- 1Department of Plastic and Cosmetic Surgery, Xinqiao Hospital, The Second Affiliated Hospital, Army Medical University, The Third Military Medical University, Chongqing, China
- 2Cadet Brigade 4, College of Basic Medicine, Army Medical University, The Third Military Medical University, Chongqing, China
- 3Department of Microbiology, Army Medical University, The Third Military Medical University, Chongqing, China
- 4Institute of Burn Research, State Key Laboratory of Trauma, Burns and Combined Injury, Southwest Hospital, The First Affiliated Hospital, Army Medical University, The Third Military Medical University, Chongqing, China
Introduction: Pseudomonas aeruginosa (P.aeruginosa) is an important opportunistic pathogen with broad environmental adaptability and complex drug resistance. Single-molecule real-time (SMRT) sequencing technique has longer read-length sequences, more accuracy, and the ability to identify epigenetic DNA alterations.
Methods: This study applied SMRT technology to sequence a clinical strain P. aeruginosa PA3 to obtain its genome sequence and methylation modification information. Genomic, comparative, pan-genomic, and epigenetic analyses of PA3 were conducted.
Results: General genome annotations of PA3 were discovered, as well as information about virulence factors, regulatory proteins (RPs), secreted proteins, type II toxin-antitoxin (TA) pairs, and genomic islands. A genome-wide comparison revealed that PA3 was comparable to other P. aeruginosa strains in terms of identity, but varied in areas of horizontal gene transfer (HGT). Phylogenetic analysis showed that PA3 was closely related to P. aeruginosa 60503 and P. aeruginosa 8380. P. aeruginosa's pan-genome consists of a core genome of roughly 4,300 genes and an accessory genome of at least 5,500 genes. The results of the epigenetic analysis identified one main methylation sites, N6-methyladenosine (m6A) and 1 motif (CATNNNNNNNTCCT/AGGANNNNNNNATG). 16 meaningful methylated sites were picked. Among these, purH, phaZ, and lexA are of great significance playing an important role in the drug resistance and biological environment adaptability of PA3, and the targeting of these genes may benefit further antibacterial studies.
Disucssion: This study provided a detailed visualization and DNA methylation information of the PA3 genome and set a foundation for subsequent research into the molecular mechanism of DNA methyltransferase-controlled P. aeruginosa pathogenicity.
1 Introduction
Pseudomonas aeruginosa, a gram-negative bacterium is one of the top three causes of opportunistic human infections which inherent resistance to antibiotics and disinfectants (Stover et al., 2000). P. aeruginosa takes responsibility for many nosocomial infections, including pneumonia, surgical site infection, urinary tract infection, and bacteremia (Reynolds and Kollef, 2021). In addition, P. aeruginosa is the most prevalent multidrug-resistant infection in hospitalized patients, especially in ICU or burn wards (Parkins et al., 2018). An improved understanding of the pathogenicity and resistance mechanisms of P. aeruginosa has led to the development of novel antimicrobial approaches (Dimopoulos et al., 2020). However, there is still an urgent need in investigating its genomics under the circumstances of the rapid mutation and evolution of P. aeruginosa.
Whole-genome sequencing is commonly used to analyze and learn the genomic background of an organism. Third-generation sequencing, often characterized by single-molecule sequencing, with ultra-long read lengths and higher accuracy (Ardui et al., 2018), fundamentally differs from clone-based second-generation sequencing approaches (Gu et al., 2019). The current third-generation sequencing technology, single-molecule real-time (SMRT) sequencing from Pacific Biosciences (PacBio) directly detects DNA methylation without bisulfite conversion (Eid et al., 2009). This allows single-molecule identification of epigenetic modifications at base resolution. And the long read length of SMRT sequencing may allow motif analysis in highly repetitive genomic regions (Flusberg et al., 2010). Thus SMRT sequencing has significant implications for enhancing the understanding of epigenetic and phenotypic diversity (Li et al., 2016; Attar, 2016) through whole-genome sequencing, targeted sequencing, complex population analysis, RNA sequencing, and epigenetic characterization (Nakano et al., 2017).
Restriction-modification system (RM system) in P. aeruginosa mainly consists of restriction enzymes and methyltransferases, that protect individuals from foreign DNA (such as bacteriophages) intrusion (Pleška et al., 2016). Generally, the RM system consists of a methyltransferase (MTases) with a target recognition domain (TRD) that can function independently and another restriction endonuclease that can only bind to DNA. The epigenetic control of P. aeruginosa is mainly achieved through the DNA MTases (Anton and Roberts, 2021) by transferring methyl groups from donor S-adenosyl-l-methionine (SAM) to specific positions on target bases to form distinct modifications (Gold et al., 1963). This specific location is called the motif, where MTase is capable of modifying a base (typically between 4 and 8 bp long). Recently, bacterial DNA methylation mediated by MTases has been found to play multiple important roles in regulating gene expression, virulence, pathogen-host interactions, and antimicrobial resistance (Cohen et al., 2016). Some highly conserved DNA MTases may serve as promising targets for developing novel antibiotics through epigenetic regulations (Oliveira and G Fang, 2021).
The genome of different P. aeruginosa strains vary during evolution when methylation brings even greater epigenetic adaptations. The genome-wide DNA methylation pattern of a classic laboratory strain PAO1 revealed that type I RM system methyltransferases and their corresponding motifs altered virulence and oxidative stress response (Doberenz et al., 2017). The methylation recognition motif and methyltransferases of a clinical antiphagocytosis P. aeruginosa strain TBCF10839 confirmed that the methyltransferases deletion mutant had an important impact on improving bacterial virulence and Nitric Oxide Homeostasis (Han et al., 2022). Moreover, a single and sole methylation mutation on the toxR gene caused drastically different resistance against Ceftolozane/Tazobactam, Ceftazidime/Avibactam, and Piperacillin/Tazobactam in the isogenic pair of P. aeruginosa PB367 and PB350 l strains (W et al., 2020). These genetic and/or epigenetic elements are responsible for their notorious drug resistance.
In this study, SMRT sequencing was applied to gain a whole genome picture of P. aeruginosa clinical strain PA3 and insights into its DNA methylation patterns. By obtaining the methylation sites and sequences of the entire genome motif, bioinformatics analysis revealed 16 meaningful genes with methylation sites in their coding regions. Though the methyltransferases of these motifs need to be further identified, our findings provide a fundamental basis for further studies against P. aeruginosa infections.
2 Materials and methods
2.1 Bacterial storage and growth
The P. aeruginosa PA3 strain was maintained in the laboratory of the Department of Microbiology, Army Medical University. P. aeruginosa strains were stored in our laboratory at −80°C in glycerol. P. aeruginosa was cultured aerobically in Luria-Bertani (LB) medium (5 g yeast extract, 10 g tryptone, 10 g NaCl perliter) at 37°C for all experiments (Lu et al., 2015).
2.2 DNA extraction and SMRT sequencing
The PA3 genomic DNA was extracted and purified from the stationary phase cultures grown in LB broth using a DNeasy PowerClean Pro Cleanuo Kit (Shanghai Biotechnology Corporation). Approximately 10 μg purified PA3 genomic DNA was subjected to SMRT sequencing at Shanghai Biotechnology Corporation (SHBIO, Shanghai, China) using PacBio RSII (Pacific Biosciences). PA3 genomic DNA was fragmented using g-TUBE (Covaris, U.S.), and the 8-12k fragment was purified using AMPure magnetic beads (Beckman Coulter, U.S.). SMRTbell template library containing 10kb DNA fragments was prepared using DNA Template Prep Kit 2.0 (Pacific Biosciences, U.S.). Qubit® 2.0 Fluorometer was used to measure the concentration of the SMRTbell template library, and Agilent2100 was used to measure the size of the library. SPAdes-3.5.0 software was used to accomplish de novo assembly.
2.3 Sequence analysis and genome annotation
The PA3 genome was annotated using the NCBI Prokaryotic Genome Automatic Annotation Pipeline (PGAAP) (http://www.ncbi.nlm.nih.gov/genome/annotation_prok/), which included genes, proteins, rRNAs, and tRNAs (Angiuoli et al., 2008). The genome data has been uploaded to the NCBI Genebank (CP061034.1). The Restriction-Modification (RM) system was predicted in REBASE (http://rebase.neb.com/rebase/rebase.html) (Roberts et al., 2022). Horizontal Gene Transfer (HGT) was predicted by alien_hunter 1.7 (GS and J, 2006). VirSorter 2.2.3 was used to predict dsDNA and ssDNA virus genomes (phage) (Guo et al., 2021). Mobile genetic elements (MGEs) were detected by mobileOG-db (beatrix-1.6) (Brown et al., 2022). SignalP6.0(SignalP - 6.0 - Services - DTU Health Tech) was used to annotate whether the protein sequence contains a signal peptide structure (Teufel et al., 2022). TMHMM2.0 (TMHMM - 2.0 - Services - DTU Health Tech) was used to annotate whether the protein sequence contains a transmembrane structure (Sonnhammer et al., 1998). DNA base modification detection took the splicing result as the reference genome. Through the in silico control analysis method in the RS_Modification_and_Motif_Analysis.1 process, the positive and negative strand base modification information and possible motifs of each position on the genome were obtained (Yang and Scott, 2017).
2.4 Visualized analysis of the PA3 genome
The PA3 genome was shown using the Ring Image Generator (BRIG) and Basic Local Alignment Search Tool (BLAST) (http://brig.sourceforge.net/) (NF et al., 2011). The Predicted Prokaryotic Regulatory Protein Server predicted regulatory proteins (RPs) (http://www.p2rp.org/) (Barakat et al., 2013) and visualized using Proksee (Proksee - New). The TAfinder program was used to forecast type II toxin-antitoxin (T-A) (http://202.120.12.133/TAfinder/index.php) and visualized using BRIG. IslandViewer (http://www.pathogenomics.sfu.ca/islandviewer/) was used to examine genomic islands (Bertelli et al., 2017) and visualized using Proksee.
2.5 Comparative genomic analysis
The 20 complete P. aeruginosa genome sequences were compared through BlastN by using blast 2.2.29+ (ftp://ftp.ncbi.nlm.nih.gov/blast/) (Boratyn et al., 2013) and visualized by BRIG with 80% identity cut-off. The PA3 genome was used as a reference. Pseudomonas Genome Database (http://www.pseudomonas.com/) was used to get 16S rRNA sequences and 20 full P. aeruginosa genome sequences (Winsor et al., 2016). 16S rRNA sequences were subjected to multiple sequence alignments by using ClustalW (Hung et al., 2015) with default parameters, and phylogenetic trees were constructed and displayed by MEGA 11 (http://www.megasoftware.net/) (Tamura et al., 2021) with the neighbor-joining method (Som and Fuellen, 2009). EDGAR and BPGA1.3 were used for pan-genome analysis (Chaudhari et al., 2016) with default parameters. The findings of EDGAR and BPGA were merged to present the P. aeruginosa pan-genome. The EDGAR software platform (https://edgar3.computational.bio.uni-giessen.de/cgi-bin/edgar_login.cgi?cookie_test=1) was used to create the Venn diagram (Aras et al., 2015). Since we focus more on epigenetic analysis, the description and discussion of comparative genome analysis were mainly placed in the Result section.
2.6 Methylation analysis in SMRT sequencing data
DNA methylation and motif prediction were performed using the RS_Modification_and_Motif_Analysis.1 analysis from PacBio portal 2.3. Kinetic data generated during genome sequencing was utilized for methylation detection (Flusberg et al., 2010). The default quality value (QV) of 30 is routinely used as the threshold for initial analysis. Subsequently, we increased the QV threshold to 100 to exclude incorrect motifs that occurred when the QV value was set to the default (QV>30) for highly covered regions. The QV and motif base count change is presented in Supplementary Figure 2. The screening statistics for DNA methylation sites data were implemented using R version 4.3.0.
3 Results and analysis
3.1 Visualized PA3 general genomic features
One contig with a 432-fold sequencing coverage was found after the de novo assembly of the PA3 genome. 6,550,149 bp with 66.35% G+C, make up the entire PA3 genome (Table 1). A total of 6113 genes were annotated, including 5869 protein-coding genes, and 79 RNA genes. In addition, we also predicted 16 Pseudo Genes and 1 CRISPR (Clustered Regularly Interspaced Short Palindromic Repeats) array. VirSorter 2.2.3 predicted 5 putative dsDNA phages. Alien_hunter 1.7 predicts 60 horizontal transfer (HGT) regions. REBASE found 5 type II DNA methyltransferases predicted in the genome. In addition, mobileOG-db revealed 276 putative mobile gene elements (MGES) in the genome, including 53 IEs (integration/excision), 57 RRRs (replication/recombination/repair), 98 phages, 10 STDs (stability/transfer/defense), 58 transfers. These elements from different databases overlapped in the PA3 genome and are displayed in Figure 1 for a global view.
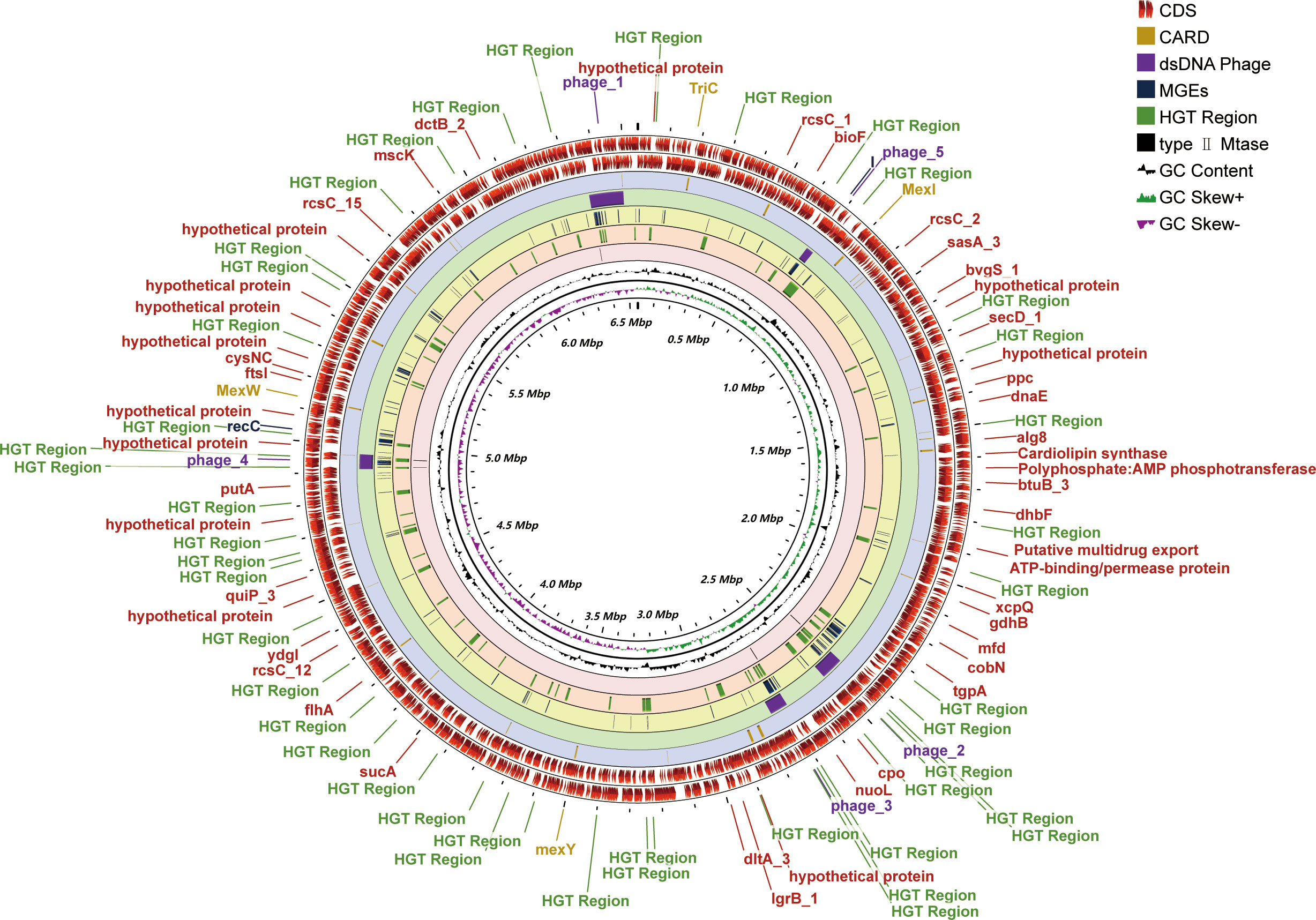
Figure 1 Genome-wide circular map of P. aeruginosa PA3. PA3 Genome Visualization Circle Diagram. In the outermost region, protein-coding regions (CDS) are indicated in red. From outside to inside, the outermost loop shows the CDS on the negative strand, followed by the CDS on the positive strand, the Comprehensive Antibiotic Resistance Database (CARD) resistance gene cite ds DNA phage sequence, MGEs, HGT region, putative MTase, GC content (black), and GC bias (purple/green).
3.1.1 Virulence factors
11 types of virulence factors of PA3 are predicted, including adherence, antimicrobial activity, anti-phagocytosis, biosurfactant, enzyme, iron uptake, protease, quorum sensing, regulation, secretion system, and toxin (Details in Table 2 and Supplementary Table 1). Compared with the standard P. aeruginosa strain PAO1, PA3 has the same virulence factors except for fimT (encoding type IV pilus biosynthesis), five Phenazines biosynthesis genes (phzC2, phzD2, phzE2, phzF2, phzG1), and pscE (encoding the P. aeruginosa TTSS (Type Three Secretion System) secretion system).
3.1.2 Regulatory proteins
Regulatory proteins (RPs) cause bacteria to adapt to changes in their environment. PA3 can be predicted to encode 614 regulatory proteins. Two-Component Systems (TCS) of PA3 contain 64 histidine kinases, 76 response regulators, and 5 phosphotransfer proteins, which are evenly distributed in the genome (Figures 2A, B). 181 transcriptional regulators, 175 single-component systems, 49 response regulators, and 23 sigma factors were among the transcription factors (TFs) of PA3 (Figures 2C, D). Along with these 41 additional DNA-binding proteins (ODPs, other DNA-binding proteins), PA3 also encodes 20 unclassified ODPs, Bhl, DnaA, Fis, and Hns (Figures 2E, F). Supplementary Tables 2-4 show detailed information about the RPs of PA3 for further research.
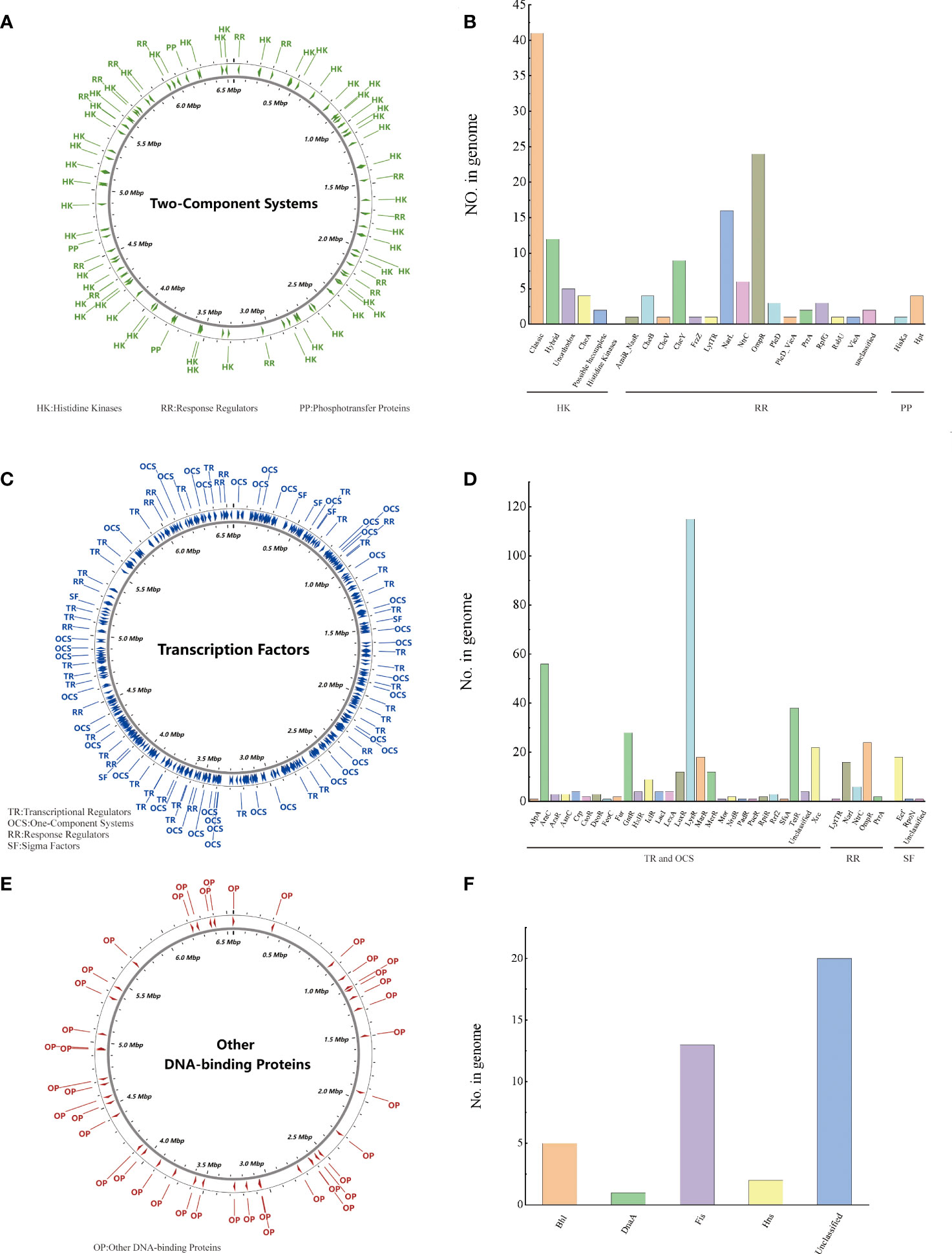
Figure 2 Regulatory proteins of P. aeruginosa PA3. (A) Distribution of TCS in the PA3 genome. (B) Detailed number and species of TCS proteins encoded by PA3. (C) Distribution of TFs in the PA3 genome. (D) Detailed number and species of TFs encoded by PA3. (E) Distribution of ODP in the PA3 genome. (F) Detailed quantities and types of ODPs encoded by PA3.
3.1.3 Secretory proteins
Secretory Protein refers to a protein that is secreted to function outside the cell after being synthesized in the cell. The secretory proteins of PA3 were detected when the protein contains signal peptide structures (annotated by SignalP) without transmembrane structures (annotated by TMHMM). A total of 895 protein sequences containing signal peptides were detected, 69 of which were predicted to have transmembrane helical regions. Thus, we found 826 secretory proteins in the PA3 genome (see Figure 3 and Supplementary Table 5 for details).
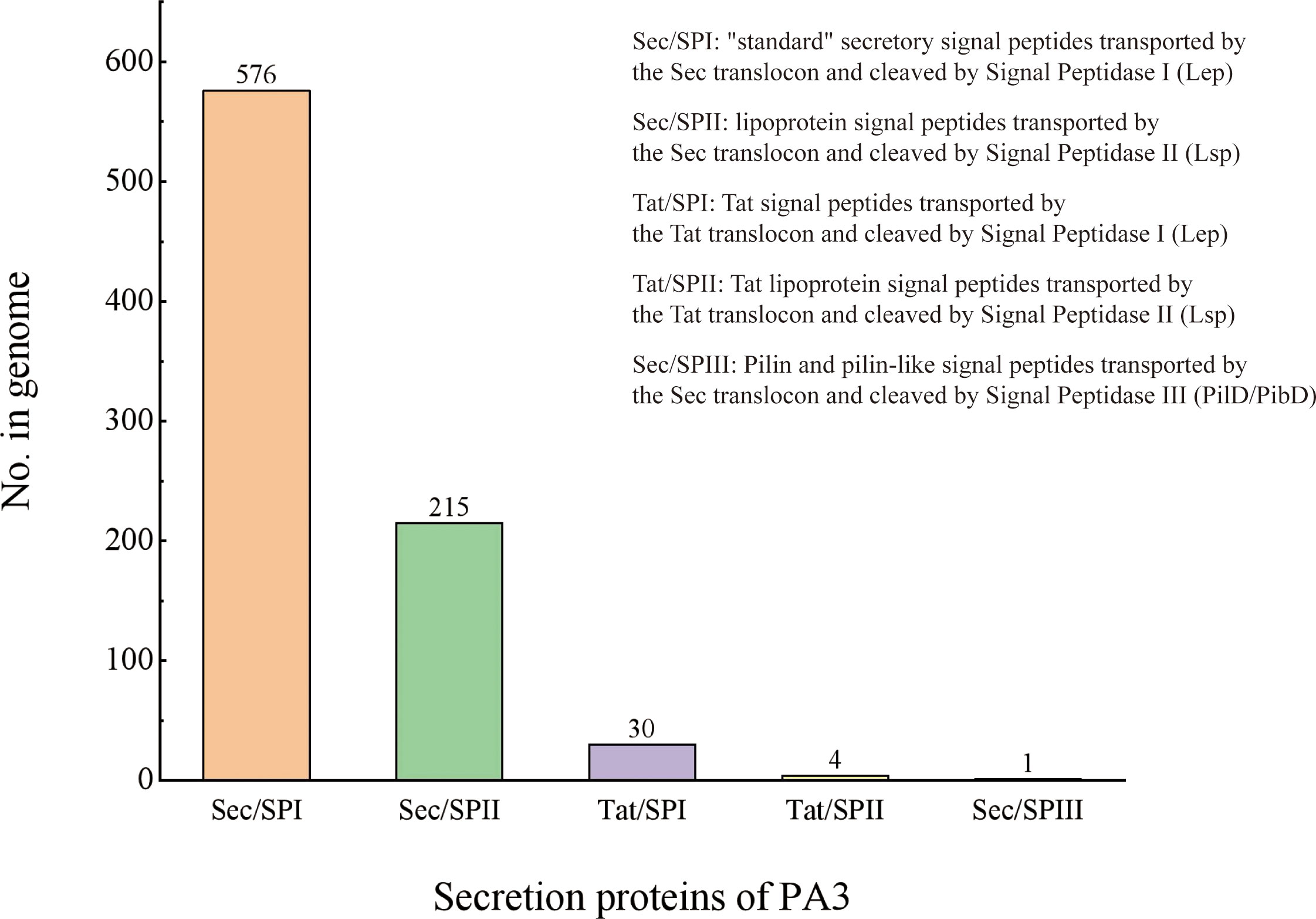
Figure 3 Secretory proteins of P. aeruginosa PA3. PA3 contains 576 Sec/SPI [“standard” secretory signal peptides transported by the Sec translocon and cleaved by Signal Peptidase I (Lep)], 215 Sec/SPII [lipoprotein signal peptides transported by the Sec translocon and cleaved by Signal Peptidase II (Lsp)], 30 Tat/SPI [Tat signal peptides transported by the Tat translocon and cleaved by Signal Peptidase I (Lep)], 4 Tat/SPII [Tat lipoprotein signal peptides transported by the Tat translocon and cleaved by Signal Peptidase II (Lsp)], 1 Sec/SPIII [Pilin and pilin-like signal peptides transported by the Sec translocon and cleaved by Signal Peptidase III (PilD/PibD)].
3.1.4 Type II toxin-antitoxin
The type II toxin-antitoxin (TA) system performs several critical cellular activities, including phage defense, biofilm development, persistence, and virulence (Kamruzzaman et al., 2021). We found 15 type II TA pairs in the PA3 genome (Figure 4A). 6 type II TA pairs were predicted by BLASTP method and 9 type II TA pairs were predicted by HMM method. When P. aeruginosa strains, PAO1, PA7, and PA14 have 13, 19, and 17 type II TA pairs, respectively (detailed information in Supplementary Table 6). Based on the comparison of the conserved domains of different P. aeruginosa strains, we found a unique antitoxin domain MerR in PA3, encoded by gene orf4389. The MerR family transcriptional regulators have been shown to mediate responses to environmental stress (Kamruzzaman et al., 2021) suggesting a different environmental tolerance of PA3 from other P. aeruginosa strains.
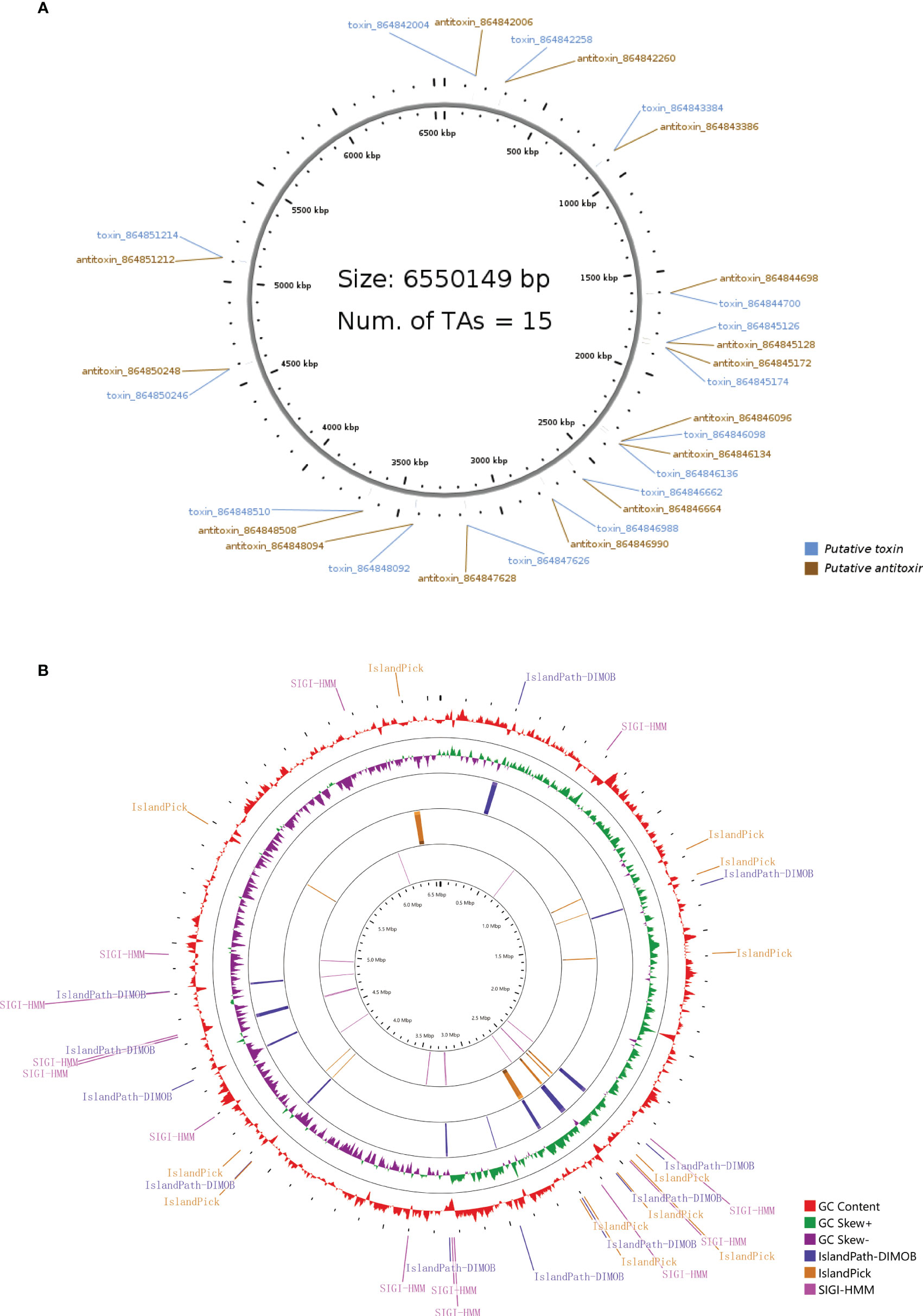
Figure 4 Type II T–A loci and genomic islands of P. aeruginosa PA3. (A) Distribution of 15 pairs of type II T–A loci in the PA3 genome. (B) Genomic islands in the PA3 genome. From outside to inside are GC content (red) and GC bias (purple/green) and three methods for predicting gene islands, IslandPath-DIMOB, IslandPick, SIGI-HMM (Single Island Genetic Island Hidden Markov Model).
3.1.5 Genomic islands
Genomic islands are groups of genes in bacterial genomes that have been acquired by horizontal gene transfer (Juhas et al., 2009). With SMRT technique, we found 36 genomic islands spanning 345 genes in PA3 (Figure 4B). Their main concentration distribution in the genome is the same as that of MGEs, HGT region, and phage gene in Figure 1. The largest genomic island was 39.7 kb in length (from 6,371,760 kb to 6,411,492 kb), named PA3_G36, and consisted of 51 genes. The PA3_G36 genomic island encodes a type II RM system methyltransferase, a Soluble lytic murein transglycosylase. See Supplementary Table 7 for specific information on the PA3 genomic island.
3.2 Comparative genomic analysis of P. aeruginosa
Visual genome comparisons are necessary to help identify genotypic differences among closely related bacteria. 20 strains of P. aeruginosa were compared by BRIG and visualized. These results showed that the P. aeruginosa genome sequences were very similar, with most of the compared genomic regions showing more than 85% identity compared with the PA1 genome (Figure 5). However, there are still several relatively large genomic regions (over 30 kb in length) unknown (less than 85% identity), as indicated by numbers 1 to 5 in Figure 4. These regions from long to short are 94.70, 52.16, 36.29, 37.86 and 39.80 kb in length, respectively.
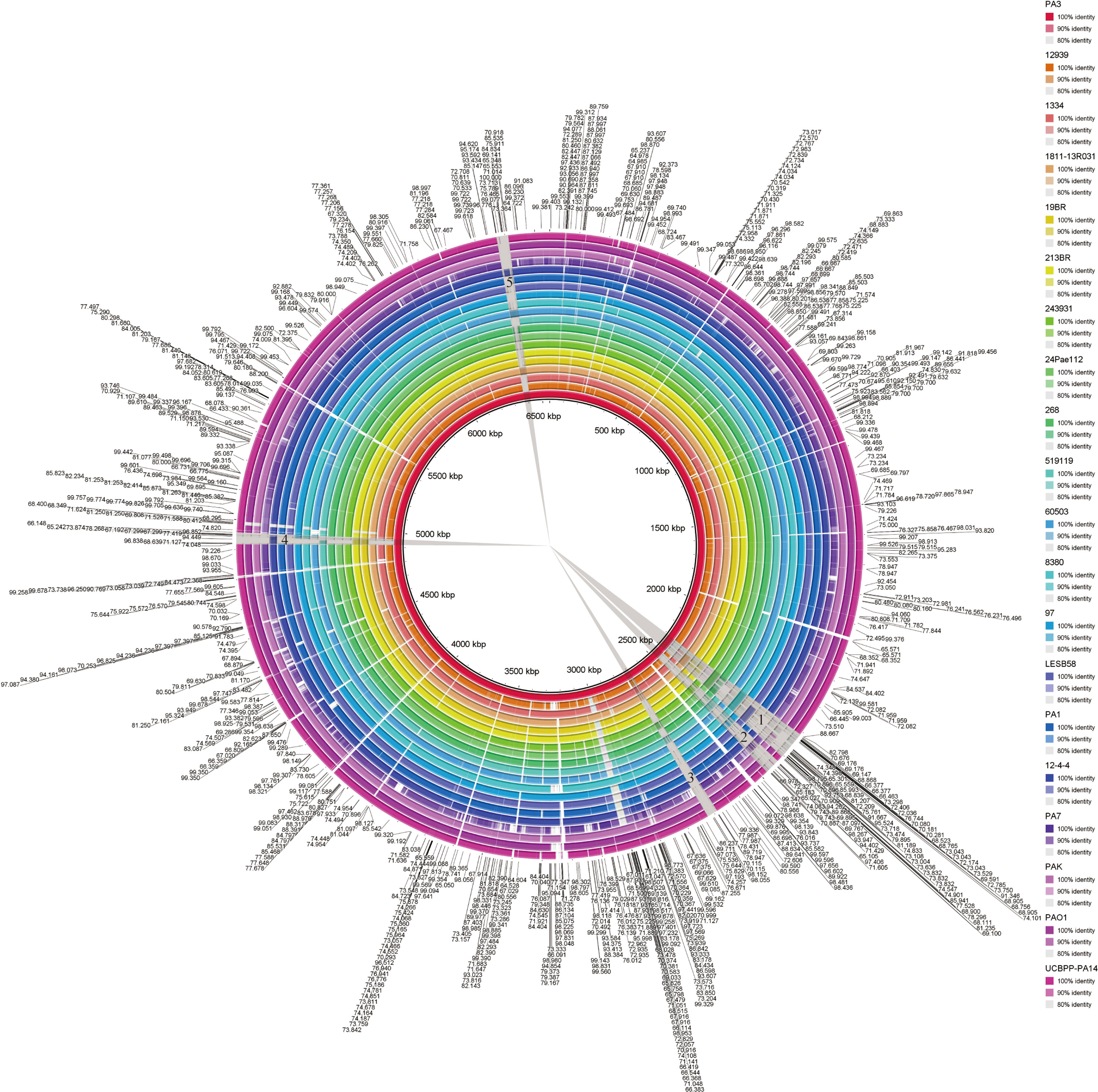
Figure 5 BLAST comparison of the complete genome of P. aeruginosa PA3 with 19 other P. aeruginosa species. Figure legends of the 20 P. aeruginosa strains are shown from innermost to outermost. Identity is indicated by color.
3.2.1 Phylogenetic analysis
Prokaryotic 16S ribosomal RNA (rRNA) sequences are widely used in environmental microbiology and molecular evolution as reliable markers for microbial taxonomy and phylogenetic analysis(Adhikari et al., 2015; Yang et al., 2016). This slight difference of 16S rRNA sequences indicated discriminable evolution processes of 126 P. aeruginosa species strains (including PA3). PA3 is closely related to P. aeruginosa 60503 and P. aeruginosa 8380 (Figure 6). The original phylogenetic tree with relative genetic distances is shown in Supplementary Figure 1.
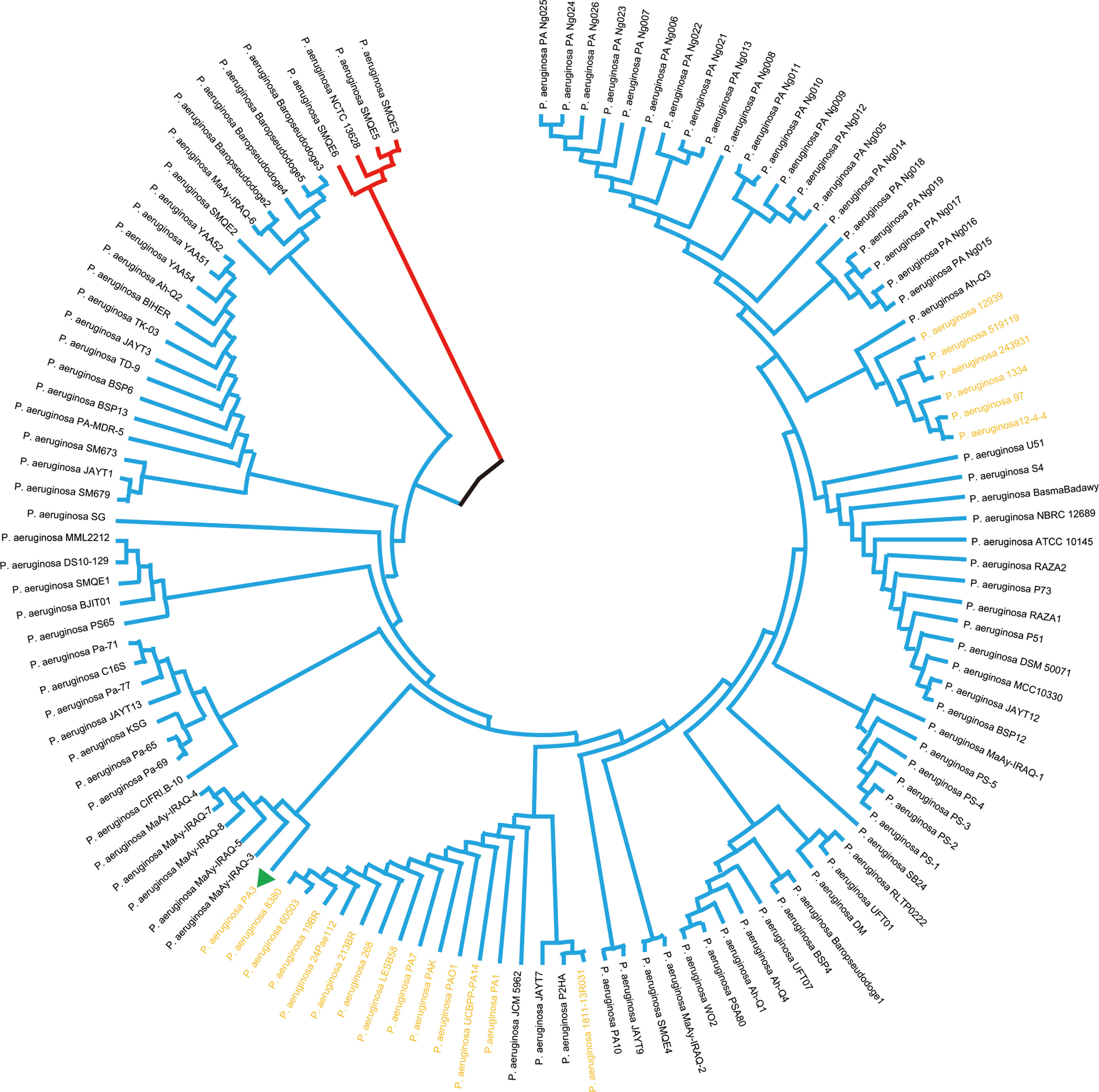
Figure 6 Phylogenetic tree of P. aeruginosa based on 16S rRNA. Phylogenetic relationships of 126 strains of P. aeruginosa (showing only topological structure). The two subgroups are shown in red and blue branches, respectively. The 20 comparative P. aeruginosa strains in Figure 4 are shown in bold yellow font.
3.2.2 Pan-genome analysis
The pan-genome can be divided into 3 parts, core genes, accessory genes, and unique genes. The core genome is the genes shared by all strains involved in basic biological processes such as gene expression, energy production, amino acid metabolism, etc. Accessory genes refer to genes present in specific strains, which are related to the diversity of the species and confer a competitive advantage on the individual. Unique genes exist only in a certain strain and are usually related to the unique phenotypic characteristics of the strain, such as adaptability to a specific environment, or unique pathogenicity (Yang et al., 2016). PA3 shares 4,865 core genes with four additional common P. aeruginosa strains, including PAO1, PA7, PAK, and UCBPP-PA14, according to the Venn diagram (Figure 7A). A total of 2,449 accessory genes were predicted, with 1,281 of these genes being unique. Figure 7B shows that the P. aeruginosa pan-genome has at least 4,300 core genes and 5,500 auxiliary genes. With the increase in genome number, the pan-genome size of P. aeruginosa also increased, suggesting that P. aeruginosa has a strong ability to integrate exogenous DNA and a high level of polymorphism.
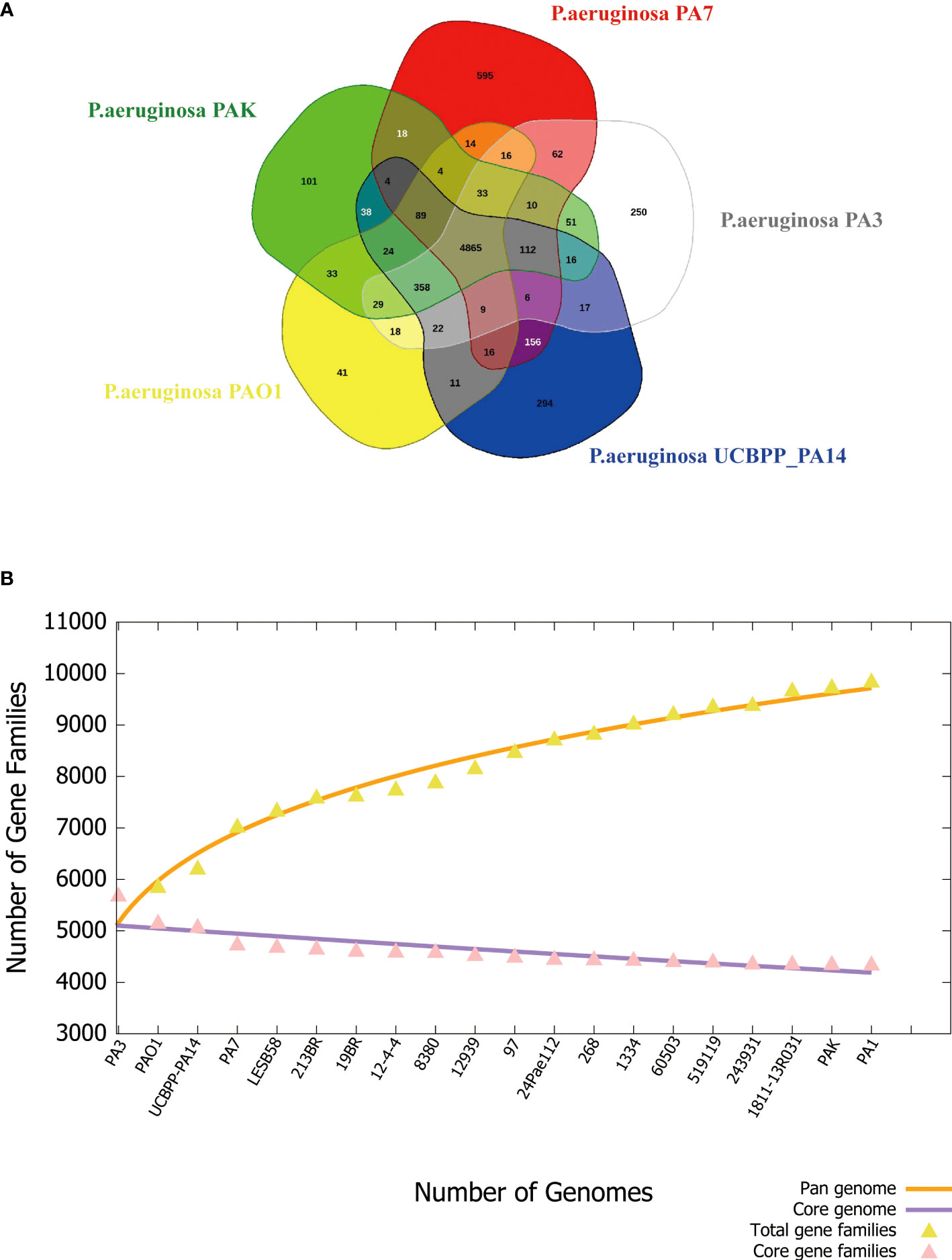
Figure 7 Phylogenetic tree of P. aeruginosa based on 16S rRNA. (A) Venn diagram of five P. aeruginosa strains showing the number of core genes, accessory genes, and their unique genes. (B) Pan- and core-genomes of 20 strains of P. aeruginosa with complete genomes.
3.3 Epigenetic analysis of P. aeruginosa PA3
We found 2032 m6A DNA methylation sites of P. aeruginosa PA3. Moreover, 1 motif (CATNNNNNNNTCCT/AGGANNNNNNNATG) was predicted and scored (Table 3). We screened out all CATNNNNNNNTCCT/AGGANNNNNNNATG sequences with m6A in the PA3 genome and found 16 corresponding genes with such m6A sites, which have high scores and coverage and we believe may have a potential role in gene regulation. (Table 4).
3.3.1 Restriction-modification system predicted
Based on REBASE analysis with PA3 methylation information uploaded, five MTase genes were predicted in the PA3 genome belonging to type II RM system named M.PaesPA3ORF11530P, M.PaesPA3ORF12905P, M.PaesPA3ORF23015P, M.PaesPA3ORF23200P, and M.PaesPA3ORF29655P (Table 5). The methylation motifs recognized by these five MTases were not predicted in REBASE. The predicted methylation motifs by SMRT were uploaded to the REBASE. Only C m6ATNNNNNNNTCCT/AGG m6ANNNNNNNATG could be detected. However, this motif was only known to belong to type I RM systems and could not be identified by any known enzymes.
3.3.2 Screened m6A sites and genes of PA3
The distributions of 16 genes with C m6ATNNNNNNNTCCT/AGG m6ANNNNNNNATG methylation sites were shown in Figure 8. These genes contain multiple functions and the most noteworthy genes are purH, phaZ, and lexA. Gene purH is a bifunctional phosphoribosyl aminoimidazole carboxamide formyltransferase/IMP cyclohydrolase, and plays a key role in maintaining folate in cells. The last two steps of de novo purine biosynthesis are catalyzed by purH. Gene phaZ is a depolymerase of polyhydroxyalkanoic acid (PHA), a storage substance synthesized by many prokaryotes. The transcriptional repressor lexA plays an important role in bacterial DNA damage repair.

Figure 8 The overview of 16 m6A methylation sites and their genes in P. aeruginosa PA3. The three tracks include 10 m6A sites on the positive strand, 6 sites on the negative strand, and their corresponding 16 genes.
4 Discussion
The benefits of SMRT sequencing are high throughput, quick turnaround time, high consistency accuracy, and a minimal quantity of DNA samples (Schadt et al., 2010). Moreover, it can accurately detect the modification of a single base, which is very suitable for bacteria sequencing and methylation analysis (Zautner et al., 2015). The study of the bacterial methylome has been revolutionized by introducing technologies capable of detecting m4C, 5-methylcytosine (m5C), and m6A at a genome-wide scale and single-nucleotide resolution. Thus resequencing projects of P. aeruginosa PA3 is necessary and advantageous to provide a better understanding of the number and diversity of genetics and epigenetics instead of the outdated sequencing techniques and mis-curated genome.
The majority of the studied P. aeruginosa genome regions are compatible with the PA3 genome. Portions of the PA3 genome that differ from those of other P. aeruginosa species are regions of horizontal gene transfer (also known as MGES) as also reported in our previous research on P. aeruginosa PA1 (Li et al., 2016). In this study, 336 putative mobile gene elements (MGES) carrying resistance genes or virulence factors were revealed in the P. aeruginosa PA3 genome. Infections caused by P. aeruginosa are attributed to virulence factors, such as flagella, alginate regulation factor, and phospholipase D. However, PA3 has the same virulence factors except for fimT (type IV pilus biosynthesis), 5 Phenazines related genes (phzC2, phzD2, phzE2, phzF2, phzG1), and pscE (TTSS secretion system). The difference in the virulence factors between P. aeruginosa PA3 and other P. aeruginosa strains suggests that the expression of virulence factors is dynamic and evolving (VV et al., 2022), which may explain the distinctive virulence characteristics between them. For instance, it has been revealed that type IV pili in the fimT mutant are not fully functional, and fimT mutation definitively affected bacterial function, including surface motility, gene expression, and virulence (Taguchi and Ichinose, 2011). The major component of the P. aeruginosa T3S needle is the PscF protein. The pscE knockout strain of P. aeruginosa lacks PscF protein and is therefore non-cytotoxic (Quinaud et al., 2005). Since a variety of MGEs or HGT regions have been isolated from clinical drug-resistant strains PA3, more in-depth research can be carried out on them.
From the epigenetic view, epigenomic changes were associated with bacterial phenotypes including antimicrobial resistance (Huang et al., 2020). Here we found 1 methylation motif in PA3. Whether there is a recognized relationship between the motif and 5 MTase has not been experimentally verified. The Mbase predicted by REBASE does not match our predicted motif, suggesting that the gene responsible for encoding this part of Mbase may be carried by a temperate bacteriophage. The identification of PA3 methyltransferases and the motifs they recognize play an important role in regulating gene methylation, which also provides new antibacterial insights. The DNA methylation pattern of PA3 was illustrated including one major methylation modification type m6A. Moreover, 16 methylated sites with high scores and coverage, which we believe may have a potential role in gene regulation were picked. Among these, purH, phaZ, and lexA are of great significance playing an important role in the drug resistance and biological environment adaptability of PA3.
Exogenous glutamine can enhance the killing of ampicillin against multi-drug-resistant P. aeruginosa and other fungi. In a mouse model of urinary tract infection, glutamine enhanced the lethality of ampicillin and was effective against systemic infection caused by P. aeruginosa. Exogenous glutamine stimulates the influx of ampicillin, accumulating intracellular antibiotic concentrations above tolerated levels. The pathway is dependent on the genes/proteins CpxA/R, OmpF, purF, purD, purL, purE, purB, purH, and purE (Zhao et al., 2021). Loss of purH affects the pathway through which exogenous glutamine regulates membrane permeability and significantly increases antibiotic resistance in antibiotic-susceptible strains (Zhao et al., 2021). Therefore, we believe that the methylation of specific sites on purH through specific methyltransferases down-regulates the expression of purH and therefore increases the multiple antibiotics resistance of PA3 (Supplementary Table 8).
The synthesis of polyhydroxyalkanoic acid (PHA) is controlled by an integrated polymerization-depolymerization system of polymerases (PhaC1 and PhaC2) and depolymerase (PhaZ). It has been found that the P. aeruginosa PA14 phaZ mutant accumulated about 300% more PHA than the PA14 wild-type strain (Choi et al., 2011). Likewise, the inactivation of phaZ in Pseudomonas putida KT2442 (Cai et al., 2009) and Pseudomonas fluorescens BM07 (Choi et al., 2010) strains resulted in increased PHA accumulation. Pseudomonas In stress tolerance and biofilm formation, PHA-negative mutants establish more complex and differentiated biofilms than wild-type, which is thought to adapt to starvation stress due to the lack of ability to reserve PHA. The PHA-negative mutant was more heat sensitive than the non-mutant strain (Pham et al., 2004). The methylation site of PhaZ gene indicates a control mechanism regulating P. aeruginosa’s response to heat and other environmental changes.
Gene lexA has been extensively researched for its prodigious role in bacterial DNA damage repair. LexA inhibits the expression of SOS genes which is important for bacteria to respond to DNA damage. When DNA damage occurs, LexA is cleaved through complex signal transduction to ensure all genes involved in the SOS response are fully expressed (Kreuzer, 2013). This essential transcription factor also regulates the induction of phages, the migration of pathogenicity islands, and the production of virulence factors and bacteriocins (Fornelos et al., 2016). Moreover, the research of lexA also involves anti-drug resistance and biofilm formation (Cirz et al., 2006). And it was predicted that lexA may play an inhibitory role in motility function (Chellappa et al., 2013). Here we revealed the methylation site in lexA. Through the methylation of lexA, the above-mentioned suppression of DNA damage repair, biofilm formation, and drug resistance can be removed, which increases the competitiveness and adaption of PA3, which requires further phenotype analysis experiments to confirm.
This study has its limitation of no experimental verification. The bioinformatics analysis can integrate existing literature and database information, reveal the association between DNA methylation and phenotype characteristics in P. aeruginosa, and provide theoretical guidance for subsequent experimental designs. Future studies will combine experimental verification and pure bioinformatics analysis to investigate the specific mechanisms of methylation regulation in bacterial adaptability and competitiveness, which is going to be reported in our next study.
In conclusion, this study mapped the genome of a clinical P. aeruginosa strain PA3 with third-generation sequencing SMRT. The genomic features were cross-annotated through several fully developed and comprehensive databases. The DNA methylation pattern of PA3 was illustrated including one major methylation modification type m6A. Moreover, 16 methylated sites with high scores and coverage, which we believe may have a potential role in gene regulation were picked. Among these, purH, phaZ, and lexA are of great significance playing an important role in the drug resistance and biological environment adaptability of PA3, and the targeting of these genes may benefit further antibacterial studies.
Data availability statement
The datasets used and/or analyzed during the current study areavailable from the corresponding author upon reasonable request.
Ethics statement
This is an original research article on bacteria sequencing data. For this type of study, the requirement for ethics approval is waived by the Medical Ethics Committee of the Second Affiliated Hospital (Xinqiao Hospital) of Army Medical University, PLA.
Author contributions
ZL and XZhou contributed equally to this work. ZY and YT contributed equally to this work. All authors contributed to the article and approved the submitted version.
Funding
This work was supported by the National Natural Science Foundation of China (grant no. 82002051 and 81772073), the Natural Science Foundation of Chongqing CSTC (cstc2021jcyj-msxmX0655), and the Doctor Through Line Project of Chongqing CSTB (CSTB2022BSXM-JCX0019), the Foundation of Open Projects from State Key Laboratory of Trauma, Burns and Combined Injury (No. SKLKF201918), and the Young Talents Program of Army medical university.
Acknowledgment
We appreciate the professional suggestions made by Ni Zhen from the Institute of Burn Research, State Key Laboratory of Trauma, Burns and Combined Injury, Southwest Hospital, the First Affiliated Hospital, Army Medical University (the Third Military Medical University) during the preparation and polishing of the manuscript.
Conflict of interest
The authors declare that the research was conducted in the absence of any commercial or financial relationships that could be construed as a potential conflict of interest.
Publisher’s note
All claims expressed in this article are solely those of the authors and do not necessarily represent those of their affiliated organizations, or those of the publisher, the editors and the reviewers. Any product that may be evaluated in this article, or claim that may be made by its manufacturer, is not guaranteed or endorsed by the publisher.
Supplementary material
The Supplementary Material for this article can be found online at: https://www.frontiersin.org/articles/10.3389/fcimb.2023.1180194/full#supplementary-material
Supplementary Figure 1 | The original phylogenetic tree of P. aeruginosa PA3.
Supplementary Figure 2 | The quality value (QV) and motif base count change of P. aeruginosa PA3.
Supplementary Table 1 | Detailed list of virulence factors of P. aeruginosa PA3.
Supplementary Table 2 | Two-component systems of P. aeruginosa PA3.
Supplementary Table 3 | Transcription factors of P. aeruginosa PA3.
Supplementary Table 4 | Other DNA-binding proteins of P. aeruginosa PA3.
Supplementary Table 5 | Secretory proteins of P. aeruginosa PA3.
Supplementary Table 6 | Type II toxin-antitoxin (TA) of P. aeruginosa PA3.
Supplementary Table 7 | Genomic islands of P. aeruginosa PA3.
Supplementary Table 8 | Drug sensitive tests of wide type PA3.
References
Adhikari, A., Nandi, S., Bhattacharya, I., Roy, M. D., Mandal, T., Dutta, S. (2015). Phylogenetic analysis based evolutionary study of 16S rRNA in known Pseudomonas sp. Bioinformation 11 (10), 474–480. doi: 10.6026/97320630011474
Alikhan, N. F., Petty, N. K., Zakour Ben, N. L., Beatson, S. A. (2011). BLAST Ring Image Generator (BRIG): simple prokaryote genome comparisons. BMC Genomics 12, 402. doi: 10.1186/1471-2164-12-402
Angiuoli, S. V., Gussman, A., Klimke, W., Cochrane, G., Field, D., Garrity, G., et al. (2008). Toward an online repository of Standard Operating Procedures (SOPs) for (meta)genomic annotation. Omics: J. Integr. Biol. 12 (2), 137–141. doi: 10.1089/omi.2008.0017
Anton, B. P., Roberts, R. J. (2021). Beyond restriction modification: epigenomic roles of DNA methylation in prokaryotes. Annu. Rev. Microbiol. 75, 129–149. doi: 10.1146/annurev-micro-040521-035040
Aras, K., Good, W., Tate, J., Burton, B., Brooks, D., Coll-Font, J., et al. (2015). Experimental data and geometric analysis repository-EDGAR. J. Electrocardiol. 48 (6), 975–981. doi: 10.1016/j.jelectrocard.2015.08.008
Ardui, S., Ameur, A., Vermeesch, J. R., Hestand, M. S. (2018). Single molecule real-time (SMRT) sequencing comes of age: applications and utilities for medical diagnostics. Nucleic Acids Res. 46 (5), 2159–2168. doi: 10.1093/nar/gky066
Attar, N. (2016). Bacterial genetics: SMRT-seq reveals an epigenetic switch. Nat. Rev. Microbiol. 14 (9), 546. doi: 10.1038/nrmicro.2016.122
Barakat, M., Ortet, P., Whitworth, D. E. (2013). P2RP: a Web-based framework for the identification and analysis of regulatory proteins in prokaryotic genomes. BMC Genomics 14, 269. doi: 10.1186/1471-2164-14-269
Bertelli, C., Laird, M. R., Williams, K. P., Lau, B. Y., Hoad, G., Winsor, G. L., et al. (2017). IslandViewer 4: expanded prediction of genomic islands for larger-scale datasets. Nucleic Acids Res. 45 (W1), W30–W35. doi: 10.1093/nar/gkx343
Boratyn, G. M., Camacho, C., Cooper, P. S., Coulouris, G., Fong, A., Ma, N., et al. (2013). BLAST: a more efficient report with usability improvements. Nucleic Acids Res. 41 (Web Server issue), W29–W33. doi: 10.1093/nar/gkt282
Brown, C. L., Mullet, J., Hindi, F., Stoll, J. E., Gupta, S., Choi, M., et al. (2022). mobileOG-db: a manually curated database of protein families mediating the life cycle of bacterial mobile genetic elements. Appl. Environ. Microbiol. 88 (18), e0099122. doi: 10.1128/aem.00991-22
Cai, L., Yuan, M. Q., Liu, F., Jian, J., Chen, G. Q. (2009). Enhanced production of medium-chain-length polyhydroxyalkanoates (PHA) by PHA depolymerase knockout mutant of Pseudomonas putida KT2442. Bioresour. Technol. 100 (7), 2265–2270. doi: 10.1016/j.biortech.2008.11.020
Chaudhari, N. M., Gupta, V. K., Dutta, C. (2016). BPGA- an ultra-fast pan-genome analysis pipeline. Sci. Rep. 6, 24373. doi: 10.1038/srep24373
Chellappa, S. T., Maredia, R., Phipps, K., Haskins, W. E., Weitao, T. (2013). Motility of Pseudomonas aeruginosa contributes to SOS-inducible biofilm formation. Res. In Microbiol. 164 (10), 1019–1027. doi: 10.1016/j.resmic.2013.10.001
Choi, M. H., Xu, J., Gutierrez, M., Yoo, T., Cho, Y. H., Yoon, S. C. (2011). Metabolic relationship between polyhydroxyalkanoic acid and rhamnolipid synthesis in Pseudomonas aeruginosa: comparative ¹³C NMR analysis of the products in wild-type and mutants. J. Biotechnol. 151 (1), 30–42. doi: 10.1016/j.jbiotec.2010.10.072
Choi, M. H., Xu, J., Rho, J. K., Zhao, X. P., Yoon, S. C. (2010). Enhanced production of longer side-chain polyhydroxyalkanoic acid with omega-aromatic group substitution in phaZ-disrupted Pseudomonas fluorescens BM07 mutant through unrelated carbon source cometabolism and salicylic acid beta-oxidation inhibition. Bioresour. Technol. 101 (12), 4540–4548. doi: 10.1016/j.biortech.2010.01.082
Cirz, R. T., O’Neill, B. M., Hammond, J. A., Head, S. R., Romesberg, F. E. (2006). Defining the Pseudomonas aeruginosa SOS response and its role in the global response to the antibiotic ciprofloxacin. J. Bacteriol. 188 (20), 7101–7110. doi: 10.1128/JB.00807-06
Cohen, N. R., Ross, C. A., Jain, S., Shapiro, R. S., Gutierrez, A., Belenky, P., et al. (2016). A role for the bacterial GATC methylome in antibiotic stress survival. Nat. Genet. 48 (5), 581–586. doi: 10.1038/ng.3530
Dan, R., Marin, K. (2021). The epidemiology and pathogenesis and treatment of Pseudomonas aeruginosa infections: an update. Drugs 81 (18), 2117–2131. doi: 10.1007/s40265-021-01635-6
Dimopoulos, G., Akova, M., Rello, J., Poulakou, G. (2020). Understanding resistance in pseudomonas. Intensive Care Med. 46 (2), 350–352. doi: 10.1007/s00134-019-05905-6
Doberenz, S., Eckweiler, D., Reichert, O., Jensen, V., Bunk, B., Spröer, C., et al. (2017). Identification of a pseudomonas aeruginosa PAO1 DNA methyltransferase, its targets, and physiological roles. MBio 8 (1), e02312-16. doi: 10.1128/mBio.02312-16
Eid, J., Fehr, A., Gray, J., Luong, K., Lyle, J., Otto, G., et al. (2009). Real-time DNA sequencing from single polymerase molecules. Sci. (New York N.Y.) 323 (5910), 133–138. doi: 10.1126/science.1162986
Flusberg, B. A., Webster, D. R., Lee, J. H., Travers, K. J., Olivares, E. C., Clark, T. A., et al. (2010). Direct detection of DNA methylation during single-molecule, real-time sequencing. Nat. Methods 7 (6), 461–465. doi: 10.1038/nmeth.1459
Fornelos, N., Browning, D. F., Butala, M. (2016). The use and abuse of LexA by mobile genetic elements. Trends In Microbiol. 24 (5), 391–401. doi: 10.1016/j.tim.2016.02.009
Gold, M., Hurwitz, J., Anders, M. (1963). The enzymatic methylation OF RNA and DNA, II. On the species specificity of the methylation enzymes. Proc. Natl. Acad. Sci. USA 50 (1), 164–169. doi: 10.1073/pnas.50.1.164
Gu, W., Miller, S., Chiu, C. Y. (2019). Clinical metagenomic next-generation sequencing for pathogen detection. Annu. Rev. Pathol. 14, 319–338. doi: 10.1146/annurev-pathmechdis-012418-012751
Guo, J., Bolduc, B., Zayed, A. A., Varsani, A., Dominguez-Huerta, G., Delmont, T. O., et al. (2021). VirSorter2: a multi-classifier, expert-guided approach to detect diverse DNA and RNA viruses. Microbiome 9 (1), 37. doi: 10.1186/s40168-020-00990-y
Han, S., Liu, J., Li, M., Zhang, Y., Duan, X., Zhang, Y., et al. (2022). DNA methyltransferase regulates nitric oxide homeostasis and virulence in a chronically adapted pseudomonas aeruginosa strain. MSystems 7 (5), e0043422. doi: 10.1128/msystems.00434-22
Huang, W., Hamouche, J. E., Wang, G., Smith, M., Yin, C., Dhand, A., et al. (2020). Integrated genome-wide analysis of an isogenic pair of Pseudomonas aeruginosa clinical isolates with differential antimicrobial resistance to Ceftolozane/Tazobactam, Ceftazidime/Avibactam, and Piperacillin/Tazobactam. Int. J. Mol. Sci. 21 (3), 1026. doi: 10.3390/ijms21031026
Hung, C. L., Lin, Y. S., Lin, C. Y., Chung, Y. C., Chung, Y. F. (2015). CUDA ClustalW: an efficient parallel algorithm for progressive multiple sequence alignment on Multi-GPUs. Comput. Biol. Chem. 58, 62–68. doi: 10.1016/j.compbiolchem.2015.05.004
Juhas, M., van der Meer, J. R., Gaillard, M., Harding, R. M., Hood, D. W., Crook, D. W. (2009). Genomic islands: tools of bacterial horizontal gene transfer and evolution. FEMS Microbiol. Rev. 33 (2), 376–393. doi: 10.1111/j.1574-6976.2008.00136.x
Kamruzzaman, M., Wu, A. Y., Iredell, J. R. (2021). Biological functions of type II toxin-antitoxin systems in bacteria. Microorganisms 9 (6), 1276. doi: 10.3390/microorganisms9061276
Kreuzer, K. N. (2013). DNA damage responses in prokaryotes: regulating gene expression, modulating growth patterns, and manipulating replication forks. Cold Spring Harbor Perspect. In Biol. 5 (11), a012674. doi: 10.1101/cshperspect.a012674
Li, J., Li, J.-W., Feng, Z., Wang, J., An, H., Liu, Y., et al. (2016). Epigenetic switch driven by DNA inversions dictates phase variation in Streptococcus pneumoniae. PloS Pathog. 12 (7), e1005762. doi: 10.1371/journal.ppat.1005762
Li, G., Shen, M., Le, S., Tan, Y., Li, M., Zhao, X., et al. (2016). Genomic analyses of multidrug resistant Pseudomonas aeruginosa PA1 resequenced by single-molecule real-time sequencing. Biosci. Rep. 36 (6), e00418. doi: 10.1042/bsr20160282
Lu, S., Le, S., Li, G., Shen, M., Tan, Y., Zhao, X., et al. (2015). Complete genome sequence of Pseudomonas aeruginosa PA1, isolated from a patient with a respiratory tract infection. Genome Announc. 3 (6), e01453–15. doi: 10.1128/genomeA.01453-15
Nakano, K., Shiroma, A., Shimoji, M., Tamotsu, H., Ashimine, N., Ohki, S., et al. (2017). Advantages of genome sequencing by long-read sequencer using SMRT technology in medical area. Hum. Cell 30 (3), 149–161. doi: 10.1007/s13577-017-0168-8
Oliveira, P. H., Fang, G. (2021). Conserved DNA methyltransferases: a window into fundamental mechanisms of epigenetic regulation in bacteria. Trends Microbiol. 29 (1), 28–40. doi: 10.1016/j.tim.2020.04.007
Parkins, M. D., Somayaji, R., Waters, V. J. (2018). Epidemiology, biology, and impact of clonal Pseudomonas aeruginosa infections in cystic fibrosis. Clin. Microbiol. Rev. 31 (4), e00019–18. doi: 10.1128/CMR.00019-18
Pham, T. H., Webb, J. S., Rehm, B. H. (2004). The role of polyhydroxyalkanoate biosynthesis by Pseudomonas aeruginosa in rhamnolipid and alginate production as well as stress tolerance and biofilm formation. Microbiol. (Reading England) 150 (Pt 10), 3405–3413. doi: 10.1099/mic.0.27357-0
Pleška, M., Qian, L., Okura, R., Bergmiller, T., Wakamoto, Y., Kussell, E., et al. (2016). Bacterial autoimmunity due to a restriction-modification system. Curr. Biol.: CB 26 (3), 404–409. doi: 10.1016/j.cub.2015.12.041
Quinaud, M., Chabert, J., Faudry, E., Neumann, E., Lemaire, D., Pastor, A., et al. (2005). The PscE-PscF-PscG complex controls type III secretion needle biogenesis in Pseudomonas aeruginosa. J. Biol. Chem. 280 (43), 36293–36300. doi: 10.1074/jbc.M508089200
Roberts, R. J., Vincze, T., Posfai, J., Macelis, D. (2022). REBASE: a database for DNA restriction and modification: enzymes, genes and genomes. Nucleic Acids Res. Null. 51 (D1), D629–D630. doi: 10.1093/nar/gkac975
Schadt, E. E., Turner, S., Kasarskis, A. (2010). A window into third-generation sequencing. Hum. Mol. Genet. 19 (R2), R227–R240. doi: 10.1093/hmg/ddq416
Som, A., Fuellen, G. (2009). The effect of heterotachy in multigene analysis using the neighbor joining method. Mol. Phylogenet. Evol. 52 (3), 846–851. doi: 10.1016/j.ympev.2009.05.025
Sonnhammer, E. L., von Heijne, G., Krogh, A. (1998). “A hidden Markov model for predicting transmembrane helices in protein sequences,” in Proceedings. International Conference on Intelligent Systems for Molecular Biology 6, 175–182.
Stover, C. K., Pham, X. Q., Erwin, A. L., Mizoguchi, S. D., Warrener, P., Hickey, M. J., et al. (2000). Complete genome sequence of Pseudomonas aeruginosa PAO1, an opportunistic pathogen. Nature 406 (6799), 959–964. doi: 10.1038/35023079
Taguchi, F., Ichinose, Y. (2011). Role of type IV pili in virulence of Pseudomonas syringae pv. tabaci 6605: correlation of motility, multidrug resistance, and HR-inducing activity on a nonhost plant. Mol. Plant-Microbe Interactions: MPMI 24 (9), 1001–1011. doi: 10.1094/mpmi-02-11-0026
Tamura, K., Stecher, G., Kumar, S. (2021). MEGA11: molecular evolutionary genetics analysis version 11. Mol. Biol. Evol. 38 (7), 3022–3027. doi: 10.1093/molbev/msab120
Teufel, F., Armenteros Almagro, J. J., Johansen, A. R., Gíslason, M. H., Pihl, SI., Tsirigos, K. D., et al. (2022). SignalP 6.0 predicts all five types of signal peptides using protein language models. Nat. Biotechnol. 40 (7), 1023–1025. doi: 10.1038/s41587-021-01156-3
Veetilvalappil, V. V., Manuel, A., Aranjani, J. M., Tawale, R., Koteshwara, A. (2022). Pathogenic arsenal of Pseudomonas aeruginosa: an update on virulence factors. Future Microbiol. 17, 465–481. doi: 10.2217/fmb-2021-0158
Vernikos, G. S., Parkhill, J. (2006). Interpolated variable order motifs for identification of horizontally acquired DNA: revisiting the Salmonella pathogenicity islands. Bioinf. (Oxford England) 22 (18), 2196–2203. doi: 10.1093/bioinformatics/btl369
Winsor, G. L., Griffiths, E. J., Lo, R., Dhillon, B. K., Shay, J. A., Brinkman, F. S. (2016). Enhanced annotations and features for comparing thousands of Pseudomonas genomes in the Pseudomonas genome database. Nucleic Acids Res. 44 (D1), D646–D653. doi: 10.1093/nar/gkv1227
Yang, B., Wang, Y., Qian, P. Y. (2016). Sensitivity and correlation of hypervariable regions in 16S rRNA genes in phylogenetic analysis. BMC Bioinf. 17, 135. doi: 10.1186/s12859-016-0992-y
Yang, X., Li, Y., Zang, J., Li, Y., Bie, P., Lu, Y., et al. (2016). Analysis of pan-genome to identify the core genes and essential genes of Brucella spp. Mol. Genet. Genomics: MGG 291 (2), 905–912. doi: 10.1007/s00438-015-1154-z
Yang, Y., Scott, SA. (2017). DNA methylation profiling using long-read single molecule real-time bisulfite sequencing (SMRT-BS). Methods Mol. Biol. (Clifton N.J.) 1654, 125–134. doi: 10.1007/978-1-4939-7231-9_8
Zautner, A. E., Goldschmidt, A. M., Thürmer, A., Schuldes, J., Bader, O., Lugert, R., et al. (2015). SMRT sequencing of the Campylobacter coli BfR-CA-9557 genome sequence reveals unique methylation motifs. BMC Genomics 16, 1088. doi: 10.1186/s12864-015-2317-3
Keywords: Pseudomonas aeruginosa, SMRT, comparative genome analysis, DNA methylation analysis, epigenetics, antibacterial
Citation: Li Z, Zhou X, Liao D, Liu R, Zhao X, Wang J, Zhong Q, Zeng Z, Peng Y, Tan Y and Yang Z (2023) Comparative genomics and DNA methylation analysis of Pseudomonas aeruginosa clinical isolate PA3 by single-molecule real-time sequencing reveals new targets for antimicrobials. Front. Cell. Infect. Microbiol. 13:1180194. doi: 10.3389/fcimb.2023.1180194
Received: 05 March 2023; Accepted: 31 July 2023;
Published: 18 August 2023.
Edited by:
Yaxian Yan, Shanghai Jiao Tong University, ChinaCopyright © 2023 Li, Zhou, Liao, Liu, Zhao, Wang, Zhong, Zeng, Peng, Tan and Yang. This is an open-access article distributed under the terms of the Creative Commons Attribution License (CC BY). The use, distribution or reproduction in other forums is permitted, provided the original author(s) and the copyright owner(s) are credited and that the original publication in this journal is cited, in accordance with accepted academic practice. No use, distribution or reproduction is permitted which does not comply with these terms.
*Correspondence: Yinling Tan, dGFueWlubGluZzIwMTFAMTYzLmNvbQ==; Zichen Yang, emljaGVuX3lhbmdAeWFob28uY29t
†These authors have contributed equally to this work