Goulson, 2003; Nayak et al., 2020) and are bred for commercial use in glasshouse environments (Velthuis and Van Doorn, 2006). On some crops, e.g. tomatoes, they are even more effective in pollination than honey bees, due to characteristics like buzz pollination (Vallejo-Marín, 2022). Given the current threats of diseases and parasites as Varroa mites to honey bees, alternative native species are in need to maintain crop and wild plant seed sets (Kevan et al., 1990; Garibaldi et al., 2013; Parreño et al., 2022). To preserve the vital services that bumble bees provide to ecosystems and agriculture, it is essential to prioritize their health and conservation. Especially in agricultural landscapes, increased land use intensity and monocultures cumulate several stressors like pesticides or lowered nutritional quality with negative effects on bumble bee health and colony fitness (Straub et al., 2023). Likewise to other insect groups, bumble bee diversity and abundance has been declining for decades with lower reproduction success in agricultural landscapes compared to urban environments (Williams and Osborne, 2009; Samuelson et al., 2018). Major issues are the reduction in floral resources and diversity of food plants as well as the lack of appropriate nesting sites (Goulson et al., 2008).
Microbes play an essential role for bee health and resistance, as they help not only with digestion and nutrient uptake (Bonilla-Rosso and Engel, 2018; Zheng et al., 2019), but provide protection against stressors like pathogens, parasites and toxins (Engel et al., 2012; Cariveau et al., 2014; Daisley et al., 2020; Motta et al., 2022). For the large earth bumble bee (B. terrestris) as well as the common eastern bumble bee (B. impatiens), the microbiota is an important driver for the resistance against infections with the parasite Crithidia bombi (Koch and Schmid-Hempel, 2011b; Koch and Schmid-Hempel, 2012; Mockler et al., 2018). Similar to the honey bee, bumble bees are well known for their simple, but distinct, gut microbiota comprised of a low diversity of characteristic groups belonging to the genera Snodgrassella (Neisseriaceae), Gilliamella (Orbaceae), Lactobacillus (Lactobacillaceae) and Bifidobacterium (Bifidobacteriaceae) (Martinson et al., 2011; Koch and Schmid-Hempel, 2011a; Powell et al., 2016; Kwong et al., 2017; Hammer et al., 2021a). These groups are considered as corbiculate bee core-bacteria as they are conserved among Bombus and Apis species (Kwong and Moran, 2016; Raymann and Moran, 2018). Still, they show high host-specificity as Snodgrassella strains from honey bees (Apis) cannot colonize bumble bees (Bombus) and vice versa (Kwong et al., 2014; Sauers and Sadd, 2019). Besides these, bumble bees contain Bombus-specific groups, which are lacking in honey bees i.e. Schmid-hempelia (Orbaceae) and Bombiscardovia (Bifido-bacteriaceae) (Killer et al., 2010; Martinson et al., 2014). Even when reared indoors, bumble bees are able to maintain large parts of their core-microbiota (Meeus et al., 2015). These are maintained through different modes of social transfer and are usually conserved over different life-stages (Billiet et al., 2017b; Su et al., 2021; Zhang and Zheng, 2022). Snodgrassella and Gilliamella for example are mainly vertically transmitted to the offspring via the queen and are the first microbes to colonize the adult gut (Sauers and Sadd, 2019). A loss of Snodgrassella and Gilliamella could result in colonies with higher parasite infection rates (Barribeau et al., 2022). Another major component of the bee microbiota are ‘lactic acid bacteria’, which are a polyphyletic grouping of Lactobacillales (Firmicutes), and Bifidobacteriales (Actinobacteria) (Olofsson and Vásquez, 2008). These groups are mainly horizontally acquired and require contact to siblings within the nest, while others can also be transmitted by contact to the nesting material (Billiet et al., 2017b).
Besides these hive-maintained core-set of microbes, bumble bees can acquire several strains from the environment, which are considered non-core members, as they are usually lacking in laboratory rearing (Hammer et al., 2021a). Environmental acquisition can have a dominant influence on the microbiota of B. terrestris (Bosmans et al., 2018; Krams et al., 2022). A shift in the bumble bee microbiota composition when moved outdoors suggests that particularly Enterobacteria are acquired from outdoor environments, which can dominate the gut microbiota of bumble bees with up to 90% relative abundance (Parmentier et al., 2016). Thus foraging behavior and limited floral sources in agroecosystems can have a relevant influence on the microbiota of pollinators (Koch et al., 2012; Newbold et al., 2015; Miller et al., 2019; Martin et al., 2022). Change of nectar source or pollen availability in agroecosystems could have an influence on the bumble bee microbiota with potentially negative consequences for bumble bee health and resistance. Hence, it is important to better understand how environmental factors and landscape level drivers influence the bumble bee microbiota.
In this study we examined, how the microbiota of the bumble bee B. terrestris changes over time when exposed to outdoor conditions. We placed ten bumble bee colonies within a semi-field experiment into separate outdoor flight cages to answer the following questions: (1) How much does the gut-microbiota composition and diversity of adult bumble bees change over time when exposed to outdoor environments? (2) Does the exposure to different flower diversities influence the gut-microbiota of adult bumble bees?
2 Material and methods
2.1 Preparation of the field plots
Experiments were conducted in 2022 at the Biocenter of the Faculty of Biology of the Ludwig-Maximilians-University of Munich. We built a total of ten free flight cages using durable and non-impregnated nets as well as pine wood poles that covered a plot area of 2 × 2 meter and 1.75 meter height. Plants that are known to be frequently visited by bumble bees were sown out in eight of the plots in advance to bumble bee hive deposition: Trifolium repens, Trifolium pratense and Brassica napus. To create plots with higher plant diversity, four of the plots included seeds of Phacelia tanacetifolia, Medicago sativa, Borago officinalis and Papaver rhoeas. In each plot 75 g of seeds were used. If necessary, plots were watered and plant growth observed on a weekly basis. As the first eight plots were built in early April, all plants growing inside were sheltered from visitation of other pollinators. About ten weeks after sowing, the plots were sorted according to the observed flower diversity including naturally growing plants. Two additional plots (9 & 10) were built around already existing native plants which were accessible to native pollinators. Pictures were taken of each plot to index the blooming plants inside, which were ranked from 0 (lowest diversity) to 9 (highest diversity). The two plots around native plants represent the most extreme conditions with rank 0 (mainly mowed grass, with few flowers of Centaurea nigra and Lotus corniculatus) until rank 9 (natural meadow with high flower diversity including Trifolium pratense, Medicago sativa, Cirsium arvense, Knautia arvensis, Lotus corniculatus, Borago officinalis, Centaurea nigra, Silene nutans & Galium mollugo). Despite this planned setup of flower diversity gradient, individual bumble bees managed to escape and foraged on an unknown diversity of flowers outside of the outdoor flight cages.
2.2 Bumble bee sampling and sample processing
We obtained large earth bumble bees (Bombus terrestris) from a commercial seller (Biobest Group NV, Westerlo, Belgium). Bumble bees were either provided as ‘Mini Hives’ containing about 30 worker bumble bees (plot 1-8) or as ‘Super Mini Hives’ with around 40 workers (plot 9-10). All mini hives were equipped with a care-free nutrition system containing 1.5 liter of sugar solution and pollen supplement to guarantee bumble bee survival during transportation. One hive was placed into each of the plots and covered with cardboard and plastic foil as protection against rain and strong sunshine exposure. Bumble bees were able to leave the mini hive and forage within the flight cages ad libitum. The experiments with the bumble bees were conducted under permit: ROB-55.1-8646.NAT_02-8-81-11 according to the nature conservation act of Bavaria (Verordnung zur Ausführung des Bayerischen Naturschutzgesetzes, AVBayNatSchG). Before placement into the plots, one bumble bee from each mini hive was sampled as time point zero (‘t0’). After the placement it took a few days for the bumble bees to adapt to outdoor conditions and actively fly within free flight cages of each plot. As soon as bumble bees were seen flying, up to two individuals were sampled per time point and plot using a sweep net. Bumble bees were transported into the laboratory using in a sterile falcon tube and frozen at -20°C. As not all adult bumble bees from every colony were foraging at the same day, we collected some samples over multiple days and binned these for the analysis into seven sampling time points since release in the outdoor flight cages on June 22nd 2023: ‘t0’ (day 0), ‘t1’ (day 13/14), ‘t2’ (day 16/17), ‘t3’ (day 20), ‘t4’ (day 23), ‘t5’ (day 27) ‘t6’ (day 35). On the final sampling day (July 27th, 2022), the hive entrances were closed in the early morning, and all animals within the colony immobilized and sacrificed at -20°C. The hives were opened and two adults as well as one larva sampled from inside of each colony. No larvae could be obtained from the hive of plot 2, as there were none inside. Due to vandalism, two of the ten colonies (9 & 10) had to be sampled earlier, so that the final sampling (‘t6’) contains four adults from inside the colony sampled at day 27.
2.3 Sample processing, library preparation and sequencing
Bumble bees were removed from the freezer and dissected using flame sterilized tweezers to obtain the entire gut including crop, foregut and hindgut. For larval samples the entire body was used for DNA isolation. In total, 118 individual guts of adults and 9 larval samples were processed. DNA isolation was performed with individual samples using the ZymoBIOMICS 96 DNA Kits (Zymo Research) including bead beating at 3200 rpm for 15 min on a grant MPS-1 multiplate shaker (Grant Instruments). Negative extraction controls (NECs) as well as mock-community positive controls (Zymo Research) were included.
We used a dual-indexing approach to amplify the V4 region of the 16S rRNA gene as done by Kozich et al. (2013). This protocol includes barcoded primers containing Illumina adapter, index sequence, pad sequence and linker, followed by the gene specific primer 515f 5´-GTGCCAGCMGCCGCGGTAA-3´ and 806r 5´-GGACTACHVGGGTWTCTAAT-3´ (Caporaso et al., 2011). PCR amplification was performed using a Phusion Plus PCR Master Mix (Thermo Scientific) with the following program: 98°C for 30 sec, followed by 30 cycles of 98°C for 10 sec, 55°C for 10 sec, 72°C for 30 sec and a final chain elongation step at 72°C for 5 min. PCR amplification was done in triplicates (3 × 10µl) following the pipetting scheme from (Sickel et al., 2015). PCR products were checked on a E-Gel Power Snap Plus Electrophoresis Device (Thermo Fisher Scientific) using a 96 well E-gel with 1% Agarose and SYBR Safe. PCR products were normalized using SequalPrep Normalisation Plates (Invitrogen) and pooled into four plate pools. Library quality and fragment size of the plate pools was checked using the High Sensitivity DNA Chip on a 2100 Bioanalyzer (Agilent Technologies). DNA concentration was measured with 1×dsDNA HS Assay Kit on a Qubit 4 Fluorometer (Thermo Fisher Scientific). The four plate pools were pooled equimolarly to a final dilution of 2 nM and paired-end sequenced (2 × 250) on an Illumina MiSeq platform (LMU Biocenter Martinsried) with 5% PhiX control spiked into the library.
2.4 Illumina sequence processing and microbiota data analysis
To prepare the sequencing data for further analysis, it was processed using VSEARCH v2.14.2 (Rognes et al., 2016) following the metabarcoding processing pipeline available at https://github.com/chiras/metabarcoding_pipeline (Leonhardt et al., 2022). Paired ends of forward and reverse reads were joined, and all reads shorter than 150 bp were removed. Furthermore, quality filtering (EE < 1) as described by Edgar and Flyvbjerg (2015) and de-novo chimera filtering following UCHIME3 (Edgar, 2016b) was performed. VSEARCH was also used to define amplicon sequence variants (ASVs) (Edgar, 2016b). By using VSEARCH against the RDP reference database, reads were directly mapped with global alignments with an identity cut-off threshold of 97%. To classify still remaining reads without taxonomic allocation at this point, SINTAX was used with the same reference database (Edgar, 2016a).
The raw dataset contained 3,887,305 reads and was clustered into 756 ASVs. Non-microbial reads of host organelles like chloroplasts were removed from the dataset. Based on prevalence abundance plots low abundant and low prevalent ASVs were filtered using a quality threshold of 100 reads minimum total abundance and a minimum prevalence of 2 samples within the entire dataset. This step removed in sum only 0.16% of reads from the Bombus samples, but eliminated all extreme low abundant and spurious phyla from the dataset (i.e. Acidobacteria, Armati-monadetes, candidate division WPS−1, Gemmati-mondetes, Planctomycetes, Tenericutes and Verrucomicrobia). The final dataset contained quality ASVs from the phyla Proteobacteria, Firmicutes, Actinobacteria and Bacteroidetes.
Further all ASVs of the mock community used as positive control were filtered from the dataset to account for possible spillover into the samples. Low throughput sample cutoff was set to a minimum of 800 reads per sample (similar as observed for NEC samples). This step removed three larvae and one adult sample with low sequencing throughput from the dataset, retaining bumble bee samples with a median sample sum of 26987 reads (117 adults and 6 larvae). ASVs were binned on genus level and low abundant genera with less than 500 reads total abundance (relative abundance RA <0.015%) were removed, filtering 0.06% of total reads from the dataset. The final dataset contained 116 ASVs of 26 genera. Most of the analysis was performed with the dataset containing only the adult samples.
For the most abundant ASVs obtained the taxonomic assignments were further manually checked against the NCBI Nucleotide Collection and RefSeq Genome Database using nucleotide BLAST (blastn). The closest matching taxa were used together with ASV sequences to construct a phylogenetic tree using the Neighbor-Joining method in MEGA11 to cross-check for a correct phylogenetic placement (Supplementary Figure S1). In this regard, ASV43 was renamed from ‘Orbus’ to ‘Schmidhempelia’ and ASV11 was renamed from ‘Bifidobacterium’ to ‘Bombiscardovia’. For ASV6 the taxonomic placement was unclear due to the lack of culturable type strains and closest match to ‘unculturable Firmicutes’ from European bumble bees (Koch and Schmid-Hempel, 2011a). It was renamed from ‘Firmicutes’ to ‘Xylocopilactobacillus cf.’ as it seems closely related to recently isolated novel Lactobacillaceae strains from carpenter bees (Kawasaki et al., 2023). While some of the ‘Snodgrassella’ and ‘Gilliamella’ ASVs were renamed to ‘Snodgrassella-like’ and ‘Gilliamella-like’ as they indicate a more distant placement with more than 5% sequence variants to these strains. Percentage identities to Snodgrassella communis of 92.94% (ASV1626), 94.49% (ASV912) and 94.88% (ASV863). Percentage identities to Gilliamella bombi of 92.13% (ASV1546), 92.52% (ASV1536) and 94.9% (ASV175).
2.5 Statistical analysis
R (version 4.3.1) was used for statistical analysis including the ‘phyloseq’ package (McMurdie and Holmes, 2013). The core microbiome was defined with a minimum prevalence of 5% and minimum relative abundance of 0.1%. We used linear mixed effect models (lmm) with ‘cage’ as random factor as implemented in the ‘nlme’ package 3.1 (Pinheiro et al., 2023) to investigate the influence of flower diversity or sampling time point on the Shannon diversity. Permutational multivariate analysis of variance using the Bray-Curtis distance matrices (PERMANOVA) was performed as implemented in the adonis2 function with 9999 permutations and sample dissimilarity over time by using the ‘betadisper’ function from the ‘vegan’ package. The influence of sampling time point on the increase and decrease of specific bacterial families and genera was tested by a generalized linear model (glm) using a quasipoisson regression. The obtained p-values from the glm analyses were corrected for multiple testing using the BH method.
3 Results
3.1 Adult bumble bees show a simple microbiota composition dominated by major core-taxa
We performed a semi-field experiment using outdoor flight cages to investigate how the provision of different flower diversities might change the gut-microbiota of the large earth bumble bee (B. terrestris) over time. Adult bees were consecutively sampled within seven sampling time points over a period of 35 days and their gut microbiota analyzed by 16S metabarcoding.
The overall community composition of adult bumble bees showed a relative low diversity and was dominated largely by the families Neisseriaceae, Orbaceae and Lactobacillaceae (Figure 1A). These families form the major core-microbiota and were found with high prevalence in nearly all individuals. Together with Bifidobacteriaceae and Weeksellaceae they are responsible for a relative abundance (RA) of 99.3% of the entire community. Across all samples, the dominating genera were Snodgrassella (RA 41.4%), Gilliamella (RA 33.1%) and Lactobacillus (RA 14.7%). The majority of reads for Snodgrassella and Gilliamella could be accounted each to a single ASV (Figure 1B), which matched to strains like S. communis (ASV1 RA 40.8%) as well as G. bombi (ASV2 RA 32.5%), both previously isolated from bumble bees (Praet et al., 2017; Cornet et al., 2022) (Supplementary Figure S1). Other Gilliamella-like and Snodgrasella-like ASVs showed a more distant placement to these type strains, but occurred in rather low abundance. The third most abundant family was Lactobacillaceae, which showed overall a high strain diversity with multiple ASVs within the genus Lactobacillus (Figure 1B). When applying the phylotype nomenclature used in the past for the honey bee (Ellegaard et al., 2015), these Lactobacillus spp. would be accounted to the ‘Firm-5’ clade closely related to Lactobacillus bombicola, L. panisapium and L. apis (Supplementary Figure S1). With 2% relative abundance Xylocopilactobacillus cf. (ASV6) was the second most abundant genus after Lactobacillus and represents probably a novel phylotype of bumble bee-related Lactobacillaceae (Supplementary Figure S1). Other characteristic Bombus-related symbionts were Bombiscardovia (RA 1.7%) (Killer et al., 2010) and Schmidhempelia (RA 0.2%) (Martinson et al., 2014) (Figure 1B). Apilactobacillus and Bombilactobacillus (‘Firm-4’) showed each with less than 0.07% only a very low relative abundance in the adults.
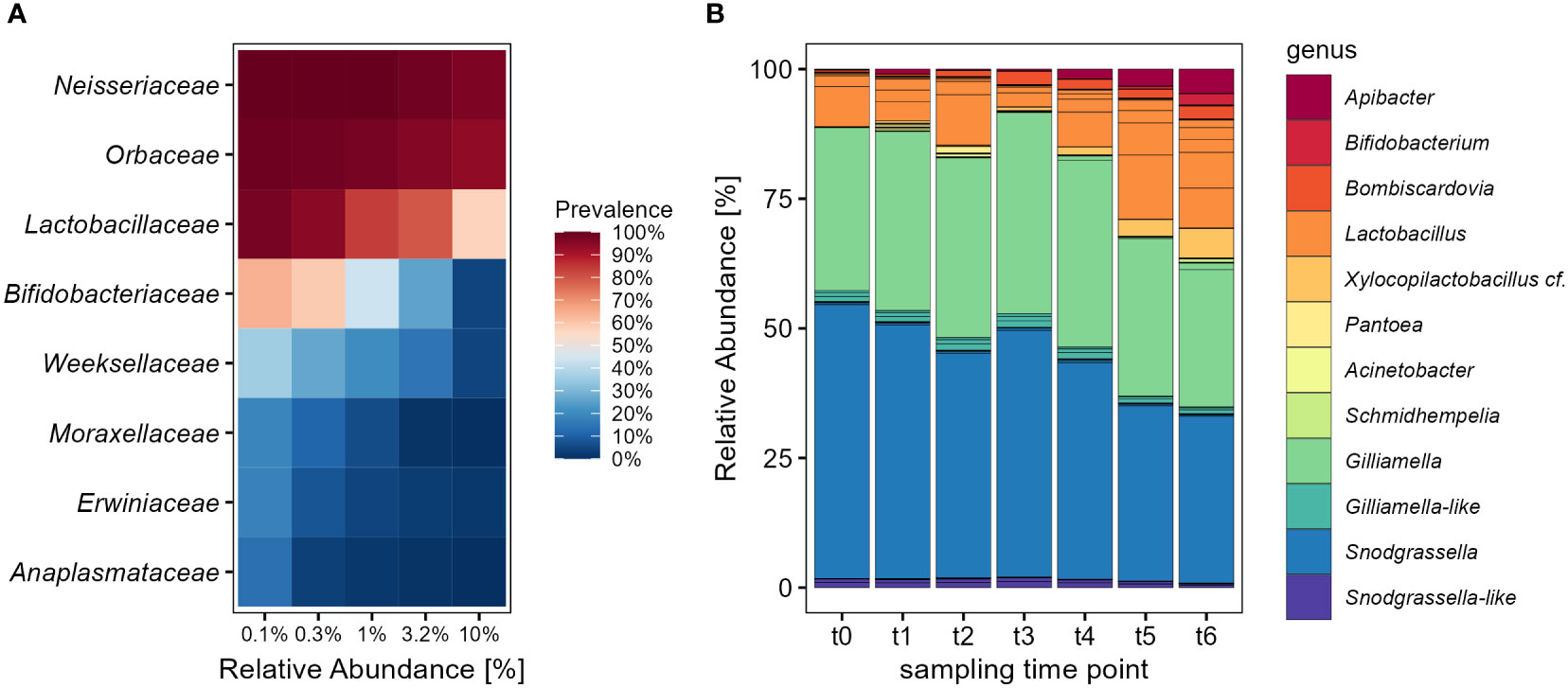
Figure 1 The composition of the large earth bumble bee (B. terrestris) gut-microbiota changes over time with a decrease of major core-taxa. (A) Core analysis of the most abundant bacterial families within the gut-microbiota of adult bumble bees across all sampling time points. The families Neisseriaceae until Weeksellaceae make up to 99.3% relative abundance. (B) Relative distribution of the bacterial community on ASV level, colored by genus level. Foraging worker of B terrestris were sampled in six sampling time points since release into outdoor flight cages for a period of 35 days. Only bacterial genera with relative abundance of >0.2% are shown.
3.2 Bumble bee microbiota increase in diversity and dissimilarity over time
Despite the simplicity of the bumble bee microbiota the genera Apibacter, Bifidobacterium, Bombiscardovia, Lactobacillus and Xylocopilactobacillus cf. indicate an increasing relative abundance over the course of the seven sampling time points (Figure 1B). We tested with linear mixed-effects models with cage as random factor, if there is a temporal change in alpha diversity of the microbial communities and found a significant influence of sampling time point on Shannon diversity. Since the release into outdoor flight cages alpha diversity increased on ASV level (lmm: t = 5.17, p < 0.0001) as well as on genus level (lmm: t = 3.73, p = 0.0003). This increase in Shannon diversity was even more pronounced on ASV level (R2 = 0.19) than on genus level (R2 = 0.11) (Figure 2). In addition, we tested whether the provision of different flower diversity ranks within the different flight cages would influence the bumble bee microbiota. When applying a linear mixed-effects model we found no significant influence of flower diversity on Shannon diversity of the bumble bee microbiota on ASV level (lmm: t = -1.149, p > 0.28) nor on genus level (lmm: t = -0.167, p > 0.87) (Supplementary Figures S3A, B). Even when comparing only the two most extreme flower diversity ranks of 0 and 9 (mowed meadow vs natural meadow flowers diversity) there was no significant influence of flower diversity on bacterial Shannon diversity on ASV level (Wilcoxon test: p > 0.75) nor genus level (Wilcoxon test, p > 0.91). Reasons for the lack of an effect within this setup is discussed later.
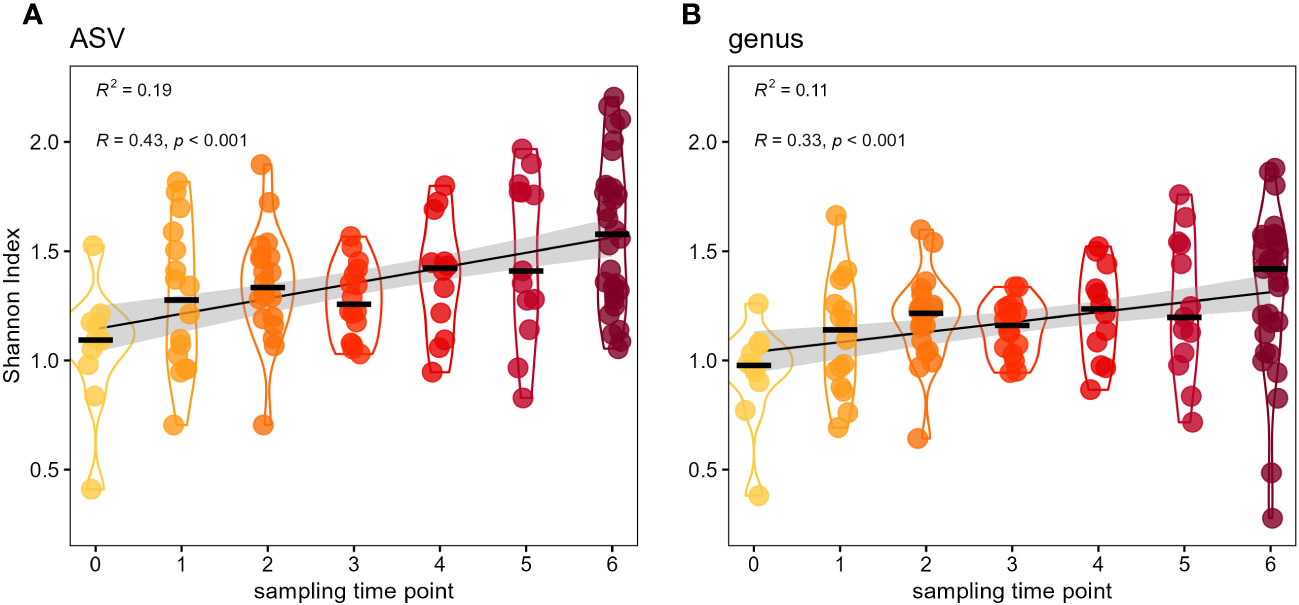
Figure 2 Diversity of the bumble bee gut-microbiota increases by sampling time point. Temporal increase in Shannon diversity of the adult bumble bee gut-microbiota on ASV level (A), as well as genus level (B). Foraging bumble bees (B. terrestris) were sampled in different sampling time points (‘t0’ to ‘t6’) since release into outdoor flight cages.
Besides this temporal progression of alpha diversity increase, we investigated whether dissimilarity among individual samples would also change over time, i.e. whether individuals from different colonies become more different to each other. Beta diversity was shown by Bray-Curtis distance using non-metric multidimensional scaling (NMDS) colored by sampling time point (Figure 3A). To better illustrate the temporal changes, each time point is shown and highlighted in an individual plot from the same NMDS (Figures 3B–H). Sampling time point had a significant influence on the Bray-Curtis distance (PERMANOVA F6,116 = 3.64, p = 0.0001). Beta diversity expanded particularly in the last two sampling time points (‘t5’ and ‘t6’), which showed the highest sample dissimilarity within the dataset (Figures 3G, H). By applying a mixed-effects model, community dissimilarity changes significantly over time independent from colony identity (lmm: t = 5.07, p < 0.0001) (Figure 3I). The largest differences in beta distance were evident between time point ‘t3’ and ‘t6’ (Wilcoxon test with BH correction p < 0.0001). These results show a temporal increase in sample variation so that the microbiota of bumble bees become more diverse over time. When applying a similar analysis using food plant provision, we found no influence of the flower diversity ranking on microbial community composition (PERMANOVA F9,116 = 1.31, p = 0.15) (Supplementary Figure S3C). Likewise, flower diversity had no significant effect on beta distance of the bumble bee microbiota (lmm: t = -1.01, p > 0.34) (Supplementary Figure S3D). Even when comparing only the two most extreme flower diversity rankings of 0 and 9, bumble bees showed no significant difference in the beta distance (Wilcoxon test: p > 0.3).
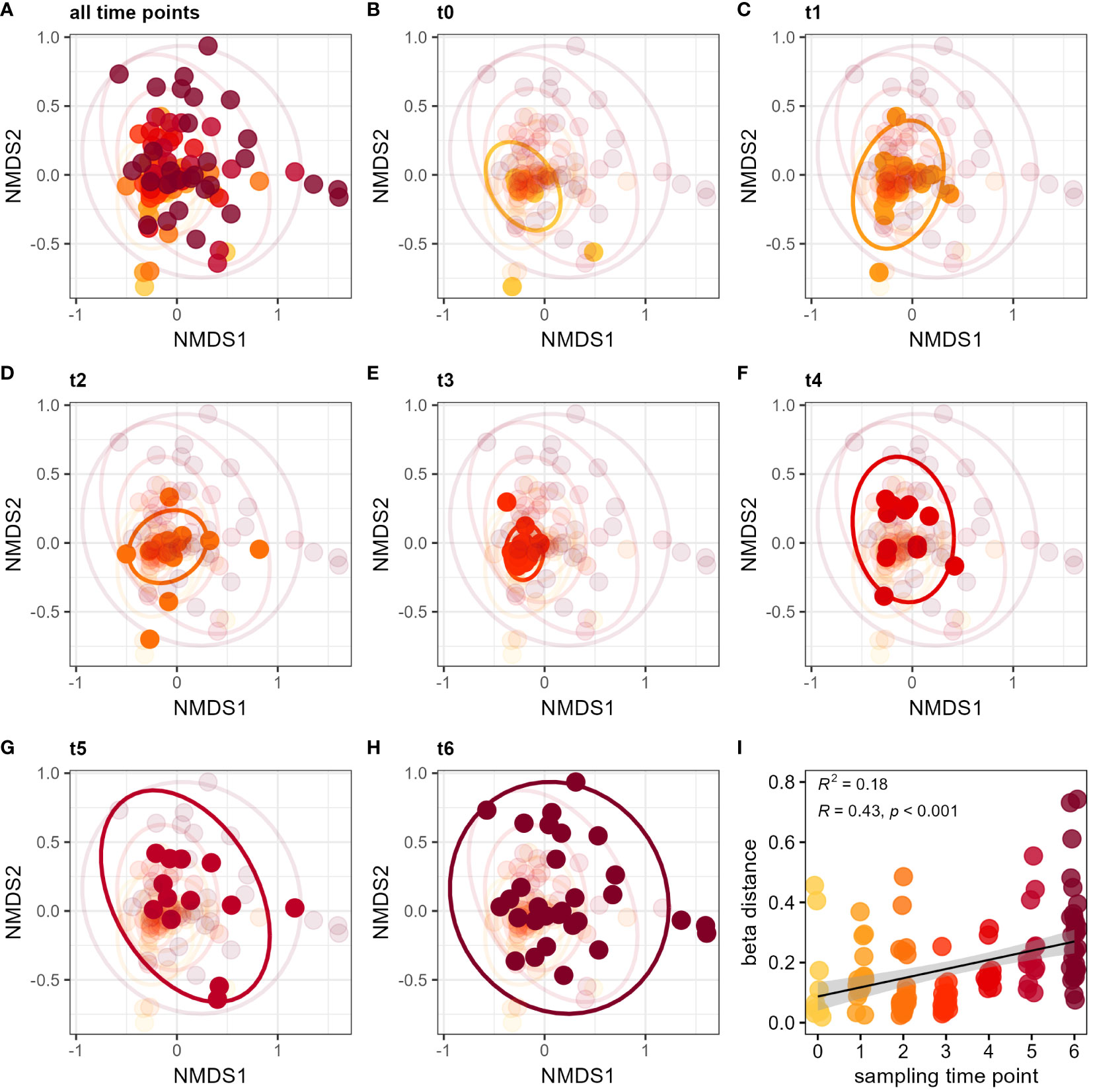
Figure 3 Diversification of the bumble bee gut-microbiota over time. NMDS plots show Bray-Curtis distance for all sampling time points (A), as well as for individual sampling time points ‘t0’ (B), ‘t1’ (C), ‘t2’ (D), ‘t3’ (E), ‘t4’ (F), ‘t5’ (G) and ‘t6’ (H). Increase of beta distance by sampling time points (I). The different time points (‘t0’ to ‘t6’) are indicated by color (yellow to red). Late sampling time points show a higher dissimilarity of the bumble bee microbiota since release into outdoor flight cages.
3.3 Temporal turnover of individual bacterial families
To further evaluate which bacterial groups were responsible for the increase in diversity and dissimilarity over time, we looked at the temporal changes in relative abundance of individual bacterial families. This showed that the families of Bifidobacteriaceae, Weeksellaceae and particularly Lactobacillaceae indicate an increase in relative abundance, while Neisseriaceae and Orbaceae tend to decrease (Figure 4). We used generalized linear models with quasi-poisson distribution and corrected p-values for multiple testing by the BH method. Here we found a positive influence of sampling time point on the relative abundance of Bifidobacteriaceae (glm: t = 4.81, p < 0.0001), Weeksellaceae (glm: t = 2.76, p = 0.01) and Lactobacillaceae (glm: t = 4.85, p < 0.0001). The latter showed such a drastic increase that some bumble bee samples from the final sampling time point (‘t6’) were even dominated by Lactobacillaceae (Figure 4). On the other hand, there was a reciprocal trend for other families to decrease in relative abundance. The core-families Neisseriaceae (glm: t = -5.63, p < 0.0001) and Orbaceae (glm: t = -2.23, p = 0.034) showed a significant decrease in their relative abundance over the course of the sampling period (Figure 4). Others, like the family of Erwiniaceae showed no temporal trend over time (glm: t = -1.75, p = 0.082), but occurred only occasionally in a few samples with low relative abundance in the entire dataset (RA <0.4%). This shows that the temporal diversification of the bumble bee microbiota was mainly due to an increase in relative abundance of the families Bifidobacteriaceae, Weeksellaceae and Lactobacillaceae, while the abundance of major core-members within the Neisseriaceae and Orbaceae decreased.
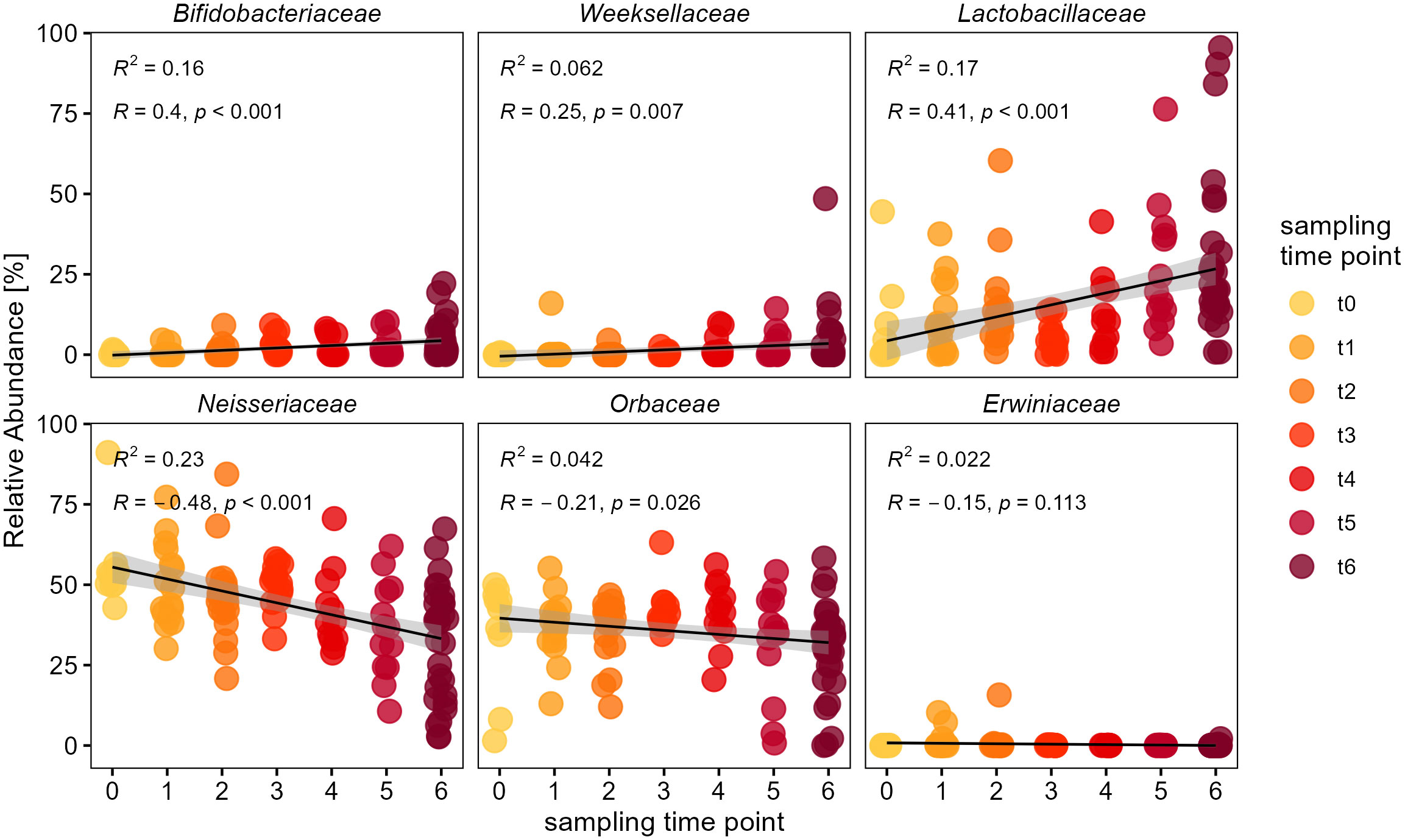
Figure 4 Temporal change of individual bacterial families within the bumble bee gut-microbiota. Relative abundances of individual bacterial families since release of bumble bees into outdoor flight cages. The families Bifidobacteriaceae, Weeksellaceae and Lactobacillaceae show an increase in relative abundance, while major core-taxa i.e. Neisseriaceae and Orbaceae show a decrease over time. The different sampling time points (‘t0’ to ‘t6’) are indicated by color (yellow to red). Only major families with a cumulative relative abundance of 99.7% are shown.
3.4 Temporal progression on genus level
For a more detailed analysis we also investigated temporal changes of the most abundant bacterial genera (Figure 5). Apibacter was the only genus among the Weeksellaceae and showed the same pattern on genus level (glm: t = 2.76, p = 0.01). Among the Bifidobacteriaceae, both genera of Bifidobacterium (glm: t = 2.96, p < 0.01) as well as Bombiscardovia (glm: t = 2.81, p < 0.01) showed a significant increase in relative abundance over time. In the family Lactobacillaceae the genera of Lactobacillus (glm: t = 3.61, p = 0.0012) as well as Xylocopilactobacillus cf. (glm: t = 4.29, p < 0.001) showed an increase in relative abundance over time (Figure 5). The family Neisseriaceae showed the strongest trend for a temporal decrease mainly due to a significant decrease of the genus Snodgrassella (glm: t = -5.40, p < 0.0001), as well as for the low abundant Snodgrassella-like ASVs (glm: t = -4.07, p < 0.001). Though overall more variable in abundance, the family of Orbaceae showed still a significant decrease of the genus Gilliamella (glm: t = -2.15, p = 0.04) as well as for the Gilliamella-like ASVs (glm: t = -3.58, p = 0.001), but not for Schmidhempelia (glm: t = 0.54, p = 0.59).
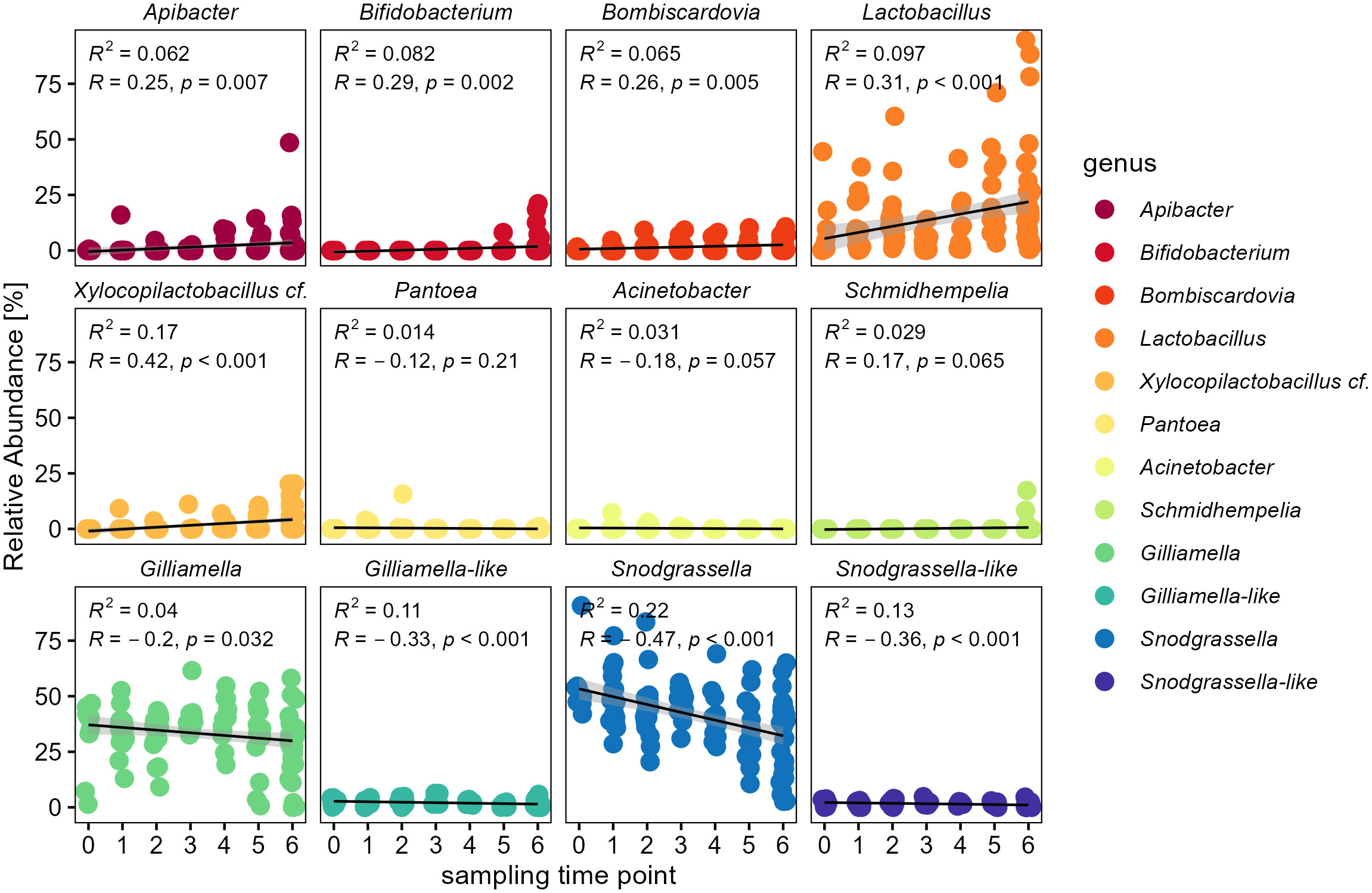
Figure 5 Temporal change of individual bacterial genera within the bumble bee gut-microbiota. Relative abundances of individual genera show an increase of Apibacter (Weeksellaceae), Bifidobacterium and Bombiscardovia (Bifidobacteriaceae), Lactobacillus and Xylocopilactobacillus cf. (Lactobacillaceae). Major core-taxa show a decrease in relative abundance: Gilliamella (Orbaceae) and Snodgrassella (Neisseriaceae). Only genera with relative abundance of >0.1% are shown.
3.5 Comparison of adults sampled outside and inside of the hives during the final sampling time point
At the final sampling time point (‘t6’) bumble bees were not only sampled outside of the colonies by a net, but as well from inside the colony. For this analysis we included the few larval samples (n=6) which have been obtained from the opened hives. We found only marginal differences in community composition among the sampling groups from the final time point (PERMANOVAt6 F2,35 = 1.93, p = 0.042). The adults sampled outside of the colony seem to contain larger abundances of Apibacter (RA 8.5%) compared to the adults sampled from inside the colony (RA 2.0%), while those from inside the colony showed higher abundance of Bifidobacterium (RA 3.8% vs 0.04%) (Supplementary Figure S2). Notably, Schmidhempelia was only detected in four individuals sampled from inside the colony (4 of 20), but not in any of the foraging adults sampled outside of the colonies (0 of 97). Similar, also Bombilactobacillus was mainly detected in adults and larvae from inside the colony (RA 0.35%) but was nearly absent in foraging adults (RA 0.01%). In contrast, the larval samples differed from the adults as they contained larger relative abundance of Pediococcus (RA 16.7% vs 0.11%), as well as higher abundance of Apilactobacillus (RA 1.01% vs 0.04%).
3.6 Turnover of individual ASV strains among Lactobacillaceae
Within the bumble bee microbiota, the family of Lactobacillaceae stood out as it contained a much higher ASV diversity compared to other bacterial families. We were interested whether these ASVs show a turnover in their abundance over the sampling time points and if only particular strains increase in abundance while other might even decrease. As we compared all major ASVs to the closest matching type strains (Supplementary Figure S1) we were able to obtain a near species level resolution among Lactobacillus spp. This allows us to have a deeper look into the dynamics within the family of Lactobacillaceae from time point ‘t0’ to ‘t6’ (Supplementary Figure S4). The observed increase in the genus Lactobacillus was mainly due to an increase in ASV7 related to Lactobacillus apis (glm: t = 4.56, p < 0.001) as well as ASV5 and ASV26 related to L. panisapium (ASV5, glm: t = 3.24, p < 0.005; ASV26, glm: t = 2.31, p = 0.051). While those ASVs related to L. bombicola showed a more variable abundance over time with no clear trend for an increase (ASV3, glm: t = -1.06, p = 0.435; ASV4, glm: t = 1.12, p = 0.435). Hence, the temporal change within the genus Lactobacillus is highly strain specific and only some ASVs within this group show an increase, while others have a more erratic occurrence pattern (Supplementary Figure S4). Even on ASV level Xylocopilactobacillus cf. (ASV6, glm: t = 4.30, p < 0.001) shows a significant increase over time and reaches a relative abundance of up to 5.9% in the final sampling time point. Other low abundant groups like Bombilactobacillus (ASV64, glm: t = 0.18, p > 0.85) or Fructobacillus (ASV55, glm: t = -0.32, p > 0.84) indicated no significant change.
4 Discussion
4.1 Environmental influence and plasticity of the bumble bee microbiota
We investigated how the exposure to outdoor environments changes the microbiota of the bumble bee B. terrestris. We found a temporal succession of the bumble bee microbiota with an increase in diversity and sample dissimilarity over time, but no significant influence of flower diversity ranking. The bumble bee microbiota in our dataset showed overall a low diversity and was mainly dominated by the genera Snodgrassella, Gilliamella and Lactobacillus (Figure 1). These are typical core-groups which could be found in most of our individuals and are known to be highly conserved among social corbiculate bees (Kwong and Moran, 2016; Kwong et al., 2017; Zhang and Zheng, 2022). We could demonstrate that the bumble bee microbiota shows a temporal succession with a reduction of prominent core-members Snodgrassella and Gilliamella, which were replaced mainly by an increasing relative abundance of Lactobacillus (Figure 5). Such a shifted microbiota composition has been previously associated with higher parasite infection rates (Barribeau et al., 2022), but it remains unclear whether community shifts are a result of the infections or would render colonies more susceptible. But following the progression of bee microbiota assembly on a temporal gradient has only been investigated in a few studies, i.e. with A. cerana (Dong et al., 2021) or B. impatiens (Hammer et al., 2023a). Temporal shifts in community composition can be explained by aging of the hives or an accumulation of a higher diversity of environmentally acquired strains, so that other core-members appear to diminish in relative abundance.
Even for the bumble bee B. terrestris with a socially maintained core-microbiota, environmental influences can have a large impact on the microbial community composition (Newbold et al., 2015; Parmentier et al., 2016). In general, mainly Entero-bacteriaceae, Apibacter (Weeksellaceae) and Fructobacillus (Lactobacillaceae) are considered as environmentally acquired strains, as these groups usually lack in laboratory environments (Newbold et al., 2015; Hammer et al., 2021a). Environmental influences can be shown by location or habitat dependence, as colonies of B. terrestris near forest environments were dominated by Fructobacillus compared to colonies in agricultural or horticultural landscapes (Krams et al., 2022). An investigation of 28 Chinese bumble bee species revealed two distinct enterotypes either dominated by core-members of the microbiota (Snodgrassella and Gilliamella) or by externally acquired microbes mainly belonging to Entero-bacteriaceae (Li et al., 2015). When moving colonies of B. terrestris outdoors, the microbiota can shift towards an increase in Enterobacteriaceae (Parmentier et al., 2016). Such a shift in wild bumble bee microbiota is often considered as a ‘disrupted’ microbiome and associated with higher pathogen load (Villabona et al., 2023). Overall, the influence of environmental microbes differs a lot between different studies, and it remains unclear what causes such community shifts. Within our dataset, Entero-bacteriales showed only a very low abundance and did not contribute to the progression in compositional turnover over time. We observed only an occasional occurrence of Pantoea (Erwiniaceae) in some of the early time points (RA <0.4%). Similar, Acinetobacter (Moraxellaceae) showed only an occasional occurrence with very low abundance (RA 0.2%), but is a common isolate of honey bees as well as floral nectar (Kim et al., 2014; Alvarez-Perez et al., 2021). Although it is putatively environmentally acquired, Apibacter can be considered as typical member of the bumble bee gut-microbiota (Praet et al., 2016; Steele and Moran, 2021; Hammer et al., 2021a). We observed an increase in relative abundance of Apibacter over time, similar as shown for the Asian honey bee A. cerana (Dong et al., 2021). We also found lower abundance of Apibacter in adults sampled from inside the colony compared to foraging adults, which is evidence that this group is mainly environmentally acquired.
4.2 Increase and high strain diversity of Lactobacillaceae
Similar as for honey bees (Ellegaard et al., 2015), we observed a high diversity of Lactobacillus strains in B. terrestris. Lactobacilli are a highly diverse group and multiple strains have been isolated from honeybees (Olofsson et al., 2014) as well as other wild bees and flowers (McFrederick et al., 2018). Several of these strains which have been previously classified as ‘Lactobacillus spp.’ showed diverging properties and have been later split into different genera (Zheng et al., 2020). These are: Apilactobacillus (previously known as the L. kunkeei group), Bombilactobacillus (previously known as L. bombi ‘Firm-4’ group) and Lactobacillus (previously known as ‘Firm-5’ group). Here, we would add Xylocopilactobacillus cf. as a novel bumble bee associated phylotype. This is probably a novel group of bumble bee-related Lactobacillaceae with yet unclear taxonomic placement (distinct from Lactobacillus, Bombi-lactobacillus and Apilactobacillus) (Supplementary Figure S1). Similar strains have been already cloned from B. terrestris in earlier studies (Mohr and Tebbe, 2006) (GenBank: AJ880198), but could not be further classified and were described until now only as ‘uncultured Firmicutes’ from bumble bees (GenBank: HM215045) (Koch and Schmid-Hempel, 2011a). This group has been occasionally reported as ‘Firm-3’ cluster (McFrederick et al., 2013; Leonhardt and Kaltenpoth, 2014) and seems to be characteristic for European bumble bee populations, as it has not been described for B. impatiens (Mockler et al., 2018; Hammer et al., 2023a). This provides opportunities to characterize a new phylotype of Bombus-associated Lactobacilli. So far, related culturable strains have only recently been isolated from carpenter bees and characterized as strictly anaerobic with auxotrophy for NAD biosynthesis (Kawasaki et al., 2023). They were proposed as a new genus of Xylocopilactobacillus gen. nov (Kawasaki et al., 2023). Although carpenter bees (Xylocopa) are not eusocial (but rather facultatively, incipiently or sub-social), their microbiota shows surprising parallels to that of Bombus species, with similar conserved core-taxa including Schmidhempelia, Bombilactobacillus and Bombiscardovia (Gu et al., 2023; Handy et al., 2023). Here it can be speculated that the long life expectancy of the females in Xylocopa species which share the nests with the offspring adult generation (Velthuis and Gerling, 1983), allows for a similar microbial transfer as otherwise only known from eusocial corbiculate bees.
For bumble bees, the relationship with lactic acid bacteria seems to be highly strain specific (McFrederick et al., 2013) and adults usually require the direct contact to nestmates for an acquisition and propagation of this group within the hive (Billiet et al., 2017b). B. terrestris cannot be colonized by any other Lactobacillus strains as a probiotic treatment, while Bombus-specific strains showed stable colonization (Billiet et al., 2017a). This shows that bee-related Lactobacillus strains cannot be replaced by other generic strains. The proliferation and diversification of lactic acid bacteria within bumble bee guts point at an important functional role of this group for host fitness. Lactic acid bacteria are known for their importance to honey bee health (Vásquez et al., 2012; Killer et al., 2014; Iorizzo et al., 2022) and resemble an important part of the bumble bee microbiota. For some ground nesting bees they can be even the dominating taxon within their gut-microbiota (Hammer et al., 2023b).
In our dataset, the genus Lactobacillus showed a high strain diversity on ASV level, which further proliferated across the sampling time points. The temporal increase in this genus could be mainly observed for the strain Lactobacillus apis (ASV7), originally isolated from honey bees (Killer et al., 2014), as well as L. panisapium (ASV5, ASV26) isolated from bee bread (Wang et al., 2018). This could be indication that these groups have been acquired via direct or indirect contact with honey bees during bumble bee foraging. Other Lactobacillus ASVs were related to L. bombicola (ASV3, ASV4), which had been previously described from bumble bees (Praet et al., 2015). These showed a more erratic occurrence within individual bumble bee samples with no clear temporal trend towards an in- or decrease in abundance. Whether this means that this strain might be hive-maintained and is not environmentally acquired is not fully clear.
As an alternative explanation, environmental temperatures could influence community composition in bumble bees when exposed to outdoor conditions. An increase in rearing temperatures had a positive effect on the proliferation of Lactobacillaceae within the gut microbiota of B. impatiens (Palmer-Young et al., 2019). Hence, even putative Bombus-specific strains like Xylocopilactobacillus cf. could proliferate in their relative abundance due to increasing temperatures without the need for an acquisition from environmental sources. However, the core-taxa Snodgrassella and Gilliamella show likewise a better growth rate at elevated temperatures (Hammer et al., 2021b), but were decreasing in relative abundance within the course of our sampling period. But even air pollution can selectively erode the microbiota of B. terrestris from important core-taxa like Snodgrassella (Seidenath et al., 2023).
Behavioral experiments with B. impatiens showed that bumble bees seem to avoid flowers inoculated with Apilactobacillus micheneri, pointing at a deterring effect of some lactic acid bacteria from environmental sources (Russell and Ashman, 2019). This strain was previously isolated as Lactobacillus micheneri from the gut of sweat bees Halictus ligatus and has been associated with flowers and other megachilid bees (McFrederick et al., 2017, 2018). In contrast, the inoculation of nectar with Fructobacillus lead to an increased nectar consumption by B. impatiens (Russell and McFrederick, 2022). These bumble bees can not only acquire, but even disperse microbes among flowers themselves (Russell et al., 2019). For solitary bees, which do not have a social microbiome, environmental acquisition is often the only source to obtain a more diverse microbiota (Voulgari-Kokota et al., 2019a, Voulgari-Kokota et al., 2019b; Cohen et al., 2020). Hence, flowers should not only be seen as a source of food provision alone, but as well as dispersal hubs for beneficial as well as detrimental microbes (McFrederick et al., 2017; Figueroa et al., 2019; Adler et al., 2021; Tehel et al., 2022). This highlights the importance of conducting microbiome studies with pollinating insects under field conditions to account for environmental acquisition and microbial transfer across plant-pollinator networks.
4.3 Temporal shifts of the bumble bee microbiota
The microbiota of bees can show dynamic plasticity over time, when followed over different life stages and seasons (Dong et al., 2021; Li et al., 2021; Su et al., 2021). For B. terrestris, developmental changes have been investigated for different larval stages, which differed clearly in their microbiota compared to the adults (Guo et al., 2023). Larvae of B. terrestris have been described to be mainly colonized by Lactobacillus (Su et al., 2021), while we found all major core groups from the adults within the larvae. The major difference was the colonization by an unspecific Pediococcus (Lactobacillaceae). But the overall lower sequencing depth in our larval samples is also indicative for a much lower microbial biomass in the larvae compared to the adults. As a result, three of the nine larval samples needed to be removed due to low sequencing depth. Upon hatching, adult bumble bees, much like honeybees, emerge bacteria-free and acquire their microbiota from their food, hive environment or nestmates (Koch and Schmid-Hempel, 2011b; Hammer et al., 2021b). This process happens within the first 4 days of the adult life so that the overall microbial load remains relatively stable with progressing adult age for B. impatiens (Hammer et al., 2023a). Hence, colony aging has some observable, but more subtle influences on microbial community composition. When reared indoors, the microbiota of B. terrestris and B. impatiens shows no larger change in alpha diversity over time (Parmentier et al., 2016; Yu et al., 2023; Hammer et al., 2023a). This was clearly different in our setup, as the placement into outdoor environments resulted in diversification of bumble bee microbiota, observable by an increase in in alpha diversity as well as an increase in sample dissimilarity over time. Especially the increase in dissimilarity from time point ‘t4’ to ‘t6’ could indicate that a new generation of worker have emerged into a more diverse hive environment.
Though diversity levels did not change, Hammer et al. (2023a) reported a community shift of the bumble bee microbiota with increasing colony age, resulting in a decrease in Schmidhempelia and the establishment of Gilliamella, while proportions of Lactobacillus remain relatively stable over a period of 60 days. Though Schmidhempelia has been described as dominant member of the microbiota of the common eastern bumble bee (B. impatiens) (Hammer et al., 2023a), we found it only with low abundances within a few individuals of B. terrestris. We observed also larger shifts in community composition with a decrease in relative abundance of Gilliamella, while Lactobacillaceae showed a strong increase within a 35 day period. Here, it is important to note that the previous study with B. impatiens was conducted in a laboratory setting which can only reflect the influence of colony aging, whereas our study used B. terrestris and was performed under environmental conditions in outdoor cages. Seasonal changes and sampling time point are strong predictor of the honeybee microbiota independent from geographic location (Almeida et al., 2023). Those phenological influences are important biological factors, which are missed in lab colony studies.
4.4 Why flower diversity had no influence on the bumble bee microbiota
There are several possible explanations why the flower diversity ranking of the provided food plants had no significant influence on the bumble bee microbiota within our setup (Supplementary Figure S3). First, only a few of the sowed plants bloomed early enough to provide nectar and pollen in sufficient quantities so that the bumble bees relied primarily on the resources provided by their mini hives. Hence, the provided flower density might have been too low to show an effect. Removing the nutrient solution from the hives could help in future experiments to force bumble bees to forage outside of the hives, but this provides the risk of introducing a bias due to malnutrition. Second, our initial setup excluded other pollinators and does not allow floral visitation and introduction of microbes from wild pollinators (but only wind-dispersed microbes). Including other insects within a modified setup could provide additional insights about the importance of cross-species transfer of microbes, since vectoring insects can move microbes along the plant-pollinator network and share them with other pollinators (Keller et al., 2021; Zemenick et al., 2021; Weinhold, 2022). As the third reason, several bumble bees manage to escape through tiny holes that have been bitten into the nets and could be observed returning from foraging flights outside of the cages. Hence, they were exposed to an unknown diversity of flowering plants outside of the assigned area and could introduce microbes from the surrounding environment. Even though they showed an excellent sense of orientation and returned precisely to their specific hives, this all blurs the influence of the provided flower diversity gradient. As a result, the ten cages with the treatment groups did not differ in their microbial diversity nor dissimilarity and conclusions about the influence of the provided flower diversity rank should be taken with caution.
4.5 Conclusions
While social transfer is the most important route for bumble bees to maintain a conserved core-microbiota, floral visitation provides further chances for microbial acquisition and transfer. As this increases also the risk of pathogen exposure from other pollinators, the maintenance of a social core that protects bumble bees during their first flights from parasite infections is of great importance. Still, bumble bees are able to acquire a more diverse microbiota from their surrounding environment. Hence laboratory rearing conditions cannot fully replicate the symbiont dynamics that include environmental acquisition routes within native ecosystems. This should be incentive for further experiments, to elucidate how much floral diversity alone can contributes to a diversification of the bumble bee microbiota, or if a combination of high flower diversity with a broad range of pollinating insects is necessary to result significant outcome. In nature, both of these factors are usually difficult to disentangle, since floral diversity has also a reciprocal influence on pollinator diversity.
Data availability statement
The datasets presented in this study can be found in online repositories. The names of the repository/repositories and accession number(s) can be found below: https://www.ncbi.nlm.nih.gov/bioproject/PRJNA1042966/.
Ethics statement
Ethical approval was not required for the study involving animals in accordance with the local legislation and institutional requirements because work with insect invertebrates does not require any approval by an animal ethics committee.
Author contributions
AW: Conceptualization, Data curation, Formal analysis, Investigation, Methodology, Supervision, Validation, Visualization, Writing – original draft, Writing – review & editing. EG: Conceptualization, Formal analysis, Investigation, Methodology, Visualization, Writing – review & editing. AK: Conceptualization, Funding acquisition, Project administration, Resources, Supervision, Writing – review & editing.
Funding
The author(s) declare financial support was received for the research, authorship, and/or publication of this article. AW acknowledges support by the LMUexcellent Postdoc Support Fund.
Acknowledgments
The authors would like to thank Lars Landgraf for help during insect sampling, Anna Preußner for help during building of the experimental setup and Uschi Schkölziger for technical assistance. Further, the authors want to thank Andreas Brachmann from the Genomics Service Unit of the LMU for help during sequencing and the government of upper Bavaria for providing sampling permits for B. terrestris.
Conflict of interest
The authors declare that the research was conducted in the absence of any commercial or financial relationships that could be construed as a potential conflict of interest.
Publisher’s note
All claims expressed in this article are solely those of the authors and do not necessarily represent those of their affiliated organizations, or those of the publisher, the editors and the reviewers. Any product that may be evaluated in this article, or claim that may be made by its manufacturer, is not guaranteed or endorsed by the publisher.
Supplementary material
The Supplementary Material for this article can be found online at: https://www.frontiersin.org/articles/10.3389/fcimb.2024.1342781/full#supplementary-material
References
Adler, L. S., Irwin, R. E., McArt, S. H., Vannette, R. L. (2021). Floral traits affecting the transmission of beneficial and pathogenic pollinator-associated microbes. Curr. Opin. Insect Sci. 44, 1–7. doi: 10.1016/j.cois.2020.08.006
Almeida, E. L., Ribiere, C., Frei, W., Kenny, D., Coffey, M. F., O’Toole, P. W. (2023). Geographical and seasonal analysis of the honeybee microbiome. Microb. Ecol. 85, 765–778. doi: 10.1007/s00248-022-01986-x
Alvarez-Perez, S., Baker, L. J., Morris, M. M., Tsuji, K., Sanchez, V. A., Fukami, T., et al. (2021). Acinetobacter pollinis sp. Nov., acinetobacter baretiae sp. nov. and acinetobacter rathckeae sp. nov., isolated from floral nectar and honey bees. Int. J. Syst. Evol. Microbiol. 71, 004783. doi: 10.1099/ijsem.0.004783
Barribeau, S. M., Schmid-Hempel, P., Walser, J. C., Zoller, S., Berchtold, M., Schmid-Hempel, R., et al. (2022). Genetic variation and microbiota in bumble bees cross-infected by different strains of C. bombi. PLoS One 17, e0277041. doi: 10.1371/journal.pone.0277041
Billiet, A., Meeus, I., Cnockaert, M., Vandamme, P., Van Oystaeyen, A., Wäckers, F., et al. (2017a). Effect of oral administration of lactic acid bacteria on colony performance and gut microbiota in indoor-reared bumblebees (Bombus terrestris). Apidologie 48, 41–50. doi: 10.1007/s13592-016-0447-5
Billiet, A., Meeus, I., Van Nieuwerburgh, F., Deforce, D., Wäckers, F., Smagghe, G. (2017b). Colony contact contributes to the diversity of gut bacteria in bumblebees (Bombus terrestris). Insect Sci. 24, 270–277. doi: 10.1111/1744-7917.12284
Bonilla-Rosso, G., Engel, P. (2018). Functional roles and metabolic niches in the honey bee gut microbiota. Curr. Opin. Microbiol. 43, 69–76. doi: 10.1016/j.mib.2017.12.009
Bosmans, L., Pozo, M. I., Verreth, C., Crauwels, S., Wilberts, L., Sobhy, I. S., et al. (2018). Habitat-specific variation in gut microbial communities and pathogen prevalence in bumblebee queens (Bombus terrestris). PloS One 13, 1–19. doi: 10.1371/journal.pone.0204612
Caporaso, J. G., Lauber, C. L., Walters, W. A., Berg-Lyons, D., Lozupone, C. A., Turnbaugh, P. J., et al. (2011). Global patterns of 16S rRNA diversity at a depth of millions of sequences per sample. Proc. Natl. Acad. Sci. U. S. A. 108, 4516–4522. doi: 10.1073/pnas.1000080107
Cariveau, D. P., Elijah Powell, J., Koch, H., Winfree, R., Moran, N. A., Article, O. (2014). Variation in gut microbial communities and its association with pathogen infection in wild bumble bees (Bombus). ISME J. 8, 2369–2379. doi: 10.1038/ismej.2014.68
Cohen, H., McFrederick, Q. S., Philpott, S. M. (2020). Environment shapes the microbiome of the blue orchard bee, osmia lignaria. Microb. Ecol. 80, 897–907. doi: 10.1007/s00248-020-01549-y
Cornet, L., Cleenwerck, I., Praet, J., Leonard, R. R., Vereecken, N. J., Michez, D., et al. (2022). Phylogenomic analyses of snodgrassella isolates from honeybees and bumblebees reveal taxonomic and functional diversity. mSystems 7, e01500–e01521. doi: 10.1128/msystems.01500-21
Daisley, B. A., Chmiel, J. A., Pitek, A. P., Thompson, G. J., Reid, G. (2020). Missing microbes in bees: how systematic depletion of key symbionts erodes immunity. Trends Microbiol. 28, 1010–1021. doi: 10.1016/j.tim.2020.06.006
Dong, Z. X., Chen, Y. F., Li, H. Y., Tang, Q. H., Guo, J. (2021). The succession of the gut microbiota in insects: A dynamic alteration of the gut microbiota during the whole life cycle of honey bees (Apis cerana). Front. Microbiol. 12. doi: 10.3389/fmicb.2021.513962
Edgar, R. (2016a). SINTAX: a simple non-Bayesian taxonomy classifier for 16S and ITS sequences. bioRxiv, 074161. doi: 10.1101/074161
Edgar, R. C. (2016b). UNOISE2: improved error-correction for Illumina 16S and ITS amplicon sequencing. bioRxiv, 081257. doi: 10.1101/081257
Edgar, R. C., Flyvbjerg, H. (2015). Error filtering, pair assembly and error correction for next-generation sequencing reads. Bioinformatics 31, 3476–3482. doi: 10.1093/bioinformatics/btv401
Ellegaard, K. M., Tamarit, D., Javelind, E., Olofsson, T. C., Andersson, S. G. E., Vásquez, A. (2015). Extensive intra-phylotype diversity in lactobacilli and bifidobacteria from the honeybee gut. BMC Genomics 16, 1–22. doi: 10.1186/s12864-015-1476-6
Engel, P., Martinson, V. G., Moran, N. A. (2012). Functional diversity within the simple gut microbiota of the honey bee. Proc. Natl. Acad. Sci. 109, 11002–11007. doi: 10.1073/pnas.1202970109
Figueroa, L. L., Blinder, M., Grincavitch, C., Jelinek, A., Mann, E. K., Merva, L. A., et al. (2019). Bee pathogen transmission dynamics: Deposition, persistence and acquisition on flowers. Proc. R. Soc. B 286, 20190603. doi: 10.1098/rspb.2019.0603
Garibaldi, L. A., Steffan-Dewenter, I., Winfree, R., Aizen, M. A., Bommarco, R., Cunningham, S. A., et al. (2013). Wild pollinators enhance fruit set of crops regardless of honey bee abundance. Sci. (80-.). 339, 1608–1611. doi: 10.1126/science.1230200
Goulson, D., Lye, G. C., Darvill, B. (2008). Decline and conservation of bumble bees. Annu. Rev. Entomol. 53, 191–208. doi: 10.1146/annurev.ento.53.103106.093454
Gu, Y., Han, W., Wang, Y., Liang, D., Gao, J., Zhong, Y., et al. (2023). Xylocopa caerulea and Xylocopa auripennis harbor a homologous gut microbiome related to that of eusocial bees. Front. Microbiol. 14. doi: 10.3389/fmicb.2023.1124964
Guo, B., Tang, J., Ding, G., Mashilingi, S. K., Huang, J., An, J. (2023). Gut microbiota is a potential factor in shaping phenotypic variation in larvae and adults of female bumble bees. Front. Microbiol. 14. doi: 10.3389/fmicb.2023.1117077
Hammer, T. J., Easton-Calabria, A., Moran, N. A. (2023a). Microbiome assembly and maintenance across the lifespan of bumble bee workers. Mol. Ecol. 32, 724–740. doi: 10.1111/mec.16769
Hammer, T. J., Kueneman, J., Argueta-Guzmán, M., McFrederick, Q. S., Grant, L., Wcislo, W., et al. (2023b). Bee breweries: The unusually fermentative, lactobacilli-dominated brood cell microbiomes of cellophane bees. Front. Microbiol. 14. doi: 10.3389/fmicb.2023.1114849
Hammer, T. J., Le, E., Martin, A. N., Moran, N. A. (2021a). The gut microbiota of bumblebees. Insectes Soc 68, 287–301. doi: 10.1007/s00040-021-00837-1
Hammer, T. J., Le, E., Moran, N. A., Hammer, T. J. (2021b). Thermal niches of specialized gut symbionts: The case of social bees. Proc. R. Soc. B 288, 20201480. doi: 10.1098/rspb.2020.1480
Handy, M. Y., Sbardellati, D. L., Yu, M., Saleh, N. W., Ostwald, M. M., Vannette, R. L. (2023). Incipiently social carpenter bees (Xylocopa) host distinctive gut bacterial communities and display geographical structure as revealed by full-length PacBio 16S rRNA sequencing. Mol. Ecol. 32, 1530–1543. doi: 10.1111/mec.16736
Iorizzo, M., Letizia, F., Ganassi, S., Testa, B., Petrarca, S., Albanese, G., et al. (2022). Functional properties and antimicrobial activity from lactic acid bacteria as resources to improve the health and welfare of honey bees. Insects 13, 1–28. doi: 10.3390/insects13030308
Kawasaki, S., Ozawa, K., Mori, T., Yamamoto, A., Ito, M., Ohkuma, M., et al. (2023). Symbiosis of carpenter bees with uncharacterized lactic acid bacteria showing NAD auxotrophy. Microbiol. Spectr. 11, 1–15. doi: 10.1128/spectrum.00782-23
Keller, A., McFrederick, Q. S., Dharampal, P., Steffan, S., Danforth, B. N., Leonhardt, S. D. (2021). (More than) Hitchhikers through the network: The shared microbiome of bees and flowers. Curr. Opin. Insect Sci. 44, 8–15. doi: 10.1016/j.cois.2020.09.007
Kevan, P. G., Clark, E. A., Thomas, V. G. (1990). Insect pollinators and sustainable agriculture. Am. J. Altern. Agric. 5, 13–22. doi: 10.1017/S0889189300003179
Killer, J., Dubná, S., Sedláček, I., Švec, P. (2014). Lactobacillus apis sp. nov., from the stomach of honeybees (Apis mellifera), having an in vitro inhibitory effect on the causative agents of American and European foulbrood. Int. J. Syst. Evol. Microbiol. 64, 152–157. doi: 10.1099/ijs.0.053033-0
Killer, J., Kopečný, J., Mrázek, J., Havlík, J., Koppová, I., Benada, O., et al. (2010). Bombiscardovia coagulans gen. nov., sp. nov., a new member of the family Bifidobacteriaceae isolated from the digestive tract of bumblebees. Syst. Appl. Microbiol. 33, 359–366. doi: 10.1016/j.syapm.2010.08.002
Kim, P. S., Shin, N. R., Kim, J. Y., Yun, J. H., Hyun, D. W., Bae, J. W. (2014). Acinetobacter apis sp. nov., isolated from the intestinal tract of a honey bee, Apis mellifera. J. Microbiol. 52, 639–645. doi: 10.1007/s12275-014-4078-0
Klein, A. M., Vaissière, B. E., Cane, J. H., Steffan-Dewenter, I., Cunningham, S. A., Kremen, C., et al. (2007). Importance of pollinators in changing landscapes for world crops. Proc. R. Soc. B 274, 303–313. doi: 10.1098/rspb.2006.3721
Koch, H., Cisarovsky, G., Schmid-Hempel, P. (2012). Ecological effects on gut bacterial communities in wild bumblebee colonies. J. Anim. Ecol. 81, 1202–1210. doi: 10.1111/j.1365-2656.2012.02004.x
Koch, H., Schmid-Hempel, P. (2011a). Bacterial communities in central european bumblebees: low diversity and high specificity. Microb. Ecol. 62, 121–133. doi: 10.1007/s00248-011-9854-3
Koch, H., Schmid-Hempel, P. (2011b). Socially transmitted gut microbiota protect bumble bees against an intestinal parasite. Proc. Natl. Acad. Sci. U. S. A. 108, 19288–19292. doi: 10.1073/pnas.1110474108
Koch, H., Schmid-Hempel, P. (2012). Gut microbiota instead of host genotype drive the specificity in the interaction of a natural host-parasite system. Ecol. Lett. 15, 1095–1103. doi: 10.1111/j.1461-0248.2012.01831.x
Kozich, J. J., Westcott, S. L., Baxter, N. T., Highlander, S. K., Schloss, P. D. (2013). Development of a dual-index sequencing strategy and curation pipeline for analyzing amplicon sequence data on the miseq illumina sequencing platform. Appl. Environ. Microbiol. 79, 5112–5120. doi: 10.1128/AEM.01043-13
Krams, R., Gudra, D., Popovs, S., Willow, J., Krama, T., Munkevics, M., et al. (2022). Dominance of fructose-associated fructobacillus in the gut microbiome of bumblebees (Bombus terrestris) inhabiting natural forest meadows. Insects 13, 98. doi: 10.3390/insects13010098
Kwong, W. K., Engel, P., Koch, H., Moran, N. A. (2014). Genomics and host specialization of honey bee and bumble bee gut symbionts. Proc. Natl. Acad. Sci. U. S. A. 111, 11509–11514. doi: 10.1073/pnas.1405838111
Kwong, W. K., Medina, L. A., Koch, H., Sing, K. W., Soh, E. J. Y., Ascher, J. S., et al. (2017). Dynamic microbiome evolution in social bees. Sci. Adv. 3, 1–17. doi: 10.1126/sciadv.1600513
Kwong, W. K., Moran, N. A. (2016). Gut microbial communities of social bees. Nat. Rev. Microbiol. 14, 374–384. doi: 10.1038/nrmicro.2016.43
Leonhardt, S. D., Kaltenpoth, M. (2014). Microbial communities of three sympatric Australian stingless bee species. PloS One 9, 1–6. doi: 10.1371/journal.pone.0105718
Leonhardt, S. D., Peters, B., Keller, A. (2022). Do amino and fatty acid profiles of pollen provisions correlate with bacterial microbiomes in the mason bee Osmia bicornis? Philos. Trans. R. Soc. B. 377, 20210171. doi: 10.1098/rstb.2021.0171
Li, J., Powell, J. E., Guo, J., Evans, J. D., Wu, J., Williams, P., et al. (2015). Two gut community enterotypes recur in diverse bumblebee species. Curr. Biol. 25, R652–R653. doi: 10.1016/j.cub.2015.06.031
Li, K., Wang, L., Zhang, Z., Guo, Y., Guo, J., Chen, Y., et al. (2021). Dynamic change of gut microbiota in the male bee of bombus terrestris (Hymenoptera: apidae). J. Agric. Sci. 13, 163. doi: 10.5539/jas.v13n9p163
Martin, V. N., Schaeffer, R. N., Fukami, T. (2022). Potential effects of nectar microbes on pollinator health. Philos. Trans. R. Soc. B. 377, 20210155. doi: 10.1098/rstb.2021.0155
Martinson, V. G., Danforth, B. N., Minckley, R. L., Rueppell, O., Tingek, S., Moran, N. A. (2011). A simple and distinctive microbiota associated with honey bees and bumble bees. Mol. Ecol. 20, 619–628. doi: 10.1111/mec.2011.20.issue-3
Martinson, V. G., Mago, T., Koch, H., Salzberg, S. L., Moran, N. A. (2014). Genomic features of a bumble bee symbiont reflect its host environment. Appl. Environ. Microbiol. 80, 3793–3803. doi: 10.1128/AEM.00322-14
McFrederick, Q. S., Cannone, J. J., Gutell, R. R., Kellner, K., Plowes, R. M., Mueller, U. G. (2013). Specificity between lactobacilli and hymenopteran hosts is the exception rather than the rule. Appl. Environ. Microbiol. 79, 1803–1812. doi: 10.1128/AEM.03681-12
McFrederick, Q. S., Thomas, J. M., Neff, J. L., Vuong, H. Q., Russell, K. A., Hale, A. R., et al. (2017). Flowers and wild megachilid bees share microbes. Microb. Ecol. 73, 188–200. doi: 10.1007/s00248-016-0838-1
McFrederick, Q. S., Vuong, H. Q., Rothman, J. A. (2018). Lactobacillus micheneri sp. nov., Lactobacillus timberlakei sp. nov. and Lactobacillus quenuiae sp. nov., lactic acid bacteria isolated from wild bees and flowers. Int. J. Syst. Evol. Microbiol. 68, 1879–1884. doi: 10.1099/ijsem.0.002758
McMurdie, P. J., Holmes, S. (2013). Phyloseq: an R package for reproducible interactive analysis and graphics of microbiome census data. PloS One 8, e61217. doi: 10.1371/journal.pone.0061217
Meeus, I., Parmentier, L., Billiet, A., Maebe, K., Van Nieuwerburgh, F., Deforce, D., et al. (2015). 16S rRNA amplicon sequencing demonstrates that indoor-reared bumblebees (Bombus terrestris) harbor a core subset of bacteria normally associated with the wild host. PloS One 10, 1–15. doi: 10.1371/journal.pone.0125152
Miller, D. L., Parish, A. J., Newton, I. L. G. (2019). Transitions and transmission: behavior and physiology as drivers of honey bee-associated microbial communities. Curr. Opin. Microbiol. 50, 1–7. doi: 10.1016/j.mib.2019.08.001
Mockler, B. K., Kwong, W. K., Moran, N. A., Koch, H. (2018). Microbiome structure influences infection by the parasite Crithidia bombi in bumble bees. Appl. Environ. Microbiol. 84, 1–11. doi: 10.1128/AEM.02335-17
Mohr, K. I., Tebbe, C. C. (2006). Diversity and phylotype consistency of bacteria in the guts of three bee species (Apoidea) at an oilseed rape field. Environ. Microbiol. 8, 258–272. doi: 10.1111/j.1462-2920.2005.00893.x
Motta, E. V. S., Gage, A., Smith, T. E., Blake, K. J., Kwong, W. K., Riddington, I. M., et al. (2022). Host-microbiome metabolism of a plant toxin in bees. Elife 11, 1–31. doi: 10.7554/eLife.82595
Nayak, R. K., Rana, K., Bairwa, V. K., Singh, P., Bharthi, V. D. (2020). A review on role of bumblebee pollination in fruits and vegetables. J. Pharmacogn. Phytochem. 9, 1328–1334. doi: 10.22271/phyto.2020.v9.i3v.11494
Newbold, L. K., Oliver, A. E., Cuthbertson, L., Walkington, S. E., Gweon, H. S., Heard, M. S., et al. (2015). Rearing and foraging affects bumblebee (Bombus terrestris) gut microbiota. Environ. Microbiol. Rep. 7, 634–641. doi: 10.1111/1758-2229.12299
Olofsson, T. C., Alsterfjord, M., Nilson, B., Butler, È., Vásquez, A. (2014). Lactobacillus apinorum sp. nov., Lactobacillus mellifer sp. nov., Lactobacillus mellis sp. nov., Lactobacillus melliventris sp. nov., Lactobacillus kimbladii sp. nov., Lactobacillus helsingborgensis sp. nov. and lactobacillus kullabergensis sp. nov., isol. Int. J. Syst. Evol. Microbiol. 64, 3109–3119. doi: 10.1099/ijs.0.059600-0
Olofsson, T. C., Vásquez, A. (2008). Detection and identification of a novel lactic acid bacterial flora within the honey stomach of the honeybee Apis mellifera. Curr. Microbiol. 57, 356–363. doi: 10.1007/s00284-008-9202-0
Palmer-Young, E. C., Ngor, L., Burciaga Nevarez, R., Rothman, J. A., Raffel, T. R., McFrederick, Q. S. (2019). Temperature dependence of parasitic infection and gut bacterial communities in bumble bees. Environ. Microbiol. 21, 4706–4723. doi: 10.1111/1462-2920.14805
Parmentier, L., Meeus, I., Mosallanejad, H., de Graaf, D. C., Smagghe, G. (2016). Plasticity in the gut microbial community and uptake of Enterobacteriaceae (Gammaproteobacteria) in Bombus terrestris bumblebees’ nests when reared indoors and moved to an outdoor environment. Apidologie 47, 237–250. doi: 10.1007/s13592-015-0393-7
Parreño, M. A., Alaux, C., Brunet, J. L., Buydens, L., Filipiak, M., Henry, M., et al. (2022). Critical links between biodiversity and health in wild bee conservation. Trends Ecol. Evol. 37, 309–321. doi: 10.1016/j.tree.2021.11.013
Pinheiro, J., Bates, D., DebRoy, S., Sarkar, D., Heisterkamp, S., Van Willigen, B., et al. (2023)nlme: linear and nonlinear mixed effects models. Available online at: https://cran.r-project.org/package=nlme.
Powell, E., Ratnayeke, N., Moran, N. A. (2016). Strain diversity and host specificity in a specialized gut symbiont of honeybees and bumblebees. Mol. Ecol. 25, 4461–4471. doi: 10.1111/mec.13787
Praet, J., Aerts, M., de Brandt, E., Meeus, I., Smagghe, G., Vandamme, P. (2016). Apibacter mensalis sp. Nov.: A rare member of the bumblebee gut microbiota. Int. J. Syst. Evol. Microbiol. 66, 1645–1651. doi: 10.1099/ijsem.0.000921
Praet, J., Cnockaert, M., Meeus, I., Smagghe, G., Vandamme, P. (2017). Gilliamella intestini sp. nov., Gilliamella bombicola sp. nov., Gilliamella bombi sp. nov. and Gilliamella mensalis sp. nov.: Four novel Gilliamella species isolated from the bumblebee gut. Syst. Appl. Microbiol. 40, 199–204. doi: 10.1016/j.syapm.2017.03.003
Praet, J., Meeus, I., Cnockaert, M., Houf, K., Smagghe, G., Vandamme, P. (2015). Novel lactic acid bacteria isolated from the bumble bee gut: Convivina intestini gen. nov., sp. nov., Lactobacillus bombicola sp. nov., and Weissella bombi sp. nov. Antonie van Leeuwenhoek. Int. J. Gen. Mol. Microbiol. 107, 1337–1349. doi: 10.1007/s10482-015-0429-z
Raymann, K., Moran, N. A. (2018). The role of the gut microbiome in health and disease of adult honey bee workers. Curr. Opin. Insect Sci. 26, 97–104. doi: 10.1016/j.cois.2018.02.012
Rognes, T., Flouri, T., Nichols, B., Quince, C., Mahé, F. (2016). VSEARCH: A versatile open source tool for metagenomics. PeerJ 2016, 1–22. doi: 10.7717/peerj.2584
Russell, A. L., Ashman, T. L. (2019). Associative learning of flowers by generalist bumble bees can be mediated by microbes on the petals. Behav. Ecol. 30, 746–755. doi: 10.1093/beheco/arz011
Russell, A. L., Rebolleda-Gómez, M., Shaible, T. M., Ashman, T. L. (2019). Movers and shakers: Bumble bee foraging behavior shapes the dispersal of microbes among and within flowers. Ecosphere 10, e02714. doi: 10.1002/ecs2.2714
Russell, K. A., McFrederick, Q. S. (2022). Elevated temperature may affect nectar microbes, nectar sugars, and bumble bee foraging preference. Microb. Ecol. 84, 473–482. doi: 10.1007/s00248-021-01881-x
Samuelson, A. E., Gill, R. J., Brown, M. J. F., Leadbeater, E. (2018). Lower bumblebee colony reproductive success in agricultural compared with urban environments. Proc. R. Soc. B 285, 2–10. doi: 10.1098/rspb.2018.0807
Sauers, L. A., Sadd, B. M. (2019). An interaction between host and microbe genotypes determines colonization success of a key bumble bee gut microbiota member. Evol. (N. Y). 73, 2333–2342. doi: 10.1111/evo.13853
Seidenath, D., Weig, A. R., Mittereder, A., Hillenbrand, T., Brüggemann, D., Opel, T., et al. (2023). Diesel exhaust particles alter gut microbiome and gene expression in the bumblebee Bombus terrestris. Ecol. Evol. 13, 1–29. doi: 10.1002/ece3.10180
Sickel, W., Ankenbrand, M. J., Grimmer, G., Holzschuh, A., Härtel, S., Lanzen, J., et al. (2015). Increased efficiency in identifying mixed pollen samples by meta-barcoding with a dual-indexing approach. BMC Ecol. 15, 1–9. doi: 10.1186/s12898-015-0051-y
Steele, M. I., Moran, N. A. (2021). Evolution of interbacterial antagonism in bee gut microbiota reflects host and symbiont diversification. mSystems. 6, e00063-21. doi: 10.1128/mSystems.00063-21
Straub, F., Birkenbach, M., Leonhardt, S. D., Ruedenauer, F. A., Kuppler, J., Wilfert, L., et al. (2023). Land-use-associated stressors interact to reduce bumblebee health at the individual and colony level. Proc. R. Soc. B 290, 20231322. doi: 10.1098/rspb.2023.1322
Su, Q., Wang, Q., Mu, X., Chen, H., Meng, Y., Zhang, X., et al. (2021). Strain-level analysis reveals the vertical microbial transmission during the life cycle of bumblebee. Microbiome 9, 1–14. doi: 10.1186/s40168-021-01163-1
Tehel, A., Streicher, T., Tragust, S., Paxton, R. J. (2022). Experimental cross species transmission of a major viral pathogen in bees is predominantly from honeybees to bumblebees. Proc. R. Soc. B 289, 20212255. doi: 10.1098/rspb.2021.2255
Vallejo-Marín, M. (2022). How and why do bees buzz? Implications for buzz pollination. J. Exp. Bot. 73, 1080–1092. doi: 10.1093/jxb/erab428
Vásquez, A., Forsgren, E., Fries, I., Paxton, R. J., Flaberg, E., Szekely, L., et al. (2012). Symbionts as major modulators of insect health: Lactic acid bacteria and honeybees. PloS One 7, e33188. doi: 10.1371/annotation/3ac2b867-c013-4504-9e06-bebf3fa039d1
Velthuis, H. H. W., Gerling, D. (1983). At the brink of sociality: Interactions between adults of the carpenter bee Xylocopa pubescens spinola. Behav. Ecol. Sociobiol. 12, 209–214. doi: 10.1007/BF00290773
Velthuis, H. H. W., Van Doorn, A. (2006). A century of advances in bumblebee domestication and the economic and environmental aspects of its commercialization for pollination. Apidologie 37, 421–451. doi: 10.1051/apido:2006019
Villabona, N., Moran, N., Hammer, T., Reyes, A. (2023). Conserved, yet disruption-prone, gut microbiomes in neotropical bumblebees. mSphere 8, e00139-23. doi: 10.1128/msphere.00139-23
Voulgari-Kokota, A., Ankenbrand, M. J., Grimmer, G., Steffan-Dewenter, I., Keller, A. (2019a). Linking pollen foraging of megachilid bees to their nest bacterial microbiota. Ecol. Evol. 9, 10788–10800. doi: 10.1002/ece3.5599
Voulgari-Kokota, A., McFrederick, Q. S., Steffan-Dewenter, I., Keller, A. (2019b). Drivers, diversity, and functions of the solitary-bee microbiota. Trends Microbiol. 27, 1034–1044. doi: 10.1016/j.tim.2019.07.011
Wang, C., Huang, Y., Li, L., Guo, J., Wu, Z., Deng, Y., et al. (2018). Lactobacillus panisapium sp. nov., from honeybee Apis cerana bee bread. Int. J. Syst. Evol. Microbiol. 68, 703–708. doi: 10.1099/ijsem.0.002538
Weinhold, A. (2022). Bowel movement: integrating host mobility and microbial transmission across host taxa. Front. Microbiol. 13. doi: 10.3389/fmicb.2022.826364
Williams, P. H., Osborne, J. L. (2009). Bumblebee vulnerability and conservation world-wide. Apidologie 40, 367–387. doi: 10.1051/apido/2009025
Yu, Q., Liu, Y., Liu, S., Li, S., Zhai, Y., Zhang, Q., et al. (2023). Lactobacillus melliventris promotes hive productivity and immune functionality in Bombus terrestris performance in the greenhouse. Insect Sci. 0, 1–16. doi: 10.1111/1744-7917.13281
Zemenick, A. T., Vanette, R. L., Rosenheim, J. A. (2021). Linked networks reveal dual roles of insect dispersal and species sorting for bacterial communities in flowers. Oikos 130, 697–707. doi: 10.1111/oik.06818
Zhang, Z. J., Zheng, H. (2022). Bumblebees with the socially transmitted microbiome: A novel model organism for gut microbiota research. Insect Sci. 29, 958–976. doi: 10.1111/1744-7917.13040
Zheng, H., Perreau, J., Elijah Powell, J., Han, B., Zhang, Z., Kwong, W. K., et al. (2019). Division of labor in honey bee gut microbiota for plant polysaccharide digestion. Proc. Natl. Acad. Sci. U. S. A. 116, 25909–25916. doi: 10.1073/pnas.1916224116
Zheng, J., Wittouck, S., Salvetti, E., Franz, C. M. A. P., Harris, H. M. B., Mattarelli, P., et al. (2020). A taxonomic note on the genus Lactobacillus: Description of 23 novel genera, emended description of the genus Lactobacillus beijerinck 1901, and union of Lactobacillaceae and Leuconostocaceae. Int. J. Syst. Evol. Microbiol. 70, 2782–2858. doi: 10.1099/ijsem.0.004107
Keywords: Bombus terrestris, microbiome, flower diversity gradient, Lactobacillaceae, core-microbiota, Lactobacillus, environmental acquisition, progression
Citation: Weinhold A, Grüner E and Keller A (2024) Bumble bee microbiota shows temporal succession and increase of lactic acid bacteria when exposed to outdoor environments. Front. Cell. Infect. Microbiol. 14:1342781. doi: 10.3389/fcimb.2024.1342781
Received: 22 November 2023; Accepted: 19 February 2024;
Published: 04 March 2024.
Edited by:
Paula Melisa Garrido, CONICET Mar del Plata, ArgentinaReviewed by:
Chinmay V. Tikhe, Johns Hopkins University, United StatesA Alwin Prem Anand, University of Tübingen, Germany
Copyright © 2024 Weinhold, Grüner and Keller. This is an open-access article distributed under the terms of the Creative Commons Attribution License (CC BY). The use, distribution or reproduction in other forums is permitted, provided the original author(s) and the copyright owner(s) are credited and that the original publication in this journal is cited, in accordance with accepted academic practice. No use, distribution or reproduction is permitted which does not comply with these terms.
*Correspondence: Arne Weinhold, YXJuZS53ZWluaG9sZEBiaW8ubG11LmRl