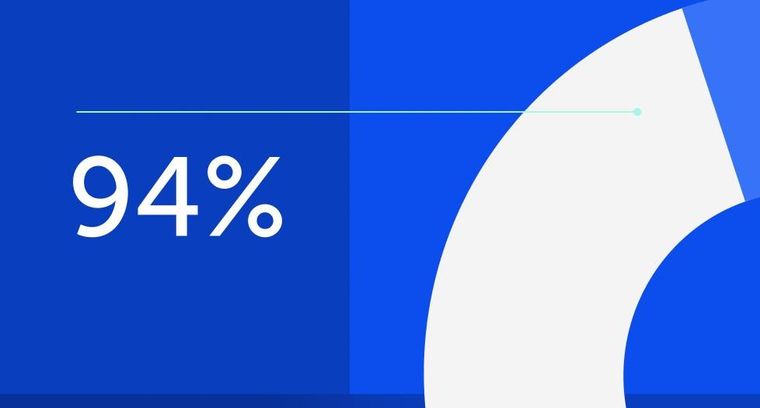
94% of researchers rate our articles as excellent or good
Learn more about the work of our research integrity team to safeguard the quality of each article we publish.
Find out more
REVIEW article
Front. Cell. Infect. Microbiol., 16 August 2023
Sec. Clinical Microbiology
Volume 13 - 2023 | https://doi.org/10.3389/fcimb.2023.1224778
This article is part of the Research TopicDesign and development of new therapeutics against infectious diseases using computational and experimental approachesView all 13 articles
Green synthesis of NPs has gained extensive acceptance as they are reliable, eco-friendly, sustainable, and stable. Chemically synthesized NPs cause lung inflammation, heart problems, liver dysfunction, immune suppression, organ accumulation, and altered metabolism, leading to organ-specific toxicity. NPs synthesized from plants and microbes are biologically safe and cost-effective. These microbes and plant sources can consume and accumulate inorganic metal ions from their adjacent niches, thus synthesizing extracellular and intracellular NPs. These inherent characteristics of biological cells to process and modify inorganic metal ions into NPs have helped explore an area of biochemical analysis. Biological entities or their extracts used in NPs include algae, bacteria, fungi, actinomycetes, viruses, yeasts, and plants, with varying capabilities through the bioreduction of metallic NPs. These biosynthesized NPs have a wide range of pharmaceutical applications, such as tissue engineering, detection of pathogens or proteins, antimicrobial agents, anticancer mediators, vehicles for drug delivery, formulations for functional foods, and identification of pathogens, which can contribute to translational research in medical applications. NPs have various applications in the food and drug packaging industry, agriculture, and environmental remediation.
Nanoparticles (NP) vary in size from 1 to 100 nm with a large surface-to-volume ratio and are currently used in translation research technology. The biological NPs employed are ecofriendly, cost effective, and have attracted substantial attention. Nanotechnology comprises various fields, including biological sciences, chemistry, physics, material science, engineering, and computational science. It has applications in various fields, such as optics, space industries, cosmetics, biomedical, chemical industries, electronics, mechanics, environmental remediation, food and feed, health care, photoelectron chemistry, numerous engineering fields, and material science (Christian et al., 2008). Nanotechnology is the most promising field in integrated engineering, physics, medicine, chemistry, and biology, and has widespread applications with increased demand for industrial-scale production of nanomaterials (NMs). However, concerns have been raised regarding the environmental safety of chemically synthesized NPs. The origin and synthesis of these chemical NPs have caused an enormous burden on the environment because of their toxins, which have harmful effects on animals and humans (Wiley et al., 2005; Vo-Dinh, 2007). Physiochemical techniques to produce NPs of metals and metal oxides require the use of corrosive and toxic reducing agents such as sodium borohydride and hydrazine hydrate, which adversely affect the atmosphere (Mandal et al., 2006). Thus, alternative methods for synthesizing harmless NPs using moderate solvents are eco-friendly reducers and stabilizers with experimental parameters or biological material applications. The most eco-friendly are the use of plants, fungal or bacterial extracts, lysates, or biomolecules, which are widely used due to their minimal side effects (Figure 1). Numerous microbes act as potential sustainable precursors that are eco-friendly and can produce stable and well-functionalized NPs (Baroumand Moghaddam et al., 2015). Thus, such environmentally safe techniques used for the synthesis of NPs are also referred to as ‘green nanotechnology’ or “clean-technology” that are feasible alternatives to chemical methods. Furthermore, nanoparticles conjugated with natural biomolecules exhibit improved bioavailability and minimal side effects. They are not only smaller in size with higher permeability, but are also important reducing and stabilizing agents and show excellent antioxidant activity. Nanoparticles serve as potential antimicrobial agents because of their affinity toward sulfur-rich amino acids, adherence to the microbial cell wall by electrostatic attraction, and disruption of the microbial cytoplasmic membrane and nucleic acids. They possess anticancer activity owing to the initiation of oxidative stress, cellular DNA damage, and lipid peroxidation. Additionally, biological synthesis is eco-friendly, cost-effective, and fast, and unlike compounds or their derivatives, it is not harmful, thus minimizing pollution. Therefore, the use of green synthetic methods holds promise as safe alternatives for healthcare and bioremediation applications. Currently, nanotechnology, being an indispensable part of the healthcare, research and innovation sector in recent years is used extensively (Manjula et al., 2022). Nanocompounds have been promising antimicrobial agents, anti-cancer mediators, vehicles for drug delivery, formulations for functional foods, in the identification of pathogens, and in the food and drug packaging industry.
Engineered nanomaterials (NMs) have gained the utmost importance in daily life and have been used in cosmetics, food packaging materials, biosensors, drug delivery systems, and therapies. Subsequently, their size is similar to that of biological macromolecules with antibacterial and anti-odor properties, which can be widely used in wound dressing, antimicrobial coatings, and detergents. However, despite innumerable applications, the biodegradation of NPs and the effect of their accumulation in the environment remain questionable. The accumulation of bio-degraded NPs within cells leads to intracellular changes, causing alterations in organelle integrity or gene alterations. Although NMs continue to be used extensively, it is important to address their toxicity, environmental impact, and possible undesirable side effects (Figure 1). In cancer nanotechnology, monoclonal antibodies, peptides, or small molecules targeting tumor ligands can be induced using NPs to target tumor antigens and tumor vasculature with high specificity and affinity. Recent developments have used bioaffinity NP probes for cellular and molecular imaging to target NP drugs for the treatment of cancers and integrated nanodevices for the early detection of cancers (Gao et al., 2004). Currently, green synthesis of nanoparticles is a novel alternative method. In addition, nanoparticles conjugated with natural biomolecules, which are easily taken up by human cells, have been reported to be more efficacious. In parallel, they act as reducers and stabilizers with smaller sizes and higher penetrating capacities proving themselves to have excellent therapeutic activity.
The synthesis of magnetic NPs has attracted a great deal of research interest owing to the utilization of synthetic, non-toxic, and biocompatible materials. The hydrophilic polymer, starch (~20% amylase) plays a substantial role in stabilizing and dispersing NPs, as observed in the synthesis of magnetite NPs (Fe3O4) NPs achieved by sodium alginate biopolymer with a redox-based hydrothermal process using FeCl3·6H2O and urea as a precursor (Jegan et al., 2011).
NP synthesis. The use of ascorbic acid as a super-paramagnetic iron oxide NPs results in a stable dispersion with an advantage in medical applications. The coated NPs were spherical with a mean particle size of 5 nm, as observed by transmission electron microscopy (TEM) (Sreeja et al., 2014).
Biological amines such as L-cysteine, L-arginine, L-glutamine, and L-glutamic acid are functionalized by magnetite NPs to produce FeNPs by a method that involves wet chemical co-precipitation along with the effect of pH (Siskova et al., 2013) (Table 1).
Table 1 Biosynthetic methods of various kinds of nanoparticles along with their morphology, size, and applications.
The synthesis of Fe-NPs from natural precursors of Fe, such as hemoglobin and myoglobin, by a single-phase chemical reaction produces Fe-NPs that are stable at room temperature (RT). This strategy is an important approach for the fabrication of bioconjugated NP for biological applications (Sayyad et al., 2012) (Figure 2).
Figure 2 Green synthesis of nanomaterials using various biological components and their applications.
A reducing agent, D-glucose and gluconic acid as a stabilizer are used for the synthesis of polycrystalline Fe3O4-NPs (Table 1). Synthesis of Fe3O4- NP is coated with glucose and gluconic acid by hydrothermal reduction of Fe3+ ions to Fe2+ followed by capping of NPs to enhance and stabilize the properties (Ramakrishnappa et al., 2022).
Fe3O4-NPs are exceedingly crystalline and monodispersed and are synthesized by ultrasonication of an aqueous suspension of tannic acid at an optimal pH of 10. These synthesized NPs were spherical with a size less than 10 nm, as revealed by high-resolution transmission electron microscopy (HR-TEM) (Herrera-Becerra et al., 2010). Figure 2 shows the green synthesis of nanomaterials from different biological agents.
Iron nanomaterials (Fe-NPs) are biosynthesized most commonly by iron-reducing bacteria, such as Actinobacteria sp., under aerobic conditions that form spherical iron oxide nanoparticles (FeO-NPs). These microbes synthesized magnetic NPs when exposed to an aqueous ferric salt solution under aerobic conditions within 48–72 h. The FeO-NPs that exhibited a color change from medium to brown were analyzed by TEM, X-ray diffraction (XRD), Fourier Transform Infrared Spectroscopy (FTIR), magnetic measurements, and other methods. The synthesis of magnetic NPs in bacteria such as Actinobacter sp. is a highly complex phenomenon that involves iron reductase enzymes in the presence of excess iron salts (Bharde et al., 2008). Iron reductase reduces Fe3+ to Fe2+ extracellularly to produce magnetic NPs. Additionally, the activity of Fe3+ reductase was confirmed by a ferrisiderophore reductase assay, indicating extracellular synthesis (Bharde et al., 2005) (Table 2).
Magnetic NPs are produced extracellularly by a combination of fungi of different sizes, such as Fusarium oxysporum and Verticillium sp., using ferric and ferrous salts at room temperature. These microbes secrete extracellular cationic proteins that hydrolyze anionic iron complexes to crystalline magnetite particles at low temperatures via means of spontaneous magnetization and ferrimagnetic transition signatures (Bharde et al., 2006). Various species of fungal and bacterial strains, such as Pochonia chlamydosporium, Aspergillus fumigatus, A. wentii, Curvularia lunata, Chaetomium globosum, Alcaligenes faecalis, Bacillus faecalis, and Bacillus coagulans, have been tested to produce iron NP (Kaul et al., 2012) (Table 2).
The biosynthesis of Fe3O4- NP involves ferric chloride reduction in macroalgae of brown seaweed, Sargassum muticum extracts containing sulfated polysaccharides that help facilitate the reduction of iron salt. XRD analysis of the NPs synthesized using this method indicated the presence of crystalline and cube-shaped particles (Table 2). The TEM images of these microalgae revealed the presence of intracellular and extracellular iron. FTIR analysis confirmed that carbonyl and amine groups reduced the iron salts (Mahdavi et al., 2013). Algae are potential phototrophic organisms known for the greater production of several metabolites, pigments, polysaccharides, fibers, and secondary metabolites, yielding larger production of NPs. Hence, these biofactories are expected to contribute more competent and harmless bioactive compounds for various applications.
Green synthesis of metallic NPs from various plant parts, such as leaves, stems, roots, and seeds, is the simple, inexpensive, and reproducible. However, plants as natural sources will certainly produce the added advantage of stable metal NPs. Hence, green-synthesized NPs have proven to be the best candidates for fast and large-scale production in comparison to microbes that require specific environmental conditions (Kalaiarasi et al., 2010). Extracts from the seeds, fruits, leaves, stems of different herbs and higher plants are rich in antioxidants that help boost the capacity of NPs. Therefore, the use of plant-based phytochemicals for the synthesis of NPs unites natural/plant sciences and nanotechnology, and enables safe, environmentally friendly, justifiable, and economically feasible green technologies (Mayegowda et al., 2022a; Mayegowda et al., 2023).
Fe-NPs were produced using green tea (Camellia sinensis) extracts, which are rich in polyphenols. Polyphenols are excellent reducing and capping agents, resulting in the formation of stable, green, nanoscale zero-valent iron particles (Rotti et al., 2023). Similarly, crystalline monodisperse magnetite [Fe3O4] NPs were synthesized using the carob leaf in a one-step reaction (Awwad and Salem, 2012). FeO/Fe3O4 NPs were synthesized using pomegranate (Punica granatum) leaf extract and heat-killed yeast (Yarrowia lipolytica), as biosorbents to remove hexavalent chromium. Mössbauer spectroscopy revealed the presence of FeO/Fe3O4, and SEM images displayed even the dispersal of Fe-NPs on the outer surface of yeast cells (Rao et al., 2013).
Pd and Fe-NPs were prepared using aqueous fruit extracts of Terminalia chebula. Stable Fe-NPs were synthesized by the reduction of a FeSO4·7H2O solution by T. chebula extract (Kumar et al., 2013). Fe3O4 NPs were synthesized from the fruit extracts of Passiflora tripartita var. mollissima are 22.3 nm in size and show significant nanocatalytic activity (Kumar et al., 2014).
A previous study demonstrated the production of FeO NPs using Syzygium cumini (Malabar plum) as a stabilizing agent. The seed extract was used as a reducing agent, and sodium acetate was used as an electrostatic stabilizing agent. The presence of polyphenols, such as flavonoids, was confirmed by FTIR spectroscopy, which is important in serving the role as reducing agents (Venkateswarlu et al., 2014).
NPs also exhibit antibacterial properties. Silver (Ag) NPs continuously release Ag ions that adhere to the bacterial cell wall and cell membrane, leading to disruption of the bacterial envelope (Khorrami et al., 2018). Once inside the cell, Ag deactivates respiratory enzymes by producing reactive oxygen species (ROS) (Ramkumar et al., 2017). The accumulation of ROS causes damage to DNA, RNA, and proteins, resulting in cell death. The Ag released from NPs binds the sulfur and phosphorus of DNA and blocks replication and cell division, eventually leading to cell death. Ag ions also cause denaturation of ribosomes in the cytoplasm, leading to inhibition of protein synthesis (Durán et al., 2016; Jadimurthy et al., 2022) (Figure 3).
Figure 3 Illustration of the mechanism of action of nanoparticles in the destruction of bacterial cells. 1. Metal nanoparticles destroyed the cell wall and cell membrane. 2. Ions generated from metal NP bind to and denature ribosomes. 3. ROS production. 4. Accumulation of nanoparticles rupturing the cell membrane 5. Alteration of the protein structure leading to damage. 6. DNA denaturation. 7. Metal ions that inhibit the electron transport chain. 8. Metal NPs bind to the receptor, causing a change in confirmation.
The accumulation of AgNPs alters the structure of the cell membrane and promotes cell lysis (Liao et al., 2019). The surface charge of the NP plays a significant role in determining its interaction with the cell membrane. Cationic NPs infiltrate cells and cause extensive damage. Although anionic NPs do not penetrate the plasma membrane, they destabilize at specific concentrations. While this property of charged NPs suggests potential damage to human cells, it offers opportunities for use as a vehicle for drug delivery to cancerous cells. Denaturation of the cytoplasmic membrane often leads to the rupture of organelles, which ultimately results in cell lysis. Bacterial signal transduction involves phosphorylation events that enable bacteria to sense, adapt, and respond to environmental changes. NPs cause dephosphorylation and disruption of signal transduction, leading to apoptosis (Li et al., 2019). Ag NPs are spherical or quasi spherical and can easily release Ag ions owing to their large surface (Shanmuganathan et al., 2018). To avoid agglomeration, capping agents are used to coat the NPs, which modifies their surfaces and affects their dissolution. The presence of organic or inorganic in the medium can also be responsible for the dissolution of NPs by aggregation.
It has been demonstrated that Ag NPs release Ag ions in the aqueous solution and this is quite faster when the pH is acidic (below 5) than in neutral solution (Jacob et al., 2019). Differential mode of action for NPs has been reported for Gram-positive and Gram-negative bacteria and has been attributed to the presence of peptidoglycan in Gram-positive bacteria which hinders efficient penetration (Meikle et al., 2020). NPs with a size less than 10 nm can directly penetrate the cell and alter cell permeability, enter bacteria, and cause cellular destruction, suggesting a direct correlation between NP uptake and antibacterial properties (Noronha et al., 2017). Hence, Ag NPs have a great potential as antibacterial agents. However, the application of Ag NPs as biofilm inhibitors warrants further investigation (Pugazhendhi et al., 2018). Magnesium oxide NPs have been reported to possess anti-biofilm and anti-adhesion potential, which are efficient against drug-resistant bacteria (Hayat et al., 2018).
Repetitive damage to the environment due to hazardous chemicals, such as excessive use of pesticides, poses a great threat to agriculture worldwide. The use of NPs to target plant pathogens appears to be a safe alternative to chemically synthesized pesticides (Kim et al., 2012). Ag NPs, with varied modes of action, are suggested to be safe agents against plant pathogens, particularly commercially relevant fungal pathogens, as compared to artificial antifungal agent (Manjula et al., 2023). Metal NPs are non-toxic, eco-friendly, and widely used as disinfectants and investigational antifungal mediators (Borehalli Mayegowda et al., 2022). Zinc NPs have potential applications in the pharmaceutical sector, as potent antimicrobial agents and in water disinfection. Studies demonstrating the antifungal activity of Ag NPs are limited. Kim and colleagues have however, suggested a concentration dependent inhibitory activity, probably due to saturation of fungal hyphae with higher density NPs leading to deactivation of the disease-causing fungi (Kim et al., 2012).
While the effect of Ag ions on fungi is limited, reports suggest their inhibitory effect on DNA replication (Feng et al., 2000) and impairment of ribosomal attachment, blocking protein synthesis, enzymes, and additional proteins involved in the production of ATP (Yamanaka et al., 2005). Ag NPs synthesized using ribose sugar and sodium dodecyl sulfate acting like reducing agent and capping agent, respectively demonstrating antifungal activity against highly resistant human pathogenic fungi such as Candida albicans and Candida tropicalis (Mallmann et al., 2015). Similar results have been reported by other researchers (Kim et al., 2009). Fungal cells maintain an ion gradient, trehalose and glucose protects the biological viability of cells from protein denaturation caused by environmental stress such as heat, cold, high pH, dehydration, oxidative stress, and lethal agents (Alvarez-Peral et al., 2002). NPs disrupt the membrane structure and permeability, leading to leakage of intracellular contents and loss of membrane potential. TEM observations revealed the formation of pits in the fungal membrane when treated with Ag ions, leading to cell cycle arrest and cell death in C. albicans. Additionally, destruction of the membrane and inhibition of budding have been noted (Endo et al., 1997). Palladium NPs demonstrated effective antifungal activity against Colletotrichum gloeosporioides and F. oxysporum although in a size-dependent manner (Osonga et al., 2020). Therefore, NPs may induce antifungal activity by disrupting cellular integrity, generating reactive species, and creating osmotic imbalances in pathogens.
While several viral diseases have been eradicated, emerging threats from novel viruses cannot be ignored because of their adaptability and mutagenic ability (Esteban, 2010). Hence, these viruses pose a continuous challenge to the scientific community. Enfuvirtide is a synthetic peptide drug, approved by the US Food and Drug Administration that targets a specific HIV protein named the gp41 coding envelope protein and prevents its fusion (Galdiero et al., 2011).
Under such challenging circumstances, NPs have been developed as promising antiviral agents owing to their increased surface area and unique chemical and physical properties (Elechiguerra et al., 2005). NPs block viral infections by having their mode of action at the time of attachment as well as entry by obstructing polyvalent interactions across viral surface components in interaction with host cell membrane receptors (Figure 4) (Helenius, 2007). Previous studies have demonstrated the efficacy of NPs against HIV-1 (Lara et al., 2010a; Lara et al., 2010b), hepatitis B virus (Lu et al., 2008), herpes simplex virus type 1 (Baram-Pinto et al., 2009), monkey pox virus (Rogers et al., 2008), and influenza virus (Papp et al., 2010). While the interaction and efficacy of NPs against viruses were largely dependent on NP size, they also interacted at specific sites. NPs also bind strongly to the sulfur-containing residues of GP120 (Lara et al., 2010a). Both Ag and Au NPs have promising antiviral properties, particularly against enveloped DNA/RNA viruses. A recent study on COVID-19 viral disease explains about the neurotropism property demonstrating the application of NPs by inhibiting replication by cellular transcytosis blockade when given in the form of encapuslted particles (Ren et al., 2021).
Figure 4 The mode of action of Ag nanoparticles through attachment to the surface proteins and viral genome leads to the blockage of replication.
NPs are classified based on their composition into three classes: a) organic-based, which comprises proteins, lipids, carbohydrates, polymers, or any other organic compounds that are nontoxic, ecofriendly, such as dendrimers, liposomes, micelles, and protein complexes (Bharde et al., 2005; Bharde et al., 2008; Rotti et al., 2023), and carbon-based are solely made of carbon such as fullerenes, carbon black NPs, and quantum dots (Savasari et al., 2015). Inorganic nanoparticles (NPs) including metals, ceramics, and semiconductors. These inorganic metal NPs can be synthesized from monometallic or bimetallic alloys (Mayegowda et al., 2022b). Owing to the varying properties of NPs, different techniques can be used for characterization and analysis to determine their potential applications. Morphological and topological features were established to check the size, shape, dispersity, localized, surface topography, surface area and porosity of NPs, which0 can be determined by TEM and SEM (Herrera-Becerra et al., 2010; Sreeja et al., 2014). Other techniques include dynamic light scattering (DLS) and nanoparticle tracking analysis (NTA), which involve the light interference measured on the suspended NPs for Brownian motion with the correlation of its velocity with their size using the Stokes–Einstein equation (Bharde et al., 2008). However, the structural and chemical characterization of NPs can be carried out by XRD, energy-dispersive X-ray spectroscopy (EDX), high-angle annular dark-field imaging (HAADF), and FTIR for the phase, composition, crystallinity, chemical state (oxidation), functional groups, surface charges, and electrochemical characteristics. Zeta potential analysis can be used to determine the surface charge of NPs (Manjula et al., 2023; Mayegowda et al., 2023). The characterization of NPs for optical, electronic, and electrical properties was carried out by Raman spectroscopy and SERS for absorption, luminescence, electronic state, photoactivity, and electrical conductivities. UV–vis and photoluminescence spectroscopy, which transitions electrons from the ground to an excited state, as measured by absorption spectroscopy (Manjula et al., 2023). Magnetic properties have great advantages and are quantified by magnetic force microscopy or electron spin resonance spectroscopy.
Although NPs hold tremendous promise owing to their ease of synthesis and wide industrial applications, their synthesis using chemical approaches results in toxic side effects. Therefore, green synthesis is promising as an alternative and safe process. The following section highlights the commercial, medicinal, and other applications of green synthetic NPs.
In agriculture, nanotechnology is primarily used for the synthesis of pesticides and fertilizers. NPs can be used as fertilizers to facilitate crop improvement, seed germination, and root growth, while also reducing eco-toxicity. Ag NPs were shown to improve wheat growth and yield. One of the essential micronutrients for plant metabolic processes is zinc, which is involved in the synthesis of auxins such as indole acetic acid and carbohydrates, and in the formation of chlorophyll. Many NPs have been used in the form of pesticides, such as green peach aphids (Ghidan et al., 2018; Mayegowda et al., 2022b). Decreased plant mortality for granulomatosis with polyangiitis (GPA), a condition in which the blood vessels become swollen, was achieved by the synthesis of NPs using aqueous peel extracts of Punica granatum, leaf extracts of Olea europaea, and flower extract of Chamaemelum nobile. GPA infection and the absence of metal accumulation suggest the advantages of bio-NPs (Ghidan et al., 2017). Ag NPs also function as fungicides and have been effective against C. gloeosporioides which causes bitter rot in various crops (Aguilar-Méndez et al., 2010).
Nanotechnology in the food industry can escalate their shelf life of various foods and reduce their depletion due to contagious infestation (Pradhan et al., 2015). Encapsulation of biomolecules, such as lipids proteins, carbohydrates, and vitamins, using NPs can protect them from the acidic environment of the stomach and intestine and can improve their assimilation (Singh et al., 2017). However, the use of NPs in food packaging materials aids in the preservation and protection from several of these issues.
Essential oils obtained from organic compounds, organic acids, and bacteriocins have been extensively studied for use in polymeric matrices as antimicrobial packaging material (Gálvez et al., 2007). Many NPs, such as Ag, copper, biopolymer chitosan, and metal-oxide derived NPs, such as titanium oxide (TiO2) and zinc oxide (ZnO), have been shown to have antibacterial activity (Manjula et al., 2022). Nanobiosensors are used in the food industry for pathogen detection during processing (Mayegowda et al., 2022). Nano-biosensor can detect changes in the environment, such as moisture content or temperature fluctuations in storage rooms, microbial contamination, or product deprivation. NPs play a vital a role in the food industry, and their green synthesis offers additional advantages.
The use of NPs is most popular in the cosmetic industry. Leading brands such as L’Oreal, Avon, and Johnson and Johnson have patents on nanoparticles. NPs are employed in sunscreens, skin creams, skin lotions, and dye-based products such as fabric colors, skin tanning, and whitening lotions. Sunscreens are used as UV filters and provide broad UV protection with minimal or no adverse effects. NMs labeled in cosmetics can be similar to vesicles in cells, such as liposomes, nano-emulsions, and nano-capsules. Other solid lipid NP conjugates, include nanocrystals and NP with metal-like nanosilver and nanogold, dendrimers, cubosomes, liquid-based hydrogels, and buckyballs (Raj et al., 2012; Ananda et al., 2022). NPs utilized in cosmetics are generally chemically synthesized, but there is an emerging shift towards green synthesis. Xia et al. demonstrated the use of ivy plant-derived NPs as an alternative to oxide particles in blocking UV rays (Bezbaruah et al., 2022).
Recently, NPs have been used for detection, diagnosis, and tumor therapy. NPs are excellent tools for cancer drug delivery because of their small size, which facilitates targeted delivery. NPs have also been used to mitigate adverse effects of photodynamic cancer therapy. The hydrophilic antigenic drug Mosqurix® is used to treat malaria caused by Plasmodium falciparum and hepatitis B virus. This liposomal drug, modified as a phospholipid intrinsic adjuvant, has greater stability in the gastrointestinal tract for absorption. A nanogel made up of polyethylenimine along with cationic coated alginate, which is given intraperitonially, produces anti-ovalbumin IgG, which is promising for drug delivery (Adarsha et al., 2022). A dye used in photodynamic therapy migrates to the skin and eyes, leading to sensitivity. Partial encapsulation of the dye in NPs helped reduce sensitivity (Roy et al., 2003). Furthermore, NPs, such as gold NPs, have research applications, such as immunohistochemistry and identification of protein–protein interactions. Cao et al. (2003) synthesized a AuNP probe with catalytic activity for protein identification (Cao et al., 2003; Yadav et al., 2021). The extensive medical use of chemically made NPs has been reported to have adverse effects. Therefore, there has been a shift toward safer green synthetic bio-NPs. A study reported the use of nano-encapsulated AgNPs by green-synthesized pcDNA3.1/H5 for immunization through humoral and cell-mediated immune responses. Thus, green NPs can be used as an alternative DNA vaccine through nanotechnology for the oral administration of vaccines in a more effective way. AgNPs are an attractive and unique alternative delivery method for oral DNA vaccinations. NP-based vaccination showed a similar effect in the elucidation of the immune response as traditional vaccination procedures. In addition, it exhibits greater stability, cost-effectiveness, and target specificity in minimizing various disease types (Jazayeri et al., 2012).
Fungal based synthesized ZnNPs showed excellent antimicrobial property against pathogenic fish bacteria in comparison with chemical ZnNPs. This study also highlights the increase in immune response in organisms by the released Zn2+ hindering active transport as well as inhibiting bacterial enzymes in metabolism, leading to the release of ROS, causing death of the organism (El-Saadony et al., 2021). Similarly, biosynthesis of NPs from microbes and plant extracts has also shown vast application in modulating immune responses through innate and adaptive immunity across conditions, such as inflammation, cell differentiation and signaling during cancer, autoimmune disease, and allergic reactions. Hence, describing the ecofriendly and safer approach to green NPs is a novel therapeutic approach in drug delivery and therapeutics (Cai et al., 2022).
Despite the innumerable advantages of NPs, as listed above, they can still be toxic at higher concentrations, if overused, or accumulate within the system. However, the toxicity levels in the environment depend largely on the type of NPs and the properties that have been used.
The size of the NP decreased from 30 to 3 nm, and the number of surface molecules increased from 10% to 50%. Metal NPs are the most common type of NPs employed in all industries. Studies on cellular uptake of gold NPs indicated their extracellular aggregation followed by enmass transport into the cells with resultant reactive species production and cytotoxicity (Figure 5) (Goodman et al., 2004; Chithrani et al., 2006). Studies have shown that the formation of abnormal actin filaments results in decreased cell adhesion, proliferation, and motility (Pernodet et al., 2006). While gold nanospheres capped with citrate were non-toxic to baby hamster kidney and human hepatocellular liver carcinoma cells, they were toxic to human carcinoma lung cell lines at certain concentrations, signifying their effect on cell type (Pernodet et al., 2006).
Large concentrations of Ag in atmospheric air led to breathing abnormalities and lung, throat, and stomach discomforts. Ag n skin contact can occasionally lead to insignificant allergic conditions, such as rashes, swelling, and inflammation. However, several studies have been conducted (Carlson et al., 2008). However, studies have not proven the toxicity of spherical AgNPs and Ag nano-prisms in human skin keratinocytes. TiO2 NPs induce oxidative stress by inducing changes in gene expression (Park et al., 2008). ZnO also induced toxicity by generating reactive oxygen species, oxidant injury, and inflammation, leading to cell death (El-Saadony et al., 2021).
In addition to humans, other animals and microbes such as zebra fish, daphnids, and algal species were exposed to silver, copper, aluminum, nickel, cobalt, and TiO2 nanoparticles. Griffitt et al. (2008) detected toxicity levels in aquatic organisms owing to the presence of metallic NPs. In contrast, no toxicity was observed for TiO2 NPs. These results were corroborated by another study on zebrafish embryos that suggested the dose-dependent developmental toxicity of Ag NPs (Asharani et al., 2008). The toxicity level of AgNPs in the aquatic environment can be denoted by the concentration accumulated by aquatic organisms. The liver is the initial accumulator organ for NPs in these aquatic organisms, followed by the gills, intestine, and muscles, causing cell necrosis and lysis in the intestinal villi, followed by progressive release of Ag ions (Liu et al., 2015). AgNPs are frequently used in apparel owing to their antimicrobial properties. However, the release of Ag, in both colloidal and ionic forms, from the fabric during washing causes its accumulation in the wastewater, leading to toxicity (Benn and Westerhoff, 2008).
ZnO NPs are readily used in cosmetics and, as such, are a large constituent of factory run off from the cosmetic industries. These NPs are toxic to Lolium perenne (ryegrass). ZnO-NP, along with the ryegrass biomass, has been able to suggestively reduce, characterized by shrinking the root tips, and highly vacuolated or collapsed epidermal or cortical cells (Lin and Xing, 2008). However, a minor reduction in root cells was detected upon exposure to uncoated alumina NPs (Yang and Watts, 2005).
Recently, there has been a burst of commercial applications for NPs. However, green synthesis of NPs is still in its infancy. Green synthesis offers a safe mechanism for producing nontoxic NPs with additional beneficial effects. Green synthesis has already been applied in several fields owing to the use of natural alternatives. Bio-NPs have been used as artificial food colors, strengthening agents for skincare products, antimicrobial agents embedded in fabrics, core molecules for drugs, and therapeutic molecules. However, their global application is limited by technology. Further research in this nascent field is warranted. Thus, green synthesis has the potential to reduce environmental pollution by employing commercially viable yet safe biomaterials.
NPs are generally used for their antimicrobial properties, which can act against bacteria, fungi, and certain viruses. This generally results from the metal component, but bio-NPs also contain other biomolecules as vital components. The antimicrobial properties of NPs are used in various industries, such as food packaging, active agents in skincare products, disease treatment, and drug delivery. It should be noted that the overuse and extensive deployment of NPs could lead to toxicity owing to the accumulation of metals and ions being released. However, lethal effects on humans at the currently used concentrations have not been reported. In conclusion, green synthesis is a largely positive and significant venture in all fields of science, in which the use of environmentally friendly resources and biodegradable materials in the synthesis of NPs will undoubtedly lead to an eco-friendly era with reduced industrial and environmental pollution.
SB, AR, and MG conceptualized, designed, and wrote the initial manuscript draft the manuscript. SP, SA, MAlm, MAll, NSA and RS prepared the figures and tables, edited, and revised the manuscript critically. Final manuscript has been approved by all the authors.
The authors extend their appreciation to the Deanship of Scientific Research at King Khalid University for supporting this work through research groups program under grant number RGP.2/273/44.
The authors declare that the research was conducted in the absence of any commercial or financial relationships that could be construed as a potential conflict of interest.
All claims expressed in this article are solely those of the authors and do not necessarily represent those of their affiliated organizations, or those of the publisher, the editors and the reviewers. Any product that may be evaluated in this article, or claim that may be made by its manufacturer, is not guaranteed or endorsed by the publisher.
Adarsha, J. R., Ravishankar, T. N., Ananda, A., Manjunatha, C. R., Shilpa, B. M., Ramakrishnappa, T. (2022). Hydrothermal synthesis of novel heterostructured Ag/TiO 2 /CuFe 2 O 4 nanocomposite: Characterization, enhanced photocatalytic degradation of methylene blue dye, and efficient antibacterial studies. Water Environ. Res. 94 (6), e10744. doi: 10.1002/wer.10744
Aguilar-Méndez, M. A., Martín-Martínez, E. S., Ortega-Arroyo, L., Coblan-Portillo, G., Sanchez-Espindola, E. (2010). Synthesis and Characterization of silver nanoparticles: effect on phytopathogen Colletotrichum gloesporoides. J. Nanoparticle Re-search; 152, 404–416. doi: 10.1007/s11051-010-0145-6
Alvarez-Peral, F. J., Zaragoza, O., Pedreno, Y., Argüelles, J. (2002). Protective role of trehalose during severe oxidative stress caused by hydrogen peroxide and the adaptive oxidative stress response in Candida albicans. Microbiology 148, 2599–2606. doi: 10.1099/00221287-148-8-2599
Ananda, A., Ramakrishnappa, T., Archana, S., Reddy Yadav, L. S., Shilpa, B. M., Nagaraju, G., et al. (2022). Green synthesis of MgO nanoparticles using Phyllanthus emblica for Evans blue degradation and antibacterial activity. Materials Today: Proc. 49, 801–810. doi: 10.1016/j.matpr.2021.05.340
Asharani, P. V., Wu, Y. L., Gong, Z., Valiyaveettil, S. (2008). Toxicity of silver nanoparticles in zebrafish models. Nanotechnology 19, 255102. doi: 10.1088/0957-4484/19/25/255102
Awwad, A. M., Salem, N. M. (2012). A green and facile approach for synthesis of magnetite nanoparticles. Nanoscience Nanotechnology 2, 208–213. doi: 10.5923/j.nn.20120206.09
Baram-Pinto, D., Shukla, S., Perkas, N., Gedanken, A., Sarid, R. (2009). Inhibition of herpes simplex virus type 1 infection by silver nanoparticles capped with mercaptoethane sulfonate. Bioconjug. Bioconjugate Chem. 20, 1497–1502. doi: 10.1021/bc900215b
Baroumand Moghaddam, A., NamVar, F., Moniri, M., Azizi, S., Mohammad, R. (2015). Nanoparticles biosynthesized by fungi and yeast: A review of their preparation, properties , and medical application. Molecules 20, 16540–16565. doi: 10.3390/molecules200916540
Benn, T. M., Westerhoff, P. (2008). Nanoparticle silver released into water from commercially available sock fabrics. Environ. Sci. Technol. 42, 4133–4139. doi: 10.1021/es7032718
Bezbaruah, R., Chavda, V. P., Nongrang, L., Alom, S., Deka, K., Kalita, T., et al. (2022). Nanoparticle-based delivery systems for vaccines. Vaccines (Basel) 10, 1946. doi: 10.3390/vaccines10111946
Bharde, A. A., Parikh, R. Y., Baidakova, M., Jouen, S., Hannoyer, B., Enoki, T., et al. (2008). Bacteria-mediated precursor-dependent biosynthesis of superparamagnetic iron oxide and iron sulfide nanoparticles. Langmuir 24, 5787–5794. doi: 10.1021/la704019p
Bharde, A., Rautaray, D., Bansal, V., Ahmad, A., Sarkar, I., Yusuf, S. M., et al. (2006). Extracellular biosynthesis of magnetite using fungi. Small 2, 135–141. doi: 10.1002/smll.200500180
Bharde, A., Wani, A., Shouche, Y., Joy, P. A., Prasad, B. L. V., Sastry, M. (2005). Bacterial aerobic synthesis of nanocrystalline magnetite. J. Am. Chem. Soc. 127, 9326–9327. doi: 10.1021/ja0508469
Borehalli Mayegowda, S., Rashmi, R., Manjula, N. G., Sreekantha, A. (2022). “Bioremediation of Heavy Metal Contaminated Sites Using Phytogenic Nanoparticles,” in Phytonanotechnology. Eds. Shah, M. P., Roy, A. (Singapore: Springer Nature), 227–253. doi: 10.1007/978-981-19-4811-4_11
Cai, F., Li, S., Huang, H., Iqbal, J., Wang, C., Jiang, X. (2022). Green synthesis of gold nanoparticles for immune response regulation: Mechanisms, applications, and perspectives. J. Biomed. Materials Res. 110, 424–442. doi: 10.1002/jbm.a.37281
Cao, Y. C., Jin, R., Nam, J. M., Thaxton, C. S., Mirkin, C. A. (2003). Raman dye labeled nanoparticle probes for proteins. J. Am. Chem. Soc. 125, 14676–14677. doi: 10.1021/ja0366235
Carlson, C., Hussain, S. M., Schrand, A. M., Braydich-Stolle, L. K., Hess, K. L., Jones, R. L., et al. (2008). Unique cellular interaction of silver nanoparticles: size-dependent generation of reactive oxygen species. J. Phys. Chem. B.; 112 (43), 13608–13619. doi: 10.1021/jp712087m
Chithrani, B. D., Ghazani, A. A., Chan, W. C. W. (2006). Determining the size and shape dependence of gold nanoparticle uptake into mamMalian cells. Nano Lett. 6, 662–668. doi: 10.1021/nl052396o
Christian, P., Von der Kammer, F., Baalousha, M., Hofmann, T. (2008). Nanoparticles: Structure, properties, preparation and behaviour in environmental media. Ecotoxicology 17, 326–343. doi: 10.1007/s10646-008-0213-1
Dorniani, D., Hussein, M. Z., Kura, A. U., Fakurazi, S., Shaari, A. H., Ahmad, Z. (2012). Preparation of Fe3O4 magnetic nanoparticles coated with gallic acid for drug delivery. Int. J. Nanomedicine 7, 5745–5756. doi: 10.2147/IJN.S35746
Durán, N., Nakazato, G., Seabra, A. (2016). Antimicrobial activity of biogenic silver nanoparticles, and silver chloride nanoparticles: an overview and comments. Appl. Microbiol. Biotechnol. 100 (15), 6555–6570. doi: 10.1007/s00253-016-7657-7
Elechiguerra, J. L., Burt, J. L., Morones, J. R., Camacho-Bragado, A., Gao, X., Lara, H. H., et al. (2005). Interaction of silver nanoparticles with HIV-1. J. Nanobiotechnology 29, 3–6. doi: 10.1186/1477-3155-3-6
El-Saadony, M. T., Alkhatib, F. M., Alzahrani, S. O., Shafi, M. E., El. Abdel-Hamid, S., Taha, T. F., et al. (2021). Impact of mycogenic zinc nanoparticles on performance, behavior, immune response, and microbial load in Oreochromis niloticus. Saudi J. Biol. Sci. 28, 4592–4604. doi: 10.1016/j.sjbs.2021.04.066
Endo, M., Takesako, K., Kato, I., Yamaguchi, H. (1997). Fungicidal action of aureobasidin A, a cyclic depsipeptide antifungal antibiotic, against Saccharomyces cerevisiae. Antimicrobial Agents Chemotherapy 41, 672–676. doi: 10.1128/AAC.41.3.672
Esteban, D. (2010). Mechanisms of viral emergence. Veterinary Res. 41, 38. doi: 10.1051/vetres/2010010
Feng, Q. L., Wu, J., Chen, G. Q., Cui, F. Z., Kim, T. N., Kim, J. O. (2000). A mechanistic study of the antibacterial effect of silver ions on Escherichia coli and Staphylococcus aureus. J. Biomed. Material Res. 52, 662–668. doi: 10.1002/1097-4636(20001215)52:4<662::aid-jbm10>3.0.co;2-3
Galdiero, S., Falanga, A., Vitiello, M., Cantisani, M., Marra, V., Galdiero, M. (2011). Silver nanoparticles as potential antiviral agent. Molecules 16 (10), 8894–8918. doi: 10.3390/molecules16108894
Gálvez, A., Abriouel, H., López, R. L., Omar, N. B. (2007). Bacteriocin-based strategies for food biopreservation. Int. J. Food Microbiol. 120, 51–70. doi: 10.1016/j.ijfoodmicro.2007.06.001
Gao, X., Cui, Y., Levenson, R. M., Chung, L. W.K., Nie, S. (2004). In vivo cancer targeting and imaging with semiconductor quantum dots. Nat. Biotechnol. 22, 969–997. doi: 10.1038/nbt994
Gao, S., Shi, Y., Zhang, S., Jiang, K., Yang, S., Li, Z., et al. (2008). Biopolymer-assisted green synthesis of iron oxide nanoparticles and their magnetic properties. J. Phys. Chem. C 112, 10398–10401. doi: 10.1021/jp802500a
Ghidan, A. Y., Al-Antary, T. M., Awwad, A. M., Ayad, J. Y. (2018). Physiological effect of some nanomaterials on pepper (Capsicum annuum L.) plants. Fresenius Environ. Bull. 27 (11), 7872–7878.
Ghidan, A. Y., Al-Antary, T. M., Salem, N. M., Awwad, A. M. (2017). Facile green synthetic route to the zinc oxide (ZnONPs) nanoparticles: Effect on green peach aphid and antibacterial activity. J. Agric. Sci. 9 (2), 131–138. doi: 10.5539/jas.v9n2p131
Goodman, C. M., McCusker, C. D., Yilmaz, T., Rotello, V. M. (2004). Toxicity of gold nanoparticles functionalized with cationic and anionic side chains. Bioconjugate Chem. 15, 897–900. doi: 10.1021/bc049951i
Griffitt, R. J., Luo, J., Gao, J., Bonzongo, J.-C., Barber, D. S. (2008). Effects of particle composition and species on toxicity of metallic nanomaterials in aquatic organisms. Environ. Toxicol. Chem. 27, 1972–1978. doi: 10.1897/08-002.1
Hayat, S., Muzammil, S., Rasool, M. H., Nisar, Z., Hussain, S. Z., Sabri, A. N., et al. (2018). In vitro antibiofilm and anti-adhesion effects of magnesium oxide nanoparticles against antibiotic resistant bacteria. Microbiol. Immunol. 62, 211–220. doi: 10.1111/1348-0421.12580
He, F., Zhao, D. (2005). Preparation and characterization of a new class of starch-stabilized bimetallic nanoparticles for degradation of chlorinated hydrocarbons in water. Environ. Sci. Technol. 39, 3314–3320. doi: 10.1021/es048743y
Helenius, A. (2007). “Virus Entry and Uncoating,” in Fields “Virology“, Fifth Edition (London, UK: LWW), 99–118.
Herrera-Becerra, R., Rius, J. L., Zorrilla, C. (2010). Tannin Biosynthesis of iron oxide nanoparticles. Appl. Phys. A 100, 453–459. doi: 10.1007/s00339-010-5903-x
Jadimurthy, R., Mayegowda, S. B., Nayak, S. C., Mohan, C. D., Rangappa, K. S. (2022). Escaping mechanisms of ESKAPE pathogens from antibiotics and their targeting by natural compounds. Biotechnol. Rep. 34, e00728. doi: 10.1016/j.btre.2022.e00728
Jazayeri, S. D., Ideris, A., Zakaria, Z., Shameli, K., Moeini, H., Omar, A. R. (2012). Cytotoxicity and immunological responses following oral vaccination of nanoencapsulated avian influenza virus H5 DNA vaccine with green synthesis silver nanoparticles. J. Controlled Release 161, 116–123. doi: 10.1016/j.jconrel.2012.04.015
Jegan, A., Ramasubbu, A., Saravanan, S., Vasanthkumar, S. (2011). One-pot synthesis and characterization of biopolymer—Iron oxide nanocomposite. Int. J. Nano Dimension 2, 105–110. doi: 10.7508/IJND.2011.02.002
Kalaiarasi, R., Jayallakshmi, N., Venkatachalam, P. (2010). Phytosynthesis of nanoparticles and its applications. Plant Cell Biotechnol. Mol. Biol. 11, 1–16.
Kaul, R. K., Kumar, P., Burman, U., Joshi, P., Agrawal, A., Raliya, R., et al. (2012). Magnesium and iron nanoparticles production using microorganisms and various salts. Material Sci. Poland 30, 254–258. doi: 10.2478/s13536-012-0028-x
Khorrami, S., Zarrabi, A., Khaleghi, M., Danaei, M., Mozafari, M. (2018). Selective cytotoxicity of green synthesized silver nanoparticles against the MCF-7 tumor cell line and their enhanced antioxidant and antimicrobial properties. Int. J. Nanomedicine 13, 8013–8024. doi: 10.2147/IJN.S189295
Kim, S. W., Jung, J. H., Lamsal, K., Kim, Y. S., Min JS and Lee, Y. S. (2012). Antifungal effects of silver nanoparticles (AgNPs) against various plant pathogenic fungi. Mycobiology. 40 (1), 53–58. doi: 10.5941/MYCO.2012.40.1.053
Kim, K. J., Sung, W. S., Suh, B. K., Moon, S.-K., Choi, J.-S., Kim JG and Lee, D. G. (2009). Antifungal activity and mode of action of silver nano-particles on Candida albicans. Biometals; 22 (2), 235–242. doi: 10.1007/s10534-008-9159-2
Kumar, B., Smita, K., Cumbal, L., Debut, A. (2014). Biogenic synthesis of iron oxide nanoparticles for 2-arylbenzimidazole fabrication. J. Saudi Chem. Soc. 18, 364–369. doi: 10.1016/j.jscs.2014.01.003
Lara, H. H., Ayala-Nuñez, N. V., Ixtepan-Turrent, L., Rodriguez-Padilla, C. (2010a). Mode of antiviral action of silver nanoparticles against HIV-1. J. Nanobiotechnology 8, 1–10. doi: 10.1186/1477-3155-8-1
Lara, H. H., Ixtepan-Turrent, L., Garza-Treviño, E. N., Rodriguez-Padilla, C. (2010b). PVP-coated silver nanoparticles block the transmission of cell-free and cell-associated HIV-1 in human cervical culture. J. Nanobiotechnology 8, 15–25. doi: 10.1186/1477-3155-8-15oson
Li, L., Li, L., Zhou, X., Yu, Y., Li, Z., Zuo, D., et al. (2019). Silver nanoparticles induce protective autophagy via Ca 2+ /CaMKKβ/AMPK/mTOR pathway in SH- SY5Y cells and rat brains. Nanotoxicology 13 (3), 369–391. doi: 10.1080/17435390.2018.1550226
Liao, C., Li, Y., Tjong, S. C. (2019). Bactericidal and cytotoxic properties of silver nanoparticles. Int. J. Mol. Sci. 20 (2), 449. doi: 10.3390/ijms20020449
Lin, D., Xing, B. (2008). Root uptake and phytotoxicity of ZnO nanoparticles. Environ. Sci. Technol. 42, 5580. doi: 10.1021/es800422x
Liu, J., Hwang, Y. S., Lenhart, J. J. (2015). Heteroaggregation of bare silver nanoparticles with clay minerals. Environmental Science. Nano 2, 528–540. doi: 10.1039/C5EN00130G
Lu, W., Shen, Y., Xie, A., Zhang, W. (2010). Green synthesis and characterization of superparamagnetic Fe3O4 nanoparticles. J. Magnetism Magnetic Materials 322, 1828–1833. doi: 10.1016/j.jmmm.2009.12.035
Lu, L., Sun, R. W., Chen, R., Hui, C. K., Ho, C. M., Luk, J. M., et al. (2008). Silver nanoparticles inhibit hepatitis B virus replication. Antiviral Ther. 13, 253–262. doi: 10.1177/135965350801300210
Mahdavi, M., Namvar, F., Ahmad, M. B., Mohamad, R. (2013). Green biosynthesis and characterization of magnetic iron oxide (Fe3O4) nanoparticles using seaweed (Sargassum muticum) aqueous extract. Molecules 18, 5954–5964. doi: 10.3390/molecules18055954
Mallmann, E. J. J., Cunha, F. A., Castra, B. N. M. F., Maciel, A. M., Menezes, E. A., Fechine, P. B. A. (2015). Antifungal activity of silver nanoparticles obtained by green synthesis. Rev. do Instituto Medicina Trop. São Paulo 57 (2), 165–167. doi: 10.1590/S0036-46652015000200011
Mandal, D., Bolander, M. E., Mukhopadhyay, D., Sarkar, G., Mukherjee, P. (2006). The use of microorganisms for the formation of metal nanoparticles and their application. Appl. Microbiol. Biotechnol. 69, 485–492. doi: 10.1007/s00253-005-0179-3
Manjula, S. B. M., Bhoomika, S., Kavita Nagshetty, N. G. (2022). “Environmental Adequacy of Green Polymers and Biomaterials,” in Polymeric Biomaterials (United States: CRC Press).
Manjula, N. G., Sarma, G., Shilpa, B. M., Suresh Kumar, K. (2022). “Environmental Applications of Green Engineered Copper Nanoparticles,” in hytonanotechnology. Eds. Shah, M. P., Roy, A. (Singapore: Springer Nature Singapore), 255–276.
Manjula, N. G., Tajunnisa, Mamani, V., MeGhana, C. A., Mayegowda, S. B. (2023). “Fungal-Based Synthesis to Generate Nanoparticles for Nanobioremediation,” in Green Nanoremediation: Sustainable Management of Environmental Pollution. Eds. Policarpo Tonelli, F. M., Roy, A., Ananda Murthy, H. C. (Cham: Springer International Publishing), 83–108. doi: 10.1007/978-3-031-30558-0_4
Mayegowda, S. B., Ng, M., Alghamdi, S., Atwah, B., Alhindi, Z., Islam, F. (2022a). Role of antimicrobial drug in the development of potential therapeutics. Evidence-Based Complementary Altern. Med. 2022, 1–17. doi: 10.1155/2022/2500613
Mayegowda, S. B., Sarma, G., Gadilingappa, M. N., Alghamdi, S., Aslam, A., Refaat, B., et al. (2023). Green-synthesized nanoparticles and their therapeutic applications: A review. Green Process. Synthesis 12, 20230001. doi: 10.1515/gps-2023-0001
Mayegowda, K. M. K., Shristi Ram, N. G., Shilpa Borehalli, M. (2022). “Biopolymers and Their Applications in Biomedicine,” in Polymeric Biomaterials (United States: CRC Press).
Mayegowda, S. B., Sureshkumar, K., Yashaswini, R., Ramakrishnappa, T. (2022b). “Phytonanotechnology for the Removal of Pollutants from the Contaminated Soil Environment,” in Phytonanotechnology. Eds. Shah, M. P., Roy, A. (Singapore: Springer Nature Singapore), 319–336.
Meikle, T., Dyett, B. P., Strachan, J. B., White, J., Drummond, C. J., Conn, C. E. V. (2020). Preparation, characterization, and antimicrobial activity of cubosome encapsulated metal nanocrystals. ACS Appl. Materials Interfaces 12 (6), 6944–6954. doi: 10.1021/acsami.9b21783
Mohamed, Y. M., Azzam, A. M., Amin, B. H., Safwat, N. A. (2015). Mycosynthesis of iron nanoparticles by Alternaria alternata and its antibacterial activity. Afr. J. Biotechnol. 14, 1234–1241. doi: 10.5897/AJB2014.14286
Nadagouda, M. N., Varma, R. S. (2007). A greener synthesis of core (Fe, Cu)-shell (Au, Pt, Pd, and Ag) nanocrystals using aqueous Vitamin C. Crystal Growth Design 7, 2582–2587. doi: 10.1021/cg070554e
Noronha, V. T., Paula, A. J., Durán, G., Galembeck, A., Cogo-Muller, K., Franz-Montan, M., et al. (2017). Silver nanoparticles in dentistry. Dental Materials 33 (10), 1110–1126. doi: 10.1016/j.dental.2017.07.002
Osonga, F. J., Kalra, S., Miller, R. M., Isika, D., Sadik, O. A. (2020). Synthesis, characterization and antifungal activities of eco-friendly palladium nanoparticles. RSC Adv. 10, 5894–5904. doi: 10.1039/C9RA07800B
Papp, I., Sieben, C., Ludwig, K., Roskamp, M., Böttcher, C., Schlecht, S., et al. (2010). Inhibition of influenza virus infection by multivalent sialic-acid-functionalized gold nanoparticles. Small 6, 2900–2906. doi: 10.1002/smll.201001349
Park, E.-J., Yi, J., Chung, K.-H., Rye, D.-Y., Choi, J., Park, K. (2008). Oxidative stress and apoptosis induced by titanium dioxide nanoparticles in cultured BEAS-2B cells. Toxicol. Lett. 180, 222–229. doi: 10.1016/j.toxlet.2008.06.869
Pernodet, N., Fang, X., Sun, Y., Bakhtina, A., Ramakrishnan, A., Sokolov, J., et al. (2006). Adverse effects of citrate/gold nanoparticles on human dermal fibroblasts. Small 6, 766–773. doi: 10.1002/smll.200500492
Pradhan, N., Singh, S., Ojha, N., Srivastava, A., Barla, A., Rai, V., et al. (2015). Facets of nanotechnology as seen in food processing, packaging, and preservation industry. BioMed. Res. Int., 365672. doi: 10.1155/2015/365672
Pugazhendhi, A., Prabakar, D., Jacob, J. M., Karuppusamy, I., Saratale, R. G. (2018). Synthesis and characterization of silver nanoparticles using Gelidium amansii and its antimicrobial property against various pathogenic bacteria. Microbial Pathogenesis 114, 41–45. doi: 10.1016/j.micpath.2017.11.013
Raj, S., Jose, S., Sumod, U. S., Sabitha, M. (2012). Nanotechnology in cosmetics: Opportunities and challenges. J. Pharm. BioAllied Sci. 4 (3), 186–193. doi: 10.4103/0975-7406.99016
Ramakrishnappa, S. B. M., Kempahanumakkagari Sureshkumar, M., Thippeswamy, S. (2022). “pH and Thermo-responsive Systems,” in Polymeric Biomaterials (United States: CRC Press).
Ramkumar, V. S., Pugazhendhi, A., Gopalakrishnan, K., Sivagurunathan, P., Saratale, G. D., Dung, T. N. B., et al. (2017). Biofabrication and characterization of silver nanoparticles using aqu- eous extract of seaweed Enteromorpha compressa and its biomedical properties. Biotechnol. Rep. 14, 1–7. doi: 10.1016/j.btre.2017.02.001
Rao, A., Bankar, A., Kumar, A. R., Gosavi, S., Zinjarde, S. (2013). Removal of hexavalent chromium ions by Yarrowia lipolytica cells modified with phyto-inspired Fe0/Fe3O4 nanoparticles. J. Contaminant Hydrology 146, 63–73. doi: 10.1016/j.jconhyd.2012.12.008
Ren, M., Wang, Y., Luo, Y., Yao, X., Yang, Z., Zhang, P., et al. (2021). Functionalized nanoparticles in prevention and targeted therapy of viral diseases with neurotropism properties, special insight on COVID-19. Front. Microbiol. 12. doi: 10.3389/fmicb.2021.767104
Rogers, J. V., Parkinson, C. V., Choi, Y. W., Speshock, J. L., Hussain, S. M. (2008). A preliminary assessment of silver nanoparticles inhibition of monkeypox virus plaque formation. Nanoscale Res. Lett. 3, 129–133. doi: 10.1007/s11671-008-9128-2
Rotti, R. B., Sunitha, D. V., Manjunath, R., Roy, A., Mayegowda, S. B., Gnanaprakash, A. P., et al. (2023). Green synthesis of MgO nanoparticles and its antibacterial properties. Front. Chem. 11. doi: 10.3389/fchem.2023.1143614
Roy, I., Ohulchanskyy, T. Y., Pudavar, H. E., Bergey, E. J., Oseroff, A. R., Morgan, J., et al. (2003). Ceramic-based nanoparticles entrapping water-insoluble photosensitizing anticancer drugs: a novel drug-carrier system for photodynamic therapy. J. Am. Chem. Soc. 125, 7860–7865. doi: 10.1021/ja0343095
Saif, S., Tahir, A., Chen, Y. (2016). Green synthesis of iron nanoparticles and their environmental applications and implications. Nanomaterials 6 (11), 209. doi: 10.3390/nano6110209
Savasari, M., Emadi, M., Bahmanyar, M. A., Biparva, P. (2015). Optimization of Cd(II) removal from aqueous solution by ascorbic acid-stabilized zero valent iron nanoparticles using response surface methodology. J. Ind. Engeneering Chem. 21, 1403–1409. doi: 10.1016/j.jiec.2014.06.014
Sayyad, A. S., Balakrishnan, K., Ci, L., Kabbani, A. T., Vajtai, R., Ajayan, P. M. (2012). Synthesis of iron nanoparticles from hemoglobin and myoglobin. Nanotechnology 23, 55602. doi: 10.1088/0957-4484/23/5/055602
Shanmuganathan, R., MubarakAli, D., Prabakar, D., Muthukumar, H., Thajuddin, N., Kumar, S. S., et al. (2018). An enhancement of antimicrobial efficacy of biogenic and ceftriaxone-conjugated silver nanoparticles: green approach. Environ. Sci. pollut. Res. Int. 25 (11), 10362–10370. doi: 10.1007/s11356-017-9367-9
Singh, T., Shukla, S., Kumar, P., Wahla, V., Bajpai, V. K., Rather, I. A. (2017). Application of nanotechnology in food science: Perception and overview. Front. Microbiology; 8. doi: 10.3389/fmicb.2017.01501
Siskova, K. M., Straska, J., Krizek, M., Tucek, J., Machala, L., Zboril, R. (2013). Formation of zero-valent iron nanoparticles mediated by amino acids. Proc. Environ. Sci. 18, 809–817. doi: 10.1016/j.proenv.2013.04.109
Sreeja, V., Jayaprabha, K. N., Joy, P. A. (2014). Water-dispersible ascorbic-acid-coated magnetite nanoparticles for contrast enhancement in MRI. Appl. Nanoscience 5, 435–441. doi: 10.1007/s13204-014-0335-0
Subramaniyam, V., Subashchandrabose, S. R., Thavamani, P., Megharaj, M., Chen, Z., Naidu, R. (2015). Chlorococcum sp. MM11—A novel phyco-nanofactory for the synthesis of iron nanoparticles. J. Appl. Phycology 27, 1861–1869. doi: 10.1007/s10811-014-0492-2
Sundaram, P. A., Augustine, R., Kannan, M. (2012). Extracellular biosynthesis of iron oxide nanoparticles by Bacillus subtilis strains isolated from rhizosphere soil. Biotechnol. Bioprocess Eng. 17, 835–840. doi: 10.1007/s12257-011-0582-9
Venkateswarlu, S., Natesh Kumar, B., Prasad, C. H., Venkateswarlu, P., Jyothi, N. V. V. (2014). Bio-inspired green synthesis of Fe3O4 spherical magnetic nanoparticles using Syzygium cumini seed extract. Physica B Condensed Matter 449, 67–71. doi: 10.1016/j.physb.2014.04.031
Vines, J. B., Yoon, J.-H., Ryu, N.-E., Lim, D.-J., Park, H. (2019). Gold nanoparticles for photothermal cancer therapy. Front. Chem. 7 (April). doi: 10.3389/fchem.2019.00167
Vo-Dinh, T. (2007). Nanotechnology in Biology and Medicine: Methods, Devices, and Applications (Boca Raton, FL: CRC Press).
Wiley, B. Y., Mayers, B., Xia, Y. (2005). shape-Controlled. The case of silver. Chem. Eur.J 11, 454–463. doi: 10.1002/chem.200400927
Yadav, L. S. R., Shilpa, B. M., Suma, B. P., Venkatesh, R., Nagaraju, G. (2021). Synergistic effect of photocatalytic, antibacterial and electrochemical activities on biosynthesized zirconium oxide nanoparticles. Eur. Phys. J. Plus 136 (7), 764. doi: 10.1140/epjp/s13360-021-01606-6
Yamanaka, M., Hara, K., Kudo, J. (2005). Bactericidal actions of a silver ion solution on Escherichia coli, studied by energy- filtering transmission electron microscopy and proteomic analysis. Appl. Environ. Microbiology; 71, 7589–7593. doi: 10.1128/AEM.71.11.7589-7593.2005
Yan, Q., Street, J., Yu, F. (2015). Synthesis of carbon-encapsulated iron nanoparticles from wood derived sugars by hydrothermal carbonization (HTC) and their application to convert bio-syngas into liquid hydrocarbons. Biomass Bioenergy 83, 85–95. doi: 10.1016/j.biombioe.2015.09.002
Keywords: green synthesis, antimicrobial agents, anticancer agents, antioxidant activity, drug delivery, DNA damage, eco-friendly
Citation: Borehalli Mayegowda S, Roy A, N. G. M, Pandit S, Alghamdi S, Almehmadi M, Allahyani M, Awwad NS and Sharma R (2023) Eco-friendly synthesized nanoparticles as antimicrobial agents: an updated review. Front. Cell. Infect. Microbiol. 13:1224778. doi: 10.3389/fcimb.2023.1224778
Received: 18 May 2023; Accepted: 18 July 2023;
Published: 16 August 2023.
Edited by:
Parth Sarthi Sen Gupta, D Y Patil International University, IndiaReviewed by:
Vicky Mody, Philadelphia College of Osteopathic Medicine (PCOM), United StatesCopyright © 2023 Borehalli Mayegowda, Roy, N. G., Pandit, Alghamdi, Almehmadi, Allahyani, Awwad and Sharma. This is an open-access article distributed under the terms of the Creative Commons Attribution License (CC BY). The use, distribution or reproduction in other forums is permitted, provided the original author(s) and the copyright owner(s) are credited and that the original publication in this journal is cited, in accordance with accepted academic practice. No use, distribution or reproduction is permitted which does not comply with these terms.
*Correspondence: Shilpa Borehalli Mayegowda, c2hpbHBhLmJvcmVoYWxsaUBjaHJpc3R1bml2ZXJzaXR5Lmlu; Arpita Roy, YXJidDIwMTRAZ21haWwuY29t
Disclaimer: All claims expressed in this article are solely those of the authors and do not necessarily represent those of their affiliated organizations, or those of the publisher, the editors and the reviewers. Any product that may be evaluated in this article or claim that may be made by its manufacturer is not guaranteed or endorsed by the publisher.
Research integrity at Frontiers
Learn more about the work of our research integrity team to safeguard the quality of each article we publish.