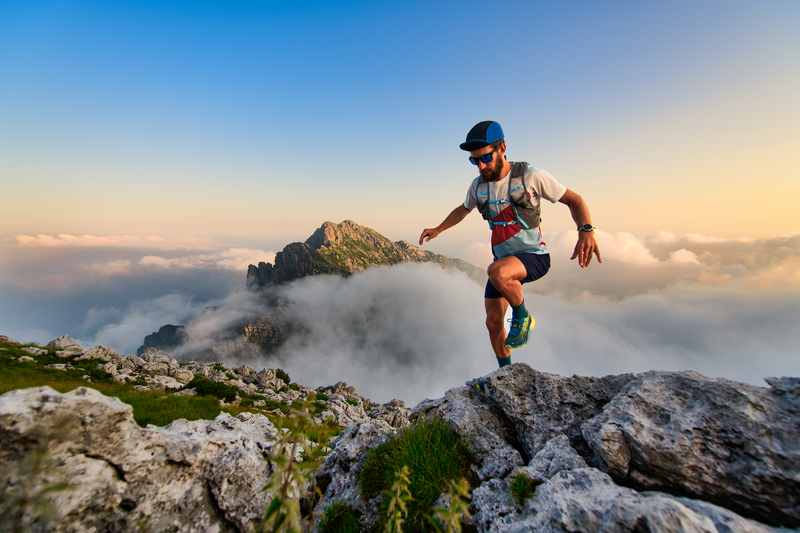
95% of researchers rate our articles as excellent or good
Learn more about the work of our research integrity team to safeguard the quality of each article we publish.
Find out more
ORIGINAL RESEARCH article
Front. Cell. Infect. Microbiol. , 31 July 2023
Sec. Biofilms
Volume 13 - 2023 | https://doi.org/10.3389/fcimb.2023.1229460
This article is part of the Research Topic The ever so elusive pathogen-harboring biofilms on abiotic surfaces in the food and clinical sectors: the good, the bad and the slimy View all 6 articles
Food processing lines represents a suitable environment for bacterial biofilm formation. One of the most common biofilm-forming genera in dairy processing plants is Bacillus, which includes species that may have a negative impact on safety and/or quality of dairy products. In the current study, we evaluated the biofilm forming ability and molecular characteristics of dairy Bacillus spp. isolates (B. cereus and B. subtilis). Reference strains (B. cereus ATCC 14579 and B. subtilis NCTC 3610) were also included in the experiment. All isolates were screened by micro-titer plate (96 wells) to assess their ability to form biofilm. Then, they were tested on two common food contact surfaces (polystyrene and stainless steel) by using 6-well plates and AISI 316 stainless steel coupons. Biofilm formation, expressed as biofilm production index (BPI), was higher on polystyrene than stainless steel (except for B. cereus ATCC 14579). These observations were further confirmed by scanning electron microscopy, which allowed the microscopy observation of biofilm structure. Moreover, a possible correlation among total viable cell counts (CFU) and BPI was examined, as well as a connection among biofilm formation and bacterial cell hydrophobicity. Finally, whole genome sequencing was performed highlighting a genetic similarity among the strains belonging to the same species. The presence of selected genes involved in biofilm formation was also examined showing that strains with a greater presence of these genes were able to produce more biofilm in the tested materials. Additionally, for B. cereus strains enterotoxin genes were detected.
Many microorganisms can adhere to surfaces organizing in multicellular communities called biofilms (Costerton et al., 1999). The formation of biofilm is a multi-step process starting with the bacterial attachment to surfaces, and then progressing with the production of extracellular polymeric substances (EPS) (O'Toole et al., 2000; López et al., 2010). The EPS is a complex system constituted of polysaccharides, protein, lipids, nucleic acids and various heteropolymers secreted by the microorganisms in the extracellular environment (Branda et al., 2005; Flemming et al., 2007; Di Martino, 2018).
Within biofilm, bacteria can resist nutrient absence, pH changes better than planktonic organisms (Jefferson, 2004). Moreover, in biofilm state, microorganisms are more resilient to antimicrobial agents compared to planktonic cells (Srey et al., 2013).
Biofilm allows bacteria to bind on a wide range of common food contact surfaces (plastic, stainless steel etc.) and to persist in manufacturing plants after the application of cleaning and disinfection procedures (Hall-Stoodley et al., 2004; Simões et al., 2009). Consequently, once the biofilm is produced, it can be responsible for cross-contamination when food products are exposed to contaminated equipment surfaces (Anand et al., 2014).
Biofilms may develop in a wide range of food processing plants such as meat/poultry (Nikolaev et al., 2022) or dairy (Gopal et al., 2015) and seafood processing (Mizan et al., 2015). Dairy plants could provide an appropriate environment for biofilm development. The temperature, humidity, presence of water, in fact, allows bacteria to grow in dairy processing facilities. In addition, milk waste enriched in fats, proteins, and carbohydrates, could facilitate microbial growth and multiplication (Carrascosa et al., 2021). In addition, it is known that cell hydrophobicity could influence the adhesion of bacteria to a surface and therefore the formation of biofilm (Krasowska and Sigler, 2014).
Under favorable conditions, both spoilage and pathogenic microorganisms may form biofilms and persist in the environment (Galié et al., 2018). As for as the dairy processing plant is concerned, one of the most common biofilm-forming genera is Bacillus (Ostrov et al., 2019). Bacillus species are ubiquitous, gram-positive, motile, and characterized by a high versatility and adaptability to different environmental conditions (Ehling-Schulz et al., 2015; Gopal et al., 2015). Moreover, they are spore-forming bacteria commonly isolated from both raw and pasteurized milk, pre- and post-pasteurization segments of dairy plants (Shemesh and Ostrov, 2020). Among Bacillus species, B. cereus may cause food poisoning, because some isolates may produce diarrheal enterotoxins, nonhemolytic enterotoxin (Nhe), cytotoxin K (CytK), hemolysin BL (Hbl), cell wall peptidase (EntFM) and emetic toxin (ces) (StenforsArnesen et al., 2008). It can form biofilm on various materials such as stainless-steel pipes, conveyor belts and storage tanks and it can also form floating or immersed biofilms (Grigore-Gurgu et al., 2019). B. subtilis, especially in the biofilm state, can produce a vast array of enzymes, such as heat stable proteases and lipases, which can affect food organoleptic properties of dairy products (Karaka and Güven, 2018). Previous studies investigated biofilm formation of Bacillus spp. isolates from dairy sector. To date, few data on molecular characteristics of these biofilm-forming dairy isolates are available (Yuan et al., 2018; Elegbeleye and Buys, 2020; Zhao et al., 2020).
In regard to this, the aims of our study were: i) to evaluate the biofilm forming ability of dairy Bacillus spp. isolates (B. cereus and B. subtilis) in conditions simulating the ones naturally encountered in dairy processing plants; ii) to examine the possible correlation among biofilm formation ability and the hydrophobicity of bacterial cells; iii) to observe the biofilm produced by selected isolates using SEM analysis; iv) to analyze the genetic characteristics of isolates by Whole Genome Sequencing (WGS).
Bacillus spp. strains (four B.cereus and four B.subtilis), previously collected from processed cheeses in an Italian dairy processing plant, were used in this study. These isolates were cultured on Plate Count Agar (Oxoid, United Kingdom) by incubation at 30°C and colonies were identified using the Matrix-Assisted Laser Desorption Ionization-Time of Flight (MALDI-TOF) mass spectrometry (Catania et al., 2021). Species’ confirmation was carried out by PCR amplification of the 16S rRNA gene using the universal primers 8F and 533R (Catania et al., 2021). The B. cereus ATCC 14579 and B. subtilis NCTC 3610 were also included in this study as reference strains.
The biofilm-forming ability of dairy Bacillus spp. isolates and reference strains (B. cereus ATCC 14579 and B. subtilis NCTC 3610) was evaluated following a previous protocol (Stepanović et al., 2000; Stepanović et al., 2007), with some modifications. All isolates were classified in weak, moderate, strong or no biofilm producers (Stepanović et al., 2000).
In this step, two growth media (Brain Heart Infusion- BHI and Broth and Tryptone Soya Broth - TSB), and two incubation times (24h - 48h), were tested at 30°C to identify the optimal conditions for biofilm formation of the strains included in this experiment.
Briefly, overnight bacterial cultures, in BHI or TSB, were standardized to 0,5 McFarland standard (cell concentration~108 CFU/mL). Subsequently, 200 μL of bacterial suspension was added to 96-well polystyrene microplates (Sarstedt, Germany) with three replicates for each isolate, while the negative control wells contained only the broths. Microtiter plates (96 wells) were incubated for 24 h and 48 h at 30°C. After incubation, medium was discarded and wells were rinsed three times with 300 μL of sterile phosphate buffer saline solution (PBS, pH 7.3, Oxoid, England). Biofilms were heat-fixed at 60°C for 1 h and then stained with 150 μL of 2% w/v crystal violet solution (Chem-lab, Belgium) for 15 min. Unbound crystal violet was discarded by rinsing wells 3 times with distilled water and dried at 37°C for 15 min. To quantify the biofilm formation, 150 μL of 95% ethanol solution (Honeywell, USA) was added to each well. Plates were left at least 30 min at room temperature and then the absorbance was measured at 595 nm on a microplate reader (iMark plate reader, Bio-Rad, Australia). A mean of OD values was calculated for each strain (ODs), while the cut-off OD (ODc) was calculated as three standard deviations above the mean OD of the negative control (Stepanović et al., 2000). According to their OD value, the strains were classified as weak (ODc< ODs ≤ 2 x ODc), moderate (2 x ODc< ODs ≤ 4 x ODc), strong (4 x ODc< ODs) or no (ODs ≤ ODc) biofilm producers. Three independent experiments were performed.
Dairy Bacillus spp. isolates and reference strains, previously classified in weak, moderate or strong biofilm forming strains (see section 2.2), were tested on Nunc™ polystyrene cultures plates (6-well) (ThermoFisher Scientific, USA) (961 mm2) and AISI 316 stainless-steel coupons (530 mm2) following previous protocols with some modifications (Di Bonaventura et al., 2008; Di Ciccio et al., 2015).
Briefly, overnight bacterial cultures at 30°C in BHI broth (Oxoid, England) were centrifuged at 4000 rpm for 10 min, rinsed thrice with PBS solution (Oxoid, England), and then re-suspended in BHI broth (Oxoid, England). Cultures were diluted to reach 550 nm OD of approximately 0.125 corresponding to a cell concentration of 108 CFU/mL. Three milliliters of each diluted culture were then added to polystyrene tissue culture plates (3 wells for each strain), with or without stainless steel coupons, place horizontally, and then incubated at 30°C for 24 h. After incubation, each PS well was rinsed three times with 3 mL of sterile PBS (Oxoid, England) whereas each SS coupon was rinsed by dipping it three times in different sterile PBS containers to remove non-adherent cells. SS coupons were then, transferred in a new microtiter plate (ThermoFisher Scientific, USA).
After the fixing phase at 60°C for 1 h, three milliliter of crystal violet (2% - Chem-lab Belgium) in 95% ethanol (Honeywell, USA) was added in each well with or without stainless steel coupons. After 20min, the wells were rinsed thrice with distilled water and dried at 37°C for 15 min. Then, 3 ml of a 33% acetic acid solution (Merck, Darmstadt, Germany) were added to each well with or without stainless steel coupons. After 20 min, 0.2 mL from each sample were transferred to a 96-well microtiter plate (Sarstedt, Germany), and the OD level of the destaining solution was measured at 490 nm. Considering the different growth area of tested surface (polystyrene: 961 mm2 and stainless steel: 530 mm2), results were normalized calculating the biofilm production index (BPI) as follows:
To determine culturable cells in biofilm, polystyrene wells and stainless-steel coupons were rinsed by dipping 3 times in PBS (Oxoid, England) as previously described (see Section 2.3). Cells were removed using a cell scraper. Serial dilutions were performed in sterile physiological saline peptone (PS) (0.85% NaCl, Carlo Erba; 0.1% Bacteriological Peptone, Oxoid) and spread on BHI-agar plates. Colonies forming units (CFU) were counted after 24 h of incubation at 30°C and the counts were expressed as Log CFU/mm2.
The hydrophobicity of bacterial cells was evaluated by Microbial Adhesion to hydrocarbon (MATH) assay as previously described by Rosenberg et al., 1980, with some modifications. Briefly, overnight bacterial cultures in BHI were centrifuged and then resuspended in PBS (Oxoid, England) to an optical density of 0.8 at 550 nm, this value represents A1 (Absorbance 1). Followingly, 1 ml of n-hexadecane (Fisher Scientific, Italy) was added to1 ml each bacterial suspension, vortex for 1 minute and incubate at room temperature for 15 min, allowing the separation of aqueous phase. After this time, the absorbance of aqueous phase (Absorbance 2) was measured at 550 nm. Each sample was tested in three independent experiments. The relative hydrophobicity (RH) was expressed as a percentage according to the formula:
Isolates were classified as: highly hydrophobic for values >50%; moderately hydrophobic for values ranging from 20 to 50% and hydrophilic for values<20%.
Biofilm formation was further examined by scanning electron microscopy (SEM). Two reference strains (B. cereus ATCC 14579 and B. subtilis NCTC 3610) and two dairy isolates (BC_14 and BS_42) were selected for the SEM analysis based on their BPI index.
The microbial cells were grown at 37°C for 24 h on polystyrene tissue plates and stainless-steel coupons (see Section 2.3) then rinsed by dipping 3 times in sterile PBS in order to remove non adherent cells and heat-fixed at 60°C for 1 h. Biomass were fixed with 2.5% glutaraldehyde (Sigma Aldrich, Germany) in 0.1 M sodium cacodylate buffer (pH 7.2) (Sigma Aldrich, Germany) for 30 min at room temperature and then fixed in 1% osmium tetroxide (Sigma Aldrich, Germany) for 1 h. Samples were then rinsed with 0.1 M cacodylate buffer for 1 h to remove any unreacted glutaraldehyde before rinsing and dehydration. Samples were dehydrated through an ascending series of ethanol (30%, 50%, 70% and 80%) for 15 min for each concentration, and then three times for 15 min in 100% ethanol. Finally, they were overnight air-dried. Specimens were then sputter-coated with a gold-palladium layer using an Emitech K575X Peltier-cooled (EM Technologies, England). Finally, selected samples were observed using the Jeol LV300 Scanning Electron Microscope at an accelerating voltage of 25kV and working distance of 6 mm, with a probe current of about 100 pA. All SEM analyses were performed in two independent experiments.
For DNA extraction, isolated colonies were inoculated in brain heart infusion (BHI) broth and incubated at 30°C, overnight. The DNA of B. cereus and B. subtilis strains was extracted using the DNeasy Blood and Tissue Kit (Qiagen Ltd., UK), according to the manufacturer’s instructions. DNA concentration and purity were determined respectively using the Qubit Fluorometer (Thermo Fisher Scientific, USA) with the Qubit dsDNA HS Assay Kit and NanoDrop spectrophotometer (ThermoFisher Scientific, USA).
Library preparation, WGS and control steps were performed by genomics service company Novogene (Cambridge, United Kingdom). Samples were run on Illumina NovaSeq 6000 platform to generate 2 × 150 bp paired end (PE) reads.
Reads were first trimmed for low quality (Phred score< 20) by using SolexaQA++ software v3.1.7.1 (Cox et al., 2010), and reads shorter than 60 bp were discarded with PRINSEQ v0.20.4 (Schmieder and Edwards, 2011). Clean reads were then assembled using SPAdes v3.14.1 (Nurk et al., 2017) and contigs shorter than 500 nt were discarded. Genes were annotated with Prokka v1.14.5 (Seemann, 2014) with default parameters. QUAST v5.0.2 software was then used to check the quality of the contigs (Gurevich et al., 2013).
Draft genomes of B. cereus ATCC 14579 and B. subtilis NCTC 3610 were downloaded from NCBI, and genes were annotated with Prokka. The pan genome calculation and phylogenetic analysis of Bacillus strains were obtained by Roary v. 3.11.2 (Page et al., 2015). Phylogenetic tree was then visualized in iTOL6 (https://itol.embl.de/).
Venn diagrams were obtained by Venn Diagram Maker (https://goodcalculators.com/venn-diagram-maker/).
Predicted genes were then aligned against genes involved on biofilm formations by BLASTn tools (e-value cutoff of 1e-5, requiring a hit to display >90% of identity over at least 30% of the query length).
The data shown are average values obtained in at least three independent experiments with standard deviations. For assessment of significant differences, one-way analysis of variance (ANOVA) with Tukey’s post hoc test was performed. Statistical analyses and graphing were conducted with GraphPad Prism version 8.4.3 (GraphPad Software, San Diego,CA, USA). Differences were considered statistically significant when p values were less than 0.05.
The biofilm formation of dairy isolates (four B. cereus and four B. subtilis) and reference strains (B. cereus ATCC 14579 and B. subtilis NCTC 3610) was evaluated in BHI broth (Oxoid, England) and TSB (Oxoid, England) for 24h and 48h, at 30°C.
After 24h, all isolates were able to produce biofilm, with statistically significant differences (p< 0.05) in relation to the growth media and incubation time (Figure 1).
Figure 1 Biofilm forming ability of (A) Bacillus cereus (BC) dairy isolates and ATCC 14579 reference strain and (B) Bacillus subtilis (BS) dairy isolates and NCTC 3610 reference strain in different growth media (BHI and TSB) and incubation time (24h and 48h). The threshold of biofilm formation (dotted line) is equal to OD values ≤ 0.11. Error bars indicate standard deviation. Different letters indicated significant difference among the isolates, p< 0.05.
According to their OD values (see Section 2.2),B. cereus BC_2 and BC_14 isolates were classified as strong biofilm producers in BHI, at 24 and 48h, whereas they are respectively moderate and weak producers in TSB, with no statistically significant differences as a function of the incubation time (Figure 1A). Strain BC_36 was a weak producer in all conditions; BC_44 isolate was classified as a moderate biofilm producer, with a biofilm production slightly higher in TSB after 24h, but without statistical difference compared to the same time in BHI (Figure 1A). ATCC 14579 reference strain was a moderate producer in BHI after 24h, whereas at the same incubation time, a weak biofilm production was assessed in TSB, no statistically significant difference was highlighted for the two growth media after 48h (Figure 1A).
On the other hand, all B. subtilis isolates resulted strong producers in BHI, except BS_8 strain which after 24h in BHI, resulted as weak biofilm producer (Figure 1B). The reference strain NCTC 3610showed moderate biofilm-forming ability in BHI, while in TSB was classified as strong producer (Figure 1B).
The BPI index of each strain was evaluated on two common food contact surfaces: polystyrene and stainless steel at 30°C(see Section 2.3). Reference strains (B. cereusATCC 14579 and B. subtilisNCTC 3610) were included. A higher biofilm production on polystyrene was observed for all isolates, except for B. cereus ATCC 14579 (Figure 2). The BPI indices of B. subtilis were higher than B. cereus in both examined surfaces (Figure 2).
Figure 2 Biofilm formation of reference strains ATCC 14579 and NCTC 3610, Bacillus cereus (BC) and Bacillus subtilis (BS) food isolates, on polystyrene and stainless-steel coupons. Biofilms were formed in BHI at 30°C for 24 h and biofilm production index (BPI) was measured by the crystal violet assay. Each bar represents the result of the average of three biological experiments. Error bars indicate standard deviation. Letters on the top of the bars indicate significant statistically differences (p< 0.05).
Cell counts were performed to assess the number of culturable cells in the biomass of each sample. The load of Bacillus cereus cells ranging from 5,74 ± 0,16 and 6,78 ± 0,27 Log CFU/mm2 on polystyrene wells and 5,77 ± 0,18 and 7,81 ± 0,13 Log CFU/mm2 on stainless steel coupons respectively. Among materials (polystyrene and stainless steel) used in this study, a statistically significant difference was observed, except for BC_44 isolate (Figure 3). The reference strain ATCC 14579 showed the lowest bacterial count on polystyrene and highest bacterial count on stainless steel (Figure 3), in accordance with BPI values (see Section 3.2).
Figure 3 Total CFU counts in the biofilms of reference strains, B. cereus (BC) and B. subtilis (BS). Biofilms were grown on polystyrene wells and stainless-steel coupons in BHI at 30°C for 24 h. Each bar represents the result of the average of three biological experiments and standard deviation for each strain. To compare the number of sessile cells yielded among strains, one-way ANOVA and Tukey’s post hoc test were performed. Groups with different alphabets indicate significant difference (p< 0.05).
As for as Bacillus subtilis is concerned, cell counts ranged from 8,08 ± 0,26 to 8,7 ± 0,10 Log CFU/mm2 on polystyrene wells, and from 7,12 ± 0,08 and 7,95 ± 0,23 on stainless steel coupons. A statically significant difference was observed among materials, except for BS_23 isolate (Figure 3).
With MATH assay, the cell surface hydrophobicity of dairy isolates and reference strains was tested. The percentage of hydrophobicity, jointly to the BPI values in polystyrene and stainless steel, is reported in Table 1. Among the tested strains, all B. subtilis, including NCTC 3610 reference strain, were evaluated as hydrophobic. A variability in the percentage of adhesion was observed for B. cereus strains: two isolates (BC_36 and BC_44) revealed a hydrophobic character, one strain resulted hydrophilic (BC_14), and other two (BC_2 and B. cereus ATCC 14579 reference strain) moderately hydrophobic (Table 1).
Table 1 Hydrophobicity of B. cereus and B. subtilis including the reference strains (ATCC 14579 and NCTC 3610).
To observe biofilm architecture of Bacillus spp., SEM analysis was performed. Two isolates of dairy origin (B. subtilisBS_42 and B. cereusBC_14) were selected based on their BPI index on polystyrene and stainless steel. Reference strains were also included in this analysis.
Representative micrographs of biofilms produced by tested isolates are shown in Figures 4, 5. Bacillus subtilis reference strain NCTC 3610 and dairy isolate BS_42, showed a complex tridimensional meshwork-like structure of cells at high density, embedded in a network of extracellular polymeric substances on polystyrene surface compared to stainless steel surface (Figure 4). Considering B. cereus, fibrillar materials were visible on polystyrene for reference strain ATCC 14579 and dairy strain BC_14, (Figures 5A, C), whereas a partial biofilm consisting of sparse aggregates of cells bound by few or absent extracellular polymeric substances was observed on stainless steel (Figures 5B, D).
Figure 4 Scanning electron microscope (SEM) images of biofilms formed by reference B. subtilis strain NCTC 3610 in (A) polystyrene and (B) stainless-steel and dairy B. subtilis isolate BS_42 in (C) polystyrene and (D) stainless-steel. On the left the representation of 2000x, on the right 5000x magnification. The white arrows show the EPS matrix. Biofilms were formed in BHI broth at 30°C for 24h. a,b,c,d: structure details and biofilm matrix visualized by SEM.
Figure 5 Scanning electron microscope (SEM) images of biofilms formed by reference B. cereus strain ATCC 14579 in (A) polystyrene and (B) stainless-steel and dairy B. cereus isolate BC_14 in (C) polystyrene and (D) stainless-steel. On the left the representation of 2000x, on the right 10000x magnification for (a, c) and 5000x magnification for (b, d). The white arrows show the EPS matrix. Biofilms were formed in BHI broth at 30°C for 24h.
Strains were de novo sequenced, assembled and subjected to comparative analysis. B. cereus genomes display a GC content between 35,09 and 35,16% and a length ranging from 5.7 Mb to 6.4 Mb (Table 2). B. subtilis genomes display a GC content between 38.74 and 43.66% and a length ranging from 4.1 Mb to 9.5 Mb (Table 2).
A potential functional diversity was observed across the different strains. Venn diagram depicted the overlap among B. cereus genes common or unique between strains (Figure 1A). 1977 genes were shared among strains. BC_14 isolate displayed the unique presence of Cytochrome f gene, BC_44 the presence of 12 unique genes, BC_2 of 67 genes, while type strain showed the presence of 100 unique genes (Figure 6A)
Figure 6 Venn diagrams depicting the overlap between genes common or unique between (A) B. cereus or (B) B. subtilis genomes. Phylogenetic tree built on concatenated (C) B. cereus or (D) B. subtilis genes.
When comparing B. subtilis strains, a total of 2368 genes were shared, while three were unique to BS_23, 500 to BS_8 and 91 were unique in the type strain (Figure 6B).
Phylogenetic tree showed that B. cereus genomes BC_36 and BC_14 and BC_2 and B. cereus ATCC 14579 clustered together, while BC_44 belonged to a different cluster (Figure 6C). The same behavior was observed between B. subtilis genomes BS_42 and BS_54 and BS_8 and B. subtilis NCTC3610, while BS_23 genome was different (Figure 6D).
The presence of biofilm related genes in tested isolates was evaluated extrapolating the data from WGS (Table 3); moreover, for B. cereus strains, toxin profile was also assessed (Table 4).
Food isolates display a variety of biofilm-regulated genes. The frequency of these genes was higher in B. subtilis compared to B. cereus, with differences depending on strains (Table 3).
The presence of hemolysin hbl was not detected, all isolates were positive for non-hemolysin enterotoxin nhe. Positivity for entFM and cytK was also detected in all analyzed bacterial isolates. In contrast, the bceT gene and emetic strains (ces gene) were not identified (Table 4).
Although the presence of Bacillus spp. biofilms on equipment surfaces in dairy processing industries have been reported by several authors, few studies described the differences among Bacillus spp. isolates on the ability to form biofilms (Hayrapetyan et al., 2015; Elegbeleye and Buys, 2020). Members of the Bacillus genus are considered one of the most harmful dairy biofilm formers, which may threaten the quality and safety of milk products (Shemesh and Ostrov, 2020), thanks to their ability to resist to severe thermal processing (Hanson et al., 2005; Luu et al., 2015).
In previous research, we demonstrated the presence of Bacillus isolates in processed cheese, hence survived to a series of heat treatment (Catania et al., 2021). In this research, we evaluated their ability to produce biofilm.
It is well known that the formation of biofilm can be influenced by various factors, including time of incubation and composition of growth media (Wijman et al., 2007). To determine the optimal conditions for biofilm production, we evaluated the ability of these dairy Bacillus spp. isolates and reference strains to form biofilm in different environmental conditions, i.e., different growth media (BHI and TSB) and time of incubation (24h - 48h) at the optimal temperature (30°C) by using a previously described biofilm method (Stepanović et al., 2000; Stepanović et al., 2007). This practice represents one of the most common approaches used as screening method to assess biofilm formation ability of bacterial isolates (Azeredo et al., 2017). Our results showed that after 24h all tested strains were able to produce biofilm, with differences depending on growth media and incubation time (see section 3.1). A slight amount of biofilm formation was assessed after 24h in BHI for ATCC 14579 reference strain. B. cereus BC_2 and BC_14 dairy isolates were more effective in biofilm formation in BHI broth, compared to TSB, while BC_36 strains was a weak producer in both growth media and, for BC_44 isolate, no statistically significant difference was observed after 24 h in BHI broth and TSB. After 24 h, all B. subtilis isolates were strong biofilm produced in BHI, compared to TSB, except B. subtilis BS_8 strain and NCTC 3610 reference strain. Our results are in accordance with previous studies in which the biofilm production of food isolated strains was higher in BHI (Hayrapetyan et al., 2015; Kowalska et al., 2020). Conversely, in another study authors showed that there was no difference in biofilm formation in BHI and TSB for B. cereus strains after 24 h, whereas after 48 h of incubation, the biofilms formed were significantly higher in TSB than BHI (Kwon et al., 2017). A possible explanation of a greater biofilm production in BHI, assessed for the most of tested isolates, could be related to its richness of nutrients. The high number of proteins contained in BHI, especially leucine, proline, serine, and aspartate, as well as the presence of lipids such as choline, sphingosine and sugars, may increase the biofilm expression (Stepanović et al., 2000). In addition, since after 24h in BHI most of tested isolates were classified as strong/moderate biofilm producers, for the next experiments (macro-method assay), we evaluated the biofilm production after 24h of incubation.
Various studies reported the ability of bacteria to adhere on different surfaces, including polystyrene (PS), stainless steel (SS), glass, rubber, aluminum, titanium, and ceramic (Donlan, 2001; Simões et al., 2010). We investigated the biofilm formation of Bacillus spp. isolates on PS and SS. The choice of polystyrene was based on its extensive use in the food industry mainly for packaging purposes (Genualdi et al., 2014), whereas stainless steel is widely employed in food industry equipment, such as tanks and pipes (Dewangan et al., 2015). To simulate conditions the naturally encountered in industrial plants, the experiments carried out after the screening method were performed by using 6-well plates. This system overcomes the limitation of the basic microtiter plate assay (96 wells format) concerning possible nutrient limitation and the inability to observe biofilm structure by direct microscopy (SEM). In addition, it increases the surface area for biofilm formation. In accordance with the literature, our results showed that the surface properties affected the biofilm production by Bacillus spp. isolates (Simões et al., 2010). Biofilm formation was higher on PS compared to SS for all tested isolates, except for B. cereus ATCC 14579. Previous studies reported a robust biofilm formed by ATCC 14579 on SS, compared with plastic and glass surface (Kwon et al., 2017). The great biofilm formation was particularly evident for B. subtilis strains (see Section 3.2). On the contrary, previous studies on Bacillus spp. of dairy origin reported a higher biofilm production on SS compared to PS (Hayrapetyan et al., 2015; Kwon et al., 2017); whereas in a recent study authors highlighted the ability of dairy-related B. subtilis isolates to form moderate and/or strong biofilms on PS (Elegbeleye and Buys, 2020).
To evaluate a possible correlation among the biomass, expressed as BPI index, and the number of viable cells, total bacterial counts (CFU) were performed both in PS and SS. Our results showed that there was not a correlation among CFUs and BPI indices. These findings are in accordance with other studies which highlighted that biofilm biomass is not directly related to viable bacterial cells (Monteiro et al., 2015; Stiefel et al., 2016; Rodríguez-Lázaro et al., 2018; Panebianco et al., 2021). This result could be explained since the crystal violet staining, used to measure the total biomass, includes both viable and non-viable bacteria, as well as the polymeric matrix (Monteiro et al., 2015; Stiefel et al., 2016; Rodríguez-Lázaro et al., 2018; Panebianco et al., 2021).
The variation in biofilm production on PS and SS could be related to the physicochemical characteristics of the substrates which could influence microorganisms’ adhesion (Legeay et al., 2010). Previous studies highlighted that biofilm formation on hydrophobic substrata (PS) occurred greater than that on hydrophilic ones (SS) (Cerca et al., 2005; Pagedar et al., 2010; Di Ciccio et al., 2015). The hydrophobicity seems to be a relevant factor contributing to the biofilm formation ability, and it plays a role in the early stage of bacterial adhesion, for this reason we investigated the relationships among bacterial cell hydrophobicity and biofilm formation of dairy isolates. All tested B. subtilis isolates, including NCTC 3610 reference strain, were highly hydrophobic (see Table 1). These data agreed with the high BPI values observed on PS (see Figure 2 and Table 1). Interestingly, B. cereus strains showed greater variability i.e., two isolates were classified as hydrophobic, two as moderately hydrophobic and one as hydrophilic (see Table 1). In a recent study, Elegbeleye and Buys (2020) observed that bacterial cell surface hydrophobicity is strain-specific, and intrinsic and/or extrinsic factors play a role in the biomass and biofilm’s structure. Overall, B. subiltis isolates were the species more adherent to hexadecane, and they developed more abundant biofilms on the more hydrophobic biomaterial (polystyrene). On the contrary, less abundant biofilms were observed for the most hydrophilic strains. In this sense, hydrophobicity seems influence biofilm formation.
Development of biofilm is closely associated with the generation of EPS, mainly composed of polysaccharide material. Several microscopy techniques have been developed aimed at a deeper understanding about the composition, properties, and function of biofilm formation (Wolf et al., 2002; Neu and Lawrence, 2014). Among them, SEM microscopy has been employed by several authors to observe the structure of biofilm-forming bacteria (El Abed et al., 2012). Thus, in our study to observe the presence of EPS in Bacillus spp. strains organized in biofilm state, we performed a SEM microscopic analysis of a 24-h-old mature biofilm of selected isolates based on their BPI values. Briefly, we attempted to correlate the biofilm formation, expressed as BPI indices, with the SEM images. The SEM analysis revealed a considerable amount of exopolysaccharide matrix on both surfaces in B. subtilis isolate and reference strain (NCTC 3610) as shown in Figure 4. This observation was in accordance with the BPI values calculated for these strains. On the contrary, the biofilm architecture and structure formed by B. cereus reference strain (ATCC 14579) and a dairy selected isolate was less complex on polystyrene (see Figure 5) compared to stainless steel (24 h of incubation at 30°C) in terms of amount of extracellular polysaccharides produced. These findings were evident by SEM analysis especially for ATCC 14579 (see Figure 5) and in accordance with BPI values obtained.
Genetic profile of isolates was also assessed by WGS analysis. Results showed a similarity of strains among the same species, both for B. cereus and B. subtilis. Considering toxin profile in food isolates, all B. cereus strains carrying nhe genes that are associated with diarrheal syndrome (Guinebretière et al., 2010), previous studies reported that B. cereus strains isolated from food surfaces harbored nhe genes (Carter et al., 2018). Moreover, entFM and cytK genes were also identified, a similar result was reported by Tirloni for cytK gene (Tirloni et al., 2020). Conversely, hbl, bceT and ces genes were not detected. The presence of emetic strains in the dairy production chain is considered rare (Svensson et al., 2006). Anyway, the positivity for toxin genes is not necessarily correlated to their production, and thus, further studies should be performed to elucidate this issue.
Moreover, the presence of selected genes related to biofilm formation was investigated and a significant difference among the two species was assessed. Only abrB gene, known to play a central role in biofilm formation by regulating cell mobility and differentiation, was identified in all tested isolates (Ma et al., 2017). Spo0A and comER genes were not detected in B. cereus strains while they were present in all B. subtilis, except for one dairy isolate where comER was absent. The comER gene plays an important role in the regulation of biofilm formation and sporulation in both B. subtilis and B. cereus (Yan et al., 2016). Results of a recent study carried on both bacterial species, suggest that comER may be part of the regulatory pathway that controls activation of Spo0A (Yan et al., 2016). Spo0A governs the genetic pathway controlling the matrix production, its importance as key regulator of biofilm formation was described in B. subtilis and B. cereus (Gao et al., 2015). In the study of Gao et al. (2015) produced a mutant with a deletion of Spo0A, they examined the ability of this mutant strain to generate spore and form biofilm by microscopy analysis and we reported that Δspo0A strain exhibited a defect in sporulation and biofilm production. Regarding comER, in 2016 Yan and colleagues (2016) investigated the role of this gene, showing that comER mutant in B. cereus, as much as in B. subtilis strains, showed a defect in biofilm formation. The sipW gene encodes a peptidase that is specifically required for the processing and secretion of the encoded EPS matrix proteins (Branda et al., 2006). Our results showed its presence only in B. subtilis strains. Furthermore, in all B. cereus, except dairy isolate BC_2, the codY gene was not identified, whereas it is present in all B. subtilis. In a previous study, authors showed that in B. cereus strains which lack codY gene, a decreased biofilm forming capacity was shown (Hsueh et al., 2008). On the other hand, the plcR and calY genes were mainly identified in B. cereus isolates. The development of biofilm is associated with EPS production (Branda et al., 2001). Among genes involved in matrix production, epsG and epsH were also examined and only observed in B. subtilis isolates. The biofilm matrix of B. cereus is similar to other Bacillus, but unlike B. subtilis, genes responsible for the EPS synthesis are not essential for B. cereus (Gao et al., 2015). Molecular analysis showed a greater presence of genes related to biofilm formation for B. subtilis strains, this result agreed with phenotypic results. However, despite the presence of biofilm-related genes in the genome of isolates, the concrete expression level of these genes remains unknown. Hence, additional gene expression studies are necessary to elucidate this issue.
The management of microbial contamination in the context of the dairy processing industry is crucial. Bacillus spp. such as B. subtilis and B. cereus can survive even after severe heat treatments, and they are known to be biofilm forming bacteria in the dairy equipment. The presence of Bacillus spp. biofilm is considered a significant concern to the dairy industry because it represents a potential source of continuous contamination in the working environment with implications for the safety and spoilage of dairy products. In the current study, all B. subtilis and B. cereus strains, isolated from processed cheese products and surviving after the heat treatments, were able to form biofilm on common food contact surfaces, with species-specific variation. B. subtilis strains produced a robust biofilm compared to B. cereus isolates. These differences can be correlated with presence or absence of biofilm related determinants in the genome, and they are also influenced by cell surface properties. Moreover, the SEM analysis highlighted a complex biofilm structural architecture and an extracellular matrix which covered and embedded the bacterial cells. It is known that Bacillus spp. strains organized in a biofilm state are more difficult to eradicate than planktonic cells. Regarding this, biofilm produced by Bacillus spp. in pipeline and tank systems in dairy processing equipment represents a big issue because of their resistance to common cleaning in place practices routinely applied in many dairy plants. Considering these aspects, the importance of expanding our knowledge on biofilm formation and its relationship with the molecular characteristics of isolates is crucial to better understand the complex mechanisms underlying this phenomenon. Finally, further investigations should be performed to elucidate molecular mechanisms involved in biofilm formation of Bacillus spp. to find novel strategies to minimize the risk of biofilm in dairy processing equipment.
The datasets presented in this study can be found in online repositories. The names of the repository/repositories and accession number(s) can be found below: https://www.ncbi.nlm.nih.gov/, PRJNA90254.
AC: investigation, methodology, data curation, formal analysis, writing—original draft. AD and PC: conceptualization, supervision, writing—review and editing. IF: data curation, bioinformatics analysis, review and editing. TC: resources and funding acquisition and project administration. FC: methodology, review and editing. All authors contributed to the article and approved the submitted version.
This work was supported by European Regional Development Funds (FESR 2014-2020—D24I19000980002)—TECH4MILK.
The authors are thankful to Emanuele Costa based at the Department of Earth Sciences, University of Torino, for guidance and support on SEM analysis.
The authors declare that the research was conducted in the absence of any commercial or financial relationships that could be construed as a potential conflict of interest.
All claims expressed in this article are solely those of the authors and do not necessarily represent those of their affiliated organizations, or those of the publisher, the editors and the reviewers. Any product that may be evaluated in this article, or claim that may be made by its manufacturer, is not guaranteed or endorsed by the publisher.
Anand, S., Singh, D., Avadhanula, M., Marka, S. (2014). Development and control of bacterial biofilms on dairy processing membranes. Compr. Rev. Food Sci. Food Saf. 13, 18–33. doi: 10.1111/1541-4337.12048
Azeredo, J., Azevedo, N. F., Briandet, R., Cerca, N., Coenye, T., Costa, A. R., et al. (2017). Critical review on biofilm methods. Crit. Rev. Microbiol. 43, 313–351. doi: 10.1080/1040841X.2016.1208146
Branda, S. S., Chu, F., Kearns, D. B., Losick, R., Kolter, R. (2006). A major protein component of the Bacillus subtilis biofilm matrix. Mol. Microbiol. 59, 1229–1238. doi: 10.1111/j.1365-2958.2005.05020.x
Branda, S. S., González-Pastor, J. E., Ben-Yehuda, S., Losick, R., Kolter, R. (2001). Fruiting body formation by Bacillus subtilis. Proc. Natl. Acad. Sci. U.S.A. 98, 11621–11626. doi: 10.1073/pnas.191384198
Branda, S. S., Vik, S., Friedman, L., Kolter, R. (2005). Biofilms: The matrix revisited. Trends Microbiol. 13, 20–26. doi: 10.1016/j.tim.2004.11.006
Caro-Astorga, J., Perez-García, A., de Vicente, A., Romero, D. (2014). A genomic region involved in the formation of adhesin fibers in Bacillus cereus biofilms. Front. Microbiol. 5. doi: 10.3389/fmicb.2014.00745
Carrascosa, C., Raheem, D., Ramos, F., Saraiva, A., Raposo, A. (2021). Microbial biofilms in the food industry—a comprehensive review. Int. J. Environ. Res. Public Health 18, 2014. doi: 10.3390/ijerph18042014
Carter, L., Chase, H. R., Gieseker, C. M., Hasbrouck, N. R., Stine, C. B., Khan, A., et al. (2018). Analysis of enterotoxigenic Bacillus cereus strains from dried foods using whole genome sequencing, multi-locus sequence analysis and toxin gene prevalence and distribution using endpoint PCR analysis. Int. J. Food Microbiol. 284, 31–39. doi: 10.1016/j.ijfoodmicro.2018.06.016
Catania, A. M., Civera, T., Di Ciccio, P. A., Grassi, M. A., Morra, P., Dalmasso, A. (2021). Characterization of Vegetative Bacillus cereus and Bacillus subtilis Strains Isolated from Processed Cheese Products in an Italian Dairy Plant. Foods 10, 2876. doi: 10.3390/foods10112876
Cerca, N., Pier, G. B., Vilanova, M., Oliveira, R., Azeredo, J. (2005). Quantitative analysis of adhesion and biofilm formation on hydrophilic and hydrophobic surfaces of clinical isolates of Staphylococcus epidermidis. Res. Microbiol. 156, 506–514. doi: 10.1016/j.resmic.2005.01.007
Chastanet, A., Losick, R. (2011). Just-in-time control of Spo0A synthesis in Bacillus subtilis by multiple regulatory mechanisms. J. Bacteriol. 193, 6366–6374. doi: 10.1128/JB.06057-11
Costerton, J. W., Stewart, P. S., Greenberg, E. P. (1999). Bacterial biofilms: a common cause of persistent infections. Science 284, 1318–1322. doi: 10.1126/science.284.5418.1318
Cox, M. P., Peterson, D. A., Biggs, P. J. (2010). SolexaQA: at-a-glance quality assessment of Illumina second-generation sequencing data. BMC Bioinf. 11, 485. doi: 10.1186/1471-2105-11-485
Dewangan, A. K., Patel, A. D., Bhadania, A. G. (2015). Stainless steel for dairy and food industry: a review. J. Mater. Sci. Eng. 4, 1–4. doi: 10.4172/2169-0022.1000191
Di Bonaventura, G., Piccolomini, R., Paludi, D., D’orio, V., Vergara, A., Conter, M., et al. (2008). Influence of temperature on biofilm formation by Listeria monocytogenes on various food-contact surfaces: relationship with motility and cell surface hydrophobicity. J. Appl. Microbiol. 104, 1552–1561. doi: 10.1111/j.1365-2672.2007.03688.x
Di Ciccio, P., Vergara, A., Festino, A. R., Paludi, D., Zanardi, E., Ghidini, S., et al. (2015). Biofilm formation by Staphylococcus aureus on food contact surfaces: Relationship with temperature and cell surface hydrophobicity. Food Control 50, 930–936. doi: 10.1016/j.foodcont.2014.10.048
Di Martino, P. (2018). Extracellular polymeric substances, a key element in understanding biofilm phenotype. AIMS Microbiol. 4, 274–288. doi: 10.3934/microbiol.2018.2.274
Donlan, R. M. (2001). Biofilms and device-associated infections. Emerg. Infect. Dis. 7, 277–281. doi: 10.3201/eid0702.010226
Ehling-Schulz, M., Frenzel, E., Gohar, M. (2015). Food–bacteria interplay: pathometabolism of emetic Bacillus cereus. Front. Microbiol. 6. doi: 10.3389/fmicb.2015.00704
Ehling-Schulz, M., Guinebretiere, M. H., Monthán, A., Berge, O., Fricker, M., Svensson, B. (2006). Toxin gene profiling of enterotoxic and emetic Bacillus cereus. FEMS Microbiol. Lett. 260, 232–240. doi: 10.1111/j.1574-6968.2006.00320.x
El Abed, S., Ibnsouda, S. K., Latrache, H., Hamadi, F. (2012). “Scanning electron microscopy (SEM) and environmental SEM: suitable tools for study of adhesion stage and biofilm formation,” in Scanning electron microscopy. Ed Kazmiruk V. (Intechopen, London). doi: 10.5772/34990
Elegbeleye, J. A., Buys, E. M. (2020). Molecular characterization and biofilm formation potential of Bacillus subtilis and Bacillus velezensis in extended shelf-life milk processing line. J. Dairy Sci. 103, 4991–5002. doi: 10.3168/jds.2019-17919
Fagerlund, A., Dubois, T., Økstad, O. A., Verplaetse, E., Gilois, N., Bennaceur, I., et al. (2014). SinR controls enterotoxin expression in Bacillus thuringiensis biofilms. PloS One 9, e87532. doi: 10.1371/journal.pone.0087532
Flemming, H. C., Neu, T. R., Wozniak, D. J. (2007). The EPS matrix: the “House of biofilm cells”. J. Bacteriol. 189, 7945–7947. doi: 10.1128/JB.00858-07
Galié, S., García-Gutiérrez, C., Miguélez, E. M., Villar, C. J., Lombó, F. (2018). Biofilms in the food industry: health aspects and control methods. Front. Microbiol. 9. doi: 10.3389/fmicb.2018.00898
Gao, T., Foulston, L., Chai, Y., Wang, Q., Losick, R. (2015). Alternative modes of biofilm formation by plant-associated Bacillus cereus. Microbiologyopen 4, 452–464. doi: 10.1002/mbo3.251
Genualdi, S., Nyman, P., Begley, T. (2014). Updated evaluation of the migration of styrene monomer and oligomers from polystyrene food contact materials to foods and food simulants. Food Addit. Contam. 4, 723–733. doi: 10.1080/19440049.2013.878040
Gopal, N., Hill, C., Ross, P. R., Beresford, T. P., Fenelon, M. A., Cotter, P. D. (2015). The Prevalence and Control of Bacillus and related spore-forming bacteria in the dairy industry. Front. Microbiol. 21. doi: 10.3389/fmicb.2015.01418
Grigore-Gurgu, L., Bucur, F. I., Borda, D., Alexa, E. A., Neagu, C., Nicolau, A. I. (2019). “Biofilms formed by pathogens in food and food processing environments,” in Bacterial Biofilms. Eds Dincer S, Sümengen M, Afet Arkut Ö. (Intechopen, London). doi: 10.5772/intechopen.90176
Guinebretière, M. H., Broussolle, V., Nguyen-The, C. (2002). Enterotoxigenic profiles of food-poisoning and food-borne Bacillus cereus strains. J. Clin. Microbiol. 40, 3053–3056. doi: 10.1128/JCM.40.8.3053-3056.2002
Guinebretière, M. H., Velge, P., Couvert, O., Carlin, F., Debuyser, M. L., Nguyen-The, C. (2010). Ability of Bacillus cereus group strains to cause food poisoning varies according to phylogenetic affiliation (groups I to VII) rather than species affiliation. J. Clin. Microbiol. 48, 3388–3391. doi: 10.1128/JCM.00921-10
Gurevich, A., Saveliev, V., Vyahhi, N., Tesler, G. (2013). QUAST: Quality assessment tool for genome assemblies. Bioinformatics 29, 1072–1075. doi: 10.1093/bioinformatics/btt086
Hall-Stoodley, L., William Costerton, J., Stoodley, P. (2004). Bacterial biofilms: from the Natural environment to infectious diseases. Nat. Rev. Microbiol. 2, 95–108. doi: 10.1038/nrmicro821
Hanson, M. L., Wendorff, W. L., Houck, K. B. (2005). Effect of heat treatment of milk on activation of Bacillus spores. J. Food Prot. 68, 1484–1486. doi: 10.4315/0362-028x-68.7.1484
Hayrapetyan, H., Muller, L., Tempelaars, M., Abee, T., Groot, M. N. (2015). Comparative analysis of biofilm formation by Bacillus cereus reference strains and undomesticated food isolates and the effect of free iron. Int. J. Food Microbiol. 200, 72–79. doi: 10.1016/j.ijfoodmicro.2015.02.005
Hsueh, Y. H., Somers, E. B., Lereclus, D., Wong, A. C. L. (2006). Biofilm formation by Bacillus cereus is influenced by PlcR, a pleiotropic regulator. Appl. Environ. Microbiol. 72, 5089–5092. doi: 10.1128/AEM.00573-06
Hsueh, Y. H., Somers, E. B., Wong, A. C. L. (2008). Characterization of the codY gene and its influence on biofilm formation in Bacillus cereus. Arch. Microbiol. 189, 557–568. doi: 10.1007/s00203-008-0348-8
Jefferson, K. K. (2004). What drives bacteria to produce a biofilm? FEMS Microbiol. Lett. 236, 163–173. doi: 10.1016/j.femsle.2004.06.005
Karaka, O. B., Güven, M. (2018). Effects of proteolytic and lipolytic enzyme supplementations on lipolysis and proteolysis characteristics of white cheeses. Foods 7, 125. doi: 10.3390/foods7080125
Kowalska, J., MaĆkiw, E., Stasiak, M., Kucharek, K., Postupolski, J. (2020). Biofilm-forming ability of pathogenic bacteria isolated from retail food in Poland. J. Food Prot. 83, 2032–2040. doi: 10.4315/JFP-20-135
Krasowska, A., Sigler, K. (2014). How microorganisms use hydrophobicity and what does this mean for human needs? Front. Cell. Infect. Microbiol. 4. doi: 10.3389/fcimb.2014.00112
Kwon, M., Hussain, M. S., Oh, D. H. (2017). Biofilm formation of Bacillus cereus under food-processing-related conditions. Food Sci. Biotechnol. 26 (4), 1103–1111. doi: 10.1007/s10068-017-0129-8
Legeay, G., Coudreuse, A., Poncin-Epaillard, F., Herry, J. M., Bellon-Fontaine, M. N. (2010). Surface engineering and cell adhesion. J. Adhes. Sci. Technol. 24, 2301–2322. doi: 10.1163/016942410X508037
Lindback, T., Mols, M., Basset, C., Granum, P. E., Kuipers, O. P., Kovacs, A. T. (2012). CodY, a pleiotropic regulator, influences multicellular behaviour and efficient production of virulence factors in Bacillus cereus. Environ. Microbiol. 14, 2233–2246. doi: 10.1111/j.1462-2920.2012.02766.x
López, D., Vlamakis, H., Kolter, R. (2010). Biofilms. Cold Spring Harb. Perspect. Biol. 2, a000398. doi: 10.1101/cshperspect.a000398
Luu, S., Cruz-Mora, J., Setlow, B., Feeherry, F. E., Doona, C. J., Setlow, P. (2015). The effects of heat activation on Bacillus spore germination, with nutrients or under high pressure, with or without various germination proteins. Appl. Environ. Microbiol. 81, 2927–2938. doi: 10.1128/AEM.00193-15
Ma, W., Peng, D., Walker, S. L., Cao, B., Gao, C. H., Huang, Q., et al. (2017). Bacillus subtilis biofilm development in the presence of soil clay minerals and iron oxides. NPJ Biofilms Microbiomes 3, 1–9. doi: 10.1038/s41522-017-0013-6
Mizan, M. F. R., Jahid, I. K., Ha, S. D. (2015). Microbial biofilms in seafood: a food-hygiene challenge. Food Microbiol. 49, 41–55. doi: 10.1016/j.fm.2015.01.009
Monteiro, D. R., Feresin, L. P., Arias, L. S., Barão, V. A. R., Barbosa, D. B., Delbem, A. C. B. (2015). Effect of tyrosol on adhesion of Candida albicans and Candida glabrata to acrylic surfaces. Med. Mycology 53, 656–665. doi: 10.1093/mmy/myv052
Neu, T. R., Lawrence, J. R. (2014). Investigation of microbial biofilm structure by laser scanning microscopy. Adv. Biochem. Eng. Biotechnol. 146, 1–51. doi: 10.1007/10_2014_272
Ngamwongsatit, P., Buasri, W., Pianariyanon, P., Pulsrikarn, C., Ohba, M., Assavanig, A., et al. (2008). Broad distribution of enterotoxin genes (hblCDA, nheABC, cytK, and entFM) among Bacillus thuringiensis and Bacillus cereus as shown by novel primers. Int. J. Food Microbiol. 121, 352–356. doi: 10.1016/j.ijfoodmicro.2007.11.013
Nikolaev, Y., Yushina, Y., Mardanov, A., Gruzdev, E., Tikhonova, E., El-Registan, G., et al. (2022). Microbial biofilms at meat-processing plant as possible places of bacteria survival. Microorganisms 10, 1583. doi: 10.3390/microorganisms10081583
Nurk, S., Meleshko, D., Korobeynikov, A., Pevzner, P. A. (2017). MetaSPAdes: a new versatile metagenomic assembler. Genome Res. 27, 824–834. doi: 10.1101/gr.213959.116
O'Toole, G., Kaplan, H. B., Kolter, R. (2000). Biofilm formation as microbial development. Annu. Rev. Microbiol. 54, 49–79. doi: 10.1146/annurev.micro.54.1.49
Ostrov, I., Sela, N., Belausov, E., Steinberg, D., Shemesh, M. (2019). Adaptation of Bacillus species to dairy associated environment facilitates their biofilm forming ability. Food Microbiol. 82, 316–324. doi: 10.1016/j.fm.2019.02.015
Owusu-Kwarteng, J., Wuni, A., Akabanda, F., Tano-Debrah, K., Jespersen, L. (2017). Prevalence, virulence factor genes and antibiotic resistance of Bacillus cereussensulato isolated from dairy farms and traditional dairy products. BMC Microbiol. 17, 1–8. doi: 10.1186/s12866-017-0975-9
Page, A. J., Cummins, C. A., Hunt, M., Wong, V. K., Reuter, S., Holden, M. T. G., et al. (2015). Roary: Rapid large-scale prokaryote pan genome analysis. Bioinformatics 31, 3691–3693. doi: 10.1093/bioinformatics/btv421
Pagedar, A., Singh, J., Batish, V. K. (2010). Surface hydrophobicity, nutritional contents affect Staphylococcus aureus biofilms and temperature influences its survival in preformed biofilms. J. Basic Microbiol. 50, 201000034. doi: 10.1002/jobm.201000034
Panebianco, F., Rubiola, S., Chiesa, F., Civera, T., Di Ciccio, P. A. (2021). Effect of gaseous ozone on Listeria monocytogenes planktonic cells and biofilm: An in vitro study. Foods 10 (7), 1484. doi: 10.3390/foods10071484
Rodríguez-Lázaro, D., Alonso-Calleja, C., Oniciuc, E. A., Capita, R., Gallego, D., González-Machado, C., et al. (2018). Characterization of biofilms formed by foodborne methicillin-resistant Staphylococcus aureus. Front. Microbiol. 9. doi: 10.3389/fmicb.2018.03004
Rosenberg, M., Gutnick, D., Rosenberg, E. (1980). Adherence of bacteria to hydrocarbons: a simple method for measuring cell-surface hydrophobicity. FEMS Microbiol. Lett. 9, 29–33. doi: 10.1111/j.1574-6968.1980.tb05599.x
Schmieder, R., Edwards, R. (2011). Quality control and preprocessing of metagenomic datasets. Bioinformatics 27, 863–864. doi: 10.1093/bioinformatics/btr026
Seemann, T. (2014). Prokka: rapid prokaryotic genome annotation. Bioinformatics 30, 2068–2069. doi: 10.1093/bioinformatics/btu153
Shemesh, M., Ostrov, I. (2020). Role of Bacillus species in biofilm persistence and emerging antibiofilm strategies in the dairy industry. J. Sci. Food Agric. 100, 2327–2336. doi: 10.1002/jsfa.10285
Simões, M., Bennett, R. N., Rosa, E. A. (2009). Understanding antimicrobial activities of phytochemicals against multidrug resistant bacteria and biofilms. Nat. Prod. Rep. 26, 746–757. doi: 10.1039/b821648g
Simões, M., Simões, L. C., Vieira, M. J. (2010). A review of current and emergent biofilm control strategies. LWT Food Sci. Technol. 43, 573–583. doi: 10.1016/j.lwt.2009.12.008
Srey, S., Jahid, I. K., Ha, S. D. (2013). Biofilm formation in food industries: A food safety concern. Food Control 31, 572–585. doi: 10.1016/j.foodcont.2012.12.001
StenforsArnesen, L. P., Fagerlund, A., Granum, P. E. (2008). From soil to gut: Bacillus cereus and its food poisoning toxins. FEMS Microbiol. Rev. 32, 579–606. doi: 10.1111/j.1574-6976.2008.00112.x
Stepanović, S., Vukovic, D., Dakic, I., Savic, B., Svabic-Vlahovic, M. (2000). A modified microtiter-plate test for quantification of 426 staphylococcal biofilm formation. J. Microbiol. Methods 40, 175–179. doi: 10.1016/S0167-7012(00)00122-6
Stepanović, S., Vuković, D., Hola, V., Bonaventura, G. D., Djukić, S., Ćirković, I., et al. (2007). Quantification of biofilm in microtiter plates: overview of testing conditions and practical recommendations for assessment of biofilm production by staphylococci. Apmis 115, 891–899. doi: 10.1111/j.1600-0463.2007.apm_630.x
Stiefel, P., Rosenberg, U., Schneider, J., Mauerhofer, S., Maniura-Weber, K., Ren, Q. (2016). Is biofilm removal properly assessed? Comparison of different quantification methods in a 96-well plate system. Appl. Microbiol. Biotechnol. 100, 4135–4145. doi: 10.1007/s00253-016-7396-9
Svensson, B., Monthan, A., Shaheen, R., Andersson, M. A., Salkinoja-Salonen, M., Christiansson, A. (2006). Occurrence of emetic toxin producing Bacillus cereus in the dairy production chain. Int. Dairy J. 16, 740–749. doi: 10.1016/j.idairyj.2005.07.002
Tirloni, E., Stella, S., Bernardi, C., Mazzantini, D., Celandroni, F., Ghelardi, E. (2020). Identification and pathogenic potential of Bacillus cereus strains isolated from a dairy processing plant producing PDO taleggio cheese. Microorganisms 8, 949. doi: 10.3390/microorganisms8060949
Wijman, J. G., de Leeuw, P. P., Moezelaar, R., Zwietering, M. H., Abee, T. (2007). Air-liquid interface biofilms of Bacillus cereus: formation, sporulation, and dispersion. Appl. Environ. Microbiol. 73, 1481–1488. doi: 10.1128/AEM.01781-06
Wolf, G., Crespo, J. G., Reis, M. A. (2002). Optical and spectroscopic methods for biofilm examination and monitoring. Rev. Environ. Sci. Biotechnol. 1, 227–251. doi: 10.1023/A:1021238630092
Yan, F., Yu, Y., Wang, L., Luo, Y., Guo, J. H., Chai, Y. (2016). The comER gene plays an important role in biofilm formation and sporulation in both Bacillus subtilis and Bacillus cereus. Front. Microbiol. 7. doi: 10.3389/fmicb.2016.01025
Yuan, L., Sadiq, F. A., Burmølle, M., Liu, T., He, G. (2018). Insights into bacterial milk spoilage with particular emphasis on the roles of heat-stable enzymes, biofilms, and quorum sensing. J. Food Prot. 81, 1651–1660. doi: 10.4315/0362-028X.JFP-18-094
Keywords: biofilm, Bacillus, polystyrene, stainless-steel, SEM, whole genome sequencing
Citation: Catania AM, Di Ciccio P, Ferrocino I, Civera T, Cannizzo FT and Dalmasso A (2023) Evaluation of the biofilm-forming ability and molecular characterization of dairy Bacillus spp. isolates. Front. Cell. Infect. Microbiol. 13:1229460. doi: 10.3389/fcimb.2023.1229460
Received: 26 May 2023; Accepted: 14 July 2023;
Published: 31 July 2023.
Edited by:
Olivier Habimana, Guangdong Technion-Israel Institute of Technology (GTIIT), ChinaReviewed by:
Lisa Gorski, Agricultural Research Service (USDA), United StatesCopyright © 2023 Catania, Di Ciccio, Ferrocino, Civera, Cannizzo and Dalmasso. This is an open-access article distributed under the terms of the Creative Commons Attribution License (CC BY). The use, distribution or reproduction in other forums is permitted, provided the original author(s) and the copyright owner(s) are credited and that the original publication in this journal is cited, in accordance with accepted academic practice. No use, distribution or reproduction is permitted which does not comply with these terms.
*Correspondence: Pierluigi Di Ciccio, pierluigialdo.diciccio@unito.it
Disclaimer: All claims expressed in this article are solely those of the authors and do not necessarily represent those of their affiliated organizations, or those of the publisher, the editors and the reviewers. Any product that may be evaluated in this article or claim that may be made by its manufacturer is not guaranteed or endorsed by the publisher.
Research integrity at Frontiers
Learn more about the work of our research integrity team to safeguard the quality of each article we publish.