- Departamento de Biología Molecular and Instituto de Biomedicina y Biotecnología de Cantabria (IBBTEC), Universidad de Cantabria- CSIC, Santander, Spain
Many pathogens use Type III and Type IV protein secretion systems to secrete virulence factors from the bacterial cytosol into host cells. These systems operate through a one-step mechanism. The secreted substrates (protein or nucleo-protein complexes in the case of Type IV conjugative systems) are guided to the base of the secretion channel, where they are directly delivered into the host cell in an ATP-dependent unfolded state. Despite the numerous disparities between these secretion systems, here we have focused on the structural and functional similarities between both systems. In particular, on the structural similarity shared by one of the main ATPases (EscN and VirD4 in Type III and Type IV secretion systems, respectively). Interestingly, these ATPases also exhibit a structural resemblance to F1-ATPases, which suggests a common mechanism for substrate secretion. The correlation between structure and function of essential components in both systems can provide significant insights into the molecular mechanisms involved. This approach is of great interest in the pursuit of identifying inhibitors that can effectively target these systems.
Introduction
Many Gram-negative pathogens encode virulence-associated Type III and Type IV secretion systems (T3SS and T4SS, respectively), which they use to translocate effector molecules. These systems are highly optimized multi-protein machineries that translocate effectors from the bacteria directly into the host cell cytoplasm, enabling effective manipulation of host processes (Galán and Waksman, 2018; Viana et al., 2021). T3SSs can be easily found in pathogenic bacteria such as Escherichia coli, Yersinia, Salmonella, Chlamydia or Pseudomonas (Cornelis, 2006; Coburn et al., 2007; Cornelis, 2010). T4SSs are associated to pathogens like Helicobacter, Legionella, Neisseria or Coxiella, among others (Cascales and Christie, 2003). Plant pathogens, such as Pseudomonas or Agrobacterium can also have T3- and T4SSs, respectively.
Apart from being involved in virulence, these secretion systems have other associated functions. For instance, flagellar systems, which have dynamic flagella to drive cellular motility, are related to T3SSs. In this case, the substrates translocated across the cell membranes are the components of the flagellum during biogenesis. The origin, specialization and diversification of these two different T3SS remain elusive, but they both share a high degree of homology, suggesting a common evolution from machines involved in motility (Gophna et al., 2003; Saier, 2004; Pallen et al., 2005; Abby and Rocha, 2012; Hu et al., 2017). As it happens in T3SSs, T4SSs are also versatile nanomachines, which are not only involved in virulence but also in DNA and nucleo-protein substrates delivery into bacterial or eukaryotic target cells, via a contact-dependent mechanism (Christie et al., 2014; Cabezón et al., 2015). They are ancestrally related to DNA conjugation machines involved in horizontal gene transfer and virulence (Guglielmini et al., 2013). In both cases, the architecture of these secretion systems is relatively well preserved regardless of its biological function.
T3 and T4SSs have several important features in common. They both span the inner membrane (IM), periplasm, and outer bacterial membrane (OM). Thus, the mechanism of transport involves just one step, which means that substrates are directly delivered into the host cell without the assistance of the Sec or Tat machineries (hence known as Sec- or Tat-independent protein secretion) (Christie, 2019). The internal diameter of the channel is similar in both secretion systems, less than 30 Å, which means that substrates must be unfolded to be secreted. Accordingly, both systems have specialized ATPases at the base of the secretion channel, which provide energy for substrate unfolding (T3SS) or pilus biogenesis and substrate transport (T4SS). Yet, many of the underlying molecular mechanisms that power secretion remain unclear.
The aim of this review is to provide a general overview of the two systems. Analyzing the structure-function relationship and comparing key elements shared by both systems can be extremely valuable in understanding the molecular mechanism and, more importantly, in the search of inhibitors that could target these systems.
Overview of a prototypical T3SS architecture
In recent years, cryo-electron microscopy advances have allowed the determination of the architecture of these two secretion systems revealing large, megadalton-sized macromolecular assemblies (Hu et al., 2018; Lunelli et al., 2020; Macé et al., 2022).
T3SSs, also known as injectiosomes for their syringe-shape, are large multiprotein complexes that span the inner and outer membrane of bacteria (Figure 1). Much of the structural knowledge about this secretion system is available from Salmonella and, therefore, we will use its nomenclature in this review accompanied of the unified nomenclature in parenthesis. A complex formed by eight membrane proteins spans the bacterial inner membrane (IM), periplasm and outer membrane (OM). The outer membrane protein InvG (SctC) belongs to the secretin family of β–barrel pores and it forms a ring with a 15-fold symmetry (Hu et al., 2018). In contrast, the IM ring, formed by SpaPQRS (SctRSTU) and PrgHK (SctDJ) proteins presents a 24-fold stoichiometry. Recent cryo-EM structure of the complex shed light on this symmetry mismatch puzzle between the OM and IM rings, revealing that the InvG ring incorporates an additional InvG monomer at the periplasmic site (Hu et al., 2019). This local variation in stoichiometry facilitates the interaction with the IM components.
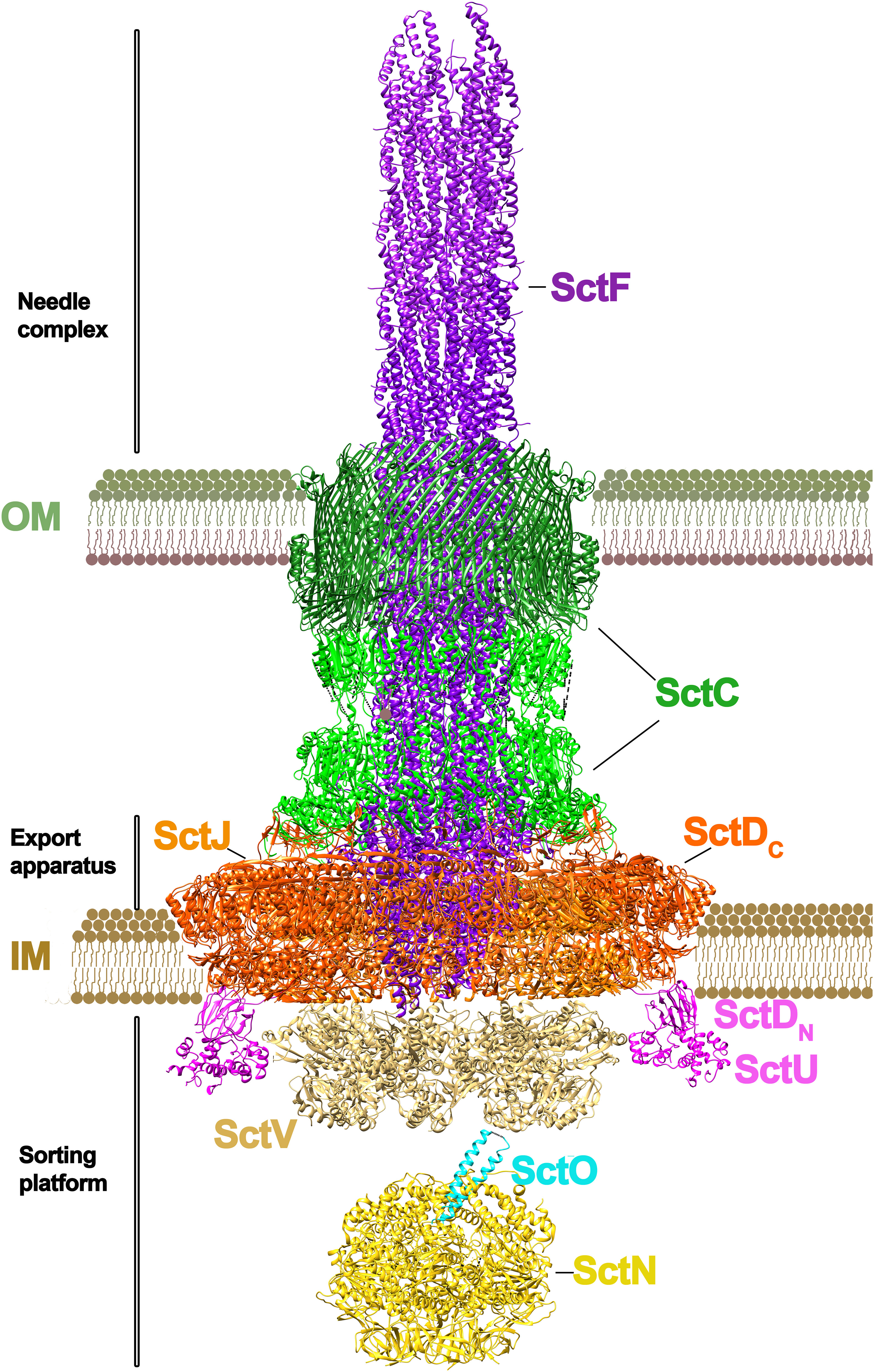
Figure 1 Architecture of a T3SS. The T3SS of Salmonella sp. is shown. The macromolecular assembly spans the inner (IM) and outer membranes (OM). Two distinct parts can be differentiated in the system: the needle complex and the sorting platform (Lara-Tejero and Galán, 2019; Soto et al., 2022). At the base of the needle complex there is a multi-ring structure that spans the inner membrane, formed by PrgH and PrgK subunits (SctD and SctJ subunits in the unified nomenclature). The IM base is connected to the outer membrane by an inner rod protein (PrgJ/SctI). At the outer membrane there is the secretin ring formed by InvG (SctC), where the needle filament protein (PgrI/SctF) is attached. The length of the ring is regulated by InvJ (SctP). The export apparatus (no shown) is formed by a number of small proteins (SpaP/SpaQ/SpaR in Salmonella; SctR/SctS/SctT in the unified nomenclature) connected to the C-ring (SpaO/SctQ) at the base of the channel by an export apparatus switch protein (SpaS/SctU). The export apparatus sorting complex is formed by a nonameric protein ring (InvA/SctV). The unfolding of the substrates is powered by a hexameric ATPase (InvC/SctN). In the lumen of this ATPase sits a stalk protein (InvI/SctO). (The figure has been created by using the PDB codes: 7ah9.pdb (Miletic et al., 2021), 7awa.pdb (Matthews-Palmer et al., 2021), 7k08.pdb (Majewski et al., 2019), 6uid.pdb and 6uie.pdb (Muthuramalingam et al., 2020).
Associated to this macromolecular complex is InvA (StcV), a transmembrane protein with a large globular domain located in the cytosol. The crystal structure of this globular domain (MxiA in Shigella fastidiosa) (Abrusci et al., 2013), as well as more recent tomography (Hu et al., 2017) and cryo-EM studies (Majewski et al., 2019; Kuhlen et al., 2021) confirm that this protein assembles as a nonameric complex. This InvA(SctV) protein, together with other small proteins such as OrgA(SctK), OrgB(SctL), SpaO(SctQ), InvI(SctO) and the ATPase InvC(SctN) form the sorting platform. The role of this platform, linked to the export apparatus, is to select effector proteins and target them for secretion. The hexameric ATPase, InvC (SctN) is involved in the unfolding of the effector proteins prior secretion (Akeda and Galán, 2005; Bergeron and Marlovits, 2022). The export apparatus SpaPQRS forms a right-handed pseudo-helical assembly, which works as a platform for PrgIJ (SctFI) needle polymerization. PrgJ (SctI) contacts with SpaPR and secretin InvG are required for needle assembly, thus providing the platform for a further polymerization of the PrgI (SctF) needle (Figure 1).
Overview of a prototypical T4SS architecture
T4SSs are also a heterogeneous group of nanomachines and can be classified in two main groups: conjugation systems and effector translocation systems (Sheedlo et al., 2022). Most simple T4SS consist of 12 proteins (named VirB1-VirB11 and VirD4 in the Agrobacterium tumefaciens unified nomenclature), also known as “minimized T4SSs” (Sheedlo et al., 2022) (Figure 2). Most recent T4SS structural data comes from the conjugative plasmid R388 (Macé et al., 2022), so this nomenclature will be used in this review, accompanied from the unified nomenclature in parenthesis. As it occurs in T3SSs, here it is also evident the existence of symmetry mismatches in the core complex. Whereas in T3SSs the OM ring is formed only by the secretin protein, three proteins are involved in the OM complex in T4SSs: TrwH (VirB7), TrwF (VirB9) and TrwE (VirB10), forming a ring with a barrel-shape architecture and a 14-fold symmetry. TrwH is completely embedded in the OM, but the N-terminal domains of TrwE and TrwF proteins also expand into the periplasmic space forming what is called the “I-layer”, differentiated from the embedded part in the OM, called “O-layer”. Intriguingly, the periplasmic N-terminal domains of these two proteins (the I-layer) form a 16-fold complex, which means that the C-terminal domains of these two heterodimers are not inserted in the O-layer. Similar symmetry mismatches have also been reported in protein translocating T4SSs, such as the C17:C14 mismatch found in H. pylori (Chung et al., 2019; Sheedlo et al., 2020) or a C18:C13 mismatch in L. pneumophila (Durie et al., 2020).
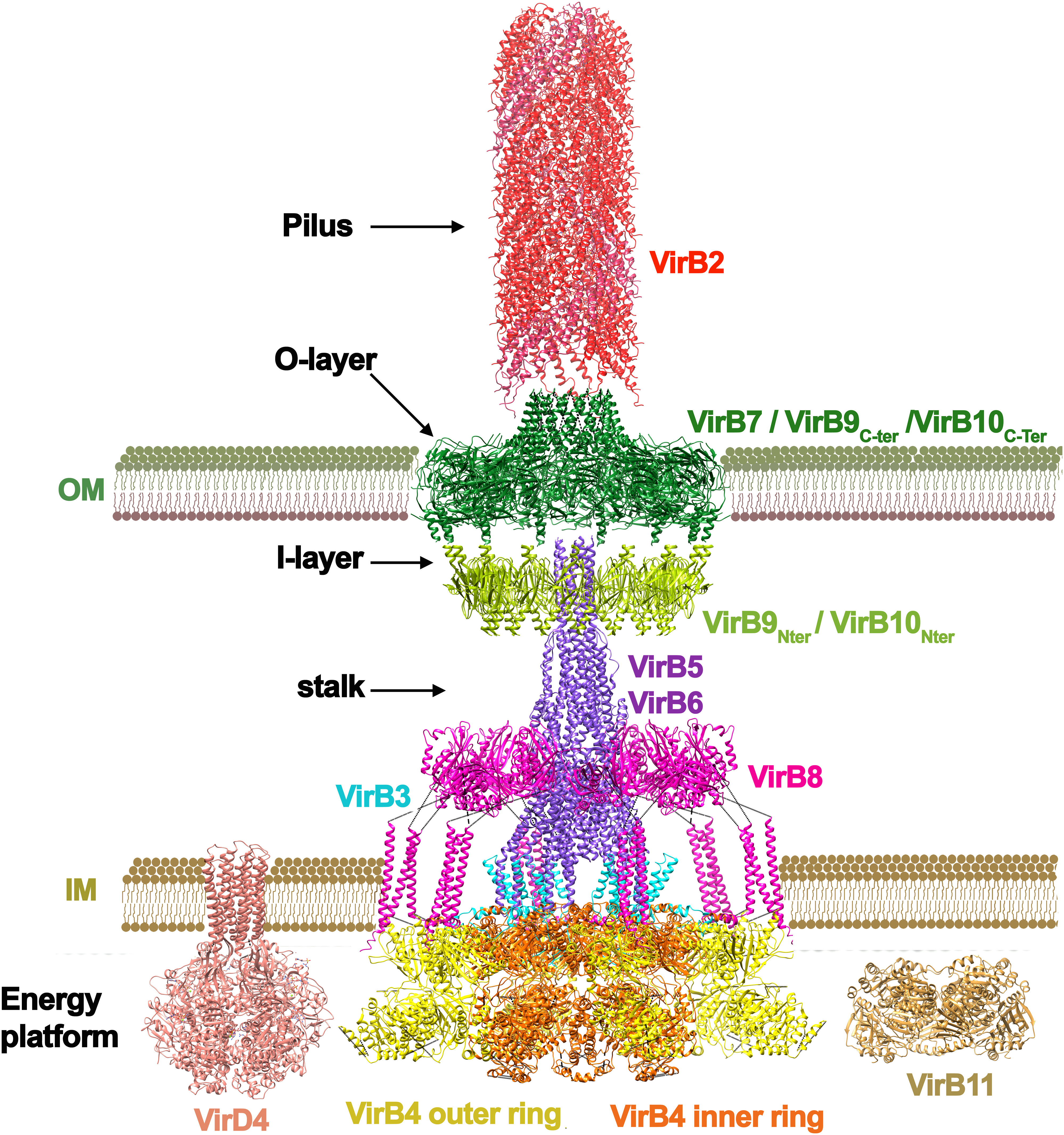
Figure 2 Architecture of a T4SS. The T4SS of the conjugative plasmid R388 is shown (Macé et al., 2022) from PDB codes: 7o3.pdb, 7o3j.pdb, 7o3v.pdb, 7q1v.pdb, 7o43.pdb, and 7oiu.pdb. The subunits are named following the Agrobacterium tumefaciens nomenclature. The system is formed by 11 different proteins (named VirB1 to VirB11). The main ATPase of the system is VirB4 (TrwK in R388), which forms a hexamer of dimers at the base of the channel. This ATPase is thought to power pilus formation but also substrate secretion. Conjugative systems have an additional ATPase, named coupling protein (Cabezon and de la Cruz, 2006), which is not present in the cryo-EM structure (pdb 1e9r.pdb taken from (Gomis-Rüth et al., 2001). Most T4SS also have another ATPase named VirB11 (TrwD in R388) suggested to act in the unfolding of the substrate (pdb obtained by molecular modelling as shown in (Ripoll-Rozada et al., 2013). VirB4 (TrwK) is attached to the inner membrane by VirB3 (TrwM), an integral membrane protein. The inner membrane platform also contains the N-terminal domain of VirB8 (TrwG), a protein that spans towards the periplasmic space forming an arched shaped structure. The periplasmic space protein (VirB6) forms a stalk structure that connects the inner membrane complex with the outer membrane ring formed by VirB7 (TrwH in R388), VirB9 (TrwF) and VirB10 (TrwE). Conjugative systems contain a pilus attached to the outer membrane formed by an oligomeric helical structure of pilin subunits (VirB2/TrwL). This pilus structure has variable length depending on the system. It is important to note that the pilus structure shown in red corresponds to that of F plasmid (pdb 5leg.pdb from (Costa et al., 2016), solved independently of the secretion system. As mentioned in the main text, the solved T4SS structure shown here might correspond to a state involved in pilus biogenesis (Macé et al., 2022), which would explain why VirB5 appears in the periplasmic space being a specific adhesin protein expected to be localized at the tip of the pilus (Aly and Baron, 2007).
In T3SSs, this local variation in stoichiometry is thought to facilitate the interaction with the IM components. However, in T4SSs, the OMC complex does not contact directly with the IM complex. Instead, a structure called “stalk” bridges the core of the OM and the IM complexes. This central, cone-shaped structure is composed of a pentamer of VirB6 (TrwI) inserted into the inner membrane, and a pentamer of VirB5 (TrwJ) mounted onto the VirB6 stalk base (Macé et al., 2022). The location of VirB5(TrwJ) has also been described to be at the T-pilus tip in the A. tumefaciens T4SS (Aly and Baron, 2007), which suggests that VirB5, also known as adhesin, changes position depending on the stage of the conjugation process. It is important to note that in the T4SS structure solved by cryo-EM (Macé et al., 2022) the pilus is absent. The pilus structure shown in Figure 2 corresponds to that of F plasmid (Costa et al., 2016), solved independently of the secretion system. In addition, it is also worth noting that in some T4SS, such as the Dot/IcM system from Legionella, an extended pilus has never been observed. Last findings suggest that, in this system, effector translocation occurs by close membrane contact (Böck et al., 2021).
In the cryo-EM structure, the stalk is surrounded by a ring complex, known as the arches, which is composed of hexamers of homotrimeric units of the periplasmic domain of VirB8 (TrwG). VirB8 protein contacts one of the main components of the IM complex: the VirB4 ATPase (TrwK). VirB4 forms a hexamer of dimers. One of the subunits of each dimer is involved in the formation of a central hexamer, attached to the inner membrane through VirB3 (TrwM). The second subunit of the dimers protrudes out, and it makes contacts with the tails of three VirB8 subunits. This oligomeric arrangement has only been found in VirB4 ATPases and it is still a controversial issue, since a previous work by electron microscopy showed this protein organized into 2 side-by-side hexameric barrels (Low et al., 2014).
It is worth noting that the described structure corresponds to a simple “minimized T4SS” (Costa et al., 2021). Examples of “expanded T4SSs” include the H. pylori Cag T4SS (Cover et al., 2020), the L. pneumophila Dot/Icm (Chetrit et al., 2018; Park et al., 2020) T4SS, and the F plasmid-encoded T4SS (Liu et al., 2022). Expanded systems have a larger size and require more proteins. In addition, none of the T4SS structures solved so far reveals a continuous central channel. OM and IM complexes are only connected by the projecting pilus structure and, therefore, the solved structures might correspond to a state involved in pilus biogenesis (Macé et al., 2022), but not in DNA-protein transfer; a state in which VirD4 (TrwB) ATPase would also be required. In addition, TrwD, a traffic ATPase related to the T2SS EpsE/PilB/PilT proteins is also missing in this cryo-EM structure.
Energy supply for substrate transport
Translocation of proteins and protein-DNA complexes across membranes is a process that requires energy. T3SS and T4SS rely on several associated ATPases and/or the proton motive force (PMF) to drive translocation (Christie, 2019). Nonetheless, the details of the secretion mechanism are still unclear.
The limited diameter of the T3SS needle channel (~ 2 nm) (Lyons et al., 2021) reflects that protein effector unfolding must occur for substrate translocation, unless the channel opens dramatically during translocation, which would compromise cell viability. In this sense, many T3SS effectors interact with chaperones, which maintain these substrates in an unfolded and secretion-competent state prior to translocation (Stebbins and Galán, 2001; Lee and Galán, 2004; Akeda and Galán, 2005). The T3SS hexameric ATPase InvC (SctN), at the base of the channel, would mediate T3SS effectors unfolding before secretion (Deng et al., 2017). It is thought that ATP hydrolysis is required for the initial step of substrate secretion, but additional force is provided by the PMF (Minamino et al., 2008) via the proton channel SctV (Minamino et al., 2011). Therefore, T3SS depends on ATP and PMF energy sources for efficient secretion (Wilharm et al., 2004; Erhardt et al., 2014; Halte and Erhardt, 2021). Nevertheless, these issues are still highly controversial. There is a large distance (over 30 nm) between the ATPases within the cytosolic side and the cell wall (Matias et al., 2003) and this primary energy supply would be inefficient for substrate transfer (Grange et al., 2008). In that sense, folding of the secreted substrate upon exit from the secretion channel might also provide energy for pulling protein effectors through the apparatus (Lee and Rietsch, 2015).
T3SS likewise, the T4SS structure also reveals a central channel with a narrow internal diameter (~2 nm)(Macé et al., 2022). Thus, substrates must be unfolded in order to be transported. Three ATPases power T4SS mediated secretion: VirD4 (TrwB), VirB4 (TrwK) and VirB11 (TrwD). All three are essential for bacterial conjugation in the reference plasmid R388 (Figure 3). Of these, the coupling protein VirD4 (TrwB) serves as the receptor to which both, DNA and protein substrates, bind before entry into the translocation channel (Cabezon and de la Cruz, 2006; Álvarez-Rodríguez et al., 2020; Costa et al., 2021). In conjugative systems, the substrate is a nucleoprotein formed by a protein called relaxase (TrwC in the case of R388 plasmid) and the conjugative DNA. This nucleoprotein substrate, together with some assistant proteins, form a complex known as the relaxosome (De La Cruz et al., 2010). Prior to translocation, the relaxase protein must be unfolded (Trokter and Waksman, 2018). The most structurally related protein to T3SS unfoldase InvC (SctN) is VirD4 (TrwB) (Figure 4). However, TrwB is a DNA-dependent ATPase (Tato et al., 2005) and, although it is directly involved in protein-DNA secretion (Llosa et al., 2002), an unfoldase role has never been assigned to this protein. In T4SS, VirB11 ATPase (TrwD) could participate in the unfolding of protein substrates (Cabezón et al., 2015). VirB11 belongs to the family of traffic ATPases (Planet et al., 2001) and shares a structural similarity to ATPases from other secretion systems, such as PilB or PilT from T4PS or GspE from T2SS (Peña and Arechaga, 2013).However, the mechanism and the forces that push and unfold substrates through the channel remain to be characterized. As in T3SS, an important aspect to be considered in powering the transport of the nucleoprotein complex is the contribution of the relaxase unfolding-refolding process. TrwC must be refolded in the recipient cell in order to re-circularize the ssDNA plasmid strand. Thus, a fast refolding of TrwC might also play an important role as a pulling force to complete the translocation process. Recent experiments, in which the co-translocational unfolding of a relaxase-DNA complex has been studied by nanopore technology, seem to point in that direction (Valenzuela-Gómez et al, 2023).
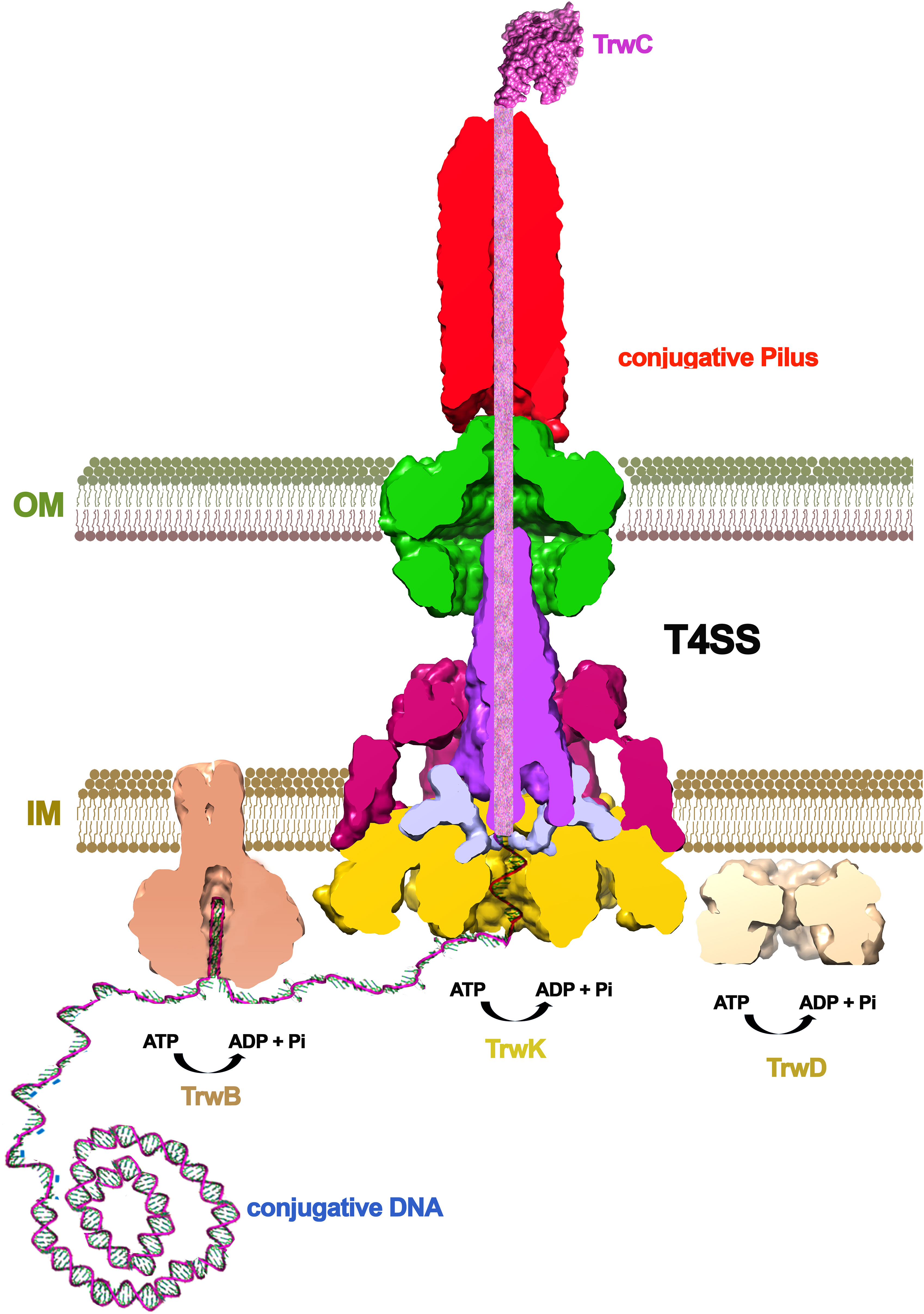
Figure 3 The conjugative mechanism. Bacterial conjugation is triggered by a signal (probably the contact with a recipient cell), which induces the binding of the relaxase protein (TrwC in R388) to the conjugative plasmid. After nicking the DNA, the relaxase remains covalently bound to the DNA, which is unwinded by the helicase domain within the same protein. DNA processing is helped by the coupling protein (VirD4/TrwB), which presents the nucleo-protein substrate to the base of the channel. TrwC relaxase is a huge protein of 966 amino acid residues (1,756 in the case of F plasmid) that has to be unfolded to cross the inner and outer membranes of both, the donor and the recipient cell. This unfolding is thought to be powered by VirB11 (TrwD), an ATPase related to T2SS proteins PilB/PilT/GspE.
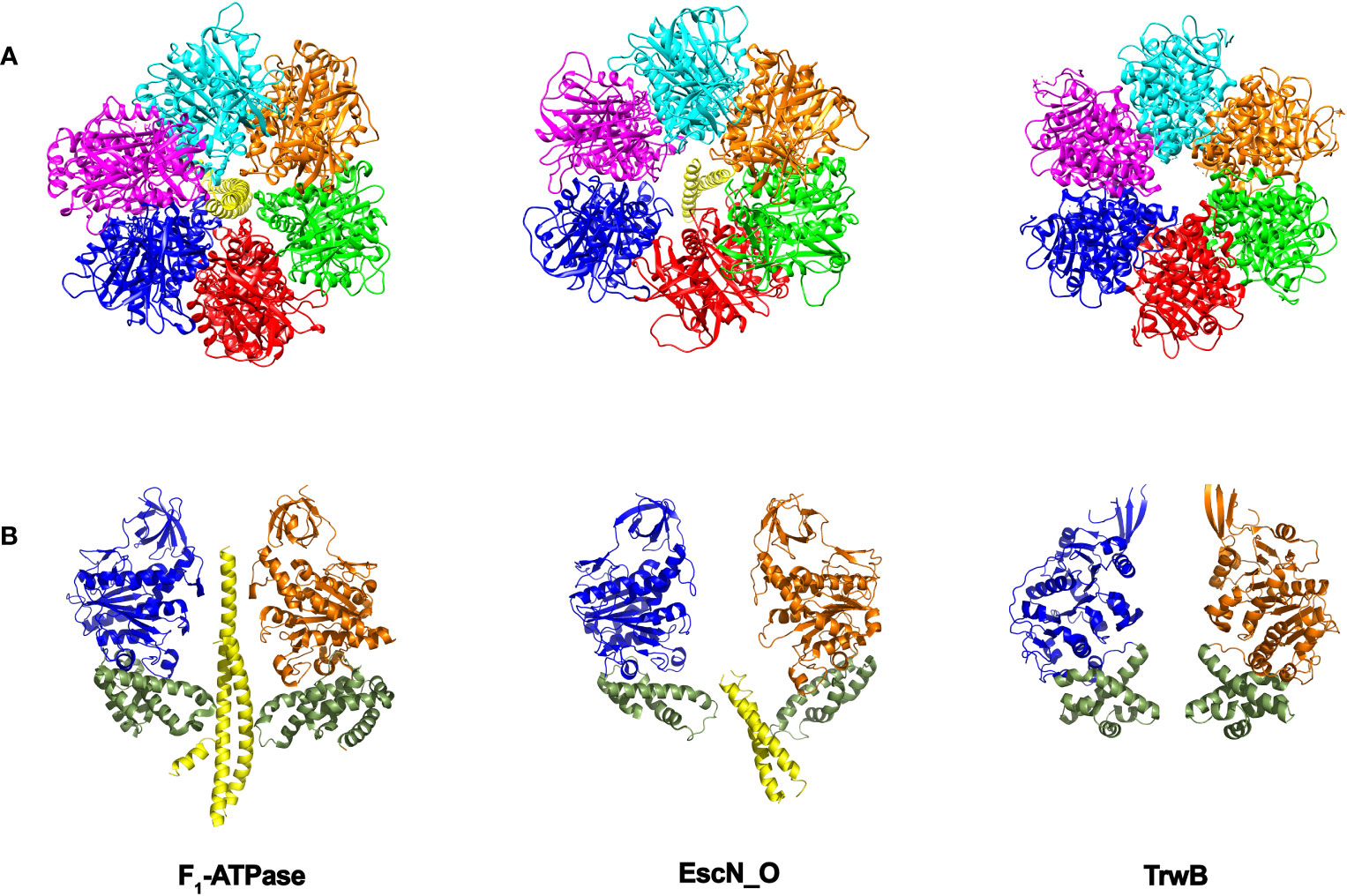
Figure 4 Structural similarities between secretion ATPases and F1-ATPase. As the structures of the secretion ATPases were unveiled, a striking similarity with the F1-ATPase structure (Abrahams et al., 1994) was emerging. The structure of the enteropathogenic E. coli T3SS ATPase EscN (Majewski et al., 2019), revealed an hexameric ring with a central stalk EscO that resembles the structure of the α/β ring with its central γ stalk in F1-ATPase. The structure of the T4SS coupling protein TrwB (Gomis-Rüth et al., 2001) also resembles F1-ATPase (Cabezon and de la Cruz, 2006). (A) Bottom view. Each subunit in the ring is shown in a different color. (B) Side view. For more clarity, only two opposite subunits of the ring are shown. The “all-α-domain” in TrwB is shown in green (residues 184–297). The equivalent domain in F1-ATPase corresponds to residues 364–474, which constitute a mobile domain that acquires a different conformation in the three catalytic subunits and contains the residues involved in the interaction with the γ-subunit. The equivalent domain in EscN ATPase includes residues 371-446. TrwB lacks a central coiled-coil subunit. Instead, one single DNA strand might be translocated through the internal cavity. DNA translocation could be driven by the movement of the “all-α-domain” as a consequence of ATPase activity (by analogy to F1-ATPase). (The figure has been created by using the PDB codes: 1e1q.pdb and 1e1r.pdb (Braig et al., 2000), 6njp.pdb (Majewski et al., 2019), and 1e9r.pdb (Gomis-Rüth et al., 2001).
Work in T3 and T4SSs has uncovered a broad range of effector functions upon translocation (Pearson et al., 2015; Pinaud et al., 2018; Cover et al., 2020; Timilsina et al., 2020; Fromm and Dehio, 2021; Nandi et al., 2021). It is clear that in order to perform their function, effectors need to be active once in the host, meaning that protein refolding must occur after translocation. Thus, effectors have evolved folds that are compatible with basic requirements: they should be able to easily unfold, pass through the narrow secretion channel, and refold to an active form when on the other side (Metcalf et al., 2016; Gazi et al., 2021; LeBlanc et al., 2021). This is specially challenging in T3SS and T4SSs, since substrates are very large proteins. For instance, the relaxase of the F plasmid (TraI) has 1,756 amino acid residues and that of the R388 plasmid (TrwC), 966. The characterization of the mechanism of substrate transport is worthwhile due to its biological relevance, but there are still important open questions about how this process is occurring.
A rotary catalytic mechanism for substrate secretion
Despite the differences in the structural architecture and in the type of substrates translocated by T3SS and T4SS, there is a significant structural homology between the main ATPase that powers substrate transfer in both secretion machineries. The cytosolic hexameric T3SS ATPase EscN from E. coli (InvC in Salmonella or SctN in the unified nomenclature) resembles the structure of the F1-ATPase (Zarivach et al., 2007; Majewski et al., 2019). Within the hexameric EscN ring there is a coiled-coil subunit named EscO (InvI/SpaM) that precludes the lumen of the ring. This arrangement is similar to that found in the F1-ATPase, where there is a γ subunit sitting in the center of the α/β heterohexamer (Figure 4). In the case of SctN, the ring is formed by a homohexamer, which also shares a high degree of structural similarity with the T4SS coupling protein TrwB (VirD4). The three proteins share a characteristic Rossmann fold, Walker A and B motifs and an hexameric stoichiometry (Figure 4). Thus, SctN and TrwB ATPases might be ancestral precursors to the heterohexameric rotary F1-ATPases, as previously proposed (Cabezon et al., 2012; Majewski et al., 2019).
EscN hexamer shows a marked asymmetry. The subunits present different occupancies in the active site and, subsequently, different conformational states. Thus, the six subunits would go through successive cycles of ATP binding, hydrolysis and ADP/phosphate release and the associated conformational changes at the C-terminal domain would be translated into torque on the EscO stalk (Majewski et al., 2019), in an analogous way to F1-ATPase. Since the interaction between the stalk and the export gate has been reported (Hara et al., 2012; Ibuki et al., 2013), it has been suggested that EscN might modulate the efficiency with which T3SS uses PMF. This finding also raises the possibility that elements of the export gate might function in a manner similar to the F0 components of the F0F1-ATPase, acting as a channel through which protons are pumped across the inner membrane (Majewski et al., 2019).
In the case of the T4SS ATPase homolog TrwB, six protein monomers associate to form an almost spherical quaternary structure that is also strikingly similar to F1-ATPase (Figure 4) (Gomis-Rüth et al., 2001), but with a six-fold symmetry. In F1- and EscN ATPases, protein structural asymmetry supports the rotary catalytic mechanism previously explained, but no structural asymmetry of the ring subunits has been found in TrwB to support a similar mechanism. However, it is tempting to propose that the asymmetry caused by the γ-subunit in F1-ATPase or the EscO subunit in EscN ATPase can also be induced in TrwB after DNA binding (Figure 4) (Cabezon and de la Cruz, 2006). Thus, such asymmetry would be observed in the active form of the protein, with a single DNA strand inside the central channel being translocated in a 5´- 3´direction. DNA might bind to a subunit in an “open” conformation (without ATP in the active site) and, following the binding-change mechanism, the subunit would change to a “closed” conformation. By doing so, the DNA would be sent into the internal cavity, being also rotated within the channel towards another catalytic subunit. Thus, a DNA pumping mechanism would take place iteratively, facilitating DNA transport through the T4SS. It is also important to note that DNA promotes TrwB oligomerization and activates its ATPase activity, in the same way as EscO enhances EscN oligomerization and ATPase activity in T3SSs. However, to date no experimental evidence is available to support this rotary mechanism for TrwB DNA pumping. Structural determination of TrwB in the presence of DNA could provide important insights in this regard.
Important aspects in the search of common inhibitors for both secretion systems
Based on the structural similarity between T3SS and T4SS, it should be possible to find inhibitors that target different pathogens simultaneously. As only pathogenic bacteria express these secretion systems, non-pathogenic bacteria would not be targeted (Blasey et al., 2023). Salicylidene acylhydrazides (SAHs), for instance, are a group of chemical inhibitors active against the T3 and T4SSs of several pathogens (Mühlen and Dersch, 2020). Interestingly, the target of some SAH derivatives in T4SS is the inner membrane protein VirB8, which does not present structural similarity with any of the inner membrane components of T3SSs (Figures 1, 2).
Other T3SS inhibitors such as thiazolidinone, which inhibits needle formation, also work on T2SSs. Since in these secretion systems the OM ring consists of pore-forming proteins that belong to the family of secretins, it is likely that this protein is the target of those compounds (Felise et al., 2008). A recent extensive review (Blasey et al., 2023) covers in depth the effects of many different inhibitors in both, T3 and T4SSs. Thus, it is not the aim of this work to focus on such analysis. While it is clear these chemical compounds inhibit effector secretion, more work is required to know the specific targets.
In that sense, ATPases are targets of great interest, since they are essential to power substrate translocation. In silico structure-based strategies to search for potential inhibitors from available libraries are a tool with great potential. Thus, it is possible to computationally screen millions of drug-like compounds and identify novel and high specific inhibitors. Ideally, inhibitors should operate through a non-competitive mechanism, in order to avoid off-target ATPases essential for cell metabolism. Small aromatic chemical compounds have been found to inhibit T3SS EscN ATPase and homologs from Shigella and Salmonella (Spa47 and FliI proteins, respectively), with an IC50 value of 25 µM (Case et al., 2020). A similar approach also allowed to identify two inhibitors of the homolog ATPase in Chlamidya (SctN), with IC50 values ~50-100 µM (Grishin et al., 2018) and in YscN ATPase from Yersinia, with IC50 values of ~20 µM (Swietnicki et al., 2011).
In T4SSs, most of the inhibitors against ATPases found so far have VirB11 ATPases as molecular target. Some examples are CHIR-1 compound (Hilleringmann et al., 2006) and also 8-amino imidazo[1,2-a]pyrazine derivatives (Sayer et al., 2014; Sayer et al., 2021), which act as inhibitors of the activity of HP0525 ATPase in Helicobacter pylori, with IC50 values in the rage 6 - 48 µM. Other examples are fatty acid derivatives, such as 2-hexadecanoic or 2-bromopalmitic acids, inhibitors of TrwD ATPase in R388 plasmid (IC50 values of ~20 µM) (Cabezón et al., 2017; García-Cazorla et al., 2018).
While micromolar IC50 values are not optimal for the development and use of these ATPase inhibitors as therapeutics, the identification of non-competitive inhibitors with low cellular toxicity is a major breakthrough, as these compounds would not affect the activity of F-type and other essential ATPases, compromising cell viability.
Conclusion
The objective of this review is to highlight common aspects shared by T3 and T4SSs that might help in understanding their molecular mechanism and, most importantly, in the pursuit of inhibitors capable of targeting these systems. Certainly, there are notable distinctions between them. For instance, T4SSs lack a continuous channel in the periplasm, unlike T3SSs. Additionally, T3SSs do not support DNA transfer and, more importantly, there is no reported utilization of PMF as an energy source for substrate transfer in T4SSs. These are just a few of the numerous differences between the two systems, with several components exhibiting no homology. However, it is worth highlighting the homology observed in one of the primary ATPases that serves as a power supply in both systems. In that sense, it is important to note that in T3SSs, EscN ATPase is capable of energizing secretion even in the absence of bulk PMF (Terashima et al., 2018). In T3SSs, a rotary catalytic mechanism for substrate transfer has been proposed, based on the strong parallelism observed with the F1-ATPase (Majewski et al., 2019). As proposed here, a similar mechanism might also work for T4SSs.
Notable progress has been made in identifying inhibitors for individual secretion systems. However, it would be of great interest to discover inhibitors that can effectively target both secretion systems simultaneously. A promising starting point for this endeavor would be EscN/TrwB ATPases, found in T3 and T4SSs, respectively. Employing structure-based drug design methods will play a crucial role in developing more efficient compounds for this shared protein target, ideally achieving IC50 values in the nanomolar range to enhance their efficacy.
Author contributions
EC: Conceptualization, Funding acquisition, Investigation, Project administration, Supervision, Writing – original draft, Writing – review & editing. FV: Investigation, Writing – original draft. IA: Investigation, Writing – original draft, Conceptualization, Funding acquisition, Supervision, Validation, Writing – review & editing.
Funding
The author(s) declare financial support was received for the research, authorship, and/or publication of this article. This work was supported by the Spanish Ministerio de Ciencia e Innovación Grant PID2019-104251GB-I00.
Conflict of interest
The authors declare that the research was conducted in the absence of any commercial or financial relationships that could be construed as a potential conflict of interest.
Publisher’s note
All claims expressed in this article are solely those of the authors and do not necessarily represent those of their affiliated organizations, or those of the publisher, the editors and the reviewers. Any product that may be evaluated in this article, or claim that may be made by its manufacturer, is not guaranteed or endorsed by the publisher.
References
Abby, S. S., Rocha, E. P. C. (2012). The non-flagellar type III secretion system evolved from the bacterial flagellum and diversified into host-cell adapted systems. PloS Genet. 8 (9), e1002983. doi: 10.1371/journal.pgen.1002983
Abrahams, J. P., Leslie, A. G.W., Lutter, R., Walker, J. E. (1994). Structure at 2.8 Â resolution of F1-ATPase from bovine heart mitochondria. Nature 370 (6491), 621–628. doi: 10.1038/370621a0
Abrusci, P., Vergara-Irigaray, M., Johnson, S., Beeby, M. D., Hendrixson, D. R., Roversi, P., et al. (2013). Architecture of the major component of the type III secretion system export apparatus. Nat. Struct. Mol. Biol. 20 (1), 99–104. doi: 10.1038/nsmb.2452
Akeda, Y., Galán, J. E. (2005). Chaperone release and unfolding of substrates in type III secretion. Nature 437 (7060), 911–915. doi: 10.1038/nature03992
Álvarez-Rodríguez, I., Ugarte-Uribe, B., de la Arada, I., Arrondo, J. L.R., Garbisu, C., Alkorta, I. (2020). Conjugative coupling proteins and the role of their domains in conjugation, secondary structure and in vivo subcellular location. Front. Mol. Biosci. 7. doi: 10.3389/fmolb.2020.00185
Aly, K. A., Baron, C. (2007). The VirB5 protein localizes to the T-pilus tips in Agrobacterium tumefaciens. Microbiology 153 (11), 3766–3775. doi: 10.1099/mic.0.2007/010462-0
Bergeron, J. R. C., Marlovits, T. C. (2022). Cryo-EM of the injectisome and type III secretion systems. Curr. Opin. Struct. Biol. 75, 102403. doi: 10.1016/j.sbi.2022.102403
Blasey, N., Rehrmann, D., Riebisch, A. K., Mühlen, S. (2023). Targeting bacterial pathogenesis by inhibiting virulence-associated Type III and Type IV secretion systems. Front. Cell. Infect. Microbiol. 12. doi: 10.3389/fcimb.2022.1065561
Böck, D., Hüsler, D., Steiner, B., Medeiros, J. M., Welin, A., Radomska, K. A., et al. (2021). The polar legionella Icm/Dot T4SS establishes distinct contact sites with the pathogen vacuole membrane. mBio 12 (5). doi: 10.1128/mBio.02180-21
Braig, K., Menz, R. I., Montgomery, M. G., Leslie, A. G., Walker, J. E. (2000). Structure of bovine mitochondrial F1-ATPase inhibited by Mg2+ADP and aluminium fluoride. Structure 8 (6), 567–573. doi: 10.1016/S0969-2126(00)00145-3
Cabezon, E., de la Cruz, F. (2006). TrwB: An F1-ATPase-like molecular motor involved in DNA transport during bacterial conjugation. Res. Microbiol. 157 (4), 299–305. doi: 10.1016/j.resmic.2005.12.002
Cabezón, E., de la Cruz, F., Arechaga, I. (2017). Conjugation inhibitors and their potential use to prevent dissemination of antibiotic resistance genes in bacteria. Front. Microbiol. 8. doi: 10.3389/fmicb.2017.02329
Cabezón, E., Ripoll-Rozada, J., Peña, A., de la Cruz, F., Arechaga, I. (2015). Towards an integrated model of bacterial conjugation. FEMS Microbiol. Rev. 39(1), 81–95. doi: 10.1111/1574-6976.12085
Cabezon, E., Lanza, V. F., Arechaga, I. (2012). Membrane-associated nanomotors for macromolecular transport. Curr. Opin. Biotechnol. 23 (4), 537–544. doi: 10.1016/j.copbio.2011.11.031
Cascales, E., Christie, P. J. (2003). The versatile bacterial type IV secretion systems. Nat. Rev. Microbiol. 1 (2), 137–149. doi: 10.1038/nrmicro753
Case, H. B., Mattock, D. S., Miller, B. R., Dickenson, N. E. (2020). Novel noncompetitive type three secretion system ATPase inhibitors shut down Shigella effector secretion. Biochemistry 59 (28), 2667–2678. doi: 10.1021/acs.biochem.0c00431
Chetrit, D., Hu, B., Christie, P. J., Roy, C. R., Liu, J. (2018). A unique cytoplasmic ATPase complex defines the Legionella pneumophila type IV secretion channel. Nat. Microbiol. 3 (6), 678–686. doi: 10.1038/s41564-018-0165-z
Christie, P. J. (2019). The rich tapestry of bacterial protein translocation systems. Protein J. 38 (4), 389–408. doi: 10.1007/s10930-019-09862-3
Christie, P. J., Whitaker, N., González-Rivera, C. (2014). Mechanism and structure of the bacterial type IV secretion systems. Biochim. Biophys. Acta (BBA) Mol. Cell Res. 1843 (8), 1578–1591. doi: 10.1016/j.bbamcr.2013.12.019
Chung, J. M., Sheedlo, M. J., Campbell, A. M., Sawhney, N., Frick-Cheng, A. E., Lacy, D. B., et al (2019). Structure of the Helicobacter pylori Cag type IV secretion system. eLife 8. doi: 10.7554/eLife.47644
Coburn, B., Sekirov, I., Finlay, B. B. (2007). Type III secretion systems and disease. Clin. Microbiol. Rev. 20 (4), 535–549. doi: 10.1128/CMR.00013-07
Cornelis, G. R. (2006). The type III secretion injectisome. Nat. Rev. Microbiol. 4 (11), 811–825. doi: 10.1038/nrmicro1526
Cornelis, G. R. (2010). The type III secretion injectisome, a complex nanomachine for intracellular “toxin” delivery. Biol. Chem. 391 (7), 745–751. doi: 10.1515/bc.2010.079
Costa, T. R. D., Ilangovan, A., Ukleja, M., Redzej, A., Santini, J. M., Smith, T. K., et al. (2016). Structure of the bacterial sex F pilus reveals an assembly of a stoichiometric protein-phospholipid complex. Cell 166 (6), 1436–1444.e10. doi: 10.1016/j.cell.2016.08.025
Costa, T. R. D., Harb, L., Khara, P., Zeng, L., Hu, B., Christie, P. J. (2021). Type IV secretion systems: Advances in structure, function, and activation. Mol. Microbiol. 115 (3), 436–452. doi: 10.1111/mmi.14670
Cover, T. L., Lacy, D. B., Ohi, M. D. (2020). The helicobacter pylori Cag type IV secretion system. Trends Microbiol. 28 (8), 682–695. doi: 10.1016/j.tim.2020.02.004
De La Cruz, F., Frost, L. S., Meyer, R. J., Zechner, E. L. (2010). Conjugative DNA metabolism in Gram-negative bacteria. FEMS Microbiol. Rev. 34 (1), 18–40. doi: 10.1111/j.1574-6976.2009.00195.x
Deng, W., Marshall, N. C., Rowland, J. L., McCoy, J. M., Worrall, L. J., Santos, A. S., et al. (2017). Assembly, structure, function and regulation of type III secretion systems. Nat. Rev. Microbiol. 15 (6), 323–337. doi: 10.1038/nrmicro.2017.20
Durie, C. L., Sheedlo, M. J., Chung, J. M., Byrne, B. G., Su, M., Knight, T., et al. (2020). Structural analysis of the Legionella pneumophila Dot/Icm type IV secretion system core complex. eLife 9. doi: 10.7554/eLife.59530
Erhardt, M., Mertens, M. E., Fabiani, F. D., Hughes, K. T. (2014). ATPase-independent type-III protein secretion in Salmonella enterica. PloS Genet. 10 (11), e1004800. doi: 10.1371/journal.pgen.1004800
Felise, H. B., Nguyen, H. V., Pfuetzner, R. A., Barry, K. C., Jackson, S. R., Blanc, M.-P., et al. (2008). An inhibitor of gram-negative bacterial virulence protein secretion. Cell Host Microbe 4 (4), 325–336. doi: 10.1016/j.chom.2008.08.001
Fromm, K., Dehio, C. (2021). The impact of Bartonella VirB/VirD4 type IV secretion system effectors on eukaryotic host cells. Front. Microbiol. 12. doi: 10.3389/fmicb.2021.762582
Galán, J. E., Waksman, G. (2018). Protein-injection machines in bacteria. Cell 172 (6), 1306–1318. doi: 10.1016/j.cell.2018.01.034
García-Cazorla, Y., Getino, M., Sanabria-Ríos, D. J., Carballeira, N. M., de la Cruz, F., Arechaga, I., et al. (2018). Conjugation inhibitors compete with palmitic acid for binding to the conjugative traffic ATPase TrwD, providing a mechanism to inhibit bacterial conjugation. J. Biol. Chem. 293 (43), 16923–16930. doi: 10.1074/jbc.RA118.004716
Gazi, A. D., Kokkinidis, M., Fadouloglou, V. E. (2021). α-helices in the type III secretion effectors: A prevalent feature with versatile roles. Int. J. Mol. Sci. 22 (11), 5412. doi: 10.3390/ijms22115412
Gomis-Rüth, F. X., Moncalián, G., Pérez-Luque, R., González, A., Cabezón, E., de la Cruz, F., et al. (2001). The bacterial conjugation protein TrwB resembles ring helicases and F1-ATPase. Nature 409 (6820), 637–641. doi: 10.1038/35054586
Gophna, U., Ron, E. Z., Graur, D. (2003). Bacterial type III secretion systems are ancient and evolved by multiple horizontal-transfer events. Gene 312, 151–163. doi: 10.1016/S0378-1119(03)00612-7
Grange, W., Duckely, M., Husale, S., Jacob, S., Engel, A., Hegner, M. (2008). VirE2: A unique ssDNA-compacting molecular machine. PloS Biol. 6 (2), e44. doi: 10.1371/journal.pbio.0060044
Grishin, A. V., Luyksaar, S. I., Kapotina, L. N., Kirsanov, D. D., Zayakin, E. S., Karyagina, A. S., et al. (2018). Identification of chlamydial T3SS inhibitors through virtual screening against T3SS ATPase. Chem. Biol. Drug Design 91 (3), 717–727. doi: 10.1111/cbdd.13130
Guglielmini, J., de la Cruz, F., Rocha, E. P. C. (2013). Evolution of conjugation and type IV secretion systems. Mol. Biol. Evol. 30 (2), 315–331. doi: 10.1093/molbev/mss221
Halte, M., Erhardt, M. (2021). Protein export via the type III secretion system of the bacterial flagellum. Biomolecules 11 (2), 186. doi: 10.3390/biom11020186
Hara, N., Morimoto, Y. V., Kawamoto, A., Namba, K., Minamino, T. (2012). Interaction of the extreme N-terminal region of FliH with FlhA is required for efficient bacterial flagellar protein export. J. Bacteriol. 194 (19), 5353–5360. doi: 10.1128/JB.01028-12
Hilleringmann, M., Pansegrau, W., Doyle, M., Kaufman, S., MacKichan, M. L., Gianfaldoni, C., et al. (2006). Inhibitors of Helicobacter pylori ATPase Cagα block CagA transport and cag virulence. Microbiology 152 (10), 2919–2930. doi: 10.1099/mic.0.28984-0
Hu, B., Lara-Tejero, M., Kong, Q., Galán, J. E., Liu, J. (2017). In situ molecular architecture of the salmonella type III secretion machine. Cell 168 (6), 1065–1074.e10. doi: 10.1016/j.cell.2017.02.022
Hu, J., Worrall, L. J., Hong, C., Vuckovic, M., Atkinson, C. E., Caveney, N., et al. (2018). Cryo-EM analysis of the T3S injectisome reveals the structure of the needle and open secretin. Nat. Commun. 9 (1), 3840. doi: 10.1038/s41467-018-06298-8
Hu, B., Khara, P., Song, L., Lin, A. S., Frick-Cheng, A. E., Harvey, M. L., et al (2019). In Situ Molecular Architecture of the Helicobacter pylori Cag Type IV Secretion System. MBio 10. doi: 10.1128/mBio.00849-19
Ibuki, T., Uchida, Y., Hironaka, Y., Namba, K., Imada, K., Minamino, T. (2013). Interaction between FliJ and FlhA, components of the bacterial flagellar type III export apparatus. J. Bacteriol. 195 (3), 466–473. doi: 10.1128/JB.01711-12
Kuhlen, L., Johnson, S., Cao, J., Deme, J. C., Lea, S. M. (2021). Nonameric structures of the cytoplasmic domain of FlhA and SctV in the context of the full-length protein. PloS One 16 (6), e0252800. doi: 10.1371/journal.pone.0252800
Lara-Tejero, M., Galán, J. E. (2019). The injectisome, a complex nanomachine for protein injection into mammalian cells. EcoSal Plus 8 (2). doi: 10.1128/ecosalplus.ESP-0039-2018
LeBlanc, M.-A., Fink, M. R., Perkins, T. T., Sousa, M. C. (2021). Type III secretion system effector proteins are mechanically labile. Proc. Natl. Acad. Sci. 118 (12), e2019566118. doi: 10.1073/pnas.2019566118
Lee, S. H., Galán, J. E. (2004). Salmonella type III secretion-associated chaperones confer secretion-pathway specificity. Mol. Microbiol. 51 (2), 483–495. doi: 10.1046/j.1365-2958.2003.03840.x
Lee, P.-C., Rietsch, A. (2015). Fueling type III secretion. Trends Microbiol. 23 (5), 296–300. doi: 10.1016/j.tim.2015.01.012
Liu, X., Khara, P., Baker, M. L., Christie, P. J., and Hu, B. (2022). Structure of a type IV secretion system core complex encoded by multi-drug resistance F plasmids. Nat. Commun. 13 (1), 379. doi: 10.1038/s41467-022-28058-5
Llosa, M., Gomis-Ruth, F. X., Coll, M., de la Cruz, F. (2002). Bacterial conjugation: a two-step mechanism for DNA transport Mol. Microbiol. 45 (1), 1–8. doi: 10.1046/j.1365-2958.2002.03014.x
Low, H. H., Gubellini, F., Rivera-Calzada, A., Braun, N., Connery, S., Dujeancourt, A., et al. (2014). Structure of a type IV secretion system. Nature 508 (7497), 550–553. doi: 10.1038/nature13081
Lunelli, M., Kamprad, A., Bürger, J., Mielke, T., Spahn, C. M. T., Kolbe, M. (2020). Cryo-EM structure of the Shigella type III needle complex. PloS Pathogens 16 (2), e1008263. doi: 10.1371/journal.ppat.1008263
Lyons, B. J. E., Atkinson, C. E., Deng, W., Serapio-Palacios, A., Finlay, B. B., Strynadka, N. C. J. (2021). Cryo-EM structure of the EspA filament from enteropathogenic Escherichia coli: Revealing the mechanism of effector translocation in the T3SS. Structure 29 (5), 479–487.e4. doi: 10.1016/j.str.2020.12.009
Macé, K., Vadakkepat, A. K., Redzej, A., Lukoyanova, N., Oomen, C., Braun, N., et al. (2022). Cryo-EM structure of a type IV secretion system. Nature 607 (7917), 191–196. doi: 10.1038/s41586-022-04859-y
Majewski, D. D., Worrall, L. J., Hong, C., Atkinson, C. E., Vuckovic, M., Watanabe, N., et al. (2019). Cryo-EM structure of the homohexameric T3SS ATPase-central stalk complex reveals rotary ATPase-like asymmetry. Nat. Commun. 10 (1), 626. doi: 10.1038/s41467-019-08477-7
Matias, V. R. F., Al-Amoudi, A., Dubochet, J., Beveridge, T. J. (2003). Cryo-Transmission Electron Microscopy of Frozen-Hydrated Sections of Escherichia coli and Pseudomonas aeruginosa. J. Bacteriol. 185 (20), 6112–6118. doi: 10.1128/JB.185.20.6112-6118.2003
Matthews-Palmer, T. R. S., Gonzalez-Rodriguez, N., Calcraft, T., Lagercrantz, S., Zachs, T., Yu, X.-J., et al. (2021). Structure of the cytoplasmic domain of SctV (SsaV) from the Salmonella SPI-2 injectisome and implications for a pH sensing mechanism. J. Struct. Biol. 213 (2), 107729. doi: 10.1016/j.jsb.2021.107729
Metcalf, K. J., Bevington, J. L., Rosales, S. L., Burdette, L. A., Valdivia, E., Tullman-Ercek, D. (2016). Proteins adopt functionally active conformations after type III secretion. Microb. Cell Factories 15 (1), 213. doi: 10.1186/s12934-016-0606-4
Miletic, S., Fahrenkamp, D., Goessweiner-Mohr, N., Wald, J., Pantel, M., Vesper, O., et al. (2021). Substrate-engaged type III secretion system structures reveal gating mechanism for unfolded protein translocation. Nat. Commun. 12 (1), 1546. doi: 10.1038/s41467-021-21143-1
Minamino, T., Imada, K., Kinoshita, M., Nakamura, S., Morimoto, Y. V., Namba, K. (2011). Structural insight into the rotational switching mechanism of the bacterial flagellar motor. PloS Biol. 9 (5), e1000616. doi: 10.1371/journal.pbio.1000616
Minamino, T., Imada, K., Namba, K. (2008). Mechanisms of type III protein export for bacterial flagellar assembly. Mol. Biosyst. 4 (11), 1105. doi: 10.1039/b808065h
Mühlen, S., Dersch, P. (2020). Treatment strategies for infections with shiga toxin-producing Escherichia coli. Front. Cell. Infect. Microbiol. 10. doi: 10.3389/fcimb.2020.00169
Muthuramalingam, M., Whittier, S. K., Lovell, S., Battaile, K. P., Tachiyama, S., Johnson, D. K., et al. (2020). The structures of SctK and SctD from Pseudomonas aeruginosa reveal the interface of the type III secretion system basal body and sorting platform. J. Mol. Biol. 432 (24), 166693. doi: 10.1016/j.jmb.2020.10.027
Nandi, I., Aroeti, L., Ramachandran, R. P., Kassa, E. G., Zlotkin‐Rivkin, E., Aroeti, B. (2021). Type III secreted effectors that target mitochondria. Cell. Microbiol. 23 (9), e13352. doi: 10.1111/cmi.13352
Pallen, M. J., Beatson, S. A., Bailey, C. M. (2005). Bioinformatics, genomics and evolution of non-flagellar type-III secretion systems: a Darwinian perpective. FEMS Microbiol. Rev. 29 (2), 201–229. doi: 10.1016/j.femsre.2005.01.001
Park, D., Chetrit, D., Hu, B., Roy, C. R., Liu, J. (2020). Analysis of Dot/Icm type IVB secretion system subassemblies by cryoelectron tomography reveals conformational changes induced by DotB binding. mBio 11 (1), e03328-19. doi: 10.1128/mBio.03328-19
Pearson, J. S., Zhang, Y., Newton, H. J., Hartland, E. L. (2015). Post-modern pathogens: surprising activities of translocated effectors from E. coli and Legionella. Curr. Opin. Microbiol. 23, 73–79. doi: 10.1016/j.mib.2014.11.005
Peña, A., Arechaga, I. (2013). Molecular motors in bacterial secretion. Microb. Physiol. 23 (4–5), 357–369. doi: 10.1159/000351360
Pinaud, L., Sansonetti, P. J., Phalipon, A. (2018). Host cell targeting by enteropathogenic bacteria T3SS effectors. Trends Microbiol. 26 (4), 266–283. doi: 10.1016/j.tim.2018.01.010
Planet, P. J., Kachlany, S. C., DeSalle, R., Figurski, D. H. (2001). Phylogeny of genes for secretion NTPases: Identification of the widespread tadA subfamily and development of a diagnostic key for gene classification. Proc. Natl. Acad. Sci. 98 (5), 2503–2508. doi: 10.1073/pnas.051436598
Ripoll-Rozada, J., Zunzunegui, S., de la Cruz, F., Arechaga, I., Cabezón, E. (2013). Functional interactions of VirB11 traffic ATPases with VirB4 and VirD4 molecular motors in type IV secretion systems. J. Bacteriol. 195 (18), 4195–4201. doi: 10.1128/JB.00437-13
Saier, M. (2004). Evolution of bacterial type III protein secretion systems. Trends Microbiol. 12 (3), 113–115. doi: 10.1016/j.tim.2004.01.003
Sayer, J. R., Walldén, K., Pesnot, T., Campbell, F., Gane, P. J., Simone, M., et al. (2014). 2- and 3-substituted imidazo[1,2-a]pyrazines as inhibitors of bacterial type IV secretion. Bioorg. Medicinal Chem. 22 (22), 6459–6470. doi: 10.1016/j.bmc.2014.09.036
Sayer, J. R., Walldén, K., Koss, H., Allan, H., Daviter, T., Gane, P. J., et al. (2021). Design, synthesis, and evaluation of peptide-imidazo[1,2- a ]pyrazine bioconjugates as potential bivalent inhibitors of the VirB11 ATPase HP0525. J. Pept. Sci. 27 (10), e3353. doi: 10.1002/psc.3353
Sheedlo, M. J., Chung, J. M., Sawhney, N., Durie, C. L., Cover, T. L., Ohi, M. D., et al. (2020). Cryo-EM reveals species-specific components within the Helicobacter pylori Cag type IV secretion system core complex. eLife 9, e59495. doi: 10.7554/eLife.59495
Sheedlo, M. J., Ohi, M. D., Lacy, D. B., Cover, T. L. (2022). Molecular architecture of bacterial type IV secretion systems. PloS Pathog. 18 (8), e1010720. doi: 10.1371/journal.ppat.1010720
Soto, J. E., Galán, J. E., Lara-Tejero, M. (2022). Assembly and architecture of the type III secretion sorting platform. Proc. Natl. Acad. Sci. 119 (51), e2218010119. doi: 10.1073/pnas.2218010119
Stebbins, C. E., Galán, J. E. (2001). Maintenance of an unfolded polypeptide by a cognate chaperone in bacterial type III secretion. Nature 414 (6859), 77–81. doi: 10.1038/35102073
Swietnicki, W., Carmany, D., Retford, M., Guelta, M., Dorsey, R., Bozue, J., et al. (2011). Identification of small-molecule inhibitors of Yersinia pestis type III secretion system YscN ATPase. PloS One 6 (5), e19716. doi: 10.1371/journal.pone.0019716
Tato, I., Zunzunegui, S., de la Cruz, F., Cabezon, E. (2005). TrwB, the coupling protein involved in DNA transport during bacterial conjugation, is a DNA-dependent ATPase. Proc. Natl. Acad. Sci. 102, 8156–8161. doi: 10.1073/pnas.0503402102
Terashima, H., Kawamoto, A., Tatsumi, C., Namba, K., Minamino, T., Imada, K. (2018). In vitro reconstitution of functional type III protein export and insights into flagellar assembly. mBio 9 (3), e00988-18. doi: 10.1128/mBio.00988-18
Timilsina, S., Potnis, N., Newberry, E. A., Liyanapathiranage, P., Iruegas-Bocardo, F., White, F. F., et al. (2020). Xanthomonas diversity, virulence and plant–pathogen interactions. Nat. Rev. Microbiol. 18 (8), 415–427. doi: 10.1038/s41579-020-0361-8
Trokter, M., Waksman, G. (2018). Translocation through the conjugative type IV secretion system requires unfolding of its protein substrate. J. Bacteriol. 200 (6), e00615-17. doi: 10.1128/JB.00615-17
Valenzuela-Gómez, F., Arechaga, I., Cabezón, E. (2023). Nanopore sensing reveals a preferential pathway for the co-translocational unfolding of a conjugative relaxase–DNA complex. Nucleic Acids Res 51 (13), 6857–6869. doi: 10.1093/nar/gkad492
Viana, F., Peringathara, S. S., Rizvi, A., Schroeder, G. N. (2021). Host manipulation by bacterial type III and type IV secretion system effector proteases. Cell. Microbiol. 23 (11), e13384. doi: 10.1111/cmi.13384
Wilharm, G., Lehmann, V., Krauss, K., Lehnert, B., Richter, S., Ruckdeschel, K., et al. (2004). Yersinia enterocolitica Type III Secretion Depends on the Proton Motive Force but Not on the Flagellar Motor Components MotA and MotB. Infect. Immun. 72 (7), 4004–4009. doi: 10.1128/IAI.72.7.4004-4009.2004
Keywords: T3SS, T4SS, bacterial conjugation, effector delivery, ATPases
Citation: Cabezón E, Valenzuela-Gómez F and Arechaga I (2023) Primary architecture and energy requirements of Type III and Type IV secretion systems. Front. Cell. Infect. Microbiol. 13:1255852. doi: 10.3389/fcimb.2023.1255852
Received: 09 July 2023; Accepted: 08 November 2023;
Published: 27 November 2023.
Edited by:
David Chetrit, Yale University, United StatesReviewed by:
Anastasia D. Gazi, Institut Pasteur, FranceDavid A. Cisneros, Queen’s University Belfast, United Kingdom
Tomoe Kitao, Gifu University, Japan
Copyright © 2023 Cabezón, Valenzuela-Gómez and Arechaga. This is an open-access article distributed under the terms of the Creative Commons Attribution License (CC BY). The use, distribution or reproduction in other forums is permitted, provided the original author(s) and the copyright owner(s) are credited and that the original publication in this journal is cited, in accordance with accepted academic practice. No use, distribution or reproduction is permitted which does not comply with these terms.
*Correspondence: Elena Cabezón, Y2FiZXpvbmVAdW5pY2FuLmVz; Ignacio Arechaga, YXJlY2hhZ2FpQHVuaWNhbi5lcw==