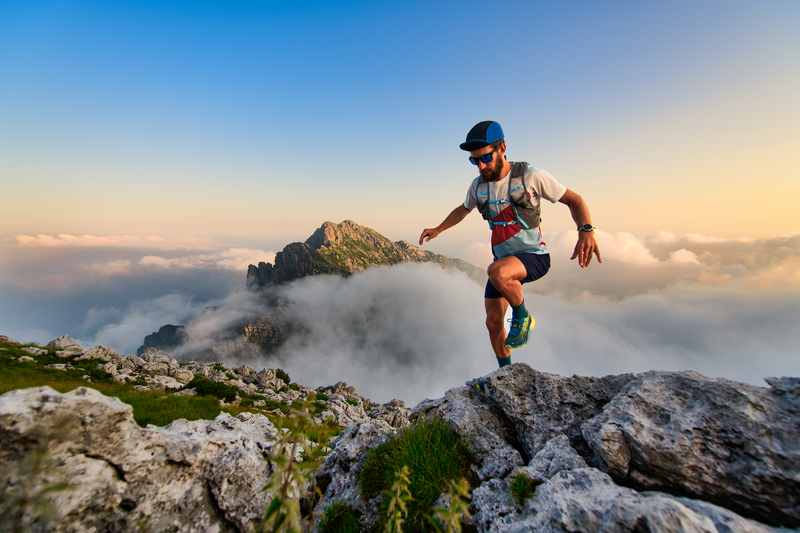
95% of researchers rate our articles as excellent or good
Learn more about the work of our research integrity team to safeguard the quality of each article we publish.
Find out more
ORIGINAL RESEARCH article
Front. Cell. Infect. Microbiol. , 11 December 2023
Sec. Fungal Pathogenesis
Volume 13 - 2023 | https://doi.org/10.3389/fcimb.2023.1254475
This article is part of the Research Topic The diversified interactions between phytopathogenic fungi, insect pests and host plants in forest health View all 5 articles
Background: ‘The fall armyworm, Spodoptera frugiperda’, represents a significant threat to maize production, a major staple crop in Asian countries.
Methods: In pursuit of more effective control of this insect pest, our study assessed the physiological and biochemical effects of the entomopathogenic fungus Metarhizium anisopliae against the larvae of S. frugiperda.
Results: Results revealed that, following nine days of treatment, a high concentration of conidia (1.5x107 conidia/mL-1) was toxic to all stages of larvae (second to fifth instar), resulting in 97% mortality of the second instar, 89% mortality of the third instar, 77% mortality of the fourth instar, and 72% mortality of fifth instar. All larval instars were found to have dose-dependent mortality effects. Treated S. frugiperda larvae further displayed significant physiological, morphological, and behavioral changes. Here, treated larvae displayed significantly lower levels of acetylcholinesterase, α-carboxylesterase, and β-carboxylesterase enzyme activity when compared to control groups. Treated larvae underwent an outward morphological change as the result of a decrease in the exterior cuticle of the anal papillae and a demelanization of the interior cuticle. Treated larvae also exhibited abnormal feeding behaviors as a consequence of the negative impact of conidia treatment on the neuromuscular system. Investigation into the effect of M. anisopliae on the non-target organism, the earthworm Eudrilus eugeniae, revealed that M. anisopliae conidia did not produce significant pathogenicity following three days of treatment. Furthermore, histological analysis revealed no significant effect of the entomopathogenic fungi on the gut tissue of the non-target organism.
Conclusion: This study highlights the potential of M. anisopliae in the control of S. frugiperda.
• Highest larval mortality (97%) of S. frugiperda 2nd instar larvae was obtained with a concentration of 1.5×107 conidia/mL.
• The activity of AChE, α-carboxylesterase, and β-carboxylesterase enzymes were reduced in larvae exposed to M. anisopliae conidia.
• Metarhizium anisopliae conidia were found to have no significant effect on the terrestrial soil bioindicator earthworm E. eugeniae.
According to United Nations forecasts, the global population has increased significantly in recent decades and could reach 9.7 billion people by 2050 (Liu et al., 2017; Fadiji and Babalola, 2020; Bamisile et al., 2021). This expansion necessitates innovative approaches and agricultural applications to secure the sustainability of the food supply (Vos and Bellù, 2019). Accordingly, reducing crop losses is a potential way to increase the quantity of obtainable food materials (Bommarco et al., 2018). The alteration of climatic conditions, outbreaks of various plant-based diseases, and pests are critical factors contributing to the decline of agricultural production globally (Bommarco et al., 2018; Savary et al., 2019). Indeed, the yield of maize, rice, wheat, soybean, potato, barley, cotton, and sugar beet has decreased as a direct result of the impact of pests, which has resulted in an estimated decline of 58% in agricultural production (Oerke, 2006).
Among the agronomy-related pests, the fall armyworm, Spodoptera frugiperda (Lepidoptera: Noctuoidea), is an insect pest endemic to both Asia and the Americas. It is a migratory species that feeds on a variety of plant materials and has been found on 353 different host plant species, spanning seventy-six different plant families (Agravante et al., 2022). Furthermore, this pest is capable of infecting cultivated grasses, most notably maize and sorghum (Montezano et al., 2018; Liu et al., 2020; Tambo et al., 2020). S. frugiperda is one of the most serious noctuidae pests of maize in Thailand, China, India, and North and South America (Tambo et al., 2020; Castro et al., 2021; Pittarate et al., 2021), owing to its ability to form large populations, the high larval voracity, and high rate of adult dispersal. Recent research reports have shown that S. frugiperda is an invasive pest in many countries in Africa and Asia (Fan et al., 2020; Firake and Behere, 2020; Jing et al., 2020; Lee et al., 2020). These countries have favorable climatic conditions and a large variety of plants that can serve as hosts, and, as such, S. frugiperda is likely to spread rapidly and cause serious damage to local plants (Wightman, 2018; EFSA, 2020). An estimated $US13 billion in crop losses occur every year in Africa as a result of the fall armyworm. This includes staple crops including corn, rice, and sorghum (Harrison et al., 2019; Tambo et al., 2020; Yigezu and Wakgari, 2020). Therefore, if effective strategies for control are not found or implemented, the pest could pose a serious threat to long-term agricultural productivity and food security.
The search for dependable biological control agents to supplement the use of chemical control began in the early 1980s, when it was discovered that the majority of the insects being managed via chemical control were developing resistance to various types of chemical insecticides and that such chemicals were having a negative impact on beneficial soil living organisms, particularly earthworms (Pelosi et al., 2021). Earthworms are considered a crucial soil indicator species due to their ability to significantly alter the chemical properties of the soil and improve overall soil health (Tondoh et al., 2007; Yuvaraj et al., 2019; Yuvaraj et al., 2020; Yuvaraj et al., 2021a; Yuvaraj et al., 2021b). Due to the widespread use of a variety of insecticides in agricultural soil, earthworm populations have been affected, which has led to a deterioration in the quality of the soil and a subsequent reduction in crop yield (Bagni et al., 2022).
In pursuit of alternative pest control methods to reduce the current overdependence on chemical control, over 750 species of entomopathogenic microorganisms from 85 genera have been found (Liu et al., 2012; Vivekanandhan et al., 2023), including fungi, nematodes, bacteria, and viruses (Shahid et al., 2012; Paschapur et al., 2021). Entomopathogenic fungi can effectively kill a wide range of economically important agricultural insect pests. Bio-insecticides made from entomopathogenic fungi are readily available on the market, including products from the genera Beauveria sp., Cordyceps sp., Isaria sp., Lecanicillium sp., Metarhizium sp., and Nomuraea sp. (Javed et al., 2019; Bara and Laing, 2020; Islam et al., 2021; Rajula et al., 2021; Shehzad et al., 2021; Shehzad et al., 2022; Tu et al., 2023). Because these entomopathogenic fungi are widespread and adaptable, they have been used successfully to eliminate insects in a wide range of habitats around the world (Roberts and Humber, 1981; Scholte et al, 2004; Ambethgar, 2009; Goble et al, 2010; Araújo and Hughes, 2016; Vivekanandhan et al., 2020a). Furthermore, the majority of the entomopathogenic fungi overwinter in the soil, ready to infect the following generation of insect hosts, making biological control an ideal and, eventually, less expensive option than chemical control (Duchowicz et al., 2009; Vivekanandhan et al., 2022a; Vivekanandhan et al., 2022b; Vivekanandhan et al., 2022c).
Specialized enzymes found in insects play vital roles in biochemical and physiological processes (Dittmer and Kanost, 2010). Acetylcholinesterase (AChE), an essential enzyme presents in the nervous system of insects, functions to modulate neurotransmission through the hydrolysis of the neurotransmitter acetylcholine (Colovic et al., 2013). AChE serves a critical role in regulating muscle activity and transmitting nerve signals, making it a target for many insecticides (Colovic et al., 2013; Thapa et al., 2017). Additional enzymes present in insects are β-carboxylesterase and α-carboxylesterase, both of which are involved in metabolic processes and detoxification. These enzymes break down ester compounds as part of a variety of metabolic processes, which also include the detoxification of insecticides and plant defense chemicals. Enzymes thus play a crucial role in insect adaptation by influencing their susceptibility or resistance to pesticides. For this reason, comprehending the biochemistry of these enzymes is imperative in order to formulate efficacious pest management strategies for the benefit of public health and agriculture.
In the current study, the entomopathogenic fungus Metarhizium anisopliae was chosen as the study biopesticide because it has been shown to be effective against a wide range of insect pests (Vivekanandhan et al., 2022a) and offers an environmentally friendly and targeted alternative to agricultural pesticides (Bapfubusa Niyibizi et al., 2023). The present investigation aimed to evaluate the physiological and biochemical effects of M. anisopliae conidia on the larvae of S. frugiperda, as well as the impact on the non-target bioindicator species Eudrilus eugeniae.
Spodoptera frugiperda eggs were procured from a local maize field in Chiang Mai, Thailand (18.7883° N, 98.9853° E). Eggs were placed in a ventilated plastic box (25×15×16 cm) and observed daily until hatching. Neonates (from first–third instars) were maintained on fresh and tender maize leaves in a plastic container. Small plastic boxes (11×8×10 cm) were used for the fourth to fifth instars to avoid cannibalism until pupation. The insect cultures were maintained at a temperature of 27°C and a 12:12 photoperiod (L:D).
From our previous research, we collected several potent strains of entomopathogenic fungi, particularly M. anisopliae (Vivekanandhan et al., 2020a; Vivekanandhan et al., 2020b) (see Supplementary Figures 1A, B). Potato dextrose agar (PDA) medium was employed to maintain the fungal culture at 27°C for 14 days. After the incubation time, a sterile loop was used to gently scrape the surface of the agar plate to remove conidia (Vivekanandhan et al., 2022a). After aggressively shaking the mixture for three minutes with a 0.05% Tween 80 solution, the hyphae were separated using a sterile millipore cloth. These conidia were spun for twenty minutes to ensure that they were free of clumps. Fungal conidia were counted using a Neubauer hemocytometer chamber, and the active dosage of conidia was estimated using light microscopy (Olympus DSX510i, Japan) at 40X magnification. By diluting the original solution in sterilized distilled water, five distinct concentrations were obtained: 1.5×103, 1.55×104, 1.5×105, 1.5×106, and 1.5×107 conidia/mL. Insecticidal bioassays were executed with these different concentrations.
Under laboratory conditions, S. frugiperda larvae (second-fifth) were tested for larvicidal activity with M. anisopliae conidial concentration for up to 9 days. Fresh, immature maize leaves were dipped with various concentrations of fungal conidia (1.5×103, 1.5×104, 1.5×105, 1.5×106, and 1.5×107 conidia/mL), and the treated containers were air dried for 10-15 minutes. Then, twenty-five (second to fifth) instar larvae of S. frugiperda were individually transferred into a (5×5×5 cm) plastic bioassay container. In the negative control group, 0.05% Tween-80 was used. Every day, the larvae were fed fresh, surface-sterilized maize leaves. Larval mortality was subsequently assessed for nine days at three-day intervals. Each concentration comprised three replications, and each replication contained twenty-five S. frugiperda larval (second-fifth) instars. The treatment bioassay container was incubated at a temperature of 27 ± 2°C.
Infected and control larvae were placed in Petri dishes lined with moist filter paper, and incubated at 27 ± 2°C. The fungal colonization of larvae was examined using a light microscope. After 5-7 days of incubation, all larval stages of S. frugiperda were evaluated for entomopathogenic fungal mycelium growth.
The hemocyte levels of leaf dip treated and control larvae were investigated. Three days post-treatment, hemolymph was collected from the control and experimental groups. The hemolymph was gently inverted two to three times in a 1.5-mL plastic tube with 1 mL of ice-cold anticoagulant buffer (0.01 mL EDTA, 0.1 mL glucose, 0.62 mL NaCl, and 0.26 mL citric acid; pH 4.6) to prevent breakdown. A modified Neubauer hemocytometer (Rohem, India) and a 50-X phase contrast microscope (Magnus MLXi, Japan) were used to count the hemocytes in each corner. The following formula was used to calculate the total hemocyte count:
X-Total number of cells counted; Dilution factor-20; Depth factor-10.
Live S. frugiperda control and conidia-treated larvae were washed with double-distilled water and wiped with tissue paper to remove excess water. Control and conidia-treated larvae were homogenized in 50 mL of ice-cold sodium phosphate buffer (20 mM, pH 7) to determine enzyme activity levels. The homogenates were previously centrifuged (8000 g for 20 min at 4°C), and the supernatants were used for further investigation. The homogenates were maintained on ice until use in subsequent experiments.
After larvicidal experiments, control and conidia-treated S. frugiperda larvae were separated. Excess water was wiped away before cleaning the larvae. Ellman et al. (1961) employed acetylcholine iodide as a substrate to evaluate the acetylcholine esterase activity of the larval homogenate. 850 mL of 100 mM sodium phosphate buffer was mixed with 50 mL of larval tissues (pH 7.5). Each reaction mixture contained 50 mL of 10 mM DTNB and 50 mL of 12.5 mM cholinergic iodide, which were kept at room temperature for 5 minutes. A suitable blank and a Multiskan EX (200–240 V) spectrophotometer were used to measure the sample’s optical density at 405 nm.
We used the method described in Van Asperen (1962) to investigate the activity of α- and β-carboxylesterase enzyme responses in larval homogenate. For the tests, 30 mL of homogenate were combined with 1 mL of sodium phosphate buffer (pH 7.0, 100 mM) containing 250 mM of α- and β-naphthyl acetate. This solution was then maintained at room temperature for 30 minutes. Each reaction mixture was then treated with 400 µL of 0.3% Fast Blue B in 3.3% SDS to block the enzymatic process. The combinations were allowed to remain at room temperature for 15 minutes to develop the distinct color. Multiskan EX-200-240V (Thermo Scientific) and the correct reagent of blank were used to determine the optical density of the sample. The activities of α- and β-carboxylesterase were then determined using a standard blot curve with naphthol as a control.
Eudrilus eugeniae were maintained at 27 ± 2°C on agricultural waste and cow dung. For experimental purposes, earthworms were removed from the soil by hand sorting. Earthworm bodies were washed with tap water to remove dirt. Earthworm cocoons were counted before being placed on separate bedding.
The artificial soil toxicity test was performed in accordance with the method detailed in Vivekanandhan et al. (2022a). The artificial soil was composed of 69% fine sand, 12% crushed sphagnum peat, and 19% kaolinite clay. Small amounts of fine sand, a chemical insecticide (Monocrotophos 250 ppm), and different amounts of fungal conidia were mixed together for each test concentration. After 0.65 kg of dirt and 15 mature earthworms had been added to each 500-mL glass jar, the jars were sealed. The jars were lightly covered with polypropylene covers to allow for ventilation. Jars were kept at a constant temperature of 27 ± 2°C and a relative humidity of 85–90%. Earthworm mortality was assessed after 9 days of treatment.
Individually, 15 adult E. eugeniae were tested for a period of 9 days with either fungal conidia, the organophosphate insecticide Monocrotophos, or distilled water (negative control). Both the control and treated earthworms were fixed in 3% formalin for 3 hours at 4°C after 9 days of treatment. The blocks were cooled for 3 hours at 4°C before being sliced into 2.5-mm-thick ribbons with a microtome (Leica, Germany). Ehrlich’s hematoxylin and eosin were used to stain E. eugeniae sections. After staining, the slides were left for drying and later viewed under a light microscope at 40X (Olympus CH20i/India).
The mortality data were subjected to analysis of variance (ANOVA) using logarithmic, arcsine, and square root transformed percentages. The mean of five replications was used to estimate the observed readings. The disparity in the data values among the various treatment groups for larvae was evaluated using Tukey’s multiple range test (p < 0.05) in conjunction with the Minitab®17 software. Sigma Plot 12 (Microcal Software) was employed to calculate the statistical changes for the estimation of mid-gut enzyme. The estimation of the lethal dosage (LC50) against larvae after nine days was performed using the Probit study in conjunction with the Minitab®17 software package, with a 95% confidence interval.
There was a significant difference in larval mortality between the control and conidia-treated groups (Figures 1A–D). For second instar larvae, the various fungal conidia concentrations produced 37-69% mortality three days post treatment (df: 5, F(5,12) =144.071; p≤ 0.01), 60–89% mortality six days post treatment (df: 5, F(5,12)=264.862; p≤ 0.01), and 62-97% mortality nine days post treatment (df: 5, F(5,12)=194.480; p≤ 0.01). When compared to the control (Figure 1A), significant differences were observed. For third instar larvae, the fungal conidia produced 25-55% mortality three days post treatment (df: 5, F(5,12)=69.700; p≤ 0.01), 48-78% mortality six days post treatment (df: 5, F(5,12)=142.322; p≤ 0.01), and 50-89% mortality nine days post treatment (df: 5, F(5,12)=101.656; p≤ 0.01) When compared to the control (Figure 1B), significant differences were observed. Notable difference in larvicidal activity were observed for forth instar larvae. The fungal conidia showed 18-50% mortality three days post treatment (df: 5, F(5,12)=210.270; p≤ 0.01), 36-66% mortality six days post treatment (df: 5, F(5,12)=454.450; p≤ 0.01), and 45-77% mortality nine days post treatment (df: 5, F(5,12)=188.677; p≤ 0.01). When compared to the control (Figure 1C), significant differences were observed. For fifth instar larvae, fungal conidia produced 9-42% mortality three days post treatment (df: 5, F(5,12)=208.200; p≤ 0.01), 29-61% six days post treatment (df: 5, F(5,12)=195.425; p≤ 0.01), and 44-72% mortality nine days post treatment (df: 5, F(5,12)=153.492; p≤ 0.01). When compared to the control (Figure 1D), significant differences were observed. Overall, significant differences in larval mortality were observed between control and treatment for all five different concentrations of fungal conidia against second to fifth larval stages of S. frugiperda.
Figure 1 Larvicidal activities of entomopathogenic fungi M. anisopliae conidia against 2nd to 5th instar larvae. (A) 2nd instar larvae; (B) 3rd instar larvae; (C) 4th instar larvae; (D) 5th instar larvae. Statistical values followed by different letters are significantly different at p≤ 0.05 according to Tukey’s test.
Spodoptera frugiperda larvae were subjected to M. anisopliae fungal conidia at various concentrations (1.5×103, 1.5×104, 1.5×105, 1.5×106, and 1.5×107 conidia/mL). When the concentrations of fungal conidia were increased, haemocyte levels gradually decreased. After three days of treatment, fungal conidia at a higher dose of 1.5×107 conidia/mL reduced haemocyte count by 25.01% and was statistically different from the control (df 5; F (5,12) = 272.004; P≤ 0.01) (Figure 2).
Figure 2 After three days of treatment, the total larval haemocyte counts in S. frugiperda larvae decreased in a dose-dependent manner. Statistical values followed by different letters are significantly different at p≤ 0.05 according to Tukey’s test.
The biochemical effects of entomopathogenic fungal conidia on S. frugiperda larvae were investigated. As conidia concentrations increased, the amount of AchE enzyme activity decreased. A maximal level of AchE was expressed at a concentration of 1.5×107 conidia/mL (df 5; F (5,12) = 170.162; p≤ 0.01) (Figure 3). The enzyme level in the control larvae was found to be normal. Enzyme expression was mainly dose-dependent, with larvae exposed to entomopathogenic fungal conidia displaying lower levels of AchE enzymes (Figure 3).
Figure 3 Three days after treatment, S. frugiperda’s acetylcholinesterase enzyme response to the insect pathogenic fungus M. anisopliae conidia and showed dose-dependent activity. Statistical values followed by different letters are significantly different at p≤ 0.05 according to Tukey’s test.
Results showed that the treatment of M. anisopliae fungal conidia against larvae of S. frugiperda resulted in a lower level of α and β-carboxylesterase enzyme activity (Figures 4A, B), with the lowest level achieved after three days of treatment. Conidia of M. anisopliae had a dose-dependent effect on the α and β-carboxylesterase enzyme activity in larvae. When larvae were exposed to fungal conidia, their α-carboxylesterase activity was considerably reduced compared to control (5.88 to 2.6 mg protein/mL of homogenate) (df 5; F(5,12) = 139.597; p≤ 0.01). (Figures 4A, B). A similar finding was observed with β-carboxylesterase, which showed a decrease in activity (from 4.9 to 1.9 mM/protein/mg/min) (df = 5; F(5, 12) = 172.778; p≤ 0.01) in S. frugiperda larvae (Figures 4A, B).
Figure 4 Three days after treatment, S. frugiperda α-carboxylesterase and β-carboxylesterase enzyme responses to insect pathogenic fungi M. anisopliae conidia caused dose-dependent activity. (A) α-carboxylesterase enzyme; (B) β-carboxylesterase enzymes. Statistical values followed by different letters are significantly different at p≤ 0.05 according to Tukey’s test.
The results of the artificial soil bioassay revealed that entomopathogenic fungi had no effect on the beneficial non-target species E. eugeniae. The results suggests that the mortality rate ranged from 2 to 8% with the treatment of fungal conidia and was not significantly different to control individuals. Fungal conidia thus had no significant effect on earthworm mortality (df = 5; F (5, 12) = 5.133; p 0.01). In contrast, more than 93% of earthworms died because of the monocrotophos treatment. The chemical pesticide, Monocrotophos caused high mortality on E. eugeniae when compared to fungal conidia treatment (df = 4; F (1, 4) = 420.250; p 0.01). Monocrotophos was substantially more toxic to the earthworm when compared to M. anisopliae conidial concentrations of 1.5×107conidia/mL. Furthermore, M. anisopliae fungal conidia were not pathogenic to earthworms after 9 days of treatment. The monocrotophos and control groups were statistically different (df= 4; F (1, 4) =420.250; p≤ 0.01). Monocrotophos was found to be the most dangerous to earthworms when compared to fungal conidia and the negative control (Figure 5).
Figure 5 A normal epidermis (E), circular muscle (CM), and longitudinal muscle (LM) can be seen in the cross section of E. eugeniae, control, and fungal spore treatments. Monocrotophos treatments resulted in abnormal histology: I, degenerated cells; II, cellular debris; III, an irregular epidermal surface; and IV, degenerated cells.
The histopathological findings revealed that M. anisopliae conidia are not toxic to earthworms as compared to Monocrotophos. In the fungal conidia group, treated earthworm gut tissues did not contain any fungal conidia, and the fungal conidia were unable to disintegrate the earthworm epidermis. The fungal conidia had no toxicity effects on earthworm gut tissues; all tissues including the epidermis, circular muscle, setae, mitochondrion, and intestinal lumen tissues were normal and similar to the negative control. In contrast, treatment with monocrotophos resulted in significant damage to the earthworm tissues including the epidermis, circular muscle, setae, mitochondrion, and intestinal lumen tissues (Figure 5).
Metarhizium anisopliae conidia with a green color mycelium completely invaded infected S. frugiperda larvae (second to fifth instar). In contrast, control insects were healthy and showed no signs of fungal infection. M. anisopliae fungal mycelium has been found to be more capable of proliferating and infiltrating host tissues, resulting in dose-dependent mortality against larvae. Larvae were found to be especially vulnerable to fungal conidia at a concentration of 1.5×107 conidia/mL-1.
The current study revealed that M. anisopliae fungal conidia caused significant insect larvicidal activity, resulting in up to 97% morality. Among the larval instars of S. frugiperda, second and third instars were found to be the most susceptible to M. anisopliae. Here, M. anisopliae conidia resulted in 97% mortality in the second instar, 87% in the third instar, 77% in the fourth instar, and 72% in the fifth instar larvae at nine days post-treatment. Insect larvae in their early instar stages are more sensitive to fungal infection than larvae in later instars as a result of the immune and physiological systems still developing (Perumal et al., 2023a; Perumal et al., 2023b). Similar to our study, the entomopathogenic fungus, M. anisopliae has been previously shown to cause 100% mortality rates in S. frugiperda under laboratory trials (Ramos et al., 2020). Furthermore, M. anisopliae has successfully suppressed Lepidopteran insects, as evidenced by research on Plutella xylostella, which showed the fungi to result in 74% larval mortality (Batta, 2013). It has been previously established that the lethal activity of M. anisopliae is proportional to its concentration, with higher concentrations shown to be more effective against insect pests (Opisa et al., 2018). Our findings confirm this, with the maximum mortality being attained with a concentration of 1×107 mL/L. Similar to the present study, M. anisopliae 1×107 conidia/mL concentration caused 100% mortality against second instar larvae of S. exigua at three days after treatment, and P. fumosoroseus caused 100% mortality at six days after treatment using 1×104 conidia/mL (Han et al., 2014). In addition, the current study found that S. frugiperda larvae treated with M. anisopliae conidia displayed significantly reduced mobility compared to the control group after three days post-treatment. The larvae were observed to be lifeless and had stopped feeding. Many larvae were dead within the first or second day of treatment.
Our results showed that the entomopathogenic fungus M. anisopliae conidia reduced larval haemocyte count when compared to the control group after three days post-treatment, with haemocyte count significantly reduced by 25.01% when compared to the control group. Similarly, Karthi et al. (2018); Balumahendhiren et al. (2019) reported that fungal conidial treatment with the entomopathogenic fungi A. flavus was capable of significantly reducing the haemocyte count of Spodoptera litura larvae at low concentrations (4×108 conidia/ml) within three days. In addition to affecting haemocyte concentration, fungi are capable of influencing the immune system of the pest (Xu et al., 2018; Bai et al., 2022). The biochemical effects of the entomopathogenic fungal conidia on S. frugiperda larvae were thus further investigated in this study. Results revealed that, when larvae were exposed to the fungal conidia of M. anisopliae, AchE enzyme activity was significantly reduced. This supports previous findings that A. flavus fungal conidia treatment reduced AchE enzyme levels in the larvae of S. litura under laboratory conditions (Karthi et al., 2018). In addition to impacting AchE enzyme levels, the current study found that the application of M. anisopliae conidia to S. frugiperda larvae led to a reduction in the levels of α- and β-carboxylesterase enzyme activities. The α-carboxylesterase enzyme levels were reduced from 5.88 to 2.6 mM/protein/mg/min and the β-carboxylesterase enzyme from 4.9 to 1.9 mM/protein/mg/min. The most significant decrease in β-carboxylesterase activity occurred after a treatment period of three days. These results support previously published studies showing that entomopathogenic fungal conidia and secondary metabolites reduce the acetylcholinesterase, α- and β-carboxylesterase enzyme levels in Spodoptera litura and Spodoptera frudiperda (Karthi et al., 2018; Karthi et al., 2019; Perumal et al., 2023a; Perumal et al., 2023b).
According to the findings of the bioassay conducted on artificial soil, entomopathogenic fungi had no effect on the E. eugeniae species, which is a beneficial non-target species. In the current studies, the mortality rate with fungal conidia treatment ranged from 2% to 8%. In contrast, more than 93% of earthworms were dead due to the Monocrotophos treatment. The results demonstrate that fungal conidia have no significant negative impact on earthworms. Similar to the current results, the entomopathogenic fungi M. anisopliae was found to have no effect on E. eugeniae earthworm species in soil condition (Karthi et al., 2019; Perumal et al., 2023a). Furthermore, M. majus conidia did not cause pathogenicity to E. eugeniae under laboratory condition (Karthi et al., 2019; Perumal et al., 2023b). Histopathological findings further support that M. anisopliae conidia are not hazardous to soil-living earthworm species when compared to chemical pesticides (Monocrotophos). Results showed that M. anisopliae conidia do not contain the inside of the earthworm gut tissues. This supports previous research that fungal conidia do not produce pathogenic effects on the earthworm gut tissues (Karthi et al., 2019; Perumal et al., 2023a). Results showed that all earthworm tissues, such as the epidermis, circular muscle, setae, mitochondrion, and intestinal lumen tissues, were normal and similar to the negative control. In contrast, the monocrotophos was completely toxic to earthworm tissues.
In conclusion, results established that entomopathogenic fungal conidia are capable of causing up to 97% mortality rates in S. frugiperda larvae, with the second and third instar larvae being the most susceptible to entomopathogenic fungi conidia treatment of the instar stages. Results demonstrated that M. anisopliae conidia induce high pathogenicity in the earlier larval stage, and it is therefore expected that the fungi colonized the larval cadaver after the treatment period. In addition, S. frugiperda larvae responded to the fungal conidia at three days post-treatment, with haemocyte count significantly reduced when compared to the control group. Results further showed that entomopathogenic fungal conidial infection reduced acetylcholinesterase, α- and β-carboxylesterase enzyme activities in S. frugiperda larvae, with enzyme activity reduced in a dose-dependent manner. This study shows that M. anisopliae is a potential candidate in the control of S. frugiperda.
The original contributions presented in the study are included in the article/Supplementary Material. Further inquiries can be directed to the corresponding authors.
PV: Conceptualization, Investigation, Methodology, Project administration, Resources, Supervision, Writing – original draft, Writing – review & editing. KS: Conceptualization, Data curation, Formal Analysis, Resources, Software, Validation, Visualization, Writing – review & editing. AL: Conceptualization, Data curation, Formal Analysis, Software, Validation, Visualization, Methodology, Writing – original draft. PS: Data curation, Formal Analysis, Writing – original draft. KP: Funding acquisition, Investigation, Project administration, Validation, Writing – original draft, Writing – review & editing.
The author(s) declare that no financial support was received for the research, authorship, and/or publication of this article.
The authors thank Dr. Yuvaraj Ananthanarayanan, Department of Zoology, Periyar University, for his valuable suggestions in nontarget earthworm species culturing, maintenance, and histopathology. We thank the Office of Research Administration, Chiang Mai University, Chiang Mai, Thailand, and the Department of Entomology and Plant Pathology at Chiang Mai University in Chiang Mai, Thailand (50200) for providing laboratory facilities and financial support.
The authors declare that the research was conducted in the absence of any commercial or financial relationships that could be construed as a potential conflict of interest.
All claims expressed in this article are solely those of the authors and do not necessarily represent those of their affiliated organizations, or those of the publisher, the editors and the reviewers. Any product that may be evaluated in this article, or claim that may be made by its manufacturer, is not guaranteed or endorsed by the publisher.
The Supplementary Material for this article can be found online at: https://www.frontiersin.org/articles/10.3389/fcimb.2023.1254475/full#supplementary-material
Agravante, A. S., Alviar, K. B., Ramirez, A. H. A., Yap, S. A. (2022). Biology of Spodoptera frugiperda (JE Smith)(Lepidoptera: Noctuidae) on selected weed species associated with corn in North Cotabato, Philippines. Philipp J. Sci. 151 (5), 2011–2020.
Ambethgar, V. (2009). Potential of entomopathogenic fungi in insecticide resistance management (IRM): A review. J. Biopest 2, 177–193.
Araújo, J. P., Hughes, D. P. (2016). Diversity of entomopathogenic fungi: which groups conquered the insect body? Adv. Gen. 94, 1–39. doi: 10.1016/bs.adgen.2016.01.001
Bagni, T., Siaussat, D., Maria, A., Couzi, P., Maïbèche, M., Massot, M. (2022). The impact of temperature on insecticide sensitivity depends on transgenerational effects. Sci. Total.Env 851, 158140. doi: 10.1016/j.scitotenv.2022.158140
Bai, J., Cao, J., Zhang, Y., Xu, Z., Li, L., Liang, L., et al. (2022). Comparative analysis of the immune system and expression profiling of Lymantria dispar infected by Beauveria bassiana. Pesticide Biochem. Physiol. 187, 105212. doi: 10.1016/j.pestbp.2022.105212
Balumahendhiran, K., Vivekanandhan, P., Shivakumar, M. S. (2019). Mosquito control potential of secondary metabolites isolated from Aspergillus flavus and Aspergillus fumigatus. Biocatal. Agric. Biotechnol. 21, 101334. doi: 10.1016/j.bcab.2019.101334
Bamisile, B. S., Akutse, K. S., Siddiqui, J. A., Xu, Y. (2021). Model application of entomopathogenic fungi as alternatives to chemical pesticides: Prospects, challenges, and insights for next-generation sustainable agriculture. Front. Plant Sci. 12, 741804. doi: 10.3389/fpls.2021.741804
Bapfubusa Niyibizi, I. A., Hanna, R., Kekeunou, S., Membang, G., Fiaboe, K. K. M., Mahot, H. C., et al. (2023). Potential of Cameroon-indigenous isolates of the entomopathogenic fungi Beauveria bassiana and Metarhizium anisopliae as microbial control agents of the flea beetle Nisotra uniformis. Biocontrol Sci. Technol. 33 no. 3, 226–240. doi: 10.1080/09583157.2023.2175784
Bara, G. T., Laing, M. D. (2020). Entomopathogens: potential to control thrips in avocado, with special reference to Beauveria bassiana. Horticul Rev. 47, 325–368. doi: 10.1002/9781119625407.ch7
Batta, Y. A. (2013). Efficacy of endophytic and applied Metarhizium anisopliae (Metch.) Sorokin (Ascomycota: Hypocreales) against larvae of Plutella xylostella L. (Yponomeutidae: Lepidoptera) infesting Brassica napus plants. Crop Protect 44, 128–134. doi: 10.1016/j.cropro.2012.11.001
Bommarco, R., Vico, G., Hallin, S. (2018). Exploiting ecosystem services in agriculture for increased food security. Glob. Food Secur 17, 57–63. doi: 10.1016/j.gfs.2018.04.001
Castro, B. M., Santos-Rasera, J. R., Alves, D. S., Marucci, R. C., Carvalho, G. A., Carvalho, H. W. (2021). Ingestion and effects of cerium oxide nanoparticles on Spodoptera frugiperda (Lepidoptera: Noctuidae). Environ. pollut. 279, 116905. doi: 10.1016/j.envpol.2021.116905
Colovic, M. B., Krstic, D. Z., Lazarevic-Pasti, T. D., Bondzic, A. M., Vasic, V. M. (2013). Acetylcholinesterase inhibitors: pharmacology and toxicology. Curr. neuropharmacology 11 (3), pp.315–pp.335. doi: 10.2174/1570159X11311030006
Dittmer, N. T., Kanost, M. R. (2010). Insect multicopper oxidases: diversity, properties, and physiological roles. Insect Biochem. Mol. Biol. 40 (3), 179–188. doi: 10.1016/j.ibmb.2010.02.006
Duchowicz, P., Goodarzi, M., Ocsachoque, M. A., Romanelli, G. P., Ortiz, E. D. V., Autino, J. C., et al. (2009). QSAR analysis on Spodoptera litura antifeedant activities for flavone derivatives. Sci. Total. Environ. 408, 277–285. doi: 10.1016/j.scitotenv.2009.09.041
Ellman, G. L., Courtney, K. D., Andres, V., Jr., Featherstone, R. M. (1961). A new and rapid colorimetric determination of acetylcholinesterase activity. Biochem. Pharmacol. 7, 88–95. doi: 10.1016/0006-2952(61)90145-9
European Food Safety Authority (EFSA), Kinkar, M., Delbianco, A., Vos, S. (2020). Pest survey card on Spodoptera frugiperda. EFSA Supp. Pub 17, 1895. doi: 10.2903/sp.efsa.2020.EN-1895
Fadiji, A. E., Babalola, O. O. (2020). Exploring the potentialities of beneficial endophytes for improved plant growth. Saudi J. Biol. Sci. 27 (12), .3622–.3633. doi: 10.1016/j.sjbs.2020.08.002
Fan, J., Wu, P., Tian, T., Ren, Q., Haseeb, M., Zhang, R. (2020). Potential distribution and niche differentiation of Spodoptera frugiperda in Africa. Insects 11, 383. doi: 10.3390/insects11060383
Firake, D. M., Behere, G. T. (2020). Natural mortality of invasive fall armyworm, Spodoptera frugiperda (JE Smith) (Lepidoptera: Noctuidae) in maize agroecosystems of northeast India. Biol. Con 148, 104303. doi: 10.1016/j.biocontrol.2020.104303
Goble, T. A., Dames, J. F., P Hill, M., Moore, S. D. (2010). The effects of farming system, habitat type and bait type on the isolation of entomopathogenic fungi from citrus soils in the Eastern Cape Province, South Africa. BioCon 55, 399–412. doi: 10.1007/s10526-009-9259-0
Han, J. H., Jin, B. R., Kim, J. J., Lee, S. Y. (2014). Virulence of entomopathogenic fungi Metarhizium anisopliae and Paecilomyces fumosoroseus for the microbial control of Spodoptera exigua. Mycobiol 42, 385–390. doi: 10.5941/MYCO.2014.42.4.385
Harrison, R. D., Thierfelder, C., Baudron, F., Chinwada, P., Midega, C., Schaffner, U., et al. (2019). Agro-ecological options for fall armyworm (Spodoptera frugiperda JE Smith) management: Providing low-cost, smallholder friendly solutions to an invasive pest. J. Environ. Manag 243, 318–330. doi: 10.1016/j.jenvman.2019.05.011
Islam, W., Adnan, M., Shabbir, A., Naveed, H., Abubakar, Y. S., Qasim, M., et al. (2021). Insect-fungal-interactions: A detailed review on entomopathogenic fungi pathogenicity to combat insect pests. Microb. Pathogen 159, 105122. doi: 10.1016/j.micpath.2021.105122
Javed, K., Javed, H., Mukhtar, T., Qiu, D. (2019). Efficacy of Beauveria bassiana and Verticillium lecanii for the management of whitefly and aphid. Pakistan J. Agric. Sci. 56 (3), 669–674. doi: 10.21162/PAKJAS/19.8396
Jing, D. P., Guo, J. F., Jiang, Y. Y., Zhao, J. Z., Sethi, A., He, K. L., et al. (2020). Initial detections and spread of invasive Spodoptera frugiperda in China and comparisons with other noctuid larvae in cornfields using molecular techniques. Insect Sci. 27, 780–790. doi: 10.1111/1744-7917.12700
Karthi, S., Senthil-Nathan, S., Kalaivani, K., Vasantha-Srinivasan, P., Chellappandian, M., Thanigaivel, A., et al. (2019). Comparative efficacy of two mycotoxins against Spodoptera litura Fab. And their non-target activity against Eudrilus eugeniae Kinb. Ecotoxicology Environ. Saf. 183, 109474. doi: 10.1016/j.ecoenv.2019109474
Karthi, S., Vaideki, K., Shivakumar, M. S., Ponsankar, A., Thanigaivel, A., Chellappandian, M., et al. (2018). Effect of Aspergillus flavus on the mortality and activity of antioxidant enzymes of Spodoptera litura Fab.(Lepidoptera: Noctuidae) larvae. Pesticide Biochem. Physiol. 149, 54–60. doi: 10.1016/j.pestbp.2018.05.009
Lee, G. S., Seo, B. Y., Lee, J., Kim, H., Song, J. H., Lee, W. (2020). First report of the fall armyworm, Spodoptera frugiperda (Smit)(Lepidoptera, Noctuidae), a new migratory pest in Korea. Korean. J. Appl. Entomol 59, 73–78. doi: 10.5656/KSAE.2020.02.0.006
Liu, H., Abrol, D. P., Shankar, U. (2012). “Microbial control of crop pests using entomopathogenic fungi,” in Integrated pest management: principles and practice (Jammu, India: CABI), 254.
Liu, H., Carvalhais, L. C., Crawford, M., Singh, E., Dennis, P. G., Pieterse, C. M., et al. (2017). Inner plant values: diversity, colonization and benefits from endophytic bacteria. Front. Microbiol. 8, 2552. doi: 10.3389/fmicb.2017.02552
Liu, T., Wang, J., Hu, X., Feng, J. (2020). Land-use change drives present and future distributions of Fall armyworm, Spodoptera frugiperda (JE Smith) (Lepidoptera: Noctuidae). Sci. Total. Environ. 706, 135872. doi: 10.1016/j.scitotenv.2019.135872
Montezano, D. G., Sosa-Gómez, D. R., Specht, A., Roque-Specht, V. F., Sousa-Silva, J. C., Paula-Moraes, S. D., et al. (2018). Host plants of Spodoptera frugiperda (Lepidoptera: Noctuidae) in the Americas. Afr. Entomol 26, 286–300. doi: 10.4001/003.026.0286
Oerke, E. C. (2006). Crop losses to pests. J. Agric. Sci. 144, 31–43. doi: 10.1017/S0021859605005708
Opisa, S., Du Plessis, H., Akutse, K. S., Fiaboe, K. K. M., Ekesi, S. (2018). Effects of Entomopathogenic fungi and Bacillus thuringiensis-based biopesticides on Spoladea recurvalis (Lepidoptera: Crambidae). J.App. Entomol 142, 617–626. doi: 10.1111/jen.12512
Paschapur, A., Subbanna, A. R. N. S., Singh, A. K., Jeevan, B., Stanley, J., Rajashekhar, H., et al. (2021). Unravelling the importance of metabolites from entomopathogenic fungi in insect pest management. In Microb. Sus lnsect Pest Manag 2, 89–120. doi: 10.1007/978-3-030-67231-7_5
Pelosi, C., Thiel, P., Bart, S., Amossé, J., Jean-Jacques, J., Thoisy, J. C., et al. (2021). The contributions of enchytraeids and earthworms to the soil mineralization process in soils with fungicide. Ecotoxicology 30, 1910–1921. doi: 10.1007/s10646-021-02452-z
Perumal, V., Kannan, S., Alford, L., Pittarate, S., Geedi, R., Elangovan, D., et al. (2023b). First report on the enzymatic and immune response of Metarhizium majus bag formulated conidia against Spodoptera frugiperda: An ecofriendly microbial insecticide. Front. Microbiol. 14, 1104079. doi: 10.3389/fmicb.2023.1104079
Perumal, V., Kannan, S., Alford, L., Pittarate, S., Mekchay, S., Reddy, G. V., et al. (2023a). Biocontrol effect of entomopathogenic fungi Metarhizium anisopliae ethyl acetate-derived chemical molecules: An eco-friendly anti-malarial drug and insecticide. Arch. Insect Biochem. Physiol. 114 (2), 1–19. doi: 10.1002/arch.22037
Pittarate, S., Rajula, J., Rahman, A., Vivekanandhan, P., Thungrabeab, M., Mekchay, S., et al. (2021). Insecticidal effect of zinc oxide nanoparticles against Spodoptera frugiperda under laboratory conditions. Insects 12, 1017. doi: 10.3390/insects12111017
Rajula, J., Pittarate, S., Suwannarach, N., Kumla, J., Ptaszynska, A. A., Thungrabeab, M., et al. (2021). Evaluation of native entomopathogenic fungi for the control of fall armyworm (Spodoptera frugiperda) in Thailand: A sustainable way for eco-friendly agriculture. J. Fungi 7, 1073. doi: 10.3390/jof7121073
Ramos, Y., Taibo, A. D., Jiménez, J. A., Portal, O. (2020). Endophytic establishment of Beauveria bassiana and Metarhizium anisopliae in maize plants and its effect against Spodoptera frugiperda (JE Smith)(Lepidoptera: Noctuidae) larvae. Egyptian J. Biol. Pest. Con 30, 1–6. doi: 10.1186/s41938-020-00223-2
Roberts, D. W., Humber, R. A. (1981). Entomogenous fungi. Biol. Conidial Fungi. 2, e236. doi: 10.1016/B978-0-12-179502-3.50014-5
Savary, S., Willocquet, L., Pethybridge, S. J., Esker, P., McRoberts, N., Nelson, A. (2019). The global burden of pathogens and pests on major food crops. Nat. Ecol. Evol. 3, 430–439. doi: 10.1038/s41559-018-0793-y
Scholte, E. J., Knols, B. G., Samson, R. A., Takken, W. (2004). Entomopathogenic fungi for mosquito control: a review. J. Insect Sci. 4, 19. doi: 10.1093/jis/4.1.19
Shahid, A. A., Rao, Q. A., Bakhsh, A., Husnain, T. (2012). Entomopathogenic fungi as biological controllers: new insights into their virulence and pathogenicity. Archi. Biol. Sci. 64, 21–42. doi: 10.2298/ABS1201021S
Shehzad, M., Tariq, M., Ali, Q., Aslam, A., Mukhtar, T., Akhtar, M. F., et al. (2022). Evaluation of insecticidal activity of Beauveria bassiana against different instar larvae of Plutella xylostella by using two different methods of application. Int. J. Trop. Insect Sci. 42, 1471–1476. doi: 10.1007/s42690-021-00665-7
Shehzad, M., Tariq, M., Mukhtar, T., Gulzar, A. (2021). On the virulence of the entomopathogenic fungi, Beauveria bassiana and Metarhizium anisopliae (Ascomycota: Hypocreales), against the diamondback moth, Plutella xylostella (L.)(Lepidoptera: Plutellidae). Egypt.J.Biol.Pest.Con 31, 1–7. doi: 10.1186/s41938-021-00428-z
Tambo, J. A., Kansiime, M. K., Mugambi, I., Rwomushana, I., Kenis, M., Day, R. K., et al. (2020). Understanding smallholders’ responses to fall armyworm (Spodoptera frugiperda) invasion: Evidence from five African countries. Sci. Total Environ. 740, 140015. doi: 10.1016/j.scitotenv.2020.140015
Thapa, S., Lv, M., Xu, H. (2017). Acetylcholinesterase: a primary target for drugs and insecticides. Mini Rev. medicinal Chem. 17 (17), pp.1665–1676. doi: 10.2174/1389557517666170120153930
Tondoh, E. J., Monin, L. M., Tiho, S., Csuzdi, C. (2007). Can earthworms be used as bio-indicators of land-use perturbations in semi-deciduous forest? Biol. Fertil. Soils. 43, 585–592.
Tu, C., Zhang, Y., Zhu, P., Sun, L., Xu, P., Wang, T., et al. (2023). Enhanced toxicity of entomopathogenic fungi Beauveria bassiana with bacteria expressing immune suppressive dsRNA in a leaf beetle. Pesticide Biochem. Physiol. 193, 105431. doi: 10.1016/j.pestbp.2023.105431
Van Asperen, K. (1962). A study of housefly esterases by means of a sensitive colorimetric method. J. Insect Physiol. 8, 401–416. doi: 10.1016/0022-1910(62)90074-4
Vivekanandhan, P., Bedini, S., Shivakumar, M. S. (2020a). Isolation and identification of entomopathogenic fungus from Eastern Ghats of South Indian forest soil and their efficacy as biopesticide for mosquito control. Parasitol. Int. 76, 102099. doi: 10.1016/j.parint.2020.102099
Vivekanandhan, P., Swathy, K., Bedini, S., Shivakumar, M. S. (2023). Bioprospecting of Metarhizium anisopliae derived crude extract: a ecofriendly insecticide against insect pest. Int. J. Trop. Insect Sci. 43, 429–440. doi: 10.1007/s42690-022-00935-y
Vivekanandhan, P., Swathy, K., Kalaimurugan, D., Ramachandran, M., Yuvaraj, A., Kumar, A. N., et al. (2020b). Larvicidal toxicity of Metarhizium anisopliae metabolites against three mosquito species and non-targeting organisms. PloS One 15 (5), e0232172. doi: 10.1371/journal.pone.0232172
Vivekanandhan, P., Swathy, K., Murugan, A. C., Krutmuang, P. (2022a). Insecticidal efficacy of Metarhizium anisopliae derived chemical constituents against disease-vector mosquitoes. J. Fungi 8, 300. doi: 10.3390/jof8030300
Vivekanandhan, P., Swathy, K., Shivakumar, M. S. (2022b). Identification of insecticidal molecule aucubin from Metarhizium anisopliae ethyl acetate crude extract against disease mosquito vector. Int. J. Trop. Insect Sci. 42, 3303–3318. doi: 10.1007/s42690-022-00828-0
Vivekanandhan, P., Swathy, K., Shivakumar, M. S. (2022c). Stability of insecticidal molecule aucubin and their toxicity on Anopheles stephensi, Aedes aEgypti, Culex quinquefasciatus and Artemia salina. Int. J. Trop. Insect Sci. 42, 3403–3417. doi: 10.1007/s42690-022-00849-9
Vos, R., Bellù, L. G. (2019). Global trends and challenges to food and agriculture into the 21st century. In: Sustain. Food Agriculture. Elsevier, 11–30. doi: 10.1016/B978-0-12-812134-4.00002-9
Wightman, J. A. (2018). Can lessons learned 30 years ago contribute to reducing the impact of the fall army worm Spodoptera frugiperda in Africa and India? Outlook Agri 47, 259–269. doi: 10.1177/0030727018814849
Xu, L., Zhang, Y., Zhang, S., Deng, J., Lu, M., Zhang, L., et al. (2018). Comparative analysis of the immune system of an invasive bark beetle, Dendroctonus valens, infected by an entomopathogenic fungus. Dev. Comp. Immunol. 88, 65–69. doi: 10.1016/j.dci.2018.07.002
Yigezu, G., Wakgari, M. (2020). Local and indigenous knowledge of farmers management practice against fall armyworm (Spodoptera frugiperda) (JE Smith) (Lepidoptera: Noctuidae): A review. J. Entomol. Zoo Stud. 8, 765–770.
Yuvaraj, A., Govarthanan, M., Karmegam, N., Biruntha, M., Kumar, D. S., Arthanari, M., et al. (2021a). Metallothionein dependent-detoxification of heavy metals in the agricultural field soil of industrial area: Earthworm as field experimental model system. Chemos 267, 129240. doi: 10.1016/j.chemosphere.2020.129240
Yuvaraj, A., Karmegam, N., Tripathi, S., Kannan, S., Thangaraj, R. (2020). Environment-friendly management of textile mill wastewater sludge using epigeic earthworms: Bioaccumulation of heavy metals and metallothionein production. J. Env. Manag 254, 109813. doi: 10.1016/j.jenvman.2019.109813
Yuvaraj, A., Thangaraj, R., Maheswaran, R. (2019). Decomposition of poultry litter through vermicomposting using earthworm Drawida sulcata and its effect on plant growth. Int.J.Env.Sci.Technol 16, 7241–7254. doi: 10.1007/s13762-018-2083-2
Yuvaraj, A., Thangaraj, R., Ravindran, B., Chang, S. W., Karmegam, N. (2021b). Centrality of cattle solid wastes in vermicomposting technology–A cleaner resource recovery and biowaste recycling option for agricultural and environmental sustainability. Env.Poll 268, 115688. doi: 10.1016/j.envpol.2020.115688
Keywords: fungal insecticide, acetylcholinesterase, α-carboxylesterase, and β-carboxylesterase, natural microbial insecticide, target specific, enzyme response
Citation: Vivekanandhan P, Swathy K, Lucy A, Sarayut P and Patcharin K (2023) Entomopathogenic fungi based microbial insecticides and their physiological and biochemical effects on Spodoptera frugiperda (J.E. Smith). Front. Cell. Infect. Microbiol. 13:1254475. doi: 10.3389/fcimb.2023.1254475
Received: 07 July 2023; Accepted: 20 November 2023;
Published: 11 December 2023.
Edited by:
Letian Xu, Hubei University, ChinaReviewed by:
Chinmay V. Tikhe, Johns Hopkins University, United StatesCopyright © 2023 Vivekanandhan, Swathy, Lucy, Sarayut and Patcharin. This is an open-access article distributed under the terms of the Creative Commons Attribution License (CC BY). The use, distribution or reproduction in other forums is permitted, provided the original author(s) and the copyright owner(s) are credited and that the original publication in this journal is cited, in accordance with accepted academic practice. No use, distribution or reproduction is permitted which does not comply with these terms.
*Correspondence: Perumal Vivekanandhan, dml2ZWthbmFuZGhhbi5wQGNtdS5hYy50aA==; Krutmuang Patcharin, cGF0Y2hhcmluLmtAY211LmFjLnRo
Disclaimer: All claims expressed in this article are solely those of the authors and do not necessarily represent those of their affiliated organizations, or those of the publisher, the editors and the reviewers. Any product that may be evaluated in this article or claim that may be made by its manufacturer is not guaranteed or endorsed by the publisher.
Research integrity at Frontiers
Learn more about the work of our research integrity team to safeguard the quality of each article we publish.