- Department of Ophthalmology, Visual and Anatomical Sciences, Wayne State University, School of Medicine, Detroit, MI, United States
Purpose: In vivo data indicate that mouse corneas exposed to PM10 showed early perforation and thinning after infection with Pseudomonas aeruginosa. To understand the mechanisms underlying this finding, we tested the effects of PM10 and the mitochondria targeted anti-oxidant SKQ1 in immortalized human corneal epithelial cells (HCET) that were challenged with Pseudomonas aeruginosa strain 19660.
Methods: Mouse corneas were infected with strain 19660 after a 2 week whole-body exposure to PM10 or control air and assessed by clinical scores, slit lamp photography and western blot. HCET were exposed to 100μg/ml PM10 for 24h before challenge with strain 19660 (MOI 20). A subset of cells were pre-treated with 50nM SKQ1 for 1h before PM10 exposure. Phase contrast microscopy was used to study cell morphology, cell viability was measured by an MTT assay, and ROS by DCFH-DA. Levels of pro-inflammatory markers and anti-oxidant enzymes were evaluated by RT-PCR, western blot and ELISA. Reduced glutathione (GSH) and malondialdehyde (MDA) levels were evaluated by assay kits.
Results: In vivo, whole body exposure to PM10 vs. control air exposed mouse corneas showed early perforation and/or corneal thinning at 3 days post infection, accompanied by increased TNF-α and decreased SOD2 protein levels. In vitro, PM10 induced a dose dependent reduction in cell viability of HCET and significantly increased mRNA levels of pro-inflammatory molecules compared to control. Exposure to PM10 before bacterial challenge further amplified the reduction in cell viability and GSH levels. Furthermore, PM10 exposure also exacerbated the increase in MDA and ROS levels and phase contrast microscopy revealed more rounded cells after strain 19660 challenge. PM10 exposure also further increased the mRNA and protein levels of pro-inflammatory molecules, while anti-inflammatory IL-10 was decreased. SKQ1 reversed the rounded cell morphology observed by phase contrast microscopy, increased levels of MDA, ROS and pro-inflammatory molecules, and restored IL-10.
Conclusions: PM10 induces decreased cell viability, oxidative stress and inflammation in HCET and has an additive effect upon bacterial challenge. SKQ1 protects against oxidative stress and inflammation induced by PM10 after bacterial challenge by reversing these effects. The findings provide insight into mechanisms underlying early perforation and thinning observed in infected corneas of PM10 exposed mice.
Introduction
Airborne particulate matter (PM) with an aerodynamic diameter <10 microns (PM10) is a complex mixture of solids and aerosols and a major component of air pollution (Crinnion, 2017). Exposure to PM10 has been linked to adverse health effects (Pope et al., 2002) and increased mortality (Pope and Dockery, 2006), chronic cardiopulmonary diseases (Nurkiewicz et al., 2006; Liu et al., 2009; Miller et al., 2009; Zhang et al., 2021), chronic obstructive pulmonary disease (Churg et al., 2003), diabetes (Wang et al., 2014) and cancer (Brunekreef and Holgate, 2002). However, few studies have investigated its effects on the eye, although the ocular surface is continuously exposed to the pollutants (Hao et al., 2022). Recently, epidemiological studies have linked PM10 exposure to an increased rate of outpatient visits for eye discomfort (Chang et al., 2012), elevated risk of Sjogren’s syndrome (Zhang et al., 2022) and worsened tear film stability in patients with dry eye disease (Kim et al., 2020).
PM can disrupt the corneal epithelial barrier function (Ko et al., 2021), thus rendering the ocular surface more prone to infections. The effects of PM10 on ocular infections have not been well investigated and there is little information on how PM10 affects disease progression and severity of ocular infections. A study from Argentina demonstrated that urban particulate matter exacerbated inflammation in a mouse model of acute herpes simplex keratitis (Sendra et al., 2021). An epidemiological study from South Korea, showed that exposure to high concentration of PM10 correlated with increased outpatient visits for ocular diseases, including emergency room visits for keratitis (Lee et al., 2018). And it is well known that dry eye increases the propensity for microbial infection (Klopfer, 1989; Torricelli et al., 2014; Mo et al., 2019; Manisalidis et al., 2020).
Microbial keratitis is a vision threatening disorder and is related to several risk factors, which include extended use of soft contact lenses (Stapleton and Carnt, 2012; Hazlett et al., 2021), ocular surface disease (O'Neill et al., 2014), ocular surgery (Keay et al., 2006) and immunosuppression (Otri et al., 2013). Pathogens such as Pseudomonas aeruginosa (P. aeruginosa) are often the causative agent in eye infections (Hilliam et al., 2020). P. aeruginosa is a gram negative bacterium and a leading cause of contact lens–induced microbial keratitis (Green et al., 2008). If untreated, these infections can lead to ocular pain, stromal destruction, corneal thinning, and/or perforation, eventually leading to vision loss (Ekanayaka et al., 2016). A critical antimicrobial mechanism employed by host immune cells to combat microbial infection is oxidative burst, a process which involves production of reactive oxygen (ROS) and reactive nitrogen (RNS) species (Lambeth, 2004). However, excessive production of ROS/RNS by activated immune cells generates a cytotoxic environment that contributes to inflammatory responses which are noxious to organs (Novaes et al., 2019). PM10 has been shown to exert its toxic effects by inducing free radical production and inflammation in cornea (Yoon et al., 2018; Somayajulu et al., 2023). Mitochondria are the main sources of free radical generation (Kowalczyk et al., 2021) and we have recently shown that PM10 exposure causes mitochondrial dysfunction by elevating superoxide free radicals and decreasing ATP levels (Somayajulu et al., 2023). Studies are ongoing in search of potential therapeutics to protect against oxidative and inflammatory damage induced by PM10. One such therapeutic compound is SKQ1 (10-(6′-plastoquinonyl) decyltriphenylphosphonium), a mitochondria-specific anti-oxidant that accumulates in the inner mitochondrial membrane (Skulachev, 2013) and has proven effective against oxidative stress in animal models of ischemia/reperfusion (Kezic et al., 2016), aging (Skulachev, 2011) and neurodegeneration (Genrikhs et al., 2015). It has been formulated as an eye drop (Visomitin) to prevent anesthesia-induced dry eye syndrome in patients after long-term general anesthesia or ocular surgery (Zernii et al., 2017). Recently, the use of Visomitin for the treatment of dry eye disease (Brzheskiy et al., 2015) has been tested in a phase 3 clinical trial in the USA (Ousler et al., 2022) with good outcome.
The purpose of this study is to determine whether exposure of the eye to PM10 has an adverse effect on the development and progression of P. aeruginosa induced keratitis. Because we have observed that P. aeruginosa infection of the mouse cornea after PM10 exposure caused earlier corneal perforation and/or thinning, we sought to determine the mechanism underlying this observation in vitro using immortalized human corneal epithelial cells. We also used this in vitro system to test the beneficial effects of SKQ1.
Materials and methods
Mice
Female C57BL/6 mice, 8 weeks of age, were purchased from the Jackson Laboratory (Bar Harbor, ME) and housed in accordance with the National Institutes of Health guidelines. Mice were humanely treated and in compliance with both the ARVO Statement for the Use of Animals in Ophthalmic and Vision Research and the Institutional Animal Care and Use Committee of Wayne State University (IACUC-21-09-4042).
Whole body exposure to PM10
Experiments in this study were performed with PM10 purchased from the National Institute of Standards and Technology, (NIST) (Standard Reference Material (SRM) 2787). A whole-body exposure chamber (CH Technologies, Westwood, NJ) was used for this study as previously described (Somayajulu et al., 2023). Briefly, mice were exposed to control air or an acute, high dose of PM10 (500µg/m3) for 2 weeks for 3h/day/5days/week and rested on the weekends. The dosage used in this study was based upon mean PM10 concentrations measured in the winter, ranging as high as 494µg/m3 in 5 Chinese cities (Cao et al., 1988).
Bacterial culture and infection
As previously described, P. aeruginosa strain ATCC (American Type Culture Collection [ATCC] Manassas, VA, USA) 19660, a cytotoxic strain (Ekanayaka et al., 2016) was grown in peptone tryptic soy broth (PTSB) medium in a rotary shaker water bath at 37°C and 150 rpm for 18h to an optical density (measured at 540 nm) between 1.3 and 1.8. Bacterial cultures were centrifuged at 5,500g for 10 min. Bacterial pellets were washed once with sterile saline, recentrifuged, resuspended, and diluted in sterile saline. Mice exposed to PM10 and control air were infected with strain 19660 24h after the last chamber exposure. Mice were infected as described previously (Moon et al., 1988; Ekanayaka et al., 2018). Briefly, mice were anesthetized with ether and placed under a stereoscopic microscope at 40 × magnification. The left cornea was scarified, and 5μl containing 1 × 106 colony-forming units (CFU)/μl of the bacterial suspension was applied topically.
Ocular response to bacterial infection
As previously described, clinical scores were designated as follows: 0 = clear or slight opacity, partially or fully covering the pupil; +1 = slight opacity, fully covering the anterior segment; +2 = dense opacity, partially or fully covering the pupil; +3 = dense opacity, covering the entire anterior segment; and +4 = corneal perforation or phthisis (Moon et al., 1988). Each mouse was scored in masked fashion at 1, and 3 days post infection (p.i.) for statistical comparison and photographed (3 days p.i.) with a slit lamp to demonstrate disease.
Tissue culture and treatments
HCET cells (HCE-2 [50.B1], ATCC, Gaithersburg, MA) were cultured in Keratinocyte-serum free (KSF) medium (Gibco, Grand Island, NY) with 5ng/ml human recombinant EGF, 0.05mg/ml bovine pituitary extract, 0.005mg/ml insulin, and 500ng/ml hydrocortisone as previously described (Somayajulu et al., 2023). Cells were treated with PM10 (0, 25, 50, 100, 200, 500, 800 and 1200µg/ml for 24h for the MTT assay. For all other experiments, cells were incubated with 100μg/ml (per cell viability data from dose curve; 75-80% viability) PM10 at 37°C and 5% CO2 for 24hr. To investigate the combined effects of PM10 on P. aeruginosa infected cells, a subset of cells were challenged with strain 19660 at a multiplicity of infection (MOI) of 20 for 3h. To assess the effects of SKQ1 (BOC Sciences, Shirley, NY, USA), another subset of cells were incubated with 50nM SKQ1, 1h before PM10 exposure (Somayajulu et al., 2023) and then challenged with strain19660 at similar MOI. Another group of HCET were challenged with strain 19660 at a MOI of 20 for 3h to assess the effects of bacteria alone on these cells. Phase contrast microscopy was used to photograph cell preparations using a Leica EL 6000 microscope (Deerfield, IL, USA). All the images were acquired at the same magnification and processed similarly.
Cell viability assays
An MTT 3-(4, 5-dimethylthiazol-2-yl)-2,5-diphenyltetrazolium bromide (ThermoFisher Scientific, Grand Island, NY) assay was used to test the effects of PM10 on HCET viability as reported before (Somayajulu et al., 2023). Briefly, 15,000 HCET cells were seeded in a 96 well plate, treated with 0, 25, 50, 100, 200, 500, 800 and 1200µg/mL PM10 for 24h or PM10 (100µg/ml) ± 50nM SKQ1 ± strain 19660 as described above in “Tissue culture and treatments”. At the end of the treatment, 5mg/ml MTT reagent was added to each well and incubated at 37°C for 4h and media removed. Dimethyl sulfoxide (DMSO, 100%) was added (50µl/well) and optical density was read at 540nm using a SpectraMax M5 microplate reader (Molecular Devices, Sunnyvale, CA). Data are shown as % cell viability + SD. To confirm the MTT data, a trypan blue exclusion cell viability assay was also performed using Countess cell counting chamber slides (Invitrogen, Carlsbad, CA) per the manufacturer’s protocol. Briefly, cells were treated as mentioned in “Tissue culture and treatments” and trypsinized 24h post treatment, centrifuged, resuspended in KSF media and incubated 1:1 with trypan blue. A 10μl aliquot was placed on the chamber slide and viable cells were counted using a Countess automated cell counter (Invitrogen, Carlsbad, CA). Data are shown as % cell viability + SD.
Western blot analysis
HCET were treated with 100µg/ml PM10 ± strain 19660 ± SKQ1, washed with ice-cold 0.1M PBS (pH 7.4), lysed in RIPA buffer with protease and phosphatase inhibitors (SantaCruz Biotech, Dallas, TX), incubated on ice 20min, centrifuged at 12,000g at 4°C for 10min and supernatant collected. Total protein was determined from the supernatants using a BCA protein kit (ThermoFisher Scientific). Briefly, samples (20μg) were run on SDS-PAGE in Tris-glycine-SDS buffer and electro-blotted onto nitrocellulose membranes (BioRad). After blocking for 1h in 5% MTBST (Tris Buffer saline containing 0.05% Tween 20 (TBST) and 5% nonfat milk), membranes were probed with primary antibodies: rabbit anti-mouse pNFκB, NFκB, iNOS, COX2 and TNF-α (1:1000; Cell Signaling Technology, Danvers, MA), in 2% MTBST overnight at 4°C. The membranes were then washed three times with TBST and incubated with HRP-conjugated anti-rabbit secondary antibody (1:2000; Cell Signaling Technology) diluted in 5% MTBST at room temperature for 2h. Bands were developed with Supersignal West Femto Chemiluminescent Substrate (ThermoFisher Scientific), visualized using an iBright™ CL1500 Imaging System (ThermoFisher Scientific), and normalized to β-tubulin (1:1000; Abcam, Waltham, MA) and intensity quantified using ImageJ software. Data are shown as mean integrated density values (IDV) + SD.
RT-PCR
Total RNA was isolated from cells treated with 100µg/ml PM10, ± strain 19660 ± SKQ1 and control HCET (n=3/group/treatment) using RNA STAT-60 (Tel-Test, Friendswood, TX) per the manufacturer’s instructions as reported before (Huang et al., 2005). Briefly, reverse transcription was performed with one μg of RNA from each sample using Moloney-murine leukemia virus (M-MLV) reverse transcriptase (Invitrogen, Carlsbad, CA) to produce a cDNA template. A 2μl aliquot of diluted cDNA (1:20 in DEPC-treated water) was used for the RT-PCR reaction. SYBR green/fluorescein PCR master mix (Bio-Rad Laboratories, Richmond, CA) and primer concentrations of 10μM were used in a total 10μl volume. After a pre-programmed hot start cycle (3min at 95°C), PCR amplification was repeated for 45 cycles with parameters: 15s at 95°C and 60s at 60°C. Levels of high mobility group box 1 (HMGB1), inducible nitric oxide synthase (iNOS), cyclooxygenase 2 (COX2), interleukin (IL)-1β, IL-6, IL-10, toll-like receptor (TLR)4, tumor necrosis factor (TNF)-α, nicotinamide adenine dinucleotide phosphate (NADPH) quinone dehydrogenase 1 (NQO1), glutathione peroxidase (GPX4), glutamate-cysteine ligase modifier subunit (GCLM), and catalase were tested by real-time RT-PCR (CFX Connect real-time PCR detection system; Bio-Rad Laboratories). The fold differences in gene expression were calculated using the formula: Fold change= 2-ΔΔCT and expressed as relative mRNA levels + SD. Primer pair sequences used are shown in Table 1.
ROS assay
Cells (n=3/group/treatment) were treated as described in tissue culture and lysed in RIPA buffer with protease and phosphatase inhibitors (SantaCruz Biotech, Dallas, TX). A previously published method was used to detect ROS (Carion et al., 2018). Briefly, 8μl of cell lysates were incubated in a reaction buffer [130mM KCl, 5mM MgCl2, 20mM NaH2PO4, 20mM Tris-HCl, pH 7.4, 30mM glucose, 7.5uM 2’,7’-dichlorofluorescein diacetate (DCFH-DA)] for 1h at 37°C. Fluorescence was measured at 485nm (excitation) and 590nm (emission) using SpectraMax Gemini EM spectrophotometer (Molecular Devices, Sunnyvale, CA). The level of ROS is proportional to fluorescence intensity. As a control, 8μl of homogenate from each group was also incubated with the reaction buffer without DCFH-DA. The protein concentration of the supernatant was determined by the Bradford method (Bio-Rad, Hercules, CA), using bovine serum albumin (Sigma-Aldrich, St. Louis, MO) as the standard (Somayajulu et al., 2023). Data were normalized to protein and are represented as mean + SD.
MitoSOX staining
Mitochondrial ROS was analyzed by fluorescence with a MitoSOX assay (ThermoFisher Scientific) according to the manufacturer’s protocol. Briefly, HCET cells were plated onto coverslips, and treated with 100µg/mL PM10 for 6h. Cells were washed with PBS and incubated with 10µM MitoSOX at 37°C for 10 min. Cells were then washed twice with PBS and imaged live using a Zeiss Apotome equipped with an AxioCam HRM camera and processed with photoshop (version 7.0.1). The level of ROS is proportional to fluorescence intensity. All images were acquired at the same magnification and processed similarly.
GSH assay
Total GSH levels were analyzed by a glutathione assay kit (Cayman Chemical, Ann Arbor, MI) per the manufacturer’s protocol and as described before (Kimura and Kimura, 2004). Briefly, cells (n=3/group/treatment) treated with 100µg/ml PM10 ± strain 19660 ± SKQ1 were harvested in 500µl of ice cold 50mM MES (2-(N-morpholino) ethanesulphonic acid) containing 1mM EDTA, homogenized and centrifuged at 10,000g at 4°C for 10min and supernatant collected. Total GSH levels were determined by Ellman’s reagent using a standard curve per manufacturer’s protocol and then normalized to total protein in each sample. Final GSH levels were expressed as mean + SD.
MDA assay
Lipid peroxidation was examined by measuring the malondialdehyde (MDA) levels by a TBARS assay kit (Cayman Chemical, Ann Arbor, MI) per the manufacturer’s protocol. Briefly, cells (n=3/group/treatment) treated with 100µg/ml PM10 ± strain 19660 ± SKQ1 were harvested in 500µl of ice cold PBS with protease inhibitor, homogenized and centrifuged at 10,000g at 4°C for 10mins and supernatant collected. The supernatants were incubated with thiobarbituric acid (TBA) for 1h at 90°C under acidic conditions. The MDA-TBA adduct formed was measured calorimetrically at 53-540nm. MDA levels were calculated using a standard curve per manufacturer’s protocol and then normalized to total protein in each sample. MDA levels were expressed as mean + SD
ELISA
An ELISA kit was used to measure protein levels of IL-10 (Duoset ELISA kit, R&D systems, Minneapolis, MN). Briefly, HCET cells were treated as mentioned above and cells (n=3/group/treatment) from the control or 100µg/ml PM10 ± strain 19660 ± SKQ1 were lysed in PBS containing 0.1% Tween 20 and protease inhibitors. All assays were run per the manufacturers’ protocol and data were expressed as mean + SD.
Statistical analysis
In vivo comparison of clinical scores between two groups at each time was tested by the Mann-Whitney U test. For in vitro studies, significance of RT-PCR between control vs. PM10 treated groups was determined by a Student’s t-test. To demonstrate significance between 3 or more groups (in vitro), a one-way ANOVA followed by the Bonferroni’s multiple comparison test was used for MTT, GSH, MDA assays, ROS measurements, ELISA and western blots. Data were considered significant at p<0.05. All experiments were repeated at least once to ensure reproducibility and data are shown as mean ± SEM (in vivo) and mean +SD (in vitro).
Results
PM10 exposure in vivo affects ocular response to infection
Figures 1A–C show slit lamp photographs of normal cornea (A), cornea exposed to control air (B) and cornea exposed to PM10 before infection (C). Corneas (A-C) appear clear. Figures 1D–F are slit lamp photographs of typical eyes from P. aeruginosa infected mice. PM10 exposure (500μg/m3) resulted in earlier perforation (Figure 1E) or thinning (Figure 1F) vs. control air exposure that showed dense opacity (Figure 1D) over the entire anterior segment. Clinical scores (Figure 1G) showed disease in both control air and PM10 exposed infected eyes at 3 days p.i. Although not significant, differences were observed between the two infected groups with a trend of worsening disease in the PM10 exposed group (greater than +3 and thinning of the cornea). No differences were observed between infected eyes in control air and PM10 exposed groups at day 1 p.i. (Figure 1G) where the score was +1 for both groups. Figures 1H, I revealed that downstream protein, pro-inflammatory TNF-α is significantly (p<0.05) increased, and the anti-oxidant enzyme, SOD2 is decreased significantly (p<0.001) after PM10 exposure and infection vs. control air, setting the stage for the following studies.
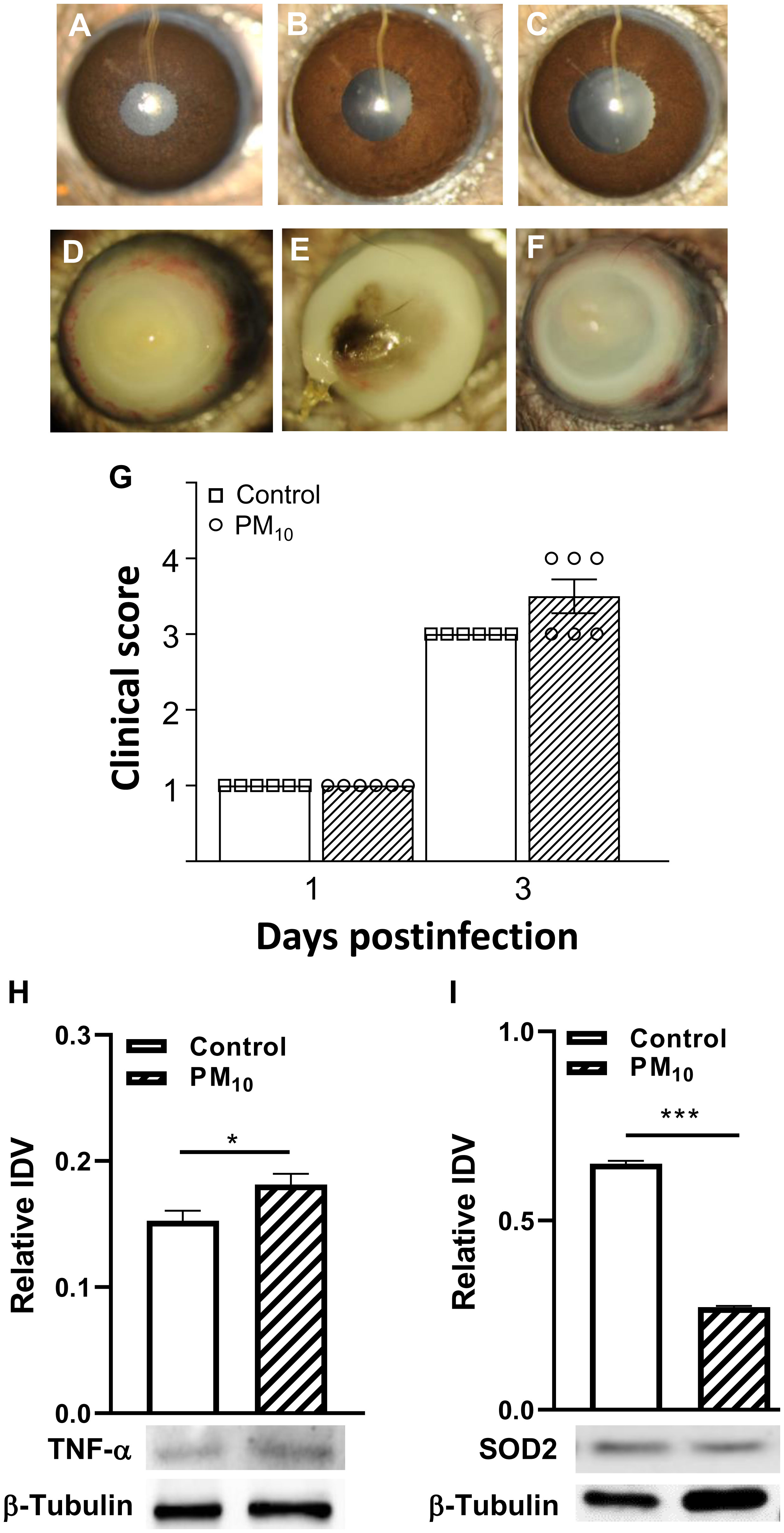
Figure 1 Effects of control air and PM10 on mouse corneas infected with strain 19660. (A-C) shows normal cornea (A), control air cornea (B) and PM10 exposed cornea (C) are all clear. Slit lamp photographs from control air exposed (D) showed opacity over the entire cornea, while PM10 exposed showed perforation (E), and central corneal thinning (F) at 3 days p.i. (n=5/group/time). Clinical scores (G) at 1 and 3 days p.i. No significant differences in clinical scores were observed between control and PM10 exposed corneas at 1 day p.i. However, at 3 days p.i., the PM10 exposed group had 50% more corneas with a higher clinical score. PM10 exposure and infection caused a significant increase in protein levels of pro-inflammatory modulator TNF-α (H) and a significant decrease in the anti-oxidant enzyme SOD2 (I). *p<0.05, ***p<0.001.
PM10 exposure decreases cell viability, anti-oxidant enzyme levels, increases ROS and inflammation in vitro in HCET
In vitro, the effects of PM10 on HCET were evaluated by testing cell viability (Figure 2A), ROS levels (Figure 2B) and pro-inflammatory modulators (Figure 2C). HCET exposed to PM10 for 24h showed a concentration dependent decrease (p<0.001) in live cells (Figure 2A). At concentrations of 25-100μg/ml, 75-90% of the cells were viable compared to controls. At 1200μg/ml, the highest concentration tested, less than 50% remained viable. Figure 2B shows PM10 significantly reduced the mRNA levels of anti-oxidant enzymes: NQO1 (p<0.001), GPX4 (p<0.001), catalase (p<0.001) and GCLM (p<0.001). Figure 2C indicates increased mRNA levels of pro-inflammatory markers in HCET exposed to PM10 for HMGB1 (p<0.001), iNOS (p<0.001), COX2 (p<0.001), IL-1β (p<0.05) and TLR4 (p<0.001 vs. control HCET. ROS production by mitochondria visualized by fluorescence microscopy using MitoSOX red dye is shown in Figure 2D. Data clearly show increased fluorescence indicating higher superoxide produced by mitochondria of HCET exposed to PM10 compared to control HCET.
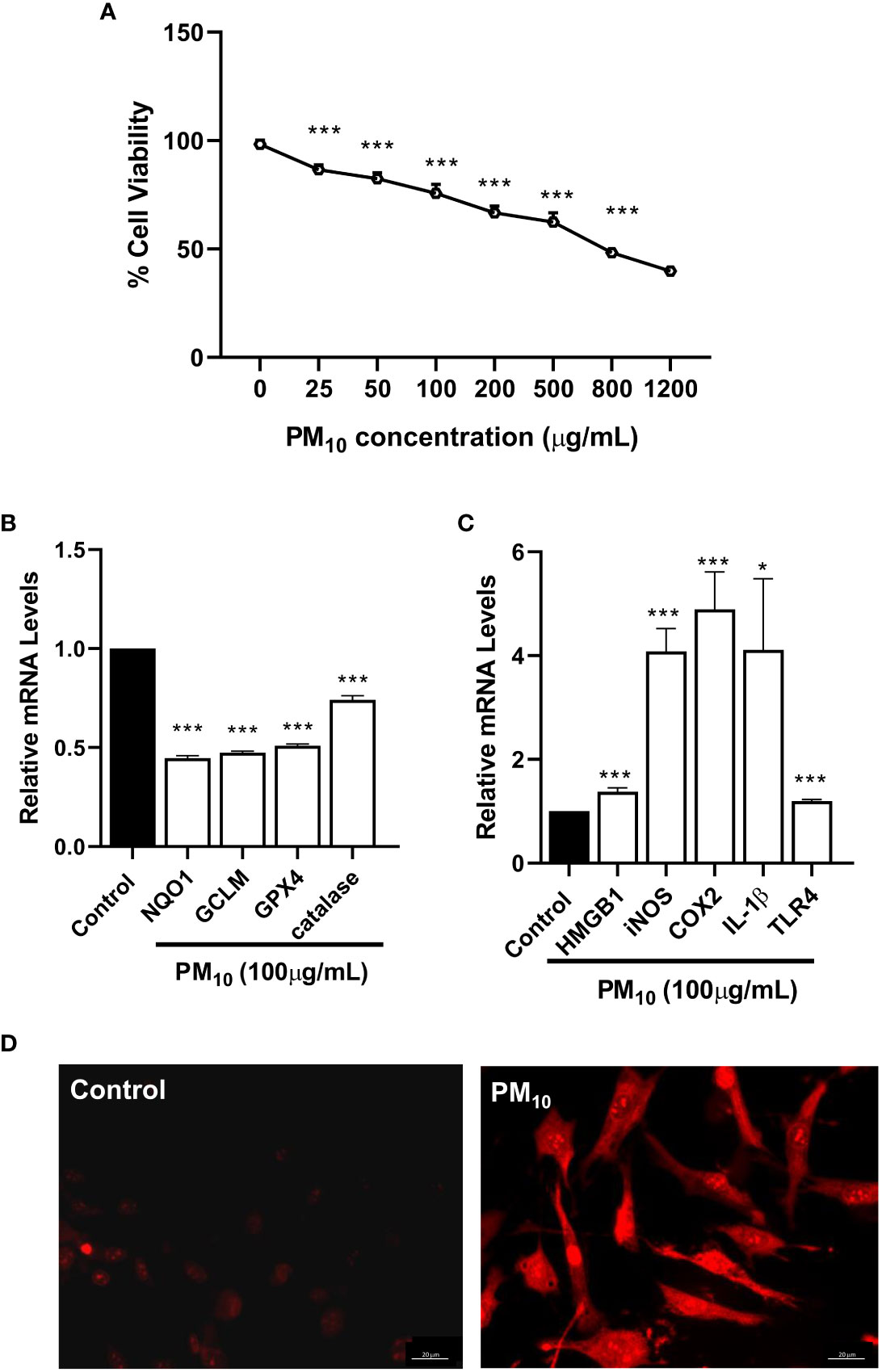
Figure 2 In vitro effects of PM10 on HCET: cell viability, anti-oxidant enzymes, inflammation and mitochondrial ROS. (A). Cells exposed to PM10 for 24h showed a concentration dependent decrease in cell viability measured by MTT assay. At 1200μg/ml, the highest concentration tested, approximately 50% remained viable. (B). RT-PCR showed that PM10 exposure (100μg/ml) significantly reduced mRNA levels of antioxidant enzymes compared to control after 24h. (C). RT-PCR showed that PM10 exposure (100μg/ml) significantly increased mRNA levels of pro-inflammatory molecules compared to control after 24h. (D). MitoSOX staining shows PM10 exposed (100μg/ml) cells have increased fluorescence vs. control indicating higher mitochondrial ROS levels. Scale bar = 20μm. Data are expressed as mean + SD. (*p<0.05, ***p<0.001, n=3).
PM10 effects cell viability of HCET challenged with P. aeruginosa
The effects of PM10 on the response of HCET to P. aeruginosa were examined by phase contrast microscopy and cell viability tested. Phase contrast microscopy (Figure 3A) clearly shows that controls have prominent nuclei and appear spindle shaped. Cells exposed to PM10 appear to be enlarged and flattened with enlarged nuclei. After P. aeruginosa challenge, a small number of rounded cells (indicated by arrows) were seen. When PM10 exposed cells, were challenged with the bacteria, more cells appeared rounded and nuclei were difficult to observe. Cells pre-treated with SKQ1, a mitochondria targeted antioxidant, appeared spindle-shaped and similar to the control group, with fewer rounded cells. Cell viability data (Figure 3B) indicate that compared to controls, exposure to PM10 or challenge with strain 19660 significantly lowered HCET viability (p<0.001). Significant additional reduction in cell viability was observed in PM10 exposed cells challenged with strain 19660 compared to cells in control, PM10 or bacteria challenged groups (p<0.001). SKQ1 pre-treatment did not protect against the PM10 induced additional loss in cell viability after bacterial challenge. Similar effects of PM10 and SKQ1 were observed on cells challenged with strain 19660 and cell viability was analyzed with the trypan blue dye exclusion test shown in Supplementary Figure 1.
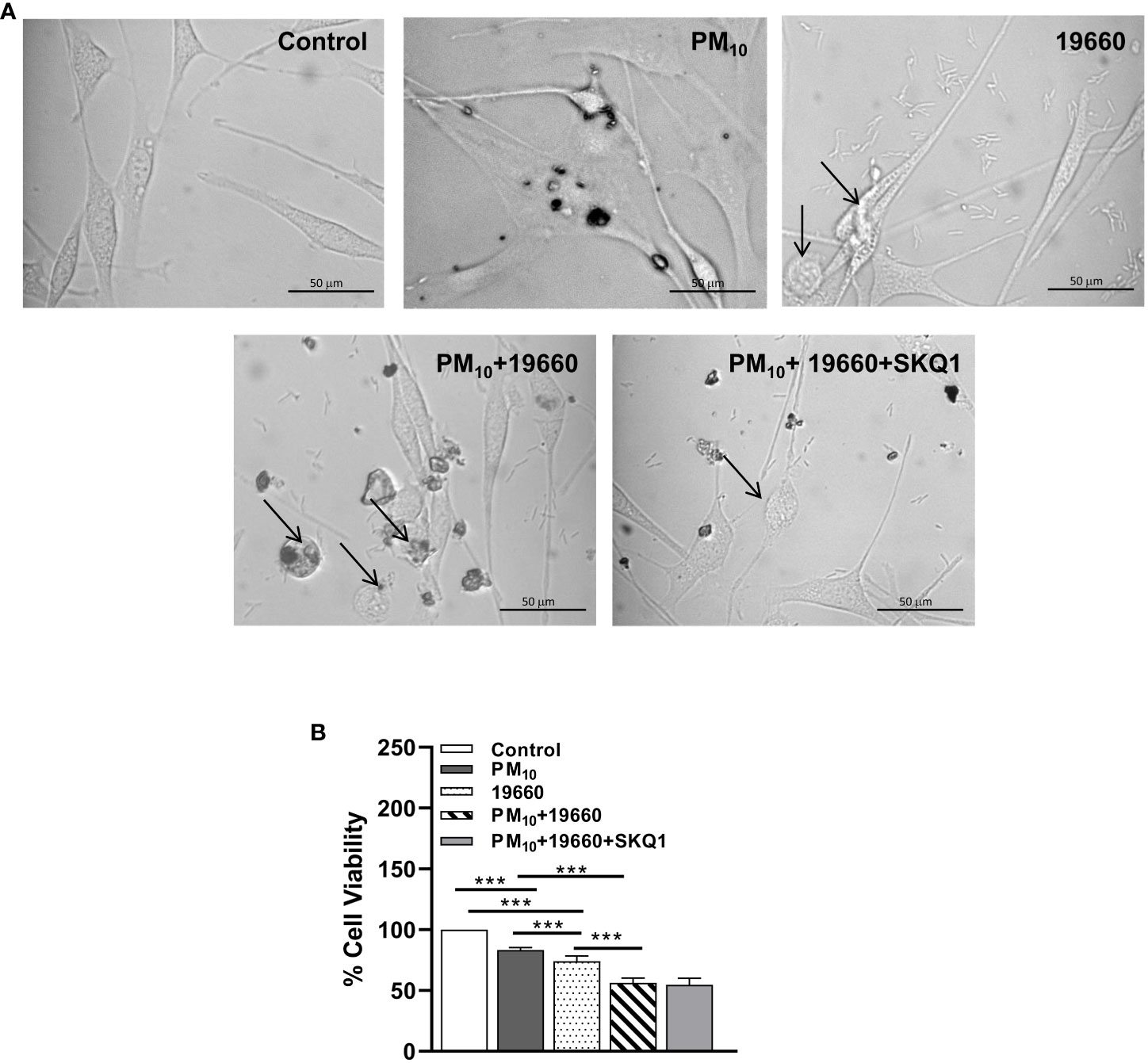
Figure 3 In vitro effects of strain 19660 after PM10 exposure on HCET: morphology and cell viability. (A). Phase contrast images of cells challenged with strain 19660 after PM10 exposure and treatment with SKQ1. Cells in the media control group appear spindle shaped with prominent nuclei. Cells exposed to PM10 appear flattened and enlarged. More cells appear rounded and thinned upon 19660 challenge after PM10 exposure compared to cells exposed to bacteria alone (arrows). SKQ1 pre-treated cells appear spindle shaped with prominent nuclei and have fewer rounded cells, similar to control. (B). Cells exposed to PM10 (100μg/ml) and then challenged with strain 19660 show a significant reduction in cell viability (MTT assay) as compared to control cells. SKQ1 pre-treatment (50nM) doesn’t restore cell viability. Scale bar=50μm. Data are expressed as mean + SD. (***p<0.001, n=3).
PM10 upregulates P. aeruginosa induced oxidative stress
We have previously shown that PM10 exposure induces oxidative stress in HCET (Somayajulu et al., 2023). To assess the effects of PM10 on P. aeruginosa induced oxidative stress the levels of ROS (Figure 4A), lipid peroxidation end product MDA (Figure 4B) and GSH (Figure 4C) were analyzed. Data show that compared to control, ROS levels (Figure 4A) were significantly elevated (p<0.01) in strain 19660 challenged cells. PM10 exposure significantly upregulated this increase (p<0.001) in ROS levels in cells challenged with bacteria. Pre-treatment with SKQ1 significantly reduced ROS levels (p<0.001) in bacteria challenged cells exposed to PM10. Figure 4B shows significantly elevated levels of MDA (p<0.001 after cells were challenged with strain 19660 vs. control. Exposure to PM10 before bacterial challenge caused an additional increase in MDA levels compared to bacterial challenge alone (p<0.001). SKQ1 pretreatment reduced the MDA levels significantly (p<0.001) in PM10 exposed cells challenged with strain 19660. The effects of SKQ1 on MDA levels in cells exposed to only PM10 or bacteria alone are shown in Supplementary Figure 2A. Figure 4C demonstrates that GSH levels were significantly reduced in cells challenged with bacteria vs. control (p<0.001). PM10 exposure prior to bacterial challenge caused an additional reduction in GSH levels (p<0.001) vs. bacteria alone. SKQ1 pre-treatment was able to significantly elevate (p<0.001). PM10 mediated additional loss in GSH levels. The effects of SKQ1 on GSH levels in cells exposed to only PM10 or bacteria alone are shown in Supplementary Figure 2A.
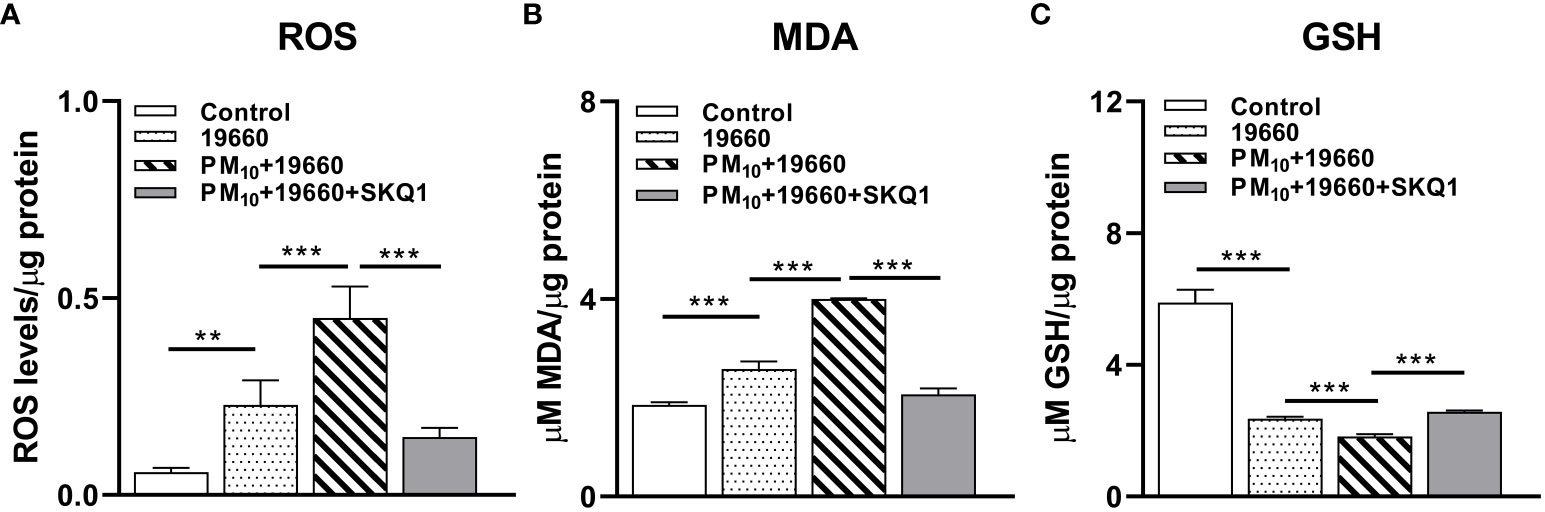
Figure 4 HCET challenged with ATCC 19660 after PM10 exposure show exacerbated oxidative stress: ROS, MDA and GSH levels. (A). Elevated ROS level in cells challenged with ATCC 19660 after PM10 exposure was significantly reduced by SKQ1. (B). MDA levels were significantly reduced by SKQ1 in cells challenged with ATCC 19660 after exposure to PM10. (C). Lowered GSH levels in cells challenged with ATCC 19660 after PM10 exposure were significantly rescued by SKQ1. Data are expressed as mean + SD. (**p<0.01, ***p<0.001, n=3).
PM10 affects the levels of anti-oxidant enzymes in cells challenged with P. aeruginosa
mRNA levels of anti-oxidant enzymes measured by RT-PCR are represented in Figure 5. Cells challenged with bacteria vs. control showed a significant increase in the levels of GPX4 (A, p<0.001), NQO1 (B, p<0.001), GCLM (C, p<0.001). However, mRNA levels of catalase were significantly lower in bacteria challenged cells vs. controls (D, p<0.001). When P. aeruginosa challenge was accompanied by PM10 exposure, a significant downregulation was observed in the expression levels of GPX4 (A, p<0.001), NQO1 (B, p<0.001), GCLM (C, p<0.01) compared to bacteria alone. PM10 exposure did not affect catalase mRNA levels in strain19660 challenged cells vs. those challenged with bacteria alone. Cells treated with SKQ1 prior to PM10 exposure and bacterial challenge had significantly higher levels of only NQO1 (B, p<0.001), GCLM (C, p<0.01) and catalase (D, p<0.01).
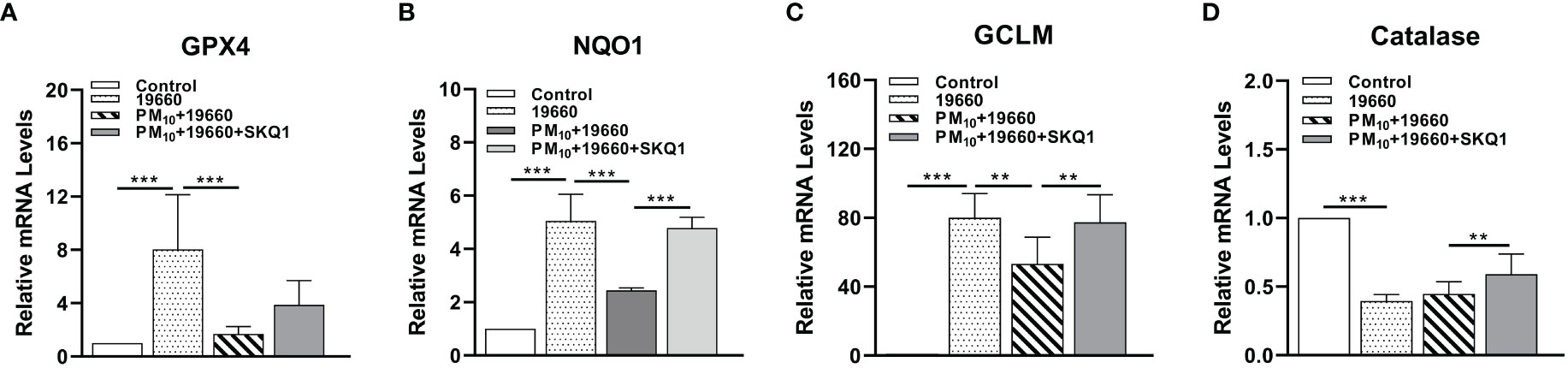
Figure 5 Effects of strain19660, PM10 and SKQ1 on mRNA levels of antioxidant enzymes. RT-PCR showed significantly lowered mRNA levels for GPX4 (A), NQO1 (B), GCLM (C), and catalase (D) in cells challenged with strain 19660 after PM10 exposure compared to cells challenged with bacteria alone. These levels were significantly restored by SKQ1. Data are expressed as mean + SD. (**p<0.01, ***p<0.001, n=3).
PM10 induces increased cell inflammation responses in cells challenged with P. aeruginosa
mRNA levels of pro-inflammatory markers measured by RT-PCR are represented in Figure 6 and protein levels analyzed by western blot are shown in Figures 7A-D. Cells challenged with bacteria vs. control demonstrated a significant increase in the level of only TLR4 (A, p<0.05), TNF-α (B, p<0.01), IL-1β (C, p<0.01), iNOS (E, p<0.001), IL-6 (F, p<0.001) and HMGB1 (G, p<0.001). A significantly upregulation in the expression level of TLR4 (A, p<0.001), TNF-α (B, p<0.001), IL-1β (C, p<0.001), COX2 (D, p<0.05), iNOS (E, p<0.05), IL-6 (F, p<0.01) and HMGB1 (G, p<0.01) was observed in the P. aeruginosa challenged group that was exposed to PM10, compared to the group challenged with bacteria alone. Cells treated with SKQ1 before PM10 exposure and bacterial challenge had significantly lower levels of TLR4 (A, p<0.001), TNF-α (B, p<0.001), IL-1β (C, p<0.01), COX2 (D, p<0.01), iNOS (E, p<0.001), IL-6 (F, p<0.001) and HMGB1 (G, p<0.001) compared vs. those without SKQ1. Figure 7A shows western blot analysis of PM10 exposed cells challenged with bacteria in the presence and absence of SKQ1. Relative IDV values (7B-D) indicate significantly elevated protein levels of p-NFκB (B, p<0.001), COX2 (C, p<0.001) and iNOS (D, p<0.001) in cells challenged with bacteria vs. control. PM10 exposure of bacteria challenged cells caused a further upregulation in the levels of p-NFκB (B, p<0.001), COX2 (C, p<0.01) but not iNOS. Cells pre-treated with SKQ1 before PM10 and bacteria showed a significant reduction in the levels of p-NFκB (A, p<0.001), COX2 (C, p<0.001) and iNOS (D, p<0.05) compared to those without SKQ1.
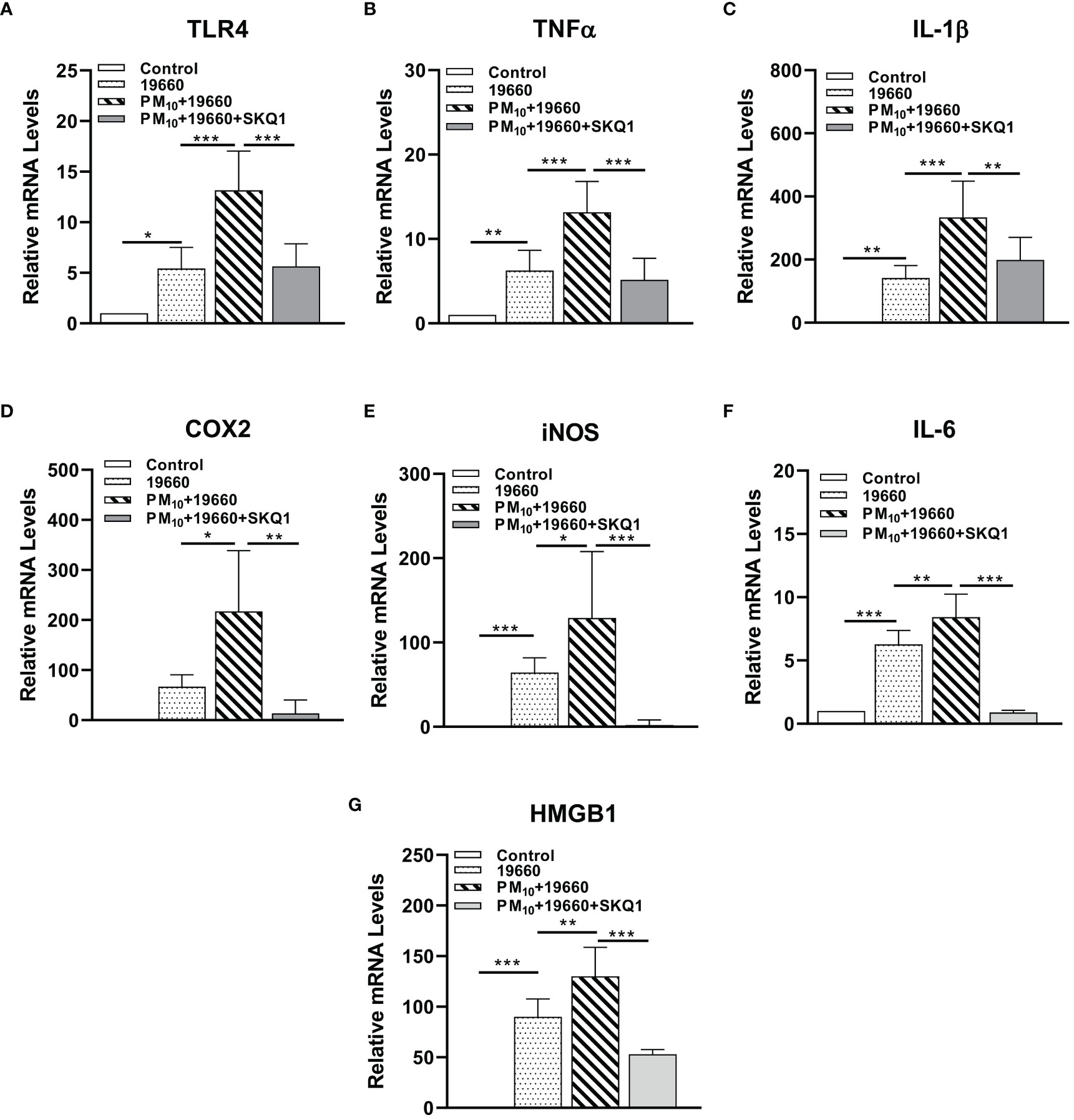
Figure 6 mRNA levels of pro-inflammatory markers are increased in cells challenged with strain 19660 after PM10 exposure: protection by SKQ1. RT-PCR showed significantly elevated mRNA levels for TLR4 (A), TNF-α (B), IL-1β (C), COX2 (D), iNOS (E), IL-6 (F) and HMGB1 (G) in cells challenged with ATCC 19660 after PM10 exposure compared to cells challenged with bacteria alone. SKQ1 pre-treatment reversed these effects. Data are expressed as mean + SD. (*p<0.05, **p<0.01, ***p<0.001, n=3).
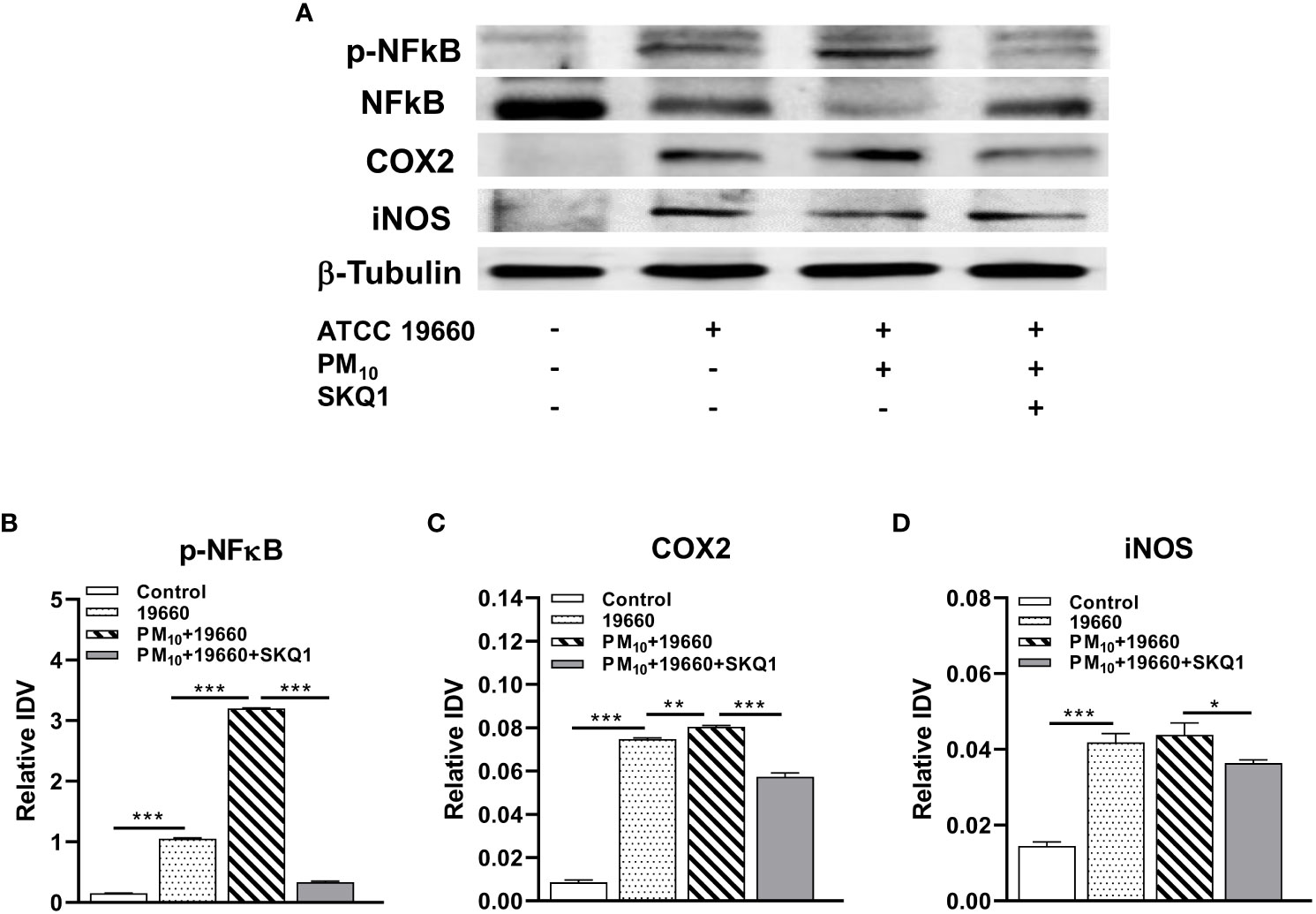
Figure 7 Protein levels of pro-inflammatory markers are increased in cells challenged with strain 19660 after PM10 exposure: protection by SKQ1. (A). Western blots used to determine the levels of pNFκB, NFκB, COX2 and iNOS. Densitometry showed significantly elevated protein levels for pNFκB (B), COX2 (C) and iNOS (D) in cells challenged with strain 19660 after PM10 exposure, which were significantly lowered by SKQ1. Data are expressed as mean + SD. (*p<0.05, **p<0.01, ***p<0.001, n=3).
IL-10 levels are lowered by PM10
IL-10 is an anti-inflammatory cytokine which plays an important role in infection by regulating the immune response against pathogens and thus avoiding damage to the host (Saraiva and O'Garra, 2010). The mRNA and protein levels of IL-10 in cells exposed to PM10 and challenged with bacteria are represented in Figures 8A, B. Figure 8A shows that compared to controls, cells exposed to PM10 or challenged with bacteria alone and bacteria plus PM10 had significantly reduced (p<0.001) IL-10 mRNA levels. A slight but not significant decline in IL-10 mRNA levels was observed in cells challenged with bacteria after PM10 exposure vs. cells challenged with bacteria alone. SKQ1 pre-treatment before PM10 and bacteria showed a significant (p<0.001) restoration of IL-10 mRNA compared to without SKQ1. Figure 8B demonstrates the levels of IL-10 protein in different treatment groups. Cells exposed to only PM10 or challenged with 19660 alone, showed a significant reduction (p<0.001) in IL-10 protein compared to control. Cells challenged with 19660 after PM10 exposure also displayed a significant decline (p<0.001) in IL-10 protein compared to control cells, PM10 exposed cells, and cells challenged with 19660 alone. SKQ1 pre-treatment significantly reversed (p<0.01) the decline in IL-10 protein in cells exposed to PM10 before strain 19660 challenge.
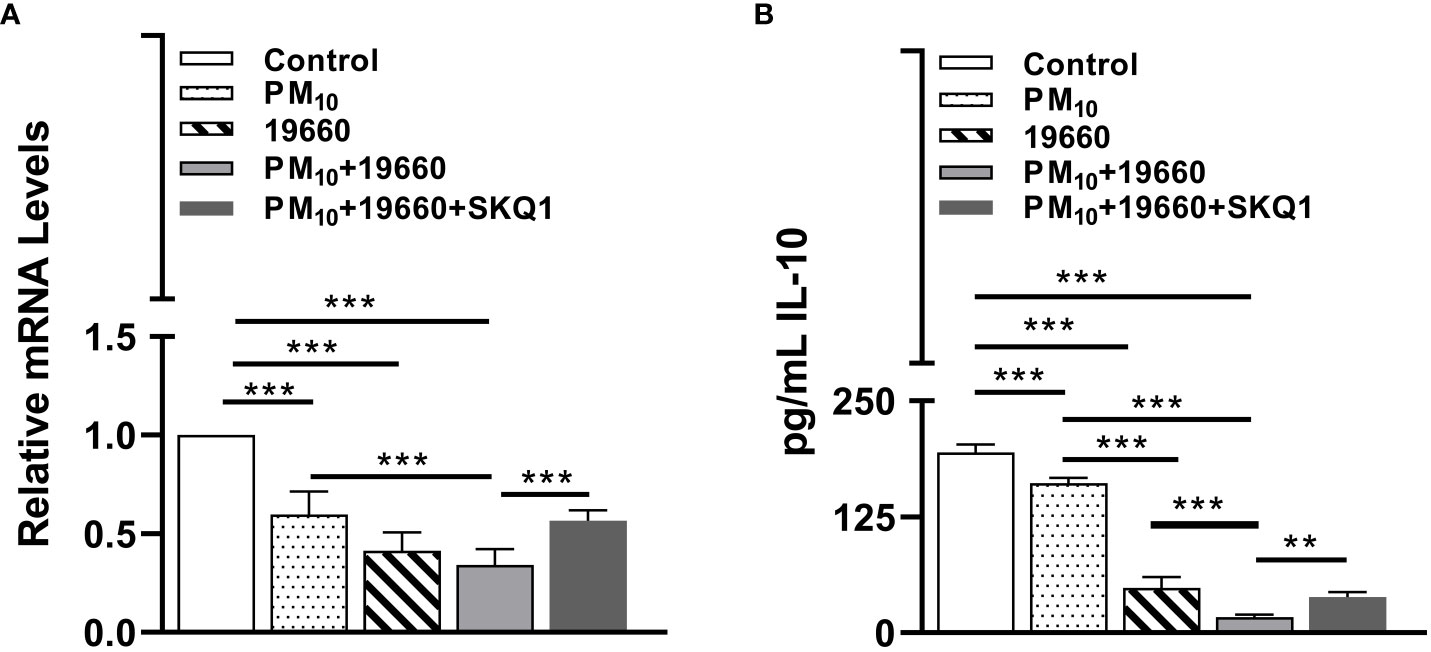
Figure 8 IL-10 levels are decreased in cells challenged with strain 19660 after PM10 exposure and SKQ1 reverses this effect. RT-PCR showed mRNA (A) and ELISA showed protein (B) levels of IL-10 were significantly lowered in cells challenged with strain 19660 after PM10 exposure compared to cells challenged with bacteria alone. SKQ1 reversed the loss of IL-10 at both mRNA and protein level. Data are expressed as mean + SD. (**p<0.01, ***p<0.001, n=3).
Discussion
The world’s population, increasingly living in urban areas, is anticipated to reach 68% by 2050 (Sendra et al., 2021) and exposure to air pollution in the urban setting is a major risk factor for disease. One of the major components of air pollution is particulate matter (PM), which is a mixture of liquid and solid particles of various sizes and chemical properties (Li et al., 2022). The negative impact of particulate matter on the human body is mainly from PM2.5 and PM10 (Li et al., 2022), with adverse events linked to cardiovascular and pulmonic diseases. Exposure to PM is also linked to dry eye (Li et al., 2017), ocular discomfort, (Novaes et al., 2010), abnormalities in tear film, reduced tear break-up time and other subclinical alterations of the ocular surface (Novaes et al., 2007). In particular, as part of the ocular surface, the cornea which must remain optically clear for good vision, is constantly exposed to the environment and serves as a barrier against the entry of harmful pathogens (Zhou et al., 2022). Exposure to particulate has been shown to compromise the barrier function in a reconstructed human corneal epithelial model by reducing the levels of proteins such as occluding zonules that are a component of the cells tight junction complex, required for maintaining tissue integrity (Alfuraih et al., 2020). Additionally, PM induced compromise in barrier function has been shown to promote P. aeruginosa infection in other tissues exposed to particulates, such as the lung (Liu et al., 2019). Whether or not exposure of the eye to particulates has a detrimental impact on the development/progression of ocular infectious diseases such as P. aeruginosa induced bacterial keratitis are currently unknown and is the purpose of this study.
In the current study, we analyzed the impact of acute exposure to PM10 on P. aeruginosa induced keratitis and also studied the ameliorative effects of SKQ1, a mitochondrial anti-oxidant. We used a whole-body exposure chamber to subject mice to a dose of PM10 of 500μg/m3 before bacterial challenge. Normally, in this model, mouse corneas perforate by day five (Hazlett et al., 2001), however, in this study we observed that they were thinned and/or perforated by day 3 after PM10 exposure. This was accompanied by significantly elevated levels of TNF-α and reduced levels of SOD2 protein that are indicative of inflammation and oxidative stress. These findings are consistent with a mouse study of herpes simplex virus (HSV)-1 induced keratitis, where mouse corneas exposed to polluted air from the city of Buenos Aires showed a more severe disease outcome than controls, including increased corneal opacity and pro-inflammatory cytokine production (Sendra et al., 2021).
This study extends previous work in which we examined the effects of topical application of PM2.5 (400μg/ml) post infection with P. aeruginosa on the mouse cornea (Somayajulu et al., 2020). Application of PM2.5 6h post infection resulted in severe corneal disease, including early perforation and worsened clinical scores at 2 days post infection (Somayajulu et al., 2020). However, the PM2.5 model had drawbacks including that it was a drop method vs. whole-body exposure and we could find no ties to oxidative stress as a contributory factor. Therefore, the model did little to clarify any mechanisms underlying the effects of particulates on keratitis and how they possibly were connected to oxidative stress. Since the main hypothesis of this study is that PM10 induces oxidative stress, which leads to inflammation that makes the cornea more susceptible to infection this was a major drawback. To uncover the mechanism underlying early perforation in the mouse cornea by PM10, we turned to an in vitro model which can be better controlled, where HCET were exposed to PM10 prior to bacterial challenge with strain 19660.
The toxic effects of PM10 include: cell viability loss, increased oxidative stress, inflammation and impaired barrier function and have been well established in different in vitro models (Li et al., 2008; Chirino et al., 2010; Yoon et al., 2018; Ko et al., 2020; Aghapour et al., 2022). Our analysis showed that PM10 caused a dose dependent loss in cell viability, reduced anti-oxidant enzyme levels, and increased ROS and pro-inflammatory modulators. Our data are consistent with a previous study which demonstrated that PM10 isolated from road dust in Seoul, South Korea decreased cell viability and increased ROS production and inflammation in HCET (Yoon et al., 2018). Other studies have also confirmed these toxic effects of PM10 in human lung epithelial cells (Chirino et al., 2010) and alveolar epithelial cells (Ko et al., 2020). Oxidative stress is caused by an imbalance between ROS production and anti-oxidant defenses (Pizzino et al., 2017). The detrimental effects of PM10 on the anti-oxidant defense system (Hatzis et al., 2006) have also been well established (Chirino et al., 2010). Studies have shown that PM10 lowered GSH levels in lung epithelial cells, which resulted in increased lipid peroxidation and protein oxidation (Chirino et al., 2010). Additionally, more recently, we have shown that PM10 reduces Nrf2 levels in both primary and immortalized human corneal epithelial cells (Somayajulu et al., 2023). Nrf2 is a master regulator of the anti-oxidant defense mechanism and is activated upon oxidative stress, in turn activating many genes, including NQO1, GCLM, and GPX4 (Pardo et al., 2020) that encode for anti-oxidant enzymes. When we measured the levels of these enzymes, we observed that PM10 reduced their levels in HCET, further strengthening our hypothesis that PM10 induces oxidative stress in these cells. PM-induced oxidative stress also increases inflammatory modulators such as TNF-α, IL-1β, IL-6, IL-8, and COX2 (Wang et al., 2017; Yoon et al., 2018; Radan et al., 2019; Ko et al., 2020; Pintha et al., 2021). When we measured this class of modulators, we observed a PM10 mediated upregulation of IL-1β, iNOS, COX2, HMGB1 and TLR4 in HCET. Our data suggest that PM10 exposure causes a pro-oxidant and pro-inflammatory milieu in the cells. To further test that PM10 exacerbates the effects of bacterial infection; we challenged cells with P. aeruginosa after PM10 exposure and also tested the effects of the anti-oxidant SKQ1 in HCET.
We observed that PM10 exposure before bacterial challenge served to further elevate P. aeruginosa induced loss in cell viability, and oxidative stress (increased ROS production, lipid peroxidation and lowered GSH levels). Furthermore, we found that the anti-oxidant SKQ1 reversed these effects on oxidative stress by reducing ROS levels and lipid peroxidation while restoring GSH levels. These findings are consistent with former reports demonstrating that urban particulate matter further escalates P. aeruginosa (strain PAO1) induced oxidative stress and ROS production (Chen et al., 2018) in human bronchial epithelial cells, which was reversed by N-acetylcysteine (Chen et al., 2018), which has anti-inflammatory and anti-oxidant capabilities. However, P. aeruginosa produces many toxic compounds, such as pyocyanin that can directly oxidize GSH and reduce its levels, producing ROS in airway epithelial cells (O'Malley et al., 2004). These findings suggest that cells infected with bacteria are already under oxidative stress and the presence of PM10 further escalates it. In response to oxidative stress, the cells deploy anti-oxidant defense mechanism based on enzymatic components which serve to protect them from ROS mediated damage (Deponte, 2013). In this study, the measurement of anti-oxidant enzyme levels showed increased GPX4, NQO1 and GCLM levels after bacterial challenge, which is indicative of the normal response to oxidative stress. However, cells exposed to PM10 before bacterial challenge showed a marked reduction of these anti-oxidant enzymes, suggesting that PM10 impaired the anti-oxidant response causing additional oxidative stress in these cells.
One of the responses to PM-mediated oxidative stress is inflammation (Wang et al., 2017). When we analyzed different immune modulators and cytokines in response to bacterial challenge after PM10 exposure, we saw an upregulation of pro-inflammatory cytokines IL-6, IL-1β, and TNF-α and other modulators: NF-κB, COX-2, iNOS and TLR4. Our data are similar to previous studies in alveolar macrophages stimulated with PM2.5 and challenged with P. aeruginosa (PAO1) that showed an upregulation of similar pro-inflammatory cytokines such TNF-α, IL-6, and NF-κB pathway-related proteins (Liu et al., 2019). We also observed that while PM10 increased the levels of pro-inflammatory cytokines, it reduced the levels of the anti-inflammatory cytokine IL-10 in cells challenged with bacteria. These findings are consistent with former reports in human bronchial epithelial cells exposed to urban particulate matter before infection with bacteria that caused an upregulation of pro-inflammatory cytokine IL-8, while downregulating anti-inflammatory cytokine IL-13 (Chen et al., 2018). Our hypothesis that oxidative stress induced by PM10 mediates inflammation is further supported by the fact that SKQ1 abrogated the increased levels of TNF-α, IL-6 and IL-1β, and restored IL-10 levels in cells challenged with bacteria and PM10. The ameliorative effects of SKQ1, a mitochondria targeted anti-oxidant against PM10 mediated toxicity, have been previously demonstrated by us in both primary and immortalized human corneal epithelial cells (Somayajulu et al., 2023).
We have used PM10 by direct application into the culture medium where we have established a functional model. However, the generalizability of the findings may prove different compared to real world scenarios in which the whole body is exposed to the particulate. The data implies that exposure to PM10 renders the eye more susceptible to infection and thus the caution is for the public to be aware that not only respiratory disease is possible with exposure. that the eye is made more vulnerable to pathogens as well. In fact exposure below 50 μg/m3 (Mu et al., 2022) is a recommended to reduce health risks whereas amounts of 494-1200 μg/m3 are observed in China and India. Thus, the mechanism of PM10 activity enhancing P. aeruginosa keratitis have been shown in a well-controlled in vitro model, but the data need to be verified by in vivo studies which are underway, to provide further understanding of the adverse effects of PM10 on keratitis.
In conclusion, PM10 impairs the anti-oxidant defense mechanism leading to increased oxidative stress, aggravating inflammation and triggering increased susceptibility to infection. The adverse effects of oxidative stress are reversed by using the anti-oxidant SKQ1 which targets mitochondrial ROS. These findings provide insight into the underlying mechanisms of early perforation and increased disease severity observed in P. aeruginosa infected corneas exposed to PM10.
Data availability statement
The raw data supporting the conclusions of this article will be made available by the authors, without undue reservation.
Ethics statement
The animal study was approved by IACUC review board Wayne State University School of Medicine. The study was conducted in accordance with the local legislation and institutional requirements.
Author contributions
Conceptualization: LH; methodology: MS, SM, and LH; formal analysis: MS, RW, FM, and SM; investigation: MS, SM, RW, and FM; resources: LH; data curation: MS; writing—original draft preparation: MS and LH; writing—review and editing: MS, SM, and LH; visualization: MS and SM; supervision: LH; project administration: LH; funding acquisition: LH. All authors contributed to the article and approved the submitted version.
Funding
This study was supported by the funding from National Institutes of Health grants: R01EY016058, R01EY035231 and P30EY004068 from the National Eye Institute (LDH), and a Research to Prevent Blindness (RPB) unrestricted grant to the Department of Ophthalmology, Visual and Anatomical Sciences and Kresge Eye Institute.
Conflict of interest
The authors declare that the research was conducted in the absence of any commercial or financial relationships that could be construed as a potential conflict of interest.
Publisher’s note
All claims expressed in this article are solely those of the authors and do not necessarily represent those of their affiliated organizations, or those of the publisher, the editors and the reviewers. Any product that may be evaluated in this article, or claim that may be made by its manufacturer, is not guaranteed or endorsed by the publisher.
Supplementary material
The Supplementary Material for this article can be found online at: https://www.frontiersin.org/articles/10.3389/fcimb.2023.1240903/full#supplementary-material
References
Aghapour, M., Ubags, N. D., Bruder, D., Hiemstra, P. S., Sidhaye, V., Rezaee, F., et al. (2022). Role of air pollutants in airway epithelial barrier dysfunction in asthma and COPD. Eur. Respir. Rev. 31 (163), 210112. doi: 10.1183/16000617.0112-2021
Alfuraih, S., Barbarino, A., Ross, C., Shamloo, K., Jhanji, V., Zhang, M., et al. (2020). Effect of high glucose on ocular surface epithelial cell barrier and tight junction proteins. Invest. Ophthalmol. Vis. Sci. 61 (11), 3. doi: 10.1167/iovs.61.11.3
Brunekreef, B., Holgate, S. T. (2002). Air pollution and health. Lancet 360, 1233–1242. doi: 10.1016/s0140-6736(02)11274-8
Brzheskiy, V. V., Efimova, E. L., Vorontsova, T. N., Alekseev, V. N., Gusarevich, O. G., Shaidurova, K. N., et al. (2015). Results of a multicenter, randomized, double-masked, placebo-controlled clinical study of the efficacy and safety of visomitin eye drops in patients with dry eye syndrome. Adv. Ther. 32 (12), 1263–1279. doi: 10.1007/s12325-015-0273-6
Cao, S. R., Chen, Y. Y., Ren, G. Y., Li, S. M. (1988). Analysis of organic and inorganic components of inhalable particles in the atmosphere. BioMed. Environ. Sci. 1 (2), 130–137.
Carion, T. W., Ebrahim, A. S., Kracht, D., Agrawal, A., Strand, E., Kaddurah, O., et al. (2018). Thymosin beta-4 and ciprofloxacin adjunctive therapy improves pseudomonas aeruginosa-induced keratitis. Cells 7 (10), 145. doi: 10.3390/cells7100145
Chang, C. J., Yang, H. H., Chang, C. A., Tsai, H. Y. (2012). Relationship between air pollution and outpatient visits for nonspecific conjunctivitis. Invest. Ophthalmol. Vis. Sci. 53 (1), 429–433. doi: 10.1167/iovs.11-8253
Chen, X., Liu, J., Zhou, J., Wang, J., Chen, C., Song, Y., et al. (2018). Urban particulate matter (PM) suppresses airway antibacterial defence. Respir. Res. 19 (1), 5. doi: 10.1186/s12931-017-0700-0
Chirino, Y. I., Sánchez-Pérez, Y., Osornio-Vargas, A. R., Morales-Bárcenas, R., Gutiérrez-Ruíz, M. C., Segura-García, Y., et al. (2010). PM (10) impairs the antioxidant defense system and exacerbates oxidative stress driven cell death. Toxicol. Lett. 193 (3), 209–216. doi: 10.1016/j.toxlet.2010.01.009
Churg, A., Brauer, M., del Carmen Avial-Casado, M., Forteoul, T. I., Wright, J. L. (2003). Chronic exposure to high levels of particulate air pollution and small airway remodeling. Environ. Health Perspect. 111, 714–718. doi: 10.1289/ehp.6042
Crinnion, W. (2017). Particulate matter is a surprisingly common contributor to disease. Integr. Med. (Encinitas) 16 (4), 8–12.
Deponte, M. (2013). Glutathione catalysis and the reaction mechanisms of glutathione-dependent enzymes. Biochim. Biophys. Acta 1830 (5), 3217–3266. doi: 10.1016/j.bbagen.2012.09.018
Ekanayaka, S. A., McClellan, S. A., Barrett, R. P., Hazlett, L. D. (2018). Topical glycyrrhizin is therapeutic for pseudomonas aeruginosa keratitis. J. Ocul Pharmacol. Ther. 34 (3), 239–249. doi: 10.1089/jop.2017.0094
Ekanayaka, S. A., McClellan, S. A., Barrett, R. P., Kharotia, S., Hazlett, L. D. (2016). Glycyrrhizin reduces HMGB1 and bacterial load in pseudomonas aeruginosa keratitis. Invest. Ophthalmol. Vis. Sci. 57 (13), 5799–5809. doi: 10.1167/iovs.16-20103
Genrikhs, E. E., Stelmashook, E. V., Popova, O. V., Kapay, N. A., Korshunova, G. A., Sumbatyan, N. V., et al. (2015). Mitochondria-targeted antioxidant SkQT1 decreases trauma-induced neurological deficit in rat and prevents amyloid-β-induced impairment of long-term potentiation in rat hippocampal slices. J. Drug Target 23 (4), 347–352. doi: 10.3109/1061186X.2014.997736
Green, M., Apel, A., Stapleton, F. (2008). Risk factors and causative organisms in microbial keratitis. Cornea 27 (1), 22–27. doi: 10.1097/ICO.0b013e318156caf2
Hao, R., Zhang, M., Zhao, L., Liu, Y., Sun, M., Dong, J., et al. (2022). Impact of air pollution on the ocular surface and tear cytokine levels: A multicenter prospective cohort study. Front. Med. (Lausanne) 9. doi: 10.3389/fmed.2022.909330
Hatzis, C., Godleski, J. J., González-Flecha, B., Wolfson, J. M., Koutrakis, P. (2006). Ambient particulate matter exhibits direct inhibitory effects on oxidative stress enzymes. Environ. Sci. Technol. 40 (8), 2805–2811. doi: 10.1021/es0518732
Hazlett, L. D., McClellan, S., Barrett, R., Rudner, X. (2001). B7/CD28 costimulation is critical in susceptibility to Pseudomonas aeruginosa corneal infection: a comparative study using monoclonal antibody blockade and CD28-deficient mice. J. Immunol. 166 (2), 1292–1299. doi: 10.4049/jimmunol.166.2.1292
Hazlett, L. D., McClellan, S., Somayajulu, M., Bessert, D. (2021). Targeting inflammation driven by HMGB1 in bacterial keratitis-A review. Pathogens 10 (10), 1235. doi: 10.3390/pathogens10101235
Hilliam, Y., Kaye, S., Winstanley, C. (2020). Pseudomonas aeruginosa and microbial keratitis. J. Med. Microbiol. 69 (1), 3–13. doi: 10.1099/jmm.0.001110
Huang, X., Barrett, R. P., McClellan, S. A., Hazlett, L. D. (2005). Silencing Toll-like receptor-9 in Pseudomonas aeruginosa keratitis. Invest. Ophthalmol. Vis. Sci. 46, 4209–4216. doi: 10.1167/iovs.05-0185
Keay, L., Edwards, K., Naduvilath, T., Taylor, H. R., Snibson, G. R., Forde, K., et al. (2006). Microbial keratitis predisposing factors and morbidity. Ophthalmology 113 (1), 109–116. doi: 10.1016/j.ophtha.2005.08.013
Kezic, A., Spasojevic, I., Lezaic, V., Bajcetic, M. (2016). Mitochondria-targeted antioxidants: future perspectives in kidney ischemia reperfusion injury. Oxid. Med. Cell Longev 2016, 2950503. doi: 10.1155/2016/2950503
Kim, Y., Choi, Y. H., Kim, M. K., Paik, H. J., Kim, D. H. (2020). Different adverse effects of air pollutants on dry eye disease: Ozone, PM2.5, and PM10. Environ. pollut. 265 (Pt B), 115039. doi: 10.1016/j.envpol.2020.115039
Kimura, Y., Kimura, H. (2004). Hydrogen sulfide protects neurons from oxidative stress. FASEB J. 18, 1165–1167. doi: 10.1096/fj.04-1815fje
Klopfer, J. (1989). Effects of environmental air pollution on the eye. J. Am. Optom Assoc. 60 (10), 773–778.
Ko, H. M., Choi, S. H., Kim, Y., An, E. J., Lee, S. H., Kim, K., et al. (2020). Effect of rosa laevigata on PM10-induced inflammatory response of human lung epithelial cells. Evid Based Complement Alternat Med. 2020, 2893609. doi: 10.1155/2020/2893609
Ko, R., Hayashi, M., Tanaka, M., Okuda, T., Nishita-Hara, C., Ozaki, H., et al. (2021). Effects of ambient particulate matter on a reconstructed human corneal epithelium model. Sci. Rep. 11 (1), 3417. doi: 10.1038/s41598-021-82971-1
Kowalczyk, P., Sulejczak, D., Kleczkowska, P., Bukowska-Ośko, I., Kucia, M., Popiel, M., et al. (2021). Mitochondrial oxidative stress-A causative factor and therapeutic target in many diseases. Int. J. Mol. Sci. 22 (24), 13384. doi: 10.3390/ijms222413384
Lambeth, J. D. (2004). NOX enzymes and the biology of reactive oxygen. Nat. Rev. Immunol. 4 (3), 181–189. doi: 10.1038/nri1312
Lee, J. Y., Kim, J. W., Kim, E. J., Li, M. Y., Nam, C. W., Chung, I. S. (2018). Spatial analysis between particulate matter and emergency room visits for conjunctivitis and keratitis. Ann. Occup. Environ. Med. 30, 41. doi: 10.1186/s40557-018-0252-x
Li, J., Tan, G., Ding, X., Wang, Y., Wu, A., Yang, Q., et al. (2017). A mouse dry eye model induced by topical administration of the air pollutant particulate matter 10. BioMed. Pharmacother. 96, 524–534. doi: 10.1016/j.biopha.2017.10.032
Li, N., Xia, T., Nel, A. E. (2008). The role of oxidative stress in ambient particulate matter-induced lung diseases and its implications in the toxicity of engineered nanoparticles. Free Radic. Biol. Med. 44 (9), 1689–1699. doi: 10.1016/j.freeradbiomed.2008.01.028
Li, T., Yu, Y., Sun, Z., Duan, J. (2022). A comprehensive understanding of ambient particulate matter and its components on the adverse health effects based from epidemiological and laboratory evidence. Part Fibre Toxicol. 19 (1), 67. doi: 10.1186/s12989-022-00507-5
Liu, J., Chen, X., Dou, M., He, H., Ju, M., Ji, S., et al. (2019). Particulate matter disrupts airway epithelial barrier via oxidative stress to promote Pseudomonas aeruginosa infection. J. Thorac. Dis. 11 (6), 2617–2627. doi: 10.21037/jtd.2019.05.77
Liu, L., Poon, R., Chen, L., Frescura, A. M., Montuschi, P., Ciabattoni, G., et al. (2009). Acute effects of air pollution on pulmonary function, air-way inflammation, and oxidative stress in asthmatic children. Environ. Health Perspect. 117, 668–674. doi: 10.1289/ehp11813
Manisalidis, I., Stavropoulou, E., Stavropoulos, A., Bezirtzoglou, E. (2020). Environmental and health impacts of air pollution: A review. Front. Public Health 8. doi: 10.3389/fpubh.2020.00014
Miller, M. R., Borthwick, S. J., Shaw, C. A., McClure, D., Mills, N. L., et al. (2009). Direct impairment of vascular function by diesel exhaust particulate through reduced bioavailability of endothelium-derived nitric oxide induced by superoxide free radicals. Environ. Health Perspect. 117, 611–616. doi: 10.1289/ehp.0800235
Mo, Z., Fu, Q., Lyu, D., Zhang, L., Qin, Z., Tang, Q., et al. (2019). Impacts of air pollution on dry eye disease among residents in Hangzhou, China: A case-crossover study. Environ. pollut. 246, 183–189. doi: 10.1016/j.envpol.2018.11.109
Moon, M. M., Hazlett, L. D., Hancock, R. E., Berk, R. S., Barret, R. (1988). Monoclonal antibodies provide protection against ocular Pseudomonas aeruginosa infection. Invest. Ophthalmol. Vis. Sci. 29 (8), 1277–1284.
Mu, N., Wang, H., Chen, D., Wang, F., Ji, L., Zhang, C., et al. (2022). A novel rat model of Dry Eye induced by aerosol exposure of particulate matter. Invest. Ophthalmol. Vis. Sci. 63 (1), 39. doi: 10.1016/j.envpol.2018.11.109
Novaes, P., do Nascimento Saldiva, P. H., Kara-José, N., Macchione, M., Matsuda, M., Racca, L., et al. (2007). Ambient levels of air pollution induce goblet-cell hyperplasia in human conjunctival epithelium. Environ. Health Perspect. 115 (12), 1753–1756. doi: 10.1289/ehp.10363
Novaes, P., Saldiva, P. H., Matsuda, M., Macchione, M., Rangel, M. P., Kara-José, N., et al. (2010). The effects of chronic exposure to traffic derived air pollution on the ocular surface. Environ. Res. 110 (4), 372–374. doi: 10.1016/j.envres.2010.03.003
Novaes, R. D., Teixeira, A. L., de Miranda, A. S. (2019). Oxidative stress in microbial diseases: pathogen, host, and therapeutics. Oxid. Med. Cell Longev 2019, 8159562. doi: 10.1155/2019/8159562
Nurkiewicz, T. R., Porter, D. W., Barger, M., Millecchia, L., Rao, K. M., Marvar, P. J., et al. (2006). Systemic microvascular disfunction and inflammation after pulmonary particulate matter exposure. Environ. Health Perspect. 114, 412–419. doi: 10.1289/ehp.8413
O'Malley, Y. Q., Reszka, K. J., Spitz, D. R., Denning, G. M., Britigan, B. E. (2004). Pseudomonas aeruginosa pyocyanin directly oxidizes glutathione and decreases its levels in airway epithelial cells. Am. J. Physiol. Lung Cell Mol. Physiol. 287 (1), L94–103. doi: 10.1152/ajplung.00025.2004
O'Neill, E. C., Yeoh, J., Fabinyi, D. C., Cassidy, D., Vajpayee, R. B., Allen, P., et al. (2014). Risk factors, microbial profiles and prognosis of microbial keratitis-associated endophthalmitis in high-risk eyes. Graefes Arch. Clin. Exp. Ophthalmol. 252 (9), 1457–1462. doi: 10.1007/s00417-014-2732-1
Otri, A. M., Fares, U., Al-Aqaba, M. A., Miri, A., Faraj, L. A., Said, D. G., et al. (2013). Profile of sight-threatening infectious keratitis: a prospective study. Acta Ophthalmol. 91 (7), 643–651. doi: 10.1111/j.1755-3768.2012.02489.x
Ousler, G. W., Watson, M., Sheppard, J. D., Karpecki, P., Friedhoff, T., Petrov, A., et al. (2022). Safety and efficacy of ophthalmic mitochondrial reactive oxygen species scavenger Visomitin for the treatment of dry eye disease: findings of VISTA-1 and VISTA-2 studies. Invest. Ophthalmol. Vis. Sci. 63 (7), 1555–A0280.
Pardo, M., Qiu, X., Zimmermann, R. (2020). Rudich Y. Particulate matter toxicity is nrf2 and mitochondria dependent: the roles of metals and polycyclic aromatic hydrocarbons. Chem. Res. Toxicol. 33 (5), 1110–1120. doi: 10.1021/acs.chemrestox.0c00007
Pintha, K., Chaiwangyen, W., Yodkeeree, S., Suttajit, M., Tantipaiboonwong, P. (2021). Suppressive effects of rosmarinic acid rich fraction from perilla on oxidative stress, inflammation and metastasis ability in A549 cells exposed to PM via C-jun, P-65-nf-Kb and akt signaling pathways. Biomolecules 11 (8), 1090. doi: 10.3390/biom11081090
Pizzino, G., Irrera, N., Cucinotta, M., Pallio, G., Mannino, F., Arcoraci, V., et al. (2017). Oxidative stress: harms and benefits for human health. Oxid. Med. Cell Longev 2017, 8416763. doi: 10.1155/2017/8416763
Pope, C. A., 3rd, Dockery, D. W. (2006). Health effects of fine particulate air pollution: lines that connect. J. Air Waste Manag Assoc. 56 (6), 709–742. doi: 10.1080/10473289.2006.10464485
Pope, C. A., 3rd, Burnett, R. T., Thun, M. J., Calle, E. E., Krewski, D., Ito, K., et al. (2002). Lung cancer, cardiopulmonary mortality, and long-term exposure to fine particulate air pollution. JAMA 287 (9), 1132–1141. doi: 10.1001/jama.287.9.1132
Radan, M., Dianat, M., Badavi, M., Mard, S. A., Bayati, V., Goudarzi, G. (2019). In vivo and in vitro evidence for the involvement of Nrf2-antioxidant response element signaling pathway in the inflammation and oxidative stress induced by particulate matter (PM10): the effective role of gallic acid. Free Radic. Res. 53 (2), 210–225. doi: 10.1080/10715762.2018.1563689
Saraiva, M., O'Garra, A. (2010). The regulation of IL-10 production by immune cells. Nat. Rev. Immunol. 10 (3), 170–181. doi: 10.1038/nri2711
Sendra, V. G., Tau, J., Zapata, G., Lasagni Vitar, R. M., Illian, E., Chiaradía, P., et al. (2021). Polluted air exposure compromises corneal immunity and exacerbates inflammation in acute herpes simplex keratitis. Front. Immunol. 12:618597. doi: 10.3389/fimmu.2021.618597
Skulachev, V. P. (2011). SkQ1 treatment and food restriction–two ways to retard an aging program of organisms. Aging (Albany NY) 3 (11), 1045–1050. doi: 10.18632/aging.100410
Skulachev, V. P. (2013). Cationic antioxidants as a powerful tool against mitochondrial oxidative stress. Biochem. Biophys. Res. Commun. 441, 275–279. doi: 10.1016/j.bbrc.2013.10.063
Somayajulu, M., Ekanayaka, S., McClellan, S. A., Bessert, D., Pitchaikannu, A., Zhang, K., et al. (2020). Airborne particulates affect corneal homeostasis and immunity. Invest. Ophthalmol. Vis. Sci. 61 (4), 23. doi: 10.1167/iovs.61.4.23
Somayajulu, M., McClellan, S. A., Wright, R., Pitchaikannu, A., Croniger, B., Zhang, K., et al. (2023). Airborne exposure of the cornea to PM(10) induces oxidative stress and disrupts nrf2 mediated anti-oxidant defenses. Int. J. Mol. Sci. 24, 4. doi: 10.3390/ijms24043911
Stapleton, F., Carnt, N. (2012). Contact lens-related microbial keratitis: how have epidemiology and genetics helped us with pathogenesis and prophylaxis. Eye (Lond) 26 (2), 185–193. doi: 10.1038/eye.2011.288
Torricelli, A. A., Matsuda, M., Novaes, P., Braga, A. L., Saldiva, P. H., Alves, M. R., et al. (2014). Effects of ambient levels of traffic-derived air pollution on the ocular surface: analysis of symptoms, conjunctival goblet cell count and mucin 5AC gene expression. Environ. Res. 131, 59–63. doi: 10.1016/j.envres.2014.02.014
Wang, J., Huang, J., Wang, L., Chen, C., Yang, D., Jin, M., et al. (2017). Urban particulate matter triggers lung inflammation via the ROS-MAPK-NF-κB signaling pathway. J. Thorac. Dis. 9 (11), 4398–4412. doi: 10.21037/jtd.2017.09.135
Wang, B., Xu, D., Jing, Z., Liu, D., Yan, S., Wang, Y. (2014). Effect of long-term exposure to air pollution on type 2 diabetes mellitus risk: a systemic review and meta-analysis of cohort studies. Eur. J. Endocrinol. 171 (5), R173–R182. doi: 10.1530/EJE-14-0365
Yoon, S., Han, S., Jeon, K. J., Kwon, S. (2018). Effects of collected road dusts on cell viability, inflammatory response, and oxidative stress in cultured human corneal epithelial cells. Toxicol. Lett. 284, 152–160. doi: 10.1016/j.toxlet.2017.12.012
Zernii, E. Y., Gancharova, O. S., Baksheeva, V. E., Golovastova, M. O., Kabanova, E. I., Savchenko, M. S., et al. (2017). Mitochondria-targeted antioxidant skQ1 prevents anesthesia-induced dry eye syndrome. Oxid. Med. Cell Longev 2017, 9281519. doi: 10.1155/2017/9281519
Zhang, T. P., Dou, J., Wang, L., Wang, S., Wang, P., Zhou, X. H., et al. (2022). Exposure to particulate pollutant increases the risk of hospitalizations for Sjögren's syndrome. Front. Immunol. 13:1059981. doi: 10.3389/fimmu.2022.1059981
Zhang, Y., Ma, Y., Feng, F., Cheng, B., Shen, J., Jiao, H. (2021). Association between PM10 and specific circulatory system diseases in China. Sci. Rep. 11 (1), 12129. doi: 10.1038/s41598-021-91637-x
Keywords: PM10, P. aeruginosa, cornea, epithelium, human
Citation: Somayajulu M, McClellan SA, Muhammed F, Wright R and Hazlett LD (2023) PM10 and Pseudomonas aeruginosa: effects on corneal epithelium. Front. Cell. Infect. Microbiol. 13:1240903. doi: 10.3389/fcimb.2023.1240903
Received: 15 June 2023; Accepted: 20 September 2023;
Published: 05 October 2023.
Edited by:
Poonam Mudgil, Western Sydney University, AustraliaReviewed by:
Phillip Stephen Coburn, University of Oklahoma Health Sciences Center, United StatesMd Huzzatul Mursalin, University of Oklahoma, United States
Copyright © 2023 Somayajulu, McClellan, Muhammed, Wright and Hazlett. This is an open-access article distributed under the terms of the Creative Commons Attribution License (CC BY). The use, distribution or reproduction in other forums is permitted, provided the original author(s) and the copyright owner(s) are credited and that the original publication in this journal is cited, in accordance with accepted academic practice. No use, distribution or reproduction is permitted which does not comply with these terms.
*Correspondence: Linda D. Hazlett, bGhhemxldHRAbWVkLndheW5lLmVkdQ==