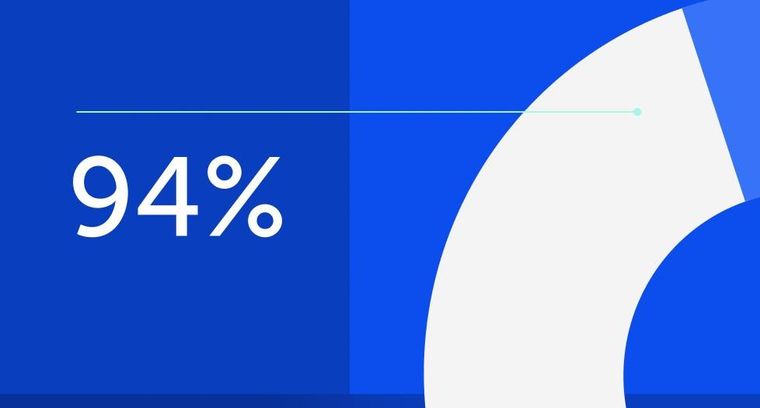
94% of researchers rate our articles as excellent or good
Learn more about the work of our research integrity team to safeguard the quality of each article we publish.
Find out more
ORIGINAL RESEARCH article
Front. Cell. Infect. Microbiol., 15 December 2023
Sec. Parasite and Host
Volume 13 - 2023 | https://doi.org/10.3389/fcimb.2023.1320160
Toxoplasmosis is a common protozoan infection that can have severe outcomes in the immunocompromised and during pregnancy, but treatment options are limited. Recently, nucleotide metabolism has received much attention as a target for new antiprotozoal agents and here we focus on pyrimidine salvage by Toxoplasma gondii as a drug target. Whereas uptake of [3H]-cytidine and particularly [3H]-thymidine was at most marginal, [3H]-uracil and [3H]-uridine were readily taken up. Kinetic analysis of uridine uptake was consistent with a single transporter with a Km of 3.3 ± 0.8 µM, which was inhibited by uracil with high affinity (Ki = 1.15 ± 0.07 µM) but not by thymidine or 5-methyluridine, showing that the 5-Me group is incompatible with uptake by T. gondii. Conversely, [3H]-uracil transport displayed a Km of 2.05 ± 0.40 µM, not significantly different from the uracil Ki on uridine transport, and was inhibited by uridine with a Ki of 2.44 ± 0.59 µM, also not significantly different from the experimental uridine Km. The reciprocal, complete inhibition, displaying Hill slopes of approximately -1, strongly suggest that uridine and uracil share a single transporter with similarly high affinity for both, and we designate it uridine/uracil transporter 1 (TgUUT1). While TgUUT1 excludes 5-methyl substitutions, the smaller 5F substitution was tolerated, as 5F-uracil inhibited uptake of [3H]-uracil with a Ki of 6.80 ± 2.12 µM (P > 0.05 compared to uracil Km). Indeed, we found that 5F-Uridine, 5F-uracil and 5F,2’-deoxyuridine were all potent antimetabolites against T. gondii with EC50 values well below that of the current first line treatment, sulfadiazine. In vivo evaluation also showed that 5F-uracil and 5F,2’-deoxyuridine were similarly effective as sulfadiazine against acute toxoplasmosis. Our preliminary conclusion is that TgUUT1 mediates potential new anti-toxoplasmosis drugs with activity superior to the current treatment.
T. gondii has the ability to infect any nucleated cell of any warm-blooded animal or bird, including those of humans, giving it possibly the widest host range of any parasite (Tenter et al., 2000). T. gondii, being acquired orally and not requiring a vector, is not geographically limited and is estimated to have infected approximately one-third of the global human population (Montoya and Liesenfeld, 2004; Hill et al., 2005). It can remain latent in the majority of its hosts, but can be deadly in immunocompromised people, and create severe adverse impacts on the unborn if acquired during pregnancy. Acute toxoplasmosis can result in severe cerebral outcomes including retinochoroiditis, encephalitis, hydrocephalus, convulsions and intracerebral calcification (Hill et al., 2005). In most immunocompetent humans, however, toxoplasmosis does not need treatment and protective immunity is naturally acquired.
However, treatment is vital for toxoplasmosis patients that are pregnant or immunocompromised. There are very few drugs for toxoplasmosis treatment which, moreover, have serious adverse effects and they are not useful for the bradyzoite stage. The first-line treatment for acute toxoplasmosis is the combination of two antimicrobial antifolate agents: pyrimethamine (PYR) or trimethoprim, which inhibit dihydrofolate reductase (DHFR), and sulphonamides such as sulfadiazine, sulfamethoxazole or sulfadoxine, which inhibit dihydropteroate synthetase (DHPS). The combination acts synergistically to disrupt the parasite’s folate metabolism (Alday and Doggett, 2017; Dunay et al., 2018). However, as pyrimethamine also inhibits the human DHFR (Heppler et al., 2022) it can cause hematological toxicity, making it necessary to give leucovorin or folinic acid together with the first-line treatment to combat the side effect (Alday and Doggett, 2017; Dunay et al., 2018).
Folate is an essential cofactor for the biosynthesis of thymidine nucleotides, and nucleotide metabolism in protozoan parasites is a vital biological process and increasingly explored as a drug target. Nucleoside antimetabolites have been shown to be effective drug candidates against various protozoan parasites, such as Trichomonas vaginalis (Natto et al., 2021a; Natto et al., 2021b), Trypanosoma brucei (Hulpia et al., 2020a; Hulpia et al., 2020b), Trypanosoma congolense (Mabille et al., 2022), Trypanosoma vivax (Ungogo et al., 2023), Trypanosoma cruzi (Fiuza et al., 2022) and apicomplexan parasites such as T. gondii (Al Safarjalani et al., 2010; Kim et al., 2010; Campagnaro et al., 2022) and Plasmodium falciparum (Cheviet et al., 2019).
Most protozoan parasites including T. gondii are able to synthesize pyrimidine nucleotides de novo as well as salvage pyrimidines such as thymidine and uracil (Finley et al., 1988; De Koning and Jarvis, 1998; Ghosh and Mukherjee, 2000; De Koning et al., 2003; Papageorgiou et al., 2005; Gudin et al., 2006; Ali et al., 2013) but some exceptions exist. Giardia lamblia, Tritrichomonas foetus, T. vaginalis (Hassan and Coombs, 1988; Berens et al., 1995) and C. parvum (Rider and Zhu, 2010) are pyrimidine auxotrophs and rely on pyrimidine uptake from the environment. In contrast, P. falciparum is incapable of pyrimidine salvage (Gutteridge and Trigg, 1970) but fully competent to synthesize pyrimidine nucleosides de novo (Polet and Barr, 1968).
UMP is the final product of pyrimidine biosynthesis, from which all other pyrimidine nucleotides, nucleosides and nucleobases can be produced. In addition, T. gondii is able to acquire uracil, (2’-deoxy)uridine and (2’-deoxy)cytidine from the host cell and transform the nucleosides to uracil using cytidine deaminase and uridine phosphorylase, and subsequently to UMP, exclusively by uridine phosphoribosyltransferase (UPRT) activity (Iltzsch, 1993; Fox and Bzik, 2020). This pyrimidine salvage strategy is known as a “salvage funnel”, routing all salvaged pyrimidines to uracil and thence to UMP (Pfefferkorn, 1988).
Purine and pyrimidine transporters have been extensively studied in kinetoplastids, particularly in Leishmania and Trypanosoma species (Aldfer et al., 2022a; Aldfer et al., 2022b; Al-Salabi et al., 2003; Al-Salabi and De Koning, 2005; Ungogo et al., 2023) as well as the apicomplexan P. falciparum (Parker et al., 2000; Quashie et al., 2008; Deniskin et al., 2015; Campagnaro and De Koning, 2020) but nucleoside and nucleobase transport in T. gondii has received relatively scant attention. However, a few studies have been conducted on purine uptake by extracellular T. gondii tachyzoites and these found a low affinity high-capacity adenosine transporter, which was named TgAT1 (Schwab et al., 1995), and was later supplemented with a high-affinity adenosine/inosine transport activity, TgAT2, and a separate high-affinity purine nucleobase (hypoxanthine/guanine) transporter, TgNBT1 (De Koning et al., 2003). The gene encoding TgAT1, Tg_244440, was identified from an adenine arabinoside (Ara-A)-resistant cell line, was characterized by expression in X. laevis oocytes, and was confirmed to be a low affinity, high-capacity transporter of adenosine (Km of 114 µM) (Chiang et al., 1999). Recently, expression of Tg_244440/TgAT1 in a T. brucei cell line provided further confirmation of the low affinity for adenosine but additionally found that the carrier displays higher affinity for oxopurine nucleobases and nucleosides (Campagnaro et al., 2022).
However, the transport of pyrimidines by T. gondii has not yet been reported and so far the only information is that uridine and thymidine appear to be inhibitors, and thus perhaps substrates, of TgAT2 (De Koning et al., 2003). Yet it is clear that at least some pyrimidines can be salvaged by intracellular trophozoites and incorporated into nucleic acids (Pfefferkorn and Pfefferkorn, 1977a). Indeed, the intracellular growth of pyrimidine auxotroph lineages can be rescued with a high concentration of uracil and uridine in the culture medium, providing conclusive proof that at least those pyrimidines can be salvaged by intracellular trophozoites (Fox and Bzik, 2002; Fox and Bzik, 2010). In contrast, thymidine is not salvageable by T. gondii, as it lacks thymidine kinase activity (Fox et al., 2001). Here, we investigate the uptake of pyrimidine nucleosides and uracil in isolated T. gondii tachyzoites and identify a previously unreported uridine/uracil carrier in T. gondii tachyzoites that appears to facilitate the uptake of potent pyrimidine antimetabolites.
The F3 (RH Δku80 TATi) strain (Sheiner et al., 2011) was first made fluorescent red through random integration of the tubTandemTomatoRFP/sagCAT (pCRT2t) plasmid (Chtanova et al., 2008) and selection via flow cytometry. This cell line was always used as the primary cell line for all transport assays and drug screening assays in this study.
T. gondii tachyzoites were cultured in human foreskin fibroblasts (HFF), sourced from ATCC (SCRC-1041). HFFs and parasites were culture in Dulbecco’s Modified Eagle’s Medium (DMEM), containing 4.5 gL-1 glucose, supplemented with 10% (v/v) fetal bovine serum, 4mM L-glutamine and penicillin/streptomycin antibiotics and grown at 37°C with 5% CO2. When needed anhydrotetracycline (ATc) was added to the medium at a final concertation of 0.5 µM.
The following tritium radiolabeled compounds were used during the project: [3H]-Uracil (40 Ci/mmol), [3H]-Thymidine (71.7 Ci/mmol), [3H]-Uridine (60 Ci/mmol) were obtained from PerkinElmer (Waltham, MA, USA). [3H]-Cytidine (20 Ci/mmol) was purchased from American Radiolabeled Chemicals Incorporated (St Louis, MO, USA). Uridine, Uracil, Thymidine, 5-Floururacil, 5-Flourouridine, 2’-Deoxyuridine, 3’-deoxythymidine, Sulfadiazine, NBMPR, Adenosine, Inosine, resazurin sodium salt and Phenyl Arsine Oxide were bought from Sigma-Aldrich (Poole, UK). 5-Flouro 2’Deoxyuridine was from VWR; 5-Methyluridine was from Alfa Aesar; 2’,3’-dideoxyuridine was from Carbosynth; Pyrimethamine was from Fluka; Ara-A was from ICN Biomedicals.
The in vitro inhibitory effects of antimicrobial nucleoside agents on T. gondii growth were determined in HFF cell cultures using 96-well black plates (Optical-Bottom Plates with Polymer Base, Thermo Fisher Scientific). 150 – 200 µL of HFF cells were seeded into 96-well black plates and incubated for 3-5 days at 37°C under 5% CO2 in a humidified incubator. Once the HFF cells became confluent the supernatant was discarded and replaced with 100 µL fresh DMEMc media. Sulfadiazine (positive control) and the nucleoside compounds were already dissolved in DMSO at known concentrations, usually prepared as 10 mM. After that, every compound was diluted in DMEMc at 4x final concentration. The assays were performed in duplicate or triplicate throughout. 100 µL of 4x compounds were transferred to the wells of the first column of a 96-well black plate of which each well contained HFF cells in 100 µL DMEMc. The compounds were mixed, and 100 µL was transferred using the multichannel pipette to the next column before being transferred to each column through the entire plate over one row, leaving the last column as a negative control without drug. After that, naturally, freshly egressed parasites were collected and filtered through a 3-µm filter (Sigma-Aldrich). ~1000 parasites/well were added in 100 µL DMEMc (giving a total volume of 200 µL/well) and incubated for 6 days at a 37°C/5% CO2 in a humidified incubator. On day 6, a PHERAstar plate reader (BMG Labtech, Germany) was used to read the fluorescence intensity at 540 nm for excitation and 590 nm for emission. The EC50 values and fluorescence data were determined and plotted by using Prism 8.0 (GraphPad). Each experiment was independently performed 3-5 times.
HFF cytotoxicity assay was done exactly as described above in section 2.4, except that no parasites were added during this assay. Phenyl Arsine Oxide (PAO) was always used as a positive control. The plates were incubated at 37°C under 5% CO2 in a humidified incubator for 6 days to be consistent with the drug screening assay against T. gondii. On day 6, 10 µL of a 12.5 mg/100 mL ddH2O resazurin solution was added to each well. In addition, 200 µL of DMEMc media was placed in one row in a new 96-well plate without any host cells to determine the level of background fluorescence for the calculation, and 10 µL of a 12.5 mg/100 mL ddH2O resazurin solution was added to those wells as well. The plates were incubated for 3-4 h at 37°C and 5% CO2 in a humidified incubator. Subsequently, fluorescence was read using the PHERAstar plate, at λexc 540 nm and λem 590 nm. The data was plotted using an equation for a sigmoid curve with variable slope (4 parameter) using Prism 8.0 (GraphPad); EC50 values were extrapolated if >50% was achieved at the highest test compound concentration. Each biological experiment was performed on 3-5 independent occasions. The background plate result was subtracted from the reading from the raw data result of the fluorescent intensity of the test compounds.
Transport of pyrimidine nucleosides/bases (uridine, uracil, thymidine and cytidine) into extracellular T. gondii tachyzoites was performed using a modified oil-stop technique previously described for transport measurements with T. gondii tachyzoites (De Koning et al., 2003), with only minor modification and optimization in the oil mixture. T. gondii tachyzoites were cultured in a confluent HFF T175 vented flask kept at 37°C and 5% CO2 in a humidified incubator until the tachyzoites parasites became extracellular and the extracellular tachyzoites culture suspension was collected and filtered through 47 mm diameter, 3 µm pore-size Nuclepore polycarbonate filters (Whatman International Ltd, UK) to remove the HFF debris, and centrifuged at 1500 x g for 15 min at 4°C. The assay buffer (AB; 33 mM HEPES, 98 mM NaCl, 4.6 mM KCl, 0.5 mM CaCl2, 0.07 mM MgSO4, 5.8 mM NaH2PO4, 0.03 mM MgCl2, 23 mM NaHCO3, 14 mM D-glucose, pH 7.3) was used to wash the pellets twice. Tachyzoites were counted using a Neubauer hemocytometer (Weber Scientific Ltd, UK) and resuspended in AB at a density of 2 × 108 cells/mL; the parasites suspension was allowed to recover from centrifugation stress for 30 min at room temperature (RT). Next, 100 µL of the AB containing radiolabeled nucleoside/nucleobase test compound at a known concentration, was layered over 200 µl of an oil mixture (1:5 mixture of mineral oil (Sigma) and di-n-butyl phthalate (BDH Ltd, UK) for tachyzoites cultures in a microfuge tube. Centrifugation was briefly applied to allow the aqueous layer with radiolabel and test compound to be layered perfectly on top of the oil. Transport assays, performed at RT, were started by adding 100 µL of the tachyzoite cell suspension in AB to the mixture of oil and radiolabel test compound, with a predetermined incubation time, taking care that the aqueous layers mixed and remained on top of the oil. Upon completion of incubation time, 750 µL of ice-cold stop solution was added to stop the transport assay or to saturate the transport. An ice-cold solution of unlabeled substrate was used as a stop solution using a concentration between 0.5 mM to 2 mM, depending on the saturation concentration of the transporter and the aqueous solubility of the compound. This step is immediately followed by centrifugation at 14800 × g for 1 min to pellet the cells under the oil layer. The microfuge tube was flash-frozen in liquid nitrogen, and the bottom of each tube, containing the cell pellet, was cut off and collected in 6 mL scintillation vials (LabLogic Systems Ltd, Sheffield, UK). The pellets were lysed using 300 µL of 2% (w/v) sodium dodecyl sulfate (SDS; 2% SDS in H2O) at RT for at least 40 min on a rocking platform (GENEO Biotech Products GmbH, Germany). To each vial a volume of 3 mL scintillation fluid (Optiphase Hisafe-2) was added, and the vials were left overnight in a dark area at RT. Vigorous shaking was done before the scintillation vials were measured using a Hidex 300 SL liquid scintillation counter (LabLogic Systems Ltd, Sheffield, UK). The assays were performed three times in independent experiments, each in triplicate.
24 C57BL/6J mice (The Jackson Laboratory) were infected intraperitoneally (i.p.) with 1000 Me.49.b7 T. gondii parasites diluted in 200 µl of 1×PBS. Body weights were determined prior to infection and once a day until euthanasia at day 10. Starting 72 h post infection, groups of 6 mice received daily drug treatment, spaced exactly 24 h, through day 9, or served as untreated control: 5-fluoro-2’deoxyuridine (5-F,2’dUrd; 25 mg/kg i.p., 200 µl in water), 5-fluorouracil (5-FU, 25 mg/kg i.p., 200 µl in water); Sulfadiazine [100 μg/L, oral in water as described (Saeij et al., 2005)]; control mice were injected with 200 µl water i.p. Following euthanasia by CO2 and cervical dislocation as per IUCUC guidelines, the lungs were dissected, removed and stored at -80°C until DNA extraction.
Daily animal health monitoring began before infection and lasted through the experiment. Mice were euthanized if predetermined welfare thresholds were exceeded. All animal experiments were carried out under University of Vermont Institutional Animal Care and Use Committee protocol PROTO202100038.
DNA was extracted using the Qiagen DNeasy kit using the manufacturer’s instructions and collected in 50 µl of elution buffer. DNA concentration was determined using a Nanodrop spectrophotometer (Thermo Fisher Scientific). Parasite burden in each lung tissue sample was determined using qPCR. 600 ng of each sample was used to normalize the relative burden within each organ and compared to a standard curve of isolated T. gondii parasites. SYBR green PCR Mastermix (Applied Biosystems) was used in a 20 µl volume, along with 500 nM of forward (5’-TCCCCTCTGCTGGCGAAAAGT-3’) and reverse (5’- AGCGTTCGTGGTCAACTATCG-3’) primer to the T. gondii B1 gene, as described (Vizcarra et al., 2023). The amplification program, ran on a Quant studio 5 thermocycler, was 50°C for 2 min, 95°C for 10 min then 40 cycles of 95°C 10 s, 60°C 30 s, followed by 60°C for 30 s and 95°C for 5 s.
Experiments were analyzed and reviewed using the Design and Analysis 2nd edition software (Thermo Fisher). Normalization and final calculations used Microsoft Excel. The standard curve was generated by serial dilution of Me49 parasite genomic DNA. Determination of parasite burden per sample was calculated as pg of parasite genomic DNA, based on the standard curve, per 600 ng of host lung tissue.
Sub-micromolar concentrations of radiolabeled pyrimidines were incubated over preset intervals with T. gondii tachyzoites and uptake was monitored. Uptake of 0.25 µM [3H]-thymidine over 10 min was barely detectable, although a line with a non-zero slope (P = 0.012, F-test) and non-significant deviation from linearity (P > 0.999, runs test) could be plotted (Figure 1A). However, this does not represent any mediated, high affinity uptake as the addition of 1 mM unlabeled thymidine did not significantly inhibit uptake over 10 min (P < 0.05, t-test, n=3). Likewise, 250 µM uridine did not inhibit the thymidine uptake and we conclude that if this does represent transporter-mediated thymidine uptake it must be very low affinity and not relevant at physiological concentrations. More likely, this represents non-specific accumulation of trace amounts of thymidine by a non-specific mechanism such as endocytosis.
Figure 1 Uptake of pyrimidines by T. gondii tachyzoites. Uptake of tritiated thymidine (A), cytidine (B), uridine (C) and uracil (D) was determined over the indicated time intervals. Details of the graphs are shown in the accompanying table. All experiments were performed in triplicate, error bars are SE and, when not shown, fall inside the symbol. Lines were calculated by linear regression in Prism 8.0; none of these lines was significantly non-linear by F-test (P >0.05). For each frame, the analysis of the linear regression is presented in the table.
Uptake of 0.2 µM [3H]-cytidine was > 4-fold faster than thymidine and while this still represents a slow rate (0.0029 pmol(107 cells)-1min-1), it was fully inhibited by the addition of either 1 mM unlabeled cytidine or 1 mM uridine (Figure 1B), showing that cytidine was taken up by a saturable transport system.
Uptake of [3H]-uridine was quite robust and easily allowed quantification at 0.1 µM over 5 min, with a rate of 0.010 pmol(107 cells)-1min-1 (Figure 1C). This uptake appeared to be somewhat inhibited by 250 µM thymidine (P > 0.05), 80% by 250 µM uracil and 90% by 1 mM unlabeled uridine (both P < 0.01); the level of inhibition by uracil was not significantly different from that by uridine (P > 0.05, unpaired t-test).
Uptake of 0.1 µM [3H]-uracil was even more robust, at a rate of ~2.5-fold that of uridine (0.026 pmol(107 cells)-1min-1), and it was fully saturable, with uptake in the presence of 1 mM unlabeled uracil being not significantly different from zero (P = 0.37, F-test) (Figure 1D). We conclude that T. gondii tachyzoites do not express any thymidine transporters but do express one or more carriers for uracil and/or uridine and appear to have a minor capacity to take up cytidine. Since the cytidine uptake was fully inhibited by uridine, our data would be consistent with a model of a single transporter with the following substrate preference: uracil > uridine > cytidine >> thymidine.
Uridine and uracil were both taken up with high affinity by tachyzoites. 0.1 µM [3H]-uridine was dose-dependently inhibited by unlabeled uridine in a mono-phasic way (Figure 2A), with an average Km of 3.34 ± 0.82 µM and Vmax of 1.21 ± 0.24 pmol(107 cells)-1min-1 (n=3). Uridine transport was also inhibited by uracil, with an average Ki of 1.15 ± 0.07 µM (n= 3; not significantly different from the uridine Km by t-test), and by 5-fluorouracil (5-FU) with a Ki of 5.24 ± 0.72 µM (n=3; P < 0.01 from uracil). These data are consistent with a model of a single uridine/uracil transporter that perhaps slightly favors uracil over uridine but is essentially a dual uridine/uracil transporter. Consistent with this model we found very similar parameters when using [3H]-uracil as the substrate (all n=3): Km 2.05 ± 0.40 µM, Ki (uridine) 2.4 ± 0.59 µM, Ki (5-FU) 6.80 ± 2.12 µM (Figure 2B). None of these values was significantly different from the equivalent values using [3H]-uridine, which confirms that the tachyzoites express a uridine/uracil transporter, which we designate TgUU1.
Figure 2 Kinetic parameters for the transport of uridine (A) and uracil (B) in T. gondii tachyzoites. Both frames depict a single representative experiment in triplicate, of dose-dependent inhibition of either 0.1 µM [3H]-uridine (frame A) or 0.1 µM [3H]-uracil (Frame B). Error bars are SEM. Insets are the conversion of the inhibition of the inhibition of the uptake of radiolabeled permeant with unlabeled permeant to a Michaelis-Menten saturation plot. Hill slopes for the 6 plots varied between -1.2 and -0.86, consistent with a model for a single transporter.
Further characterization of TgUU1 was conducted using 0.1 µM [3H]-uridine as the permeant. The transporter proved to be quite specific for pyrimidines, as inosine and adenosine, although able to inhibit uridine uptake, displayed much lower affinity than uracil, uridine and 5-FU (Figure 3A), with average Ki values of 28.2 ± 4.1 µM and 112 ± 6 µM, respectively (n=3). We next probed the reason for the high apparent selectivity for uridine over thymidine, and determined a Ki of 1010 ± 145 µM for thymidine, which corresponds to a change from -31.2 kJ/mol to -17.1 kJ/mol in Gibbs free energy of binding (δ(ΔG0) = 14.1 kJ/mol; Table 1). This is attributable to both differences between uridine and thymidine, being the presence of a 2’-OH group in uridine and 5-methyl in thymidine, as 2’-deoxyuridine and 5-methyluridine both displayed low affinity for TgUUT1, with average Ki values of 2380 ± 770 µM and 134 ± 31 µM, respectively (Figure 3B). This shows that the 5-methyl group very strongly interferes with the binding of the pyrimidine substrates, and that the 2’-OH group contributes positively to the binding of uridine, likely through a hydrogen bond (δ(ΔG0) = 9.0 kJ/mol compared with uridine). The kinetic parameters of TgUUT1 are summarized in Table 1.
Figure 3 Specificity of the TgUU1 transporter. Inhibition curves for the transport of 0.1 µM [3H]-uridine in tachyzoites by (A) purine nucleosides and (B) pyrimidine nucleosides. Graphs show results of a single experiment in triplicate, representative of several similar experiments. Error bars are SEM.
In the previous section we show that TgUUT1 recognizes 5F-pyrimidines as inhibitors, and likely, as substrates. As 5F-pyrimidines are thymidine analogues and are known cancer antimetabolites under the name Floxuridine (Power and Kemeny, 2009; Vodenkova et al., 2020) and have very significant activity against some protozoa (Ali et al., 2013; Alzahrani et al., 2017), we decided to test some of these compounds against intracellular tachyzoites in culture.
Most published protocols for in vitro drug screening against T. gondii use the MTT assay, which is a colorimetric assay that relies on tetrazolium salt and is used for accessing cell viability, cell proliferation and cytotoxicity for the host cells (Jin et al., 2012). Our protocol is an adaptation from a method that was used to evaluate antiretroviral compounds against T. gondii tachyzoites (Wang et al., 2019). These authors added 5×104 cells/mL freshly egressed tachyzoites to each well of a 96-well plate with an HFF cell monolayer, followed by incubation for 4 h to allow the cells to invade the host cells; the medium containing extracellular tachyzoites was then removed and fresh medium containing the desired concentration of the antiretroviral drugs was added to the wells and incubated for 5 days at 37°C and 5% CO2 in a humidified incubator (Wang et al., 2019). In our adaptation of this protocol, we aimed to eliminate the step of wash/removal of tachyzoites, in order to increase reproducibility. We thus prepared the serial dilutions of compounds in the 96-well plates containing the HFF monolayer cells and then added the lower number of 1×104 cells/mL freshly lysed tachyzoites to the 96-well plates, already containing both the HFF monolayer cells and tested compounds. The plates were then incubated for 6 days at 37°C and 5% CO2 in a humidified incubator. A major advantage that was introduced was detection of T. gondii in the wells, which was achieved by measuring fluorescence of the RFP-expressing parasites.
We tested our modified protocol using a set of known anti-toxoplasma compounds (sulfadiazine, pyrimethamine, Ara-A and NBMPR) against T. gondii in 2 – 4 biological repeats (Figure 4A), and the result was consistent in each repeat. Ara-A has been shown to have moderate activity against T. gondii (Pfefferkorn and Pfefferkorn, 1976). Our drug screening assay protocol was consistent with the previous reports as Ara-A showed modest activity against T. gondii with EC50 11.4 ± 1.8 µM (n=4). NBMPR has been shown to be very selectively toxic to T. gondii with EC50 values of 10.2 µM, without apparent cytotoxicity on uninfected HFF monolayer cells up to 100 µM (el Kouni et al., 1999). Our result was similar and reproducible, with EC50 of 3.19 ± 0.14 µM (n=4) and no apparent cytotoxicity for NPMPR on uninfected HFF cells at 100 µM. Both purine analogues displayed stronger activity than the current drugs sulfadiazine and pyrimethamine (Figure 4A; Table 2).
Figure 4 Effect of known anti-toxoplasma agents (A) and pyrimidine analogues (B) on intracellular tachyzoites and on uninfected HFF cells (C). Data were plotted to a log[Inhibitor] vs response sigmoid model with variable slope (A, B) or an equation for two-site competition (C) in Prism 9 to determine EC50 values. The curves are single experiments, representative of at least 2 (pyrimethamine) or 3 (all other compounds) independent, identically-performed experiments.
Table 2 In vitro EC50 values of 5-fluoropyrimidines and assorted anti-toxoplasma agents against T. gondii intracellular tachyzoites and HFF cells.
We next tested the 5-F-pyrimidines 5-FU, 5-FUrd and 5-F,2’dUrd for activity against T. gondii (Figure 4B). Table 2 shows that the three fluoropyrimidines displayed quite similar EC50 values, ranging from 0.45 µM for 5-FUrd to 1.13 µM 5-FU. As such, each of these compounds was at least an order of magnitude more active than sulfadiazine, which was included as a positive control. Other pyrimidine nucleosides tested, 3’-deoxythymidine, 2’,3’-dideoxyuridine (Figure 4B), 5’-deoxyuridine and 2’-deoxyuridine, had no effect on intracellular tachyzoites at concentrations up to 25 µM. Next, the three 5-fluoropyrimidines were tested for effects on the uninfected HFF host cells, using the same incubation times and conditions as for the infected cells; Phenylarsine oxide (PAO) was used as the positive control for cytotoxicity. Using this prolonged resazurin-based protocol, the traces for all three 5-fluoropyrimidines were bi-phasic, with low concentration EC50 values for growth inhibition (cytostatic) and a second phase yielding (higher) cytocidal EC50 values (Figure 4C), much like we previously reported for some nucleoside analogues against T. brucei (Rodenko et al., 2007). Using the cytocidal EC50s, it was observed that the two clinically used anticancer drugs, 5-FU and 5-F,2’dUrd had a high selectivity (>2000) whereas 5-FUrd was much more toxic and as a result had a poor selecivity index of just 45 (Table 2).
Groups of 6 mice were infected with T. gondii by i.p. injection and treated with either 5-FU (25 mg/kg in water, p.i.), 5-F,2’dUrd (25 mg/kg in water, p.i.), sulfadiazine (100 μg/L in water, p.o.) or water (p.i.). The treatments were administered 72 h post infection (p.i.) and thence at 24 h intervals through day 9 p.i., with mice being euthanized with CO2 on day 10 p.i. Weight of each mouse was monitored throughout the experiment (Supplementary Figure S1). In the control group, weight decreased slightly over the 10-day period (11.1%, P = 0.03, unpaired t-test), and this was less pronounced in each of the treated groups, but none of them had an average weight that was significantly different at the end of the experiment (P > 0.1).
The parasite burden was assessed by qPCR, detecting T. gondii DNA – specifically the B1 gene (Lin et al., 2000) in lung tissue dissected from the euthanized mice. In all three treated groups, the parasite burden in lung tissue was >85% reduced compared to the untreated control mice (P < 0.001; Figure 5). 5-F,2’dUrd was significantly less effective, at the chosen dose and administration protocol, than sulfadiazine (P < 0.05) but 5-FU treatment was not significantly different from sulfadiazine (P > 0.05), but it should be noted that sulfadiazine was administered orally in water, at 40 mg/kg body weight, whereas the two pyrimidines were injected i.p. at 25 mg/kg BW.
Figure 5 Quantification of T. gondii DNA in lung tissue of infected mice, dissected after 10 days of infection with or without treatment, as indicated (n = 6, error bars are SD). *P < 0.05; **P < 0.01; ns, not significant by unpaired, two-tailed t-test.
The first line treatment against acute toxoplasmosis is a combination of pyrimethamine, an inhibitor of dihydrofolate reductase (DHFR), and an inhibitor of dihydropteroate synthetase (DHPS), usually sulfadiazine (Dunay et al., 2018). This combination is only active against acute toxoplasmosis and does not affect cysts in the chronic stage of toxoplasmosis. As pyrimethamine also inhibits human DHFR it is known for its hematological toxicity, making it necessary to give leucovorin or folinic acid together with the first-line treatment to combat the side effect (Alday and Doggett, 2017). Multiple additional disadvantages have been shown for this combination, such as poor tolerance among immunocompromised individuals, toxicity, and a prolonged course of treatment that causes the patients to discontinue taking the medicine, a recent surge in cost, unavailability in some countries, the lack of an intravenous formulation, agranulocytosis, Stevens-Johnson syndrome and hepatic necrosis (Alday and Doggett, 2017; Dunay et al., 2018). Identification of new inhibitors could help improve treatments in the long term.
The combination of the two dugs pyrimethamine and sulfadiazine disrupts the parasite’s folate synthesis and utilization (Alday and Doggett, 2017; Dunay et al., 2018). In turn, this results in the inhibition of the synthesis of nucleotide thymidine monophosphate from 2’-deoxyuridine monophosphate by Thymidylate Synthase, which utilizes methylene-tetrahydrofolate as its methyl donor. T. gondii is particularly vulnerable to drugs targeting this pathway because it, unusually, lacks a thymidine kinase activity (Fox et al., 2001) and is therefore unable to incorporate any salvaged thymidine into its nucleotide pool. However, T. gondii is known to be able to grow on extracellular uridine or uracil as its only pyrimidine source (Fox and Bzik, 2002; Fox and Bzik, 2010). It is further likely that cytidine can be salvaged by T. gondii and converted to uridine by cytidine deaminase (Iltzsch, 1993), if the parasite can acquire extracellular cytidine. These observations led us to hypothesize that T. gondii tachyzoites may have one or more carriers for uracil and uridine, and possibly for cytidine, but may not possess capacity to take up thymidine.
We tested the transport by tachyzoites of uracil, uridine, cytidine and thymidine. Only the accumulation of 0.25 µM [3H]-thymidine was, though just-measurable over 10 minutes, not saturable, in that it was not inhibited by a 4000-fold excess (1 mM) of unlabeled thymidine, or by 250 µM uridine. This clearly establishes that T. gondii tachyzoites do not express a thymidine-specific thymidine transporter, although it cannot be ruled out that they express a transporter for the uptake of a different substrate, that could also have some capacity to internalize thymidine, with very low affinity and efficiency. [3H]-Cytidine uptake was also quite slow but 100% inhibited by 1 mM unlabeled cytidine and by 250 µM uridine, revealing the expression of a cytidine transporter that is highly sensitive to inhibition by uridine. Uptake of [3H]-uridine was relatively robust and displayed similarly high affinity for uridine and for uracil as an inhibitor. The same was observed using [3H]-uracil as substrate and uridine as inhibitor, establishing reciprocal inhibition with statistically identical Km and Ki values. Moreover, uridine and uracil both inhibited [3H]-uridine and [3H]-uracil completely and with Hill slopes that were consistently very close to -1. These are all parameters indicating a single transporter for uridine and uracil and there really is no reasonable alternative explanation for all the data; we have designated this T. gondii pyrimidine carrier uridine/uracil transporter 1 (TgUUT1). It is likely that this carrier also has a minor capacity to take up cytidine, but this is likely to be of less relevance physiologically due to the very poor rate of uptake, the low availability of intracellular free cytidine and the fact that cytidine needs to be converted to uridine, and subsequently to uracil, by the parasite.
A high affinity uridine/uracil transporter was recently also described in Leishmania mexicana (LmexUUT1), but that transporter also had high affinity for adenosine (Alzahrani et al., 2017). This is not the case, however, for TgUUT1, as it was inhibited only ~70% by a 2500-fold excess of unlabeled adenosine (Figure 1C) and the adenosine Ki for uridine transport was determined at 112 µM. Nor is TgUUT1 similar to the uracil transporters of other protozoa, as the uracil transporters of T. brucei (De Koning and Jarvis, 1998; Gudin et al., 2006), L. major (Papageorgiou et al., 2005) and T. vaginalis (Natto et al., 2021b) are all highly specific for uracil and exclude uridine, which is taken up by separate transporters by those species (Ali et al., 2013; Natto et al., 2021b; Aldfer et al., 2022a).
The non-specificity of TgUUT1 regarding uracil and uridine would suggest that the ribose moiety of uridine is not engaged in interactions with the transporter but essentially a tolerated substitution on N1 of the pyrimidine ring – much like the TbAT1/P2 transporter of T. brucei, which has only a marginally higher affinity for adenine than adenosine although adenine is translocated with higher efficiency as seen from the Vmax/Km (1.84 vs 1.32; De Koning and Jarvis, 1999), making TbAT1 essentially an adenine transporter that tolerates adenosine. Recently, we reported that the T. gondii transporter Tg_244441 also transported both nucleobases and nucleosides with similar affinity, albeit in this case oxopurines rather than the aminopurines taken up by TbAT1/P2 (Campagnaro et al., 2022). However, in this case, the ribose moiety was not carried passively through the transporter; instead the similar affinity was in this case the result of a different set of interactions for nucleobases and nucleosides adding up to similar levels of binding energy.
For the TgUU1 transporter reported here, the so far limited evidence would suggest that the ribose moiety, and specifically the 2’-OH group, does contribute to the binding of uridine: 2’-deoxyuridine displayed a Ki value of 134 ± 31 µM, compared to the uridine Km of 3.34 ± 0.82 µM (P < 0.01) – a loss of 9.1 kJ/mol in Gibbs free energy of interaction (δ(ΔG0) = 9.1 kJ/mol). This observation goes some way to explain the very low affinity for thymidine, which also lacks 2’-OH and displays a Ki of 1010 ± 145 µM, corresponding to δ(ΔG0) of 14.2 kJ/mol. The greater loss of affinity for thymidine than for 2’-dUrd must be attributed to the 5-methyl group, which is the only difference between the molecules. Consistent with this interpretation 5-methyluridine displayed very low affinity and it can be concluded that the 5-methyl substitution is not compatible with pyrimidine nucleoside transport by TgUUT1. The much smaller 5-fluoro substitution, however, was much better tolerated as the δ(ΔG0) amounted to only 3.0 kJ/mol (5-FU Ki compared to uracil Km), allowing the uptake of 5F-pyrimidines.
5-F-pyrimidines have been mentioned as potential therapeutic agents before. As early as 1977, Pfefferkorn and Pfefferkorn (1977b) reported that 5-F,2’dUrd inhibited T. gondii growth in vitro and rapidly inhibited its nucleic acid synthesis. A 5-F,2’dUrd resistant cell line was created and found to lack UPRT activity (Pfefferkorn, 1978). Subsequently, it was found that 5-F-pyrimidines are good substrates for uridine phosphorylase (Iltzsch and Klenk, 1993). This observation, and our finding of equal transport of uridine and uracil, together explains why we found virtually equal activity of 5-FUrd, 5-F,2’dUrd and 5-FU, as the nucleosides are both converted to 5-FU by uridine phosphorylase inside the parasite, prior to activation to 5-FUMP by UPRT.
The in vitro activity of the 5-F-pyrimidines being between 11 and 27-fold stronger than first-line drug sulfadiazine, and no data on toxoplasmosis in an animal model having been reported for these compounds, we conducted a first trial in a standardized mouse model with 5-FU and 5-F,2’dUrd; the S.I. of 5FUrd was deemed too low. The parasite load was quantified with qPCR and compared to groups of mice treated with sulfadiazine or vehicle. The length of this initial infection was ten days, limiting the test to acute toxoplasmosis (Lin et al., 2000). While the differences in dosage regime between sulfadiazine (p.o., 100 mg/kg ×7) and the 5-F-pyrimidines (i.p., 25 mg/kg ×7) makes a genuine quantitative comparison of relative efficacy tenuous, all three treatments were similarly effective in reducing the parasite burden compared to the vehicle control. This invites further investigations into 5-FU, particularly, as an anti-toxoplasmosis agent, especially since it is already in long-time clinical use as an anti-cancer drug (Vodenkova et al., 2020). Interestingly, 5-FU has already been used in one study to treat AIDS patients with cerebral toxoplasmosis, in combination with clindamycin (Dhiver et al., 1993), a second-line treatment for toxoplasmosis (Konstantinovic et al., 2019). The brief report concluded that 5-FU/clindamycin is an effective and less toxic alternative to pyrimethamine/sulfadiazine, the toxicity of 5-FU being limited because ‘an effective anti-toxoplasma activity is obtained with doses tenfold less than those used for cancer chemotherapy.’.
In summary, we studied the uptake of pyrimidine nucleosides and uracil in T. gondii and report here a novel uridine/uracil carrier, TgUUT1, which also mediates the uptake of 5-fluoropyrimidine antimetabolites, but not of thymidine. This finding strongly contributes to our understanding of pyrimidine metabolism in T. gondii and explains the basis for the strong anti-toxoplasma activity of 5-F-pyrimidines. Further in vivo studies with 5-FU, in particular, seem warranted, as well as further work on the pharmacologically relevant T. gondii nucleoside transporters.
The raw data supporting the conclusions of this article will be made available by the authors, without undue reservation.
Ethical approval was not required for the studies on humans in accordance with the local legislation and institutional requirements because only commercially available established cell lines were used. The animal study was approved by University of Vermont Institutional Animal Care and Use Committee. The study was conducted in accordance with the local legislation and institutional requirements.
HE: Formal Analysis, Funding acquisition, Investigation, Methodology, Writing – original draft. AG: Investigation, Writing – original draft. BM: Formal Analysis, Methodology, Resources, Supervision, Writing – original draft. LS: Methodology, Supervision, Writing – review & editing. HdK: Conceptualization, Formal Analysis, Methodology, Project administration, Resources, Supervision, Writing – original draft, Writing – review & editing.
The author(s) declare financial support was received for the research, authorship, and/or publication of this article. HE was supported by a studentship from the government of Libya. This work was supported by the Welccome Trust as part of the core funding for the Wellcome Centre for Integrative Parasitology [grant number 104111] Neither funder had influence on the topic or the conduct of the research.
We thank Mr Andrew Boyd for expert assistance with the cell cultures.
The authors declare that the research was conducted in the absence of any commercial or financial relationships that could be construed as a potential conflict of interest.
The author(s) declared that they were an editorial board member of Frontiers, at the time of submission. This had no impact on the peer review process and the final decision.
All claims expressed in this article are solely those of the authors and do not necessarily represent those of their affiliated organizations, or those of the publisher, the editors and the reviewers. Any product that may be evaluated in this article, or claim that may be made by its manufacturer, is not guaranteed or endorsed by the publisher.
The Supplementary Material for this article can be found online at: https://www.frontiersin.org/articles/10.3389/fcimb.2023.1320160/full#supplementary-material
Alday, P. H., Doggett, J. S. (2017). Drugs in development for toxoplasmosis: advances, challenges, and current status. Drug Des. Dev. Ther. 11, 273. doi: 10.2147/DDDT.S60973
Aldfer, M., Alfayez, I. A., Elati, H. A. A., Gayen, N., Elmahallawy, E. K., Murillo, A. M., et al. (2022a). The Trypanosoma cruzi nucleoside transporter TcrNT2 is a conduit for the uptake of 5-F-2’deoxyuridine and tubercidin analogues. Molecules 27, 8045. doi: 10.3390/molecules27228045
Aldfer, M. M., AlSiari, T. A., Elati, H. A. A., Natto, M. J., Alfayez, I. A., Campagnaro, G. D., et al. (2022b). Nucleoside transport and nucleobase uptake null mutants in Leishmania mexicana for the routine expression and characterisation of purine and pyrimidine transporters. Int. J. Mol. Sci. 23, 8139. doi: 10.3390/ijms23158139
Ali, J. A. M., Creek, D. J., Burgess, K., Allison, H. C., Field, M. C., Mäser, P., et al. (2013). Pyrimidine salvage in Trypanosoma brucei bloodstream forms and the trypanocidal action of halogenated pyrimidines. Mol. Pharmacol. 83, 439–453. doi: 10.1124/mol.112.082321
Al Safarjalani, O. N., Rais, R. H., Kim, Y. A., Chu, C. K., Naguib, F. N., El Kouni, M. H. (2010). Carbocyclic 6-benzylthioinosine analogues as subversive substrates of Toxoplasma gondii adenosine kinase: Biological activities and selective toxicities. Biochem. Pharmacol. 80, 955–963. doi: 10.1016/j.bcp.2010.06.001
Al-Salabi, M. I., De Koning, H. P. (2005). Purine nucleobase transport in amastigotes of Leishmania mexicana: involvement in allopurinol uptake. Antimicrob. Agents Chemother. 49, 3682–3689. doi: 10.1128/AAC.49.9.3682-3689.2005
Al-Salabi, M. I., Wallace, L. J. M., De Koning, H. P.. (2003). A Leishmania major nucleobase transporter responsible for allopurinol uptake is a functional homologue of the Trypanosoma brucei H2 transporter. Mol. Pharmacol 63, 814–820. doi: 10.1124/mol.63.4.814
Alzahrani, K. J. H., Ali, J. A. M., Eze, A. A., Looi, W. L., Tagoe, D. N. A., Creek, D. J., et al. (2017). Functional and genetic evidence that nucleoside transport is highly conserved in Leishmania species: implications for pyrimidine-based chemotherapy.’. Int. J. Parasitol. Drugs Drug Resist. 7, 206–226. doi: 10.1016/j.ijpddr.2017.04.003
Berens, R. L., Krug, E. C., Marr, J. J. (1995). “Purine and pyrimidine metabolism,” in Biochemistry and molecular biology of parasites. Eds. Joseph, J., Marr, Miklos, M. (London, UK: Academic Press), 89–118.
Campagnaro, G. D., De Koning, H. P. (2020). Purine and pyrimidine transporters of pathogenic protozoa – conduits for therapeutic agents. Med. Res. Rev. 40, 1679–1714. doi: 10.1002/med.21667
Campagnaro, G. D., Elati, H. A. A., Balaska, S., Martin Abril, M. E., Natto, M. J., Hulpia, F., et al. (2022). A Toxoplasma gondii oxopurine transporter binds nucleobases and nucleosides using different binding modes. Int. J. Mol. Sci. 23, 710. doi: 10.3390/ijms23020710
Chtanova, T., Schaeffer, M., Han, S. J., van Dooren, G. G., Nollmann, M., Herzmark, P., et al. (2008). Dynamics of neutrophil migration in lymph nodes during infection. Immunity 29(3), 487–496. doi: 10.1016/j.immuni.2008.07.012
Cheviet, T., Lefebvre-Tournier, I., Wein, S., Peyrottes, S. (2019). Plasmodium purine metabolism and its inhibition by nucleoside and nucleotide analogues. J. Med. Chem. 62, 8365–8391. doi: 10.1021/acs.jmedchem.9b00182
Chiang, C. W., Carter, N., Sullivan, W. J., Jr., Donald, R. G., Roos, D. S., Naguib, F. N., et al. (1999). The adenosine transporter of Toxoplasma gondii. Identification by insertional mutagenesis, cloning, and recombinant expression. J. Biol. Chem. 274, 35255–35261. doi: 10.1074/jbc.274.49.35255
De Koning, H. P., Al-Salabi, M. I., Cohen, A. M., Coombs, G. H., Wastling, J. M. (2003). Identification and characterisation of high affinity nucleoside and nucleobase transporters in Toxoplasma gondii. Int. J. Parasitol. 33, 821–831. doi: 10.1016/s0020-7519(03)00091-2
De Koning, H. P., Jarvis, S. M. (1998). A highly selective, high-affinity transporter for uracil in Trypanosoma brucei brucei: evidence for proton-dependent transport. Biochem. Cell Biol. 76, 853–858. doi: 10.1139/bcb-76-5-853
De Koning, H. P., Jarvis, S. M. (1999). ‘Adenosine transporters in bloodstream forms of T. b. brucei: Substrate recognition motifs and affinity for trypanocidal drugs. Mol. Pharmacol. 56, 1162–1170. doi: 10.1124/mol.56.6.1162
Deniskin, R., Frame, I. J., Sosa, Y., Akabas, M. H. (2015). Targeting the Plasmodium vivax equilibrative nucleoside transporter 1 (PvENT1) for antimalarial drug development. Int. J. Parasitol. Drugs Drug Resist. 6, 1–11. doi: 10.1016/j.ijpddr.2015.11.003
Dhiver, C., Milandre, C., Poizot-Martin, I., Drogoul, M. P., Gastaut, J. L., Gastuat, J. A. (1993). 5-Fluoro-uracil-clindamycin for treatment of cerebral toxoplasmosis. Aids 7, 143–144. doi: 10.1097/00002030-199301000-00034
Dunay, I. R., Gajurel, K., Dhakal, R., Liesenfeld, O., Montoya, J. G. (2018). Treatment of toxoplasmosis: historical perspective, animal models, and current clinical practice. Clin. Microbiol. Rev. 31, e00057–e00017. doi: 10.1128/CMR.00057-17
el Kouni, M. H., Guarcello, V., Al Safarjalani, O. N., Naguib, F. N.. (1999). Metabolism and selective toxicity of 6-nitrobenzylthioinosine in Toxoplasma gondii. Antimicrob. Agents Chemother 43(10), 2437–2443. doi: 10.1128/AAC.43.10.2437
Finley, R. W., Cooney, D. A., Dvorak, J. A. (1988). Nucleoside uptake in Trypanosoma cruzi: analysis of a mutant resistant to tubercidin. Mol. Biochem. Parasitol. 31, 133–140. doi: 10.1016/0166-6851(88)90164-8
Fiuza, L. F. A., Batista, D. G., Girão, R. D., Hulpia, F., Finamore-Araújo, P., Aldfer, M. M., et al. (2022). Phenotypic evaluation of nucleoside analogues against Trypanosoma cruzi infection: In vitro and in vivo approaches. Molecules 27, 8087. doi: 10.3390/molecules27228087
Fox, B. A., Belperron, A. A., Bzik, D. J. (2001). Negative selection of herpes simplex virus thymidine kinase in Toxoplasma gondii.’. Mol. Biochem. Parasitol. 116, 85–88. doi: 10.1016/S0166-6851(01)00302-4
Fox, B. A., Bzik, D. J. (2002). De novo pyrimidine biosynthesis is required for virulence of Toxoplasma gondii. Nature 415, 926–929. doi: 10.1038/415926a
Fox, B. A., Bzik, D. J. (2010). Avirulent uracil auxotrophs based on disruption of orotidine-5′-monophosphate decarboxylase elicit protective immunity to Toxoplasma gondii. Infect. Immun. 78, 3744–3752. doi: 10.1128/IAI.00287-10
Fox, B. A., Bzik, D. J. (2020). “Biochemistry and metabolism of Toxoplasma gondii: purine and pyrimidine acquisition in Toxoplasma gondii and other Apicomplexa,” in Toxoplasma gondii. Eds. Weiss, L. M., Kim, K. (San Diego: Elsevier Academic Press).
Ghosh, M., Mukherjee, T. (2000). Stage-specific development of a novel adenosine transporter in Leishmania donovani amastigotes. Mol. Biochem. Parasitol. 108, 93–99. doi: 10.1016/s0166-6851(00)00208-5
Gudin, S., Quashie, N. B., Candlish, D., Al-Salabi, M. I., Jarvis, S. M., Ranford-Cartwright, L. C., et al. (2006). Trypanosoma brucei: A survey of pyrimidine transport activities. Exp. Parasitol. 114, 103–108. doi: 10.1016/j.exppara.2006.02.018
Gutteridge, W. E., Trigg, P. I. (1970). Incorporation of radioactive precursors into DNA and RNA of Plasmodium knowlesi in vitro. J. Protozool. 17, 89–96. doi: 10.1111/j.1550-7408.1970.tb05163.x
Hassan, H. F., Coombs, G. H. (1988). Purine and pyrimidine metabolism in parasitic protozoa. FEMS Microbiol. Lett. 54, 47–83. doi: 10.1111/j.1574-6968.1988.tb02708.x-i1
Heppler, L. N., Attarha, S., Persaud, R., Brown, J. I., Wang, P., Petrova, B., et al. (2022). The antimicrobial drug pyrimethamine inhibits STAT3 transcriptional activity by targeting the enzyme dihydrofolate reductase. J. Biol. Chem. 298, 101531. doi: 10.1016/j.jbc.2021.101531
Hill, D. E., Chirukandoth, S., Dubey, J. P. (2005). Biology and epidemiology of Toxoplasma gondii in man and animals. Anim. Health Res. Rev. 6, 41–61. doi: 10.1079/AHR2005100
Hulpia, F., Bouton, J., Campagnaro, G. D., Alfayez, I. A., Mabille, D., Maes, L., et al. (2020a). C6-O-Alkylated 7-deazainosine nucleoside analogues: Discovery of potent and selective anti-sleeping sickness agents. Eur. J. Med. Chem. 188, 112018. doi: 10.1016/j.ejmech.2019.112018
Hulpia, F., Campagnaro, G. D., Alzahrani, K. J., Alfayez, I. A., Ungogo, M. A., Mabille, D., et al. (2020b). Structure-activity relationship exploration of 3’-deoxy-7-deazapurine nucleoside analogues as anti-Trypanosoma brucei agents. ACS Infect. Dis. 6, 2045–2056. doi: 10.1021/acsinfecdis.0c00105
Iltzsch, M. H. (1993). Pyrimidine salvage pathways in Toxoplasma gondii. J. Euk. Microbiol. 40, 24–28. doi: 10.1111/j.1550-7408.1993.tb04877.x
Iltzsch, M. H., Klenk, E. E. (1993). Structure-activity relationship of nucleobase ligands of uridine phosphorylase from Toxoplasma gondii. Biochem. Pharmacol. 46, 1849–1858. doi: 10.1016/0006-2952(93)90592-K
Jin, C., Jung, S. Y., Kim, S. Y., Song, H. O., Park, H. (2012). Simple and efficient model systems of screening anti-Toxoplasma drugs in vitro. Expert Opin. Drug Disc. 7, 195–205. doi: 10.1517/17460441.2012.660479
Kim, Y. A., Rawal, R. K., Yoo, J., Sharon, A., Jha, A. K., Chu, C. K., et al. (2010). Structure–activity relationships of carbocyclic 6-benzylthioinosine analogues as subversive substrates of Toxoplasma gondii adenosine kinase. Bioorg. Med. Chem. 18, 3403–3412. doi: 10.1016/j.bmc.2010.04.003
Konstinovic, N., Guegan, H., Stäjner, T., Belaz, S., Robert-Gangneux, F. (2019). Treatment of toxoplasmosis: Current options and future perspectives. Food Waterborne. Parasitol. 15, e00036. doi: 10.1016/j.fawpar.2019.e00036
Lin, M. H., Chen, T. C., Kuo, T. T., Tseng, C. C., Tseng, C. P. (2000). Real-time PCR for quantitative detection of Toxoplasma gondii. J. Clin. Microbiol. 38, 4121–4125. doi: 10.1128/JCM.38.11.4121-4125.2000
Mabille, D., Ilbeigi, K., Hendrickx, S., Ungogo, M. A., Hulpia, F., Lin, C., et al. (2022). Nucleoside analogues for the treatment of animal trypanosomiasis. Int. J. Parasitol. Drugs Drug Resist. 19, 21–30. doi: 10.1016/j.ijpddr.2022.05.001
Montoya, J., Liesenfeld, O. (2004). Toxoplasmosis. Lancet 363, 1965–1976. doi: 10.1016/S0140-6736(04)16412-X
Natto, M. J., Hulpia, F., Kalkman, E. R., Baillie, S., Alhejeli, A., Miyamoto, Y., et al. (2021a). Deazapurine nucleoside analogues for the treatment of Trichomonas vaginalis. ACS Infect. Dis. 7, 1752–1764. doi: 10.1021/acsinfecdis.1c00075
Natto, M. J., Miyamoto, Y., Munday, J. C., AlSiari, T. A., Al-Salabi, M. I., Quashie, N. B., et al. (2021b). Comprehensive characterization of purine and pyrimidine transport activities in Trichomonas vaginalis and functional cloning of a trichomonad nucleoside transporter. Mol. Microbiol. 116, 1489–1511. doi: 10.1111/mmi.14840
Papageorgiou, I. G., Yakob, L., Al-Salabi, M. I., Diallinas, G., Soteriadou, K., De Koning, H. P. (2005). Identification of the first pyrimidine nucleobase transporter in Leishmania: similarities with the Trypanosoma brucei U1 transporter and antileishmanial activity of uracil analogues. Parasitology 130, 275–283. doi: 10.1017/S0031182004006626
Parker, M. D., Hyde, R. J., Yao, S. Y., McRobert, L., Cass, C. E., Young, J. D., et al. (2000). Identification of a nucleoside/nucleobase transporter from Plasmodium falciparum, a novel target for anti-malarial chemotherapy. Biochem. J. 349, 67–75. doi: 10.1042/bj3490067
Pfefferkorn, E. R. (1978). Toxoplasma gondii: the enzymic defect of a mutant resistant to 5-fluorodeoxyuridine.’. Exp. Parasitol. 44, 26–35. doi: 10.1016/0014-4894(78)90077-2
Pfefferkorn, E. (1988). “The biology of parasitism. A molecular and immunological approach,” in Toxoplasma gondii viewed from a virological perspective. Eds. Englund, P. T., Sher, A. (New York, USA: Alan R. Liss, Inc.), 479–501.
Pfefferkorn, E., Pfefferkorn, L. C. (1976). Arabinosyl nucleosides inhibit Toxoplasma gondii and allow the selection of resistant mutants. J. Parasitol. 62, 993–999. doi: 10.2307/3279197
Pfefferkorn, E. R., Pfefferkorn, L. C. (1977a). Specific labelling of intracellular Toxoplasma gondii with uracil. J. Protozool. 24, 449–453. doi: 10.1111/j.1550-7408.1977.tb04774.x
Pfefferkorn, E. R., Pfefferkorn, L. C. (1977b). Toxoplasma gondii: characterisation of a mutant resistant to 5-fluorodeoxyuridine. Exp. Parasitol. 42, 44–55. doi: 10.1016/0014-4894(77)90060-1
Polet, H., Barr, C. F. (1968). DNA, RNA, and protein synthesis in erythrocytic forms of Plasmodium knowlesi. Am. J. Trop. Med. Hyg. 17, 672–679. doi: 10.4269/ajtmh.1968.17.672
Power, D. G., Kemeny, N. E. (2009). The role of floxuridine in metastatic liver disease. Mol. Cancer Ther. 8, 1015–1025. doi: 10.1158/1535-7163.MCT-08-0709
Quashie, N. B., Dorin-Semblat, D., Bray, P. G., Biagini, G. A., Doerig, C., Ranford-Cartwright, L. C., et al. (2008). A comprehensive model of purine uptake by the malaria parasite Plasmodium falciparum: identification of four purine transport activities in intraerythrocytic parasites. Biochem. J. 411, 287–295. doi: 10.1042/BJ20071460
Rider, S. D., Jr., Zhu, G. (2010). Cryptosporidium: genomic and biochemical features.’. Exp. Parasitol. 124, 2–9. doi: 10.1016/j.exppara.2008.12.014
Rodenko, B., van der Burg, A. M., Wanner, M. J., Kaiser, M., Brun, R., Gould, M. K., et al. (2007). 2,N6-Disubstituted adenosine analogues with antitrypanosomal and antimalarial activity. Synthesis, uptake studies and in vivo evaluation. Antimicrob. Agents Chemother. 51, 3796–3802. doi: 10.1128/AAC.00425-07
Saeij, J. P., Boyle, J. P., Grigg, M. E., Arrizabalaga, G., Boothroyd, J. C. (2005). Bioluminescence imaging of Toxoplasma gondii infection in living mice reveals dramatic differences between strains. Infect. Immun. 73, 695–702. doi: 10.1128/IAI.73.2.695-702.2005
Schwab, J. C., Afifi, M. A., Pizzorno, G., Handschumacher, R. E., Joiner, K. A. (1995). Toxoplasma gondii tachyzoites possess an unusual plasma membrane adenosine transporter. Mol. Biochem. Parasitol. 70, 59–69. doi: 10.1016/0166-6851(95)00005-L
Sheiner, L., Demerly, J. L., Poulsen, N., Beatty, W. L., Lucas, O., Behnke, M. S., et al. (2011). A systematic screen to discover and analyze apicoplast proteins identifies a conserved and essential protein import factor. PloS Pathog. 7, e1002392. doi: 10.1371/journal.ppat.1002392
Tenter, A. M., Heckeroth, A. R., Weiss, L. M. (2000). Toxoplasma gondii: from animals to humans. Int. J. Parasitol. 30, 1217–1258. doi: 10.1016/S0020-7519(00)00124-7
Ungogo, M. A., Aldfer, M. M., Natto, M. J., Zhang, H., Chisholm, R., Walsh, K., et al. (2023). Cloning and characterisation of Trypanosoma congolense and T. vivax nucleoside transporters reveal the potential of P1-type carriers for the discovery of broad-spectrum nucleoside-based therapeutics against Animal African Trypanosomiasis. Int. J. Mol. Sci. 24, 3144. doi: 10.3390/ijms24043144
Vizcarra, E. A., Goerner, A. L., Ulu, A., Hong, D. D., Bergersen, K. V., Talavera, M. A., et al. (2023). An ex vivo model of Toxoplasma recrudescence reveals developmental plasticity of the bradyzoite stage. mBio. 14, e01836-23. doi: 10.1128/mbio.01836-23
Vodenkova, S., Buchler, T., Cervena, K., Veskrnova, V., Vodicka, P., Vymetalkova, V. (2020). 5-fluorouracil and other fluoropyrimidines in colorectal cancer: Past, present and future. Pharmacol. Ther. 206, 107447. doi: 10.1016/j.pharmthera.2019.107447
Keywords: uracil transporter, Toxoplasma gondi, uridine transport, pyrimidine salvage, 5-fluorouracil, antimetabolite, fluoropyrimidines, 5-fluorodeoxyuridine
Citation: Elati HAA, Goerner AL, Martorelli Di Genova B, Sheiner L and de Koning HP (2023) Pyrimidine salvage in Toxoplasma gondii as a target for new treatment. Front. Cell. Infect. Microbiol. 13:1320160. doi: 10.3389/fcimb.2023.1320160
Received: 11 October 2023; Accepted: 01 December 2023;
Published: 15 December 2023.
Edited by:
Debanjan Mukhopadhyay, Presidency University, IndiaReviewed by:
Martin Craig Taylor, University of London, United KingdomCopyright © 2023 Elati, Goerner, Martorelli Di Genova, Sheiner and de Koning. This is an open-access article distributed under the terms of the Creative Commons Attribution License (CC BY). The use, distribution or reproduction in other forums is permitted, provided the original author(s) and the copyright owner(s) are credited and that the original publication in this journal is cited, in accordance with accepted academic practice. No use, distribution or reproduction is permitted which does not comply with these terms.
*Correspondence: Harry P. de Koning, aGFycnkuZGUta29uaW5nQGdsYXNnb3cuYWMudWs=
Disclaimer: All claims expressed in this article are solely those of the authors and do not necessarily represent those of their affiliated organizations, or those of the publisher, the editors and the reviewers. Any product that may be evaluated in this article or claim that may be made by its manufacturer is not guaranteed or endorsed by the publisher.
Research integrity at Frontiers
Learn more about the work of our research integrity team to safeguard the quality of each article we publish.