- 1Munich Medical Research School, Ludwig Maximilian University of Munich (LMU), Munich, Germany
- 2Department of Gastroenterology and Hepatology, the First Affiliated Hospital of Nanchang Medical College, Jiangxi Provincial People’s Hospital, Nanchang, Jiangxi, China
The tuberculosis (TB) burden remains a significant global public health concern, especially in less developed countries. While pulmonary tuberculosis (PTB) is the most common form of the disease, extrapulmonary tuberculosis, particularly intestinal TB (ITB), which is mostly secondary to PTB, is also a significant issue. With the development of sequencing technologies, recent studies have investigated the potential role of the gut microbiome in TB development. In this review, we summarized studies investigating the gut microbiome in both PTB and ITB patients (secondary to PTB) compared with healthy controls. Both PTB and ITB patients show reduced gut microbiome diversity characterized by reduced Firmicutes and elevated opportunistic pathogens colonization; Bacteroides and Prevotella were reported with opposite alteration in PTB and ITB patients. The alteration reported in TB patients may lead to a disequilibrium in metabolites such as short-chain fatty acid (SCFA) production, which may recast the lung microbiome and immunity via the “gut-lung axis”. These findings may also shed light on the colonization of Mycobacterium tuberculosis in the gastrointestinal tract and the development of ITB in PTB patients. The findings highlight the crucial role of the gut microbiome in TB, particularly in ITB development, and suggest that probiotics and postbiotics might be useful supplements in shaping a balanced gut microbiome during TB treatment.
1 Introduction
Tuberculosis (TB) caused by Mycobacterium tuberculosis is one of the leading infectious disease killers worldwide (Avoi and Liaw, 2021). According to the latest WHO report, it is estimated that a quarter of the global population is infected with M. tuberculosis. Even though only about 5-10% of infected people develop active TB, in 2020 alone, the incidence of TB was about 127 cases per 100,000 people, and approximately 1.3 million HIV-negative people died of TB (WHO, 2021). Furthermore, most TB cases were reported in less developed regions, especially in South-East Asia, Africa, and the Western Pacific regions (WHO, 2021). However, the incidence might be underestimated as in some areas, especially in sub-Saharan Africa, the diagnosis of TB is still a challenge, and it is estimated that approximately 50% of TB cases remain undiagnosed (Mnyambwa et al., 2021; Jayasooriya et al., 2022). In the year 2015, all WHO members adopted the WHO’s End TB strategy which aims to reduce the absolute number of TB deaths by 95% and the incidence rate by 90% by 2035 compared to the 2015 baseline. Six years have passed, and the incidence of TB has only dropped by 10%. With only 13 years left, the situation is still challenging.
TB is transmitted by cough-generated aerosols from patients, and it primarily affects the lungs, causing pulmonary tuberculosis (PTB) (Tan et al., 2020). However, it can also involve other parts of the body. TB that affects areas outside the lungs is called extrapulmonary tuberculosis. Approximately 1-3% of total TB cases (Sheer and Coyle, 2003; Cho et al., 2018) and 10% of all extrapulmonary tuberculosis cases involve the gastrointestinal tract, causing intestinal tuberculosis (ITB) (Abu-Zidan and Sheek-Hussein, 2019; Maulahela et al., 2022). Swallowing of sputum in PTB patients has a certain chance of causing ITB (Gan et al., 2016). This is because M. tuberculosis is more resistant to the gastric acid barrier due to its special cell wall structure (Vandal et al., 2009). However, not all PTB patients develop ITB, as they might benefit from the protective effect of the intestinal barrier.
The intestinal barrier is a highly complex system, including the outer mucus layer, the epithelial layer, the underlying lamina propria, and components such as commensal microbiota, antimicrobial peptides, secretory immunoglobulin A, and immune cells (König et al., 2016; Vancamelbeke and Vermeire, 2017). Intestinal microbiota with a complex and dynamic microbial community is of vital importance to human health (Chen et al., 2021). It can not only regulate host physiological processes such as digestion, nutrient absorption, and metabolism, but also modulate host immunity in protection against pathogens and toxins (Wang et al., 2017; Comberiati et al., 2021). It is of great importance in gut homeostasis and colonization resistance to exogenous pathogens (Ducarmon et al., 2019), and dysbiosis in microbiome composition can result in susceptibility to infections and disease development (Budden et al., 2017). It is reported that altered microbiota composition can cause increased epithelial permeability and disruption in the mucus layer, resulting in susceptibility to Clostridioides difficile (Bien et al., 2013) and Citrobacter rodentium infection (Wlodarska et al., 2011). A recent study in patients with COVID-19 observed significant gut dysbiosis with enrichment of opportunistic pathogens (Zuo et al., 2020). Therefore, the gut microbiome of the host might also be crucial in preventing TB infection or decelerating the disease progression (Hu et al., 2019b).
With the universal application of Next-Generation Sequencing and bioinformatic analysis, there are increasing studies investigating the association between M. tuberculosis infection and alteration of gut microbiota. Here, we reviewed all the previous reports on the intestinal microbiome in active TB patients (including PTB and ITB) without any treatment, summarized their main findings, and tried to deduce the reasons for ITB development in PTB patients.
2 Alteration of gut microbiome in active TB patients
M. tuberculosis infection is known to cause dysregulation of the immune system, resulting in dysregulation of the gut microbiome (Osei Sekyere et al., 2020). In this review, we included studies referring to the alterations in the gut microbiome of TB patients (Luo et al., 2017; Maji et al., 2018; Huang et al., 2019; Hu et al., 2019a; Hu et al., 2019b; Li et al., 2019; Namasivayam et al., 2020; Cao et al., 2021; He et al., 2021; Naidoo et al., 2021; Shi et al., 2021; Ding et al., 2022; Wang S. et al., 2022; Wang Y. et al., 2022; Yang et al., 2022; Ye et al., 2022; Yoon et al., 2022). All patients included in the study were without antibiotic treatment, as the antibiotics can result in dysbiosis and mask the results caused by M. tuberculosis infection (Hu et al., 2019a; Namasivayam et al., 2020). The main findings are summarized in Table 1 and Figure 1. The study design and sequencing techniques used in these studies are also included.
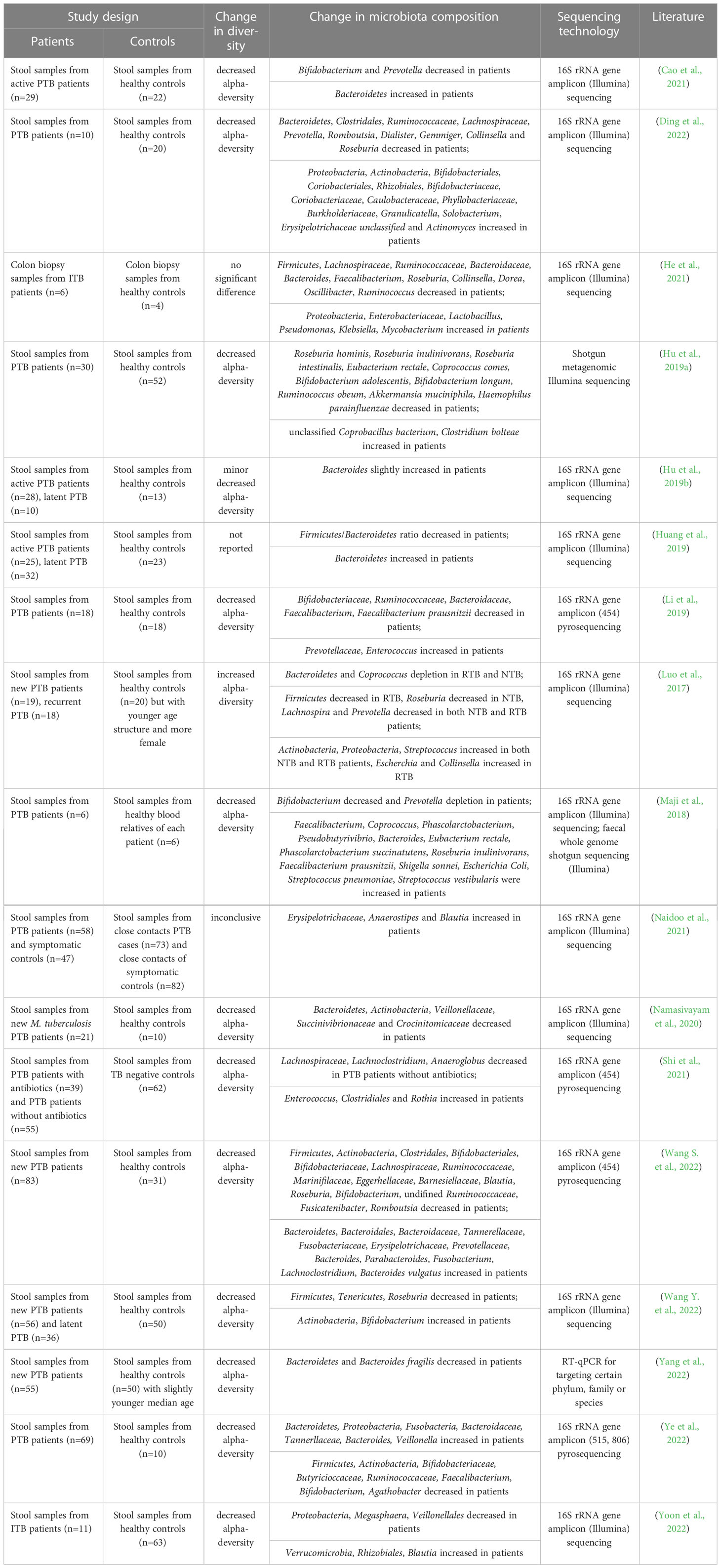
Table 1 Studies investigating the alteration of gut microbiome in pulmonary tuberculosis patients or intestinal tuberculosis patients without antibiotics comparing with the healthy controls.
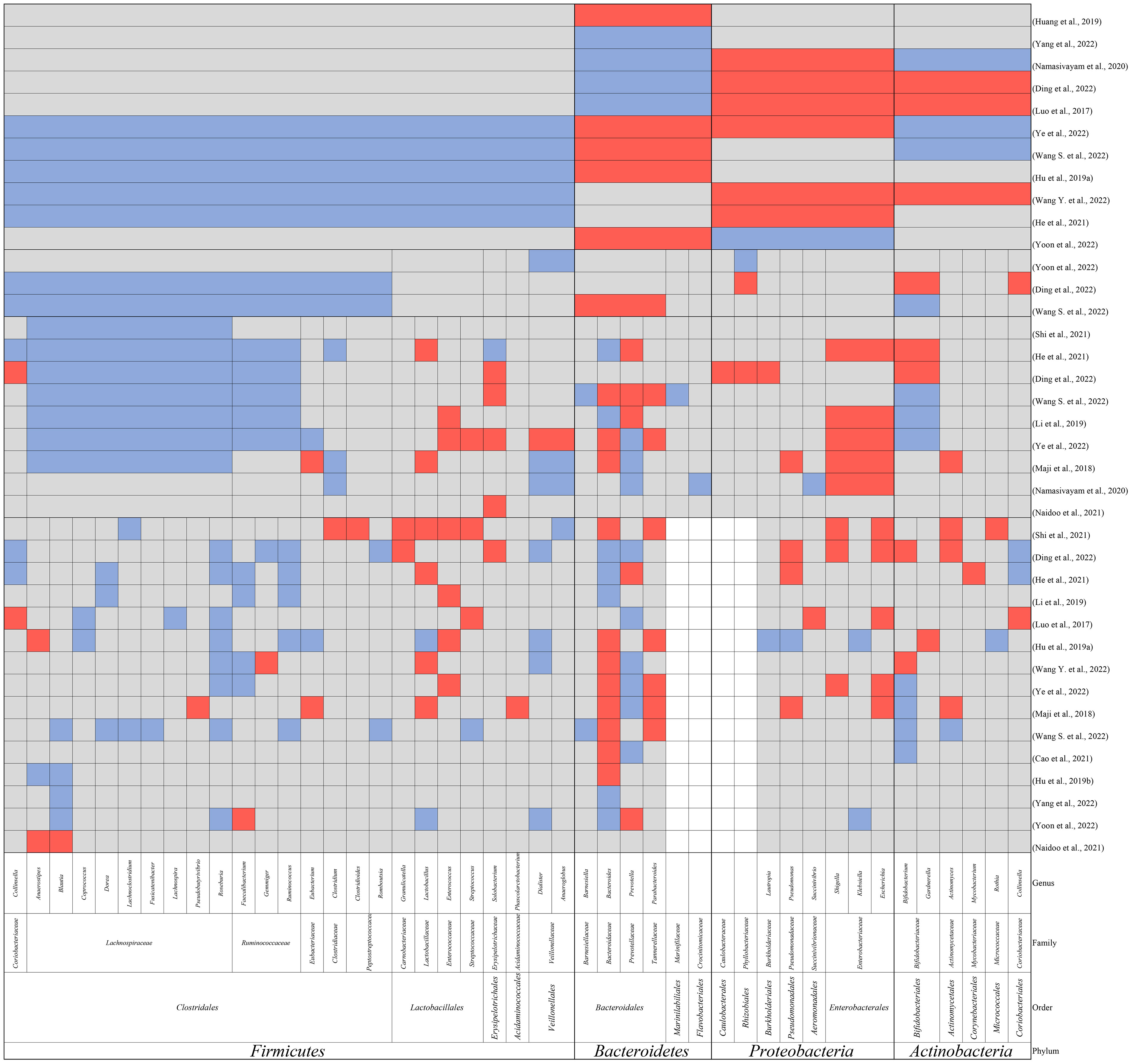
Figure 1 The main findings in alteration of gut microbiomes in TB patients compared to healthy controls at the phylum, order, family, and genus level. Red: elevation; blue: reduction; grey: not reported; white: no reported genus within the family.
Most of the studies found a decreased alpha-diversity in TB patients (Maji et al., 2018; Hu et al., 2019a; Hu et al., 2019b; Li et al., 2019; Namasivayam et al., 2020; Cao et al., 2021; He et al., 2021; Shi et al., 2021; Ding et al., 2022; Wang S. et al., 2022; Wang Y. et al., 2022; Yang et al., 2022; Ye et al., 2022; Yoon et al., 2022), with only one exception reporting increased diversity in both newly diagnosed PTB and recurrent PTB patients (Luo et al., 2017). However, it should be noted that the study by Luo et al. reported a significant difference in the age structure between the healthy control group and the two TB patient groups (Luo et al., 2017), which might have contributed to the observed enhancement in gut microbiome diversity. In a mouse model challenged with M. tuberculosis, dysbiosis resembling that observed in TB patients was observed in TB patients was reported (Winglee et al., 2014). The authors found a rapid initial post-infection reduction in alpha-diversity of the gut microbiome followed by slight recovery of diversity until death (Winglee et al., 2014). They proposed that the change in gut microbiome was due to the crosstalk between microbiota and immune system activation, while the recovery of diversity indicated the attainment of balance.
The dysbiosis observed in the gut microbiome of TB patients at the taxonomic level was mainly in the following aspects.
2.1 Firmicutes
Firmicutes, which play a role in nutrition and metabolism (Stojanov et al., 2020), are the most abundant microbiome in the healthy human colon, comprising 64% of the gut microbiome (Piccioni et al., 2022). The imbalance in the ratio of Firmicutes/Bacteroides was also reported to indicate disrupted intestinal homeostasis, pathogen invasion, or unhealthy conditions (Stojanov et al., 2020). The significant reduction in the phylum Firmicutes in TB patients was observed by several independent groups (Hu et al., 2019a; He et al., 2021; Wang S. et al., 2022; Wang Y. et al., 2022; Ye et al., 2022). The relationship between reduced Firmicutes and M. tuberculosis infection might be regarded as reciprocal causation. On one hand, the imbalanced microbiome composition caused by Firmicutes reduction might cause susceptibility to M. tuberculosis infection or the activation of TB in latent TB infection. On the other hand, the reduction of Firmicutes might also be triggered by the dysregulated immune system caused by M. tuberculosis infection.
Precisely, within Firmicutes, Clostridiales and Veillonellales were found to be decreased by some studies (Ding et al., 2022; Wang S. et al., 2022; Yoon et al., 2022). Meanwhile, many observations support the reduction of families Lachnospiraceae, Ruminococcaceae, and Clostridiaceae within Clostridiales (Maji et al., 2018; Li et al., 2019; He et al., 2021; Shi et al., 2021; Ding et al., 2022; Wang S. et al., 2022; Ye et al., 2022) and the reduction of Veillonellaceae within Veillonellales (Maji et al., 2018; Namasivayam et al., 2020). More interesting findings were observed at the genus level. Some of the most common genera in Firmicutes such as Faecalibacterium, Ruminococcus, Blautia, Roseburia, Lachnospira, Eubacterium, Coprococcus, and Dorea were all observed to be decreased (Luo et al., 2017; Hu et al., 2019a; Hu et al., 2019b; Li et al., 2019; He et al., 2021; Shi et al., 2021; Ding et al., 2022; Wang S. et al., 2022; Wang Y. et al., 2022; Yang et al., 2022; Ye et al., 2022; Yoon et al., 2022), whereas Granulicatella, Lactobacillus, Enterococcus, and Streptococcus were observed to be increased in patients (Luo et al., 2017; Maji et al., 2018; Hu et al., 2019a; Li et al., 2019; He et al., 2021; Shi et al., 2021; Ding et al., 2022; Wang Y. et al., 2022; Ye et al., 2022).
As mentioned earlier, the reduced genera primarily belong to the two most abundant families in Firmicutes, Lachnospiraceae and Ruminococcaceae. They are obligate anaerobic and butyrate-producing bacteria (Sorbara et al., 2020; Liu et al., 2021). Butyrate is a short-chain fatty acid (SCFA) that is an essential regulator for the maintenance of intestinal homeostasis (Parada Venegas et al., 2019). Butyrate can interact with G-coupled receptors such as GPR43, GPR41, and GPR109a (Hodgkinson et al., 2023), leading to increased regulatory T cells (Tregs) and dendritic cell precursors, improved epithelial barrier function, as well as the increased expression of anti-inflammatory cytokines such as IL-10 (Liu et al., 2018). Additionally, butyrate can also inhibit HDAC activity to decompact chromatin and upregulate gene expression, inducing Tregs and the antimicrobial activity in intestinal macrophages (Schulthess et al., 2019). In addition, Phenylbutyrate (PBA), a derivative of butyrate, has been found to induce the expression of antimicrobial peptides in lung epithelial cells (Steinmann et al., 2009) and directly restrict the growth of M. tuberculosis in vitro or even within macrophages (Coussens et al., 2015). In clinical trials for TB patients, PBA in combination with vitamin D has also been shown to increase the clearance of M. tuberculosis by inducing the antimicrobial peptide LL-37 (Mily et al., 2013; Mily et al., 2015), while also ameliorating inflammation and improving symptom relief (Bekele et al., 2018; Rekha et al., 2018). LL-37 was reported to disrupt the cell wall of intra- and extracellular M. tuberculosis (Deshpande et al., 2020) and also activate the autophagy of macrophages (Rekha et al., 2015). Therefore, a decreased butyrate level would result in elevated pro-inflammatory responses, reduced antimicrobial activity, and impaired epithelial barrier function (Chen et al., 2019b).
Conversely, the increased genera in patients all belong to the order Lactobacillales, a group of lactic acid-producing bacteria. Lactic acid bacteria are generally regarded as beneficial microorganisms that support the host’s gut homeostasis and enhance the epithelial barrier (Ren et al., 2020). However, it is also reported that lactic acid bacteria can induce Th1 and suppress Th2 responses during M. tuberculosis infection (Ghadimi et al., 2010). Meanwhile, it is also worth noting that some of the bacteria in Enterococcus, Streptococcus, and Granulicatella are opportunistic pathogens. The disrupted epithelial barrier caused by reduced butyrate can facilitate the colonization of these opportunistic pathogens.
2.2 Bacteroidetes
Bacteroidetes are the second most abundant microbiota in the healthy human colon, comprising 23% of the gut microbiota (Sánchez-Tapia et al., 2019). Similar to Firmicutes, alterations in Bacteroidetes are also important in metabolism and energy balance (Chen et al., 2019a). However, unlike Firmicutes, Bacteroidetes are the main producer of the other two members of SCFAs, namely acetate and propionate (Feng et al., 2018).
Despite the contradictory findings in the alteration of Bacteroidetes, the most predominant findings were related to the three most abundant genera in Bacteroidetes, namely Bacteroides, Prevotella, and Parabacteroides (Rinninella et al., 2019; Zafar and Saier, 2021). In most studies, Bacteroides and Parabacteroides were reported to be increased in TB patients while Prevotella was reported to be decreased (Maji et al., 2018; Hu et al., 2019a; Hu et al., 2019b; Shi et al., 2021; Wang S. et al., 2022; Wang Y. et al., 2022; Ye et al., 2022).
Both Bacteroides and Parabacteroides are acetate-producing bacteria. Like butyrate, acetate can enhance antimicrobial peptides such as defensins, and also increase the epithelial barrier repairment by inducing the production of IL-22 (Fachi et al., 2020). Defensin, such as defensin-1, was found to inhibit the intracellular growth of mycobacterium inside granulomas (Sharma et al., 2017). Moreover, acetate was also reported to increase phagocytosis and bacterial killing by macrophages and neutrophils (Galvão et al., 2018). In addition, Bacteroides was also one of the major sources of propionate in the gut microbiota (Louis and Flint, 2017). Propionate was also shown to have antimicrobial activity. Propionate produced by Bacteroides was reported to limit the colonization of many bacteria such as Salmonella (Jacobson et al., 2018) and E.coli (Ormsby et al., 2020) by regulating intracellular pH. However, it should not be neglected that acetate may also suppress CD4+ T cell activation and Th1 and Th17 response while propionate may suppress antigen-specific CD8+ T cell activation by alleviating the IL-12 production by dendritic cells (Nastasi et al., 2017). These effects may also increase the susceptibility of the host to infections (Ahn et al., 2017; Piccinni et al., 2019).
In contrast, studies have shown that Prevotella can augment Th17-mediated mucosal inflammation (Kempski et al., 2017) and increase epithelial permeability to bacterial products (Larsen, 2017). This might be because Prevotella can activate TLR2-signaling and induce the secretion of IL-6, IL-8, and CCL20 by epithelial cells (Tamanai-Shacoori et al., 2022), as well as the secretion of IL-1β, IL-6, and IL-23 by dendritic cells (Kwok et al., 2012). These cytokines can induce Th17 immune response and neutrophil recruitment, increasing infection severity and tissue damage (Larsen, 2017; Shen and Chen, 2018). Therefore, reduced Prevotella as well as increased Bacteroides and Parabacteroides might simultaneously exert an anti-inflammatory effect.
Intriguingly, in the context of ITB, there seems to be minor differences compared with PTB patients. The most significant observation would be the opposite trends with decreased Bacteroides and increased Prevotella in ITB patients (He et al., 2021; Yoon et al., 2022). As the major sources of both acetate and propionate, decreased Bacteroides together with downregulated Firmicutes in ITB patients would result in a dramatic depletion of SCFA production. Based on the critical role that SCFAs play in epithelial barrier function, antimicrobial protein production, and immunomodulation, this depletion would cause excessive immune responses, increased inflammatory lesions, and antimicrobial peptide production. It might also increase the invasion and colonization of M. tuberculosis and other opportunistic pathogens in the gut.
Moreover, the increased Prevotella would also increase the Th17 response inducing neutrophil accumulation and granuloma formation after M. tuberculosis infection (Seiler et al., 2003). However, when exposed to excessive IL-17 produced by Th17 cells, longer survival of neutrophils can cause increased neutrophil infiltration and the formation of pathological lesions (Torrado and Cooper, 2010). This is also in line with the observation of elevated IL-17 expression in ITB patients (Pugazhendhi et al., 2013).
2.3 Proteobacteria and Actinobacteria
At the phylum level, Proteobacteria were observed to be increased in TB patients (Luo et al., 2017; Namasivayam et al., 2020; He et al., 2021; Ding et al., 2022; Wang Y. et al., 2022), while conflicting trends were reported for Actinobacteria (Luo et al., 2017; Namasivayam et al., 2020; Ding et al., 2022; Wang S. et al., 2022; Wang Y. et al., 2022). However, at the genus level, Pseudomonas (Maji et al., 2018; He et al., 2021; Shi et al., 2021), Shigella (Shi et al., 2021; Ding et al., 2022)and Escherichia from Proteobacteria (Luo et al., 2017; Shi et al., 2021; Ding et al., 2022)and Actinomyces from Actinobacteria (Maji et al., 2018; Shi et al., 2021; Ding et al., 2022)were all reported to be increased in patients. These bacteria are all common opportunistic pathogens and are always associated with the disruption of mucosal barriers (Pujic et al., 2015). An imbalanced SCFA constitution alters the gut environment resulting in dysregulated immune response and breakdown of the epithelial barrier, causing the colonization of opportunistic pathogens.
3 Microbiome-immune crosstalk during M. tuberculosis infection
The gut microbiome and lung microbiome are not separate groups within an organism. They are tightly related by the so-called “gut-lung axis”, which means that the metabolites produced by the gut microbiome can reach the systemic circulation and shape the lung microbiome and the immune response in the lung, and vice versa (Enaud et al., 2020). Among the metabolites of the microbiome, SCFAs are the most extensively studied. SCFAs including acetate, propionate, and butyrate have been shown to have a modulatory role in the immune system and epithelial function.
In PTB patients, compared with healthy controls (Figure 2A), the main findings are the loss of Firmicutes such as Lachnospiraceae and Ruminococcaceae, and the enrichment of Bacteroidetes (Figure 2B). In the murine model challenged with M. tuberculosis, the authors also observed a post-infection reduction of butyrate-producing Lachnospiraceae and Ruminococcaceae and enrichment of acetate/propionate-producing Bacteroides, similar to the observations in humans (Winglee et al., 2014). Furthermore, two studies on the relationship between Helicobacter hepaticus and M. tuberculosis infection found that infection by Helicobacter hepaticus resulting in similar dysbiosis with increased Bacteroidaceae and decreased Clostridiales, Ruminococcaceae, Lachnospiraceae, and Prevotellaceae could cause hyperactivated immune response, overexpressed pro-inflammatory cytokines, and increased susceptibility to M. tuberculosis, resulting in severe lung damage (Arnold et al., 2015; Majlessi et al., 2017). These observations in patients and murine models may lead to the potential altered SCFA composition with decreased butyrate but increased acetate and propionate. A fecal metabolomic study also revealed slightly increased acetate and a significant decrease in butyrate in PTB patients (Wang S. et al., 2022).
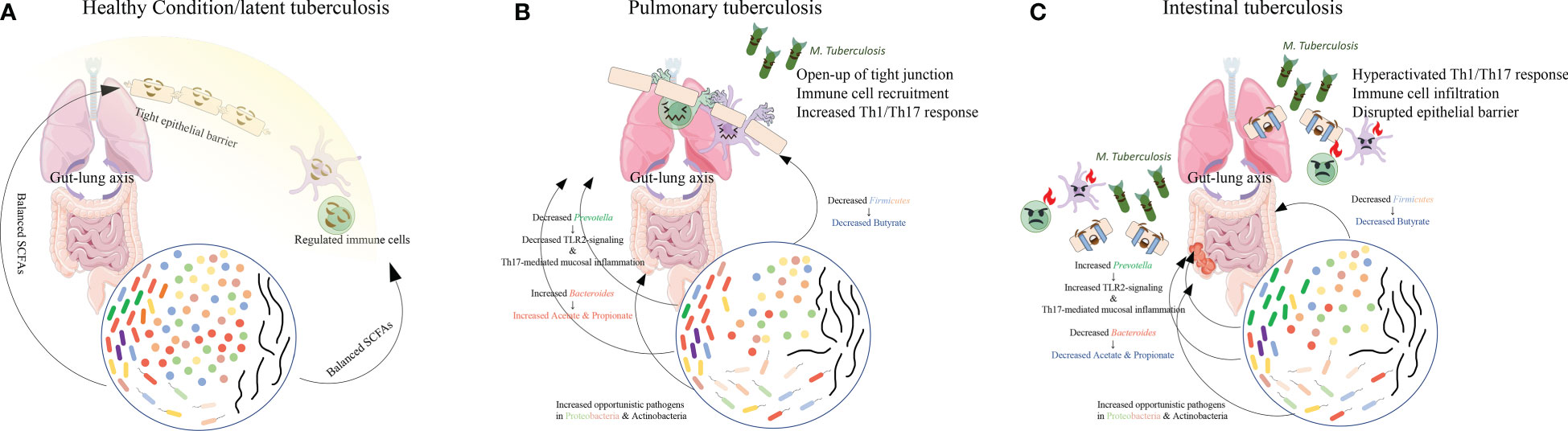
Figure 2 The main findings in gut microbiome composition in PTB and ITB patients. Compared with the healthy conditions (A), in PTB patients (B), reduced Firmicutes and Prevotella and increased Bacteroides altered the proportion of each SCFA, causing immune cell recruitment and mildly increased immune response. However, when Bacteroides decreased and Prevotella increased (C), decreased SCFAs production resulted in drastic activation of immune response and disruption of epithelial barrier, facilitating the colonization of M. tuberculosis in the intestine and the development of ITB.
Acetate, butyrate, and propionate are all SCFAs that can exert anti-inflammatory effects by binding to GPR41 and GPR43. However, butyrate is the only SCFA known to bind to GPR109A (Liu et al., 2018). In vivo experiments using Gpr109a-/- mice failed to ameliorate the inflammatory response and epithelial barrier dysfunction after sodium butyrate administration (Chen et al., 2018), indicating the importance of GPR109A in anti-inflammatory response and epithelial barrier construction. Another experiment using Gpr109a-/- mice observed dysregulated immune responses and increased M1 macrophage polarization (Zhang Z. et al., 2022). Increased acetate and propionate may remedy the loss of butyrate in GPR41 and GPR43 activation but may not rescue the loss of GPR109A activation. The loss of butyrate in the gut microbiome and further in the circulation by the “gut-lung axis” results in dysbiosis in the lung microbiome (Hu et al., 2020; Vázquez-Pérez et al., 2020; Xiao et al., 2022; Zhang M. et al., 2022), as well as the disruption of the lung epithelial barrier and upregulation of pro-inflammatory cytokines in the systemic circulation such as IFN-γ, TNF, and IL-17A (Machado et al., 2021). These pro-inflammatory cytokines and the opening up of tight junctions in the lung epithelial barrier can facilitate the migration of immune cells such as neutrophils and macrophages (Akdis, 2021). Macrophages and neutrophils are the first-line innate immune defense against M. tuberculosis by phagocytosis (Roca et al., 2019). Moreover, immune cells such as macrophages and dendritic cells can present antigens to T and B cells and augment adaptive immune responses. After infection, CD4+ T cells can not only further strengthen the innate immunity but also promote the function and survival of CD8+ T cells (Lu et al., 2021), whilst CD8+ T cells can directly kill M. tuberculosis by their cytolytic function (Lin and Flynn, 2015). Antibody opsonization was also shown to promote the phagocytosis of macrophages (Chandra et al., 2022).
However, when the SCFA level in circulation is sustainably reduced due to an imbalanced microbiome in TB, as observed in ITB patients with decreased Bacteroides (Figure 2C), the resulting depletion of IL-10 production and anti-inflammatory response can provoke the persistence of an overactivated pro-inflammatory response. Meanwhile, excessive TNF production was found to induce necroptosis of granuloma macrophages by activating the RIP1-RIP3 necroptosome (Stutz et al., 2018), which can facilitate bacterial replication and activation (Roca et al., 2019). Moreover, the increased Prevotella in ITB patients’ gut microbiota could further induce Th17 responses and aggravate neutrophil infiltration and pathological lesions in both lung and gut. The upregulated pro-inflammatory cytokine production may contribute to the overactivation of neutrophils and lead to impairment of mycobacterial controls within granulomas and thus exacerbate disease (Moreira-Teixeira et al., 2020). The observation of higher levels of neutrophils in the circulation of active TB patients also indicates the detrimental role of an overactivated immune response (Moideen et al., 2018). The uncontrolled replication and invasion of M. tuberculosis might facilitate its colonization in the gut and cause intestinal TB.
4 Perspectives and conclusions
The treatment of TB requires long-term multidrug treatment with a mixture of broad-spectrum and mycobacterial-specific antibiotics, especially for multidrug-resistant TB. However, it has also been reported that anti-TB medications can result in further dysbiosis of the intestinal microbiome in TB patients (Namasivayam et al., 2017; Wipperman et al., 2017; Hu et al., 2019b; Yoon et al., 2022). Intestinal microbiome disruption can also, in turn, limit the efficiency of treatment (Negi et al., 2020). A study of M. tuberculosis infection in mice pre-treated with isoniazid and pyrazinamide for 8 weeks also showed a higher lung bacterial burden. Besides, alleviated TNF and IL-1β production, decreased MHCII expression, and defective M. tuberculosis control were found in the alveolar macrophages of the mice. This phenotype can be partially reversed by fecal transplantation (Khan et al., 2019). Moreover, in our review, the current findings in TB patients also indicate a correlation between severely imbalanced gut microbiome with the development of ITB in PTB patients. Therefore, a balanced gut microbiome is crucial during M. tuberculosis infection. To achieve this goal, probiotics and postbiotics as potential routine supplements during TB treatment could be a one-stone-two-birds strategy.
Probiotics, such as Bacteroides fragilis and Lactobacillus plantarum, have already been considered novel probiotics in TB treatment (Liu et al., 2021; Eribo et al., 2022). B. fragilis has been reported to exert anti-inflammatory function by decreasing excessive IFN-γ and inducing IL-10 secretion in mice through its metabolite PSA (polysaccharide) (Johnson et al., 2015; Johnson et al., 2018). The study by Negi et al., also reported increased MHCII expression on lung dendritic cells and a lower M. tuberculosis burden in the lung of mice after treatment with Lactobacillus plantarum. Another in vitro study using Lacticaseibacillus rhamnosus PMC203 found a direct restriction in M. tuberculosis growth and increased killing ability in infected RAW 264.7 cells (Rahim et al., 2022).
Postbiotics, such as indole propionic acid, can inhibit M. tuberculosis by targeting tryptophan synthesis (Negatu et al., 2019). PBA as a derivative of probiotics (butyrate) has also been tested in clinical trials and observed to provide significant relief of symptoms (Bekele et al., 2018; Rekha et al., 2018). However, the usage and concentration of probiotics and postbiotics must be individualized in the context of the patients. For example, different concentrations of SCFAs might have distinct functions (Ashique et al., 2022). Another example is the usage of SCFAs, which might be helpful in normal TB patients, but detrimental in people with HIV co-infection (Machado et al., 2021)
As mentioned above, studies have shown that the gut microbiome alteration in general TB patients (PTB) is characterized by dysbiosis, which is defined as reduced butyrate-producing Firmicutes and Prevotella (Bacteroidetes), and increased lactic acid-producing Firmicutes, Bacteroides, Parabacteroides, and opportunistic pathogens in Proteobacteria and Actinobacteria. The most significant consequence of this alteration, given the abundance of Firmicutes and Bacteroidetes in the human gut microbiome, is the change in the composition of SCFAs, with reduced butyrate and increased acetate and propionate metabolite production. When acetate and propionate production is further decreased by the reduction of Bacteroides, there might be an increased susceptibility to M. tuberculosis infection in the gut, causing ITB. Therefore, the gut microbiome may act as the defense line in preventing ITB development. Probiotics and postbiotics could become potential supplements in TB treatment and ITB prevention.
Author contributions
ZY and JC designed the study. ZY and XS wrote the manuscript. AW and CH made contributions to the revision. All authors contributed to the article and approved the submitted version.
Funding
This study is supported by The National Natural Science Foundation of China (81960111) and Natural Science Foundation of Jiangxi Province (20202BABL206013). ZY has been supported by the China Scholarship Council (202008360174), XS has been supported by the China Scholarship Council (201909110092).
Acknowledgments
The authors would like to thank the reviewers of the manuscript, whose thoughtful and insightful comments helped to improve this review, and also thank the funding sources and all colleagues for useful discussion.
Conflict of interest
The authors declare that the research was conducted in the absence of any commercial or financial relationships that could be construed as a potential conflict of interest.
Publisher’s note
All claims expressed in this article are solely those of the authors and do not necessarily represent those of their affiliated organizations, or those of the publisher, the editors and the reviewers. Any product that may be evaluated in this article, or claim that may be made by its manufacturer, is not guaranteed or endorsed by the publisher.
References
Abu-Zidan, F. M., Sheek-Hussein, M. (2019). Diagnosis of abdominal tuberculosis: lessons learned over 30 years: pectoral assay. World J. Emergency Surg. 14, 1–7. doi: 10.1186/s13017-018-0220-3
Ahn, Y.-H., Jeon, S.-B., Chang, C. Y., Goh, E.-A., Kim, S. S., Kim, H. J., et al. (2017). Glatiramer acetate attenuates the activation of CD4+ T cells by modulating STAT1 and –3 signaling in glia. Sci. Rep. 7, 40484. doi: 10.1038/srep40484
Akdis, C. A. (2021). Does the epithelial barrier hypothesis explain the increase in allergy, autoimmunity and other chronic conditions? Nat. Rev. Immunol. 21, 739–751. doi: 10.1038/s41577-021-00538-7
Arnold, I. C., Hutchings, C., Kondova, I., Hey, A., Powrie, F., Beverley, P., et al. (2015). Helicobacter hepaticus infection in BALB/c mice abolishes subunit-vaccine-induced protection against m. tuberculosis. Vaccine 33, 1808–1814. doi: 10.1016/j.vaccine.2015.02.041
Ashique, S., De Rubis, G., Sirohi, E., Mishra, N., Rihan, M., Garg, A., et al. (2022). Short chain fatty acids: Fundamental mediators of the gut-lung axis and their involvement in pulmonary diseases. Chem. Biol. Interact. 368, 110231. doi: 10.1016/j.cbi.2022.110231
Avoi, R., Liaw, Y. C. (2021). Tuberculosis death epidemiology and its associated risk factors in sabah, Malaysia. Int. J. Environ. Res. Public Health 18, 9740. doi: 10.3390/ijerph18189740
Bekele, A., Gebreselassie, N., Ashenafi, S., Kassa, E., Aseffa, G., Amogne, W., et al. (2018). Daily adjunctive therapy with vitamin D3 and phenylbutyrate supports clinical recovery from pulmonary tuberculosis: a randomized controlled trial in Ethiopia. J. Internal Med. 284, 292–306. doi: 10.1111/joim.12767
Bien, J., Palagani, V., Bozko, P. (2013). The intestinal microbiota dysbiosis and clostridium difficile infection: is there a relationship with inflammatory bowel disease? Therap Adv. Gastroenterol. 6, 53–68. doi: 10.1177/1756283X12454590
Budden, K. F., Gellatly, S. L., Wood, D. L. A., Cooper, M. A., Morrison, M., Hugenholtz, P., et al. (2017). Emerging pathogenic links between microbiota and the gut–lung axis. Nat. Rev. Microbiol. 15, 55–63. doi: 10.1038/nrmicro.2016.142
Cao, D., Liu, W., Lyu, N., Li, B., Song, W., Yang, Y., et al. (2021). Gut mycobiota dysbiosis in pulmonary tuberculosis patients undergoing anti-tuberculosis treatment. Microbiol. Spectr. 9, e00615–e00621. doi: 10.1128/spectrum.00615-21
Chandra, P., Grigsby, S. J., Philips, J. A. (2022). Immune evasion and provocation by mycobacterium tuberculosis. Nat. Rev. Microbiol. 20, 750–766. doi: 10.1038/s41579-022-00763-4
Chen, L., Li, H., Li, J., Chen, Y., Yang, Y. (2019a). Lactobacillus rhamnosus GG treatment improves intestinal permeability and modulates microbiota dysbiosis in an experimental model of sepsis. Int. J. Mol. Med. 43(3), 1139–1148. doi: 10.3892/ijmm.2019.4050
Chen, G., Ran, X., Li, B., Li, Y., He, D., Huang, B., et al. (2018). Sodium butyrate inhibits inflammation and maintains epithelium barrier integrity in a TNBS-induced inflammatory bowel disease mice model. EBioMedicine 30, 317–325. doi: 10.1016/j.ebiom.2018.03.030
Chen, L., Sun, M., Wu, W., Yang, W., Huang, X., Xiao, Y., et al. (2019b). Microbiota metabolite butyrate differentially regulates Th1 and Th17 cells’ differentiation and function in induction of colitis. Inflammation Bowel Dis. 25(9), 1450–1461. doi: 10.1093/ibd/izz046
Chen, Y., Zhou, J., Wang, L. (2021). Role and mechanism of gut microbiota in human disease. Front. Cell Infect. Microbiol. 11, 625913. doi: 10.3389/fcimb.2021.625913
Cho, J.-K., Choi, Y. M., Lee, S. S., Park, H. K., Cha, R. R., Kim, W. S., et al. (2018). Clinical features and outcomes of abdominal tuberculosis in southeastern Korea: 12 years of experience. BMC Infect. Dis. 18, 1–8. doi: 10.1186/s12879-018-3635-2
Comberiati, P., Di Cicco, M., Paravati, F., Pelosi, U., Di Gangi, A., Arasi, S., et al. (2021). The role of gut and lung microbiota in susceptibility to tuberculosis. Int. J. Environ. Res. Public Health 18, 12220. doi: 10.3390/ijerph182212220
Coussens, A. K., Wilkinson, R. J., Martineau, A. R. (2015). Phenylbutyrate is bacteriostatic against mycobacterium tuberculosis and regulates the macrophage response to infection, synergistically with 25-Hydroxy-Vitamin D3. PloS Pathog. 11, e1005007. doi: 10.1371/journal.ppat.1005007
Deshpande, D., Grieshober, M., Wondany, F., Gerbl, F., Noschka, R., Michaelis, J., et al. (2020). Super-resolution microscopy reveals a direct interaction of intracellular mycobacterium tuberculosis with the antimicrobial peptide LL-37. Int. J. Mol. Sci. 21(18), 6741. doi: 10.3390/ijms21186741
Ding, X., Zhou, J., Chai, Y., Yan, Z., Liu, X., Dong, Y., et al. (2022). A metagenomic study of the gut microbiome in PTB’S disease. Microbes Infect. 24, 104893. doi: 10.1016/j.micinf.2021.104893
Ducarmon, Q. R., Zwittink, R. D., Hornung, B. V. H., Van Schaik, W., Young, V. B., Kuijper, E. J. (2019). Gut microbiota and colonization resistance against bacterial enteric infection. Microbiol. Mol. Biol. Rev. 83(3), e00007–19. doi: 10.1128/MMBR.00007-19
Enaud, R., Prevel, R., Ciarlo, E., Beaufils, F., Wieërs, G., Guery, B., et al. (2020). The gut-lung axis in health and respiratory diseases: A place for inter-organ and inter-kingdom crosstalks. Front. Cell Infect. Microbiol. 10, 9. doi: 10.3389/fcimb.2020.00009
Eribo, O. A., Du Plessis, N., Chegou, N. N. (2022). The intestinal commensal, bacteroides fragilis, modulates host responses to viral infection and therapy: Lessons for exploration during mycobacterium tuberculosis infection. Infect. Immun. 90, e0032121. doi: 10.1128/IAI.00321-21
Fachi, J. L., Sécca, C., Rodrigues, P. B., Mato, F. C. P. D., Di Luccia, B., Felipe, J. D. S., et al. (2020). Acetate coordinates neutrophil and ILC3 responses against c. difficile through FFAR2. J. Exp. Med. 217(3), jem.20190489. doi: 10.1084/jem.20190489
Feng, W., Ao, H., Peng, C. (2018). Gut microbiota, short-chain fatty acids, and herbal medicines. Front. Pharmacol. 9, 1354. doi: 10.3389/fphar.2018.01354
Galvão, I., Tavares, L. P., Corrêa, R. O., Fachi, J. L., Rocha, V. M., Rungue, M., et al. (2018). The metabolic sensor GPR43 receptor plays a role in the control of klebsiella pneumoniae infection in the lung. Front. Immunol. 9, 142. doi: 10.3389/fimmu.2018.00142
Gan, H., Mely, M., Zhao, J., Zhu, L. (2016). An analysis of the clinical, endoscopic, and pathologic features of intestinal tuberculosis. J. Clin. Gastroenterol. 50, 470–475. doi: 10.1097/MCG.0000000000000514
Ghadimi, D., De Vrese, M., Heller, K. J., Schrezenmeir, J. (2010). Lactic acid bacteria enhance autophagic ability of mononuclear phagocytes by increasing Th1 autophagy-promoting cytokine (IFN-γ) and nitric oxide (NO) levels and reducing Th2 autophagy-restraining cytokines (IL-4 and IL-13) in response to mycobacterium tuberculosis antigen. Int. Immunopharmacol. 10, 694–706. doi: 10.1016/j.intimp.2010.03.014
He, C., Wang, H., Yu, C., Peng, C., Shu, X., Liao, W., et al. (2021). Alterations of gut microbiota in patients with intestinal tuberculosis that different from crohn’s disease. Front. Bioeng Biotechnol. 9, 673691. doi: 10.3389/fbioe.2021.673691
Hodgkinson, K., El Abbar, F., Dobranowski, P., Manoogian, J., Butcher, J., Figeys, D., et al. (2023). Butyrate’s role in human health and the current progress towards its clinical application to treat gastrointestinal disease. Clin. Nutr. 42, 61–75. doi: 10.1016/j.clnu.2022.10.024
Hu, Y., Cheng, M., Liu, B., Dong, J., Sun, L., Yang, J., et al. (2020). Metagenomic analysis of the lung microbiome in pulmonary tuberculosis - a pilot study. Emerg. Microbes Infect. 9(1), 1444–1452. doi: 10.1080/22221751.2020.1783188
Hu, Y., Feng, Y., Wu, J., Liu, F., Zhang, Z., Hao, Y., et al. (2019a). The gut microbiome signatures discriminate healthy from pulmonary tuberculosis patients. Front. Cell Infect. Microbiol. 9, 90. doi: 10.3389/fcimb.2019.00090
Hu, Y., Yang, Q., Liu, B., Dong, J., Sun, L., Zhu, Y., et al. (2019b). Gut microbiota associated with pulmonary tuberculosis and dysbiosis caused by anti-tuberculosis drugs. J. Infect. 78, 317–322. doi: 10.1016/j.jinf.2018.08.006
Huang, S. F., Yang, Y. Y., Chou, K. T., Fung, C. P., Wang, F. D., Su, W. J. (2019). Systemic proinflammation after mycobacterium tuberculosis infection was correlated to the gut microbiome in HIV-uninfected humans. Eur. J. Clin. Invest. 49(5):e13068. doi: 10.1111/eci.13068
Jacobson, A., Lam, L., Rajendram, M., Tamburini, F., Honeycutt, J., Pham, T., et al. (2018). A gut commensal-produced metabolite mediates colonization resistance to salmonella infection. Cell Host Microbe 24, 296–307.e297. doi: 10.1016/j.chom.2018.07.002
Jayasooriya, S., Dimambro-Denson, F., Beecroft, C., Balen, J., Awokola, B., Mitchell, C., et al. (2022). Patients with presumed tuberculosis in sub-Saharan Africa that are not diagnosed with tuberculosis: a systematic review and meta-analysis. Thorax. 78(1), 50–60. doi: 10.1136/thoraxjnl-2021-217663
Johnson, J. L., Jones, M. B., Cobb, B. A. (2015). Bacterial capsular polysaccharide prevents the onset of asthma through T-cell activation. Glycobiology 25, 368–375. doi: 10.1093/glycob/cwu117
Johnson, J. L., Jones, M. B., Cobb, B. A. (2018). Polysaccharide-experienced effector T cells induce IL-10 in FoxP3+ regulatory T cells to prevent pulmonary inflammation. Glycobiology 28, 50–58. doi: 10.1093/glycob/cwx093
Kempski, J., Brockmann, L., Gagliani, N., Huber, S. (2017). TH17 cell and epithelial cell crosstalk during inflammatory bowel disease and carcinogenesis. Front. Immunol. 8. doi: 10.3389/fimmu.2017.01373
Khan, N., Mendonca, L., Dhariwal, A., Fontes, G., Menzies, D., Xia, J., et al. (2019). Intestinal dysbiosis compromises alveolar macrophage immunity to mycobacterium tuberculosis. Mucosal Immunol. 12, 772–783. doi: 10.1038/s41385-019-0147-3
König, J., Wells, J., Cani, P. D., García-Ródenas, C. L., Macdonald, T., Mercenier, A., et al. (2016). Human intestinal barrier function in health and disease. Clin. Transl. Gastroenterol. 7, e196. doi: 10.1038/ctg.2016.54
Kwok, S. K., Cho, M. L., Her, Y. M., Oh, H. J., Park, M. K., Lee, S. Y., et al. (2012). TLR2 ligation induces the production of IL-23/IL-17 via IL-6, STAT3 and NF-kB pathway in patients with primary sjogren’s syndrome. Arthritis Res. Ther. 14, R64. doi: 10.1186/ar3780
Larsen, J. M. (2017). The immune response to prevotella bacteria in chronic inflammatory disease. Immunology 151, 363–374. doi: 10.1111/imm.12760
Li, W., Zhu, Y., Liao, Q., Wang, Z., Wan, C. (2019). Characterization of gut microbiota in children with pulmonary tuberculosis. BMC Pediatr. 19, 445. doi: 10.1186/s12887-019-1782-2
Lin, P. L., Flynn, J. L. (2015). CD8 T cells and mycobacterium tuberculosis infection. Semin. Immunopathol. 37, 239–249. doi: 10.1007/s00281-015-0490-8
Liu, H., Wang, J., He, T., Becker, S., Zhang, G., Li, D., et al. (2018). Butyrate: A double-edged sword for health? Adv. Nutr. 9, 21–29. doi: 10.1093/advances/nmx009
Liu, Y., Wang, J., Wu, C. (2021). Microbiota and tuberculosis: A potential role of probiotics, and postbiotics. Front. Nutr. 8, 626254. doi: 10.3389/fnut.2021.626254
Louis, P., Flint, H. J. (2017). Formation of propionate and butyrate by the human colonic microbiota. Environ. Microbiol. 19, 29–41. doi: 10.1111/1462-2920.13589
Lu, Y. J., Barreira-Silva, P., Boyce, S., Powers, J., Cavallo, K., Behar, S. M. (2021). CD4 T cell help prevents CD8 T cell exhaustion and promotes control of mycobacterium tuberculosis infection. Cell Rep. 36, 109696. doi: 10.1016/j.celrep.2021.109696
Luo, M., Liu, Y., Wu, P., Luo, D. X., Sun, Q., Zheng, H., et al. (2017). Alternation of gut microbiota in patients with pulmonary tuberculosis. Front. Physiol. 8, 822. doi: 10.3389/fphys.2017.00822
Machado, M. G., Sencio, V., Trottein, F. (2021). Short-chain fatty acids as a potential treatment for infections: a closer look at the lungs. Infect. Immun. 89, e0018821. doi: 10.1128/IAI.00188-21
Maji, A., Misra, R., Dhakan, D. B., Gupta, V., Mahato, N. K., Saxena, R., et al. (2018). Gut microbiome contributes to impairment of immunity in pulmonary tuberculosis patients by alteration of butyrate and propionate producers. Environ. Microbiol. 20, 402–419. doi: 10.1111/1462-2920.14015
Majlessi, L., Sayes, F., Bureau, J. F., Pawlik, A., Michel, V., Jouvion, G., et al. (2017). Colonization with helicobacter is concomitant with modified gut microbiota and drastic failure of the immune control of mycobacterium tuberculosis. Mucosal Immunol. 10, 1178–1189. doi: 10.1038/mi.2016.140
Maulahela, H., Simadibrata, M., Nelwan, E. J., Rahadiani, N., Renesteen, E., Suwarti, S. W. T., et al. (2022). Recent advances in the diagnosis of intestinal tuberculosis. BMC Gastroenterol. 22, 89. doi: 10.1186/s12876-022-02171-7
Mily, A., Rekha, R. S., Kamal, S. M., Akhtar, E., Sarker, P., Rahim, Z., et al. (2013). Oral intake of phenylbutyrate with or without vitamin D3 upregulates the cathelicidin LL-37 in human macrophages: a dose finding study for treatment of tuberculosis. BMC Pulm Med. 13, 23. doi: 10.1186/1471-2466-13-23
Mily, A., Rekha, R. S., Kamal, S. M., Arifuzzaman, A. S., Rahim, Z., Khan, L., et al. (2015). Significant effects of oral phenylbutyrate and vitamin D3 adjunctive therapy in pulmonary tuberculosis: A randomized controlled trial. PloS One 10, e0138340. doi: 10.1371/journal.pone.0138340
Mnyambwa, N. P., Philbert, D., Kimaro, G., Wandiga, S., Kirenga, B., Mmbaga, B. T., et al. (2021). Gaps related to screening and diagnosis of tuberculosis in care cascade in selected health facilities in East Africa countries: A retrospective study. J. Clin. Tuberculosis Other Mycobacterial Dis. 25, 100278. doi: 10.1016/j.jctube.2021.100278
Moideen, K., Kumar, N. P., Nair, D., Banurekha, V. V., Bethunaickan, R., Babu, S. (2018). Heightened systemic levels of neutrophil and eosinophil granular proteins in pulmonary tuberculosis and reversal following treatment. Infect. Immun. 86(6), e00008–18. doi: 10.1128/IAI.00008-18
Moreira-Teixeira, L., Stimpson, P. J., Stavropoulos, E., Hadebe, S., Chakravarty, P., Ioannou, M., et al. (2020). Type I IFN exacerbates disease in tuberculosis-susceptible mice by inducing neutrophil-mediated lung inflammation and NETosis. Nat. Commun. 11, 5566. doi: 10.1038/s41467-020-19412-6
Naidoo, C. C., Nyawo, G. R., Sulaiman, I., Wu, B. G., Turner, C. T., Bu, K., et al. (2021). Anaerobe-enriched gut microbiota predicts pro-inflammatory responses in pulmonary tuberculosis. EBioMedicine 67, 103374. doi: 10.1016/j.ebiom.2021.103374
Namasivayam, S., Diarra, B., Diabate, S., Sarro, Y. D. S., Kone, A., Kone, B., et al. (2020). Patients infected with mycobacterium africanum versus mycobacterium tuberculosis possess distinct intestinal microbiota. PloS Negl. Trop. Dis. 14, e0008230. doi: 10.1371/journal.pntd.0008230
Namasivayam, S., Maiga, M., Yuan, W., Thovarai, V., Costa, D. L., Mittereder, L. R., et al. (2017). Longitudinal profiling reveals a persistent intestinal dysbiosis triggered by conventional anti-tuberculosis therapy. Microbiome 5, 71. doi: 10.1186/s40168-017-0286-2
Nastasi, C., Fredholm, S., Willerslev-Olsen, A., Hansen, M., Bonefeld, C. M., Geisler, C., et al. (2017). Butyrate and propionate inhibit antigen-specific CD8+ T cell activation by suppressing IL-12 production by antigen-presenting cells. Sci. Rep. 7, 14516. doi: 10.1038/s41598-017-15099-w
Negatu, D. A., Yamada, Y., Xi, Y., Go, M. L., Zimmerman, M., Ganapathy, U., et al. (2019). Gut microbiota metabolite indole propionic acid targets tryptophan biosynthesis in Mycobacterium tuberculosis. mBio 10, e02781–e02718. doi: 10.1128/mBio.02781-18
Negi, S., Pahari, S., Bashir, H., Agrewala, J. N. (2020). Intestinal microbiota disruption limits the isoniazid mediated clearance of mycobacterium tuberculosis in mice. Eur. J. Immunol. 50, 1976–1987. doi: 10.1002/eji.202048556
Ormsby, M. J., Johnson, S. A., Carpena, N., Meikle, L. M., Goldstone, R. J., Mcintosh, A., et al. (2020). Propionic acid promotes the virulent phenotype of crohn’s disease-associated adherent-invasive escherichia coli. Cell Rep. 30, 2297–2305.e2295. doi: 10.1016/j.celrep.2020.01.078
Osei Sekyere, J., Maningi, N. E., Fourie, P. B. (2020). Mycobacterium tuberculosis, antimicrobials, immunity, and lung-gut microbiota crosstalk: current updates and emerging advances. Ann. N Y Acad. Sci. 1467, 21–47. doi: 10.1111/nyas.14300
Parada Venegas, D, De la Fuente, MK, Landskron, G, González, MJ, Quera, R, Dijkstra, G, et al. Short Chain Fatty Acids (SCFAs)-Mediated Gut Epithelial and Immune Regulation and Its Relevance for Inflammatory Bowel Diseases. Front Immunol (2019) 10:277. doi: 10.3389/fimmu.2019.00277. Erratum in: Front Immunol. 2019 Jun 28;10:1486.
Piccinni, M. P., Lombardelli, L., Logiodice, F., Kullolli, O., Maggi, E., Barkley, M. S. (2019). Medroxyprogesterone acetate decreases Th1, Th17, and increases Th22 responses via AHR signaling which could affect susceptibility to infections and inflammatory disease. Front. Immunol. 10, 642. doi: 10.3389/fimmu.2019.00642
Piccioni, A., Rosa, F., Manca, F., Pignataro, G., Zanza, C., Savioli, G., et al. (2022). Gut microbiota and clostridium difficile: What we know and the new frontiers. Int. J. Mol. Sci. 23, 13323. doi: 10.3390/ijms232113323
Pugazhendhi, S., Jayakanthan, K., Pulimood, A. B., Ramakrishna, B. S. (2013). Cytokine gene expression in intestinal tuberculosis and crohn’s disease. Int. J. Tuberc Lung Dis. 17, 662–668. doi: 10.5588/ijtld.12.0600
Pujic, P., Beaman, B. L., Ravalison, M., Boiron, P., Rodríguez-Nava, V. (2015). “Chapter 40 - nocardia and actinomyces,” in Molecular medical microbiology, 2nd ed. Eds. Tang, Y.-W., Sussman, M., Liu, D., Poxton, I., Schwartzman, J. (Boston: Academic Press), 731–752.
Rahim, M. A., Seo, H., Kim, S., Tajdozian, H., Barman, I., Lee, Y., et al. (2022). In vitro anti-tuberculosis effect of probiotic lacticaseibacillus rhamnosus PMC203 isolated from vaginal microbiota. Sci. Rep. 12, 8290. doi: 10.1038/s41598-022-12413-z
Rekha, R. S., Mily, A., Sultana, T., Haq, A., Ahmed, S., Mostafa Kamal, S. M., et al. (2018). Immune responses in the treatment of drug-sensitive pulmonary tuberculosis with phenylbutyrate and vitamin D3 as host directed therapy. BMC Infect. Dis. 18, 303. doi: 10.1186/s12879-018-3203-9
Rekha, R. S., Rao Muvva, S. S., Wan, M., Raqib, R., Bergman, P., Brighenti, S., et al. (2015). Phenylbutyrate induces LL-37-dependent autophagy and intracellular killing of mycobacterium tuberculosis in human macrophages. Autophagy 11, 1688–1699. doi: 10.1080/15548627.2015.1075110
Ren, C., Zhang, Q., De Haan, B. J., Faas, M. M., Zhang, H., De Vos, P. (2020). Protective effects of lactic acid bacteria on gut epithelial barrier dysfunction are toll like receptor 2 and protein kinase c dependent. Food Funct. 11, 1230–1234. doi: 10.1039/C9FO02933H
Rinninella, E., Raoul, P., Cintoni, M., Franceschi, F., Miggiano, G., Gasbarrini, A., et al. (2019). What is the healthy gut microbiota composition? a changing ecosystem across age, environment, diet, and diseases. Microorganisms 7, 14. doi: 10.3390/microorganisms7010014
Roca, F. J., Whitworth, L. J., Redmond, S., Jones, A. A., Ramakrishnan, L. (2019). TNF induces pathogenic programmed macrophage necrosis in tuberculosis through a mitochondrial-Lysosomal-Endoplasmic reticulum circuit. Cell 178, 1344–1361.e1311. doi: 10.1016/j.cell.2019.08.004
Sánchez-Tapia, M., Tovar, A. R., Torres, N. (2019). Diet as regulator of gut microbiota and its role in health and disease. Arch. Med. Res. 50, 259–268. doi: 10.1016/j.arcmed.2019.09.004
Schulthess, J., Pandey, S., Capitani, M., Rue-Albrecht, K. C., Arnold, I., Franchini, F., et al. (2019). The short chain fatty acid butyrate imprints an antimicrobial program in macrophages. Immunity 50, 432–445.e437. doi: 10.1016/j.immuni.2018.12.018
Seiler, P., Aichele, P., Bandermann, S., Hauser, A. E., Lu, B., Gerard, N. P., et al. (2003). Early granuloma formation after aerosol mycobacterium tuberculosis infection is regulated by neutrophils via CXCR3-signaling chemokines. Eur. J. Immunol. 33, 2676–2686. doi: 10.1002/eji.200323956
Sharma, R., Saikia, U. N., Sharma, S., Verma, I. (2017). Activity of human beta defensin-1 and its motif against active and dormant mycobacterium tuberculosis. Appl. Microbiol. Biotechnol. 101, 7239–7248. doi: 10.1007/s00253-017-8466-3
Sheer, T. A., Coyle, W. J. (2003). Gastrointestinal tuberculosis. Curr. Gastroenterol. Rep. 5, 273–278. doi: 10.1007/s11894-003-0063-1
Shen, H., Chen, Z. W. (2018). The crucial roles of Th17-related cytokines/signal pathways in m. tuberculosis infection. Cell. Mol. Immunol. 15, 216–225. doi: 10.1038/cmi.2017.128
Shi, W., Hu, Y., Ning, Z., Xia, F., Wu, M., Hu, Y. O. O., et al. (2021). Alterations of gut microbiota in patients with active pulmonary tuberculosis in China: a pilot study. Int. J. Infect. Dis. 111, 313–321. doi: 10.1016/j.ijid.2021.08.064
Sorbara, MT, Littmann, ER, Fontana, E, Moody, TU, Kohout, CE, Gjonbalaj, M, et al. Functional and Genomic Variation between Human-Derived Isolates of Lachnospiraceae Reveals Inter- and Intra-Species Diversity. Cell Host Microbe (2020) 28(1):134–146.e4. doi: 10.1016/j.chom.2020.05.005
Steinmann, J., Halldórsson, S., Agerberth, B., Gudmundsson, G. H. (2009). Phenylbutyrate induces antimicrobial peptide expression. Antimicrob. Agents Chemother. 53, 5127–5133. doi: 10.1128/AAC.00818-09
Stojanov, S., Berlec, A., Štrukelj, B. (2020). The influence of probiotics on the Firmicutes/Bacteroidetes ratio in the treatment of obesity and inflammatory bowel disease. Microorganisms 8, 1715. doi: 10.3390/microorganisms8111715
Stutz, M. D., Ojaimi, S., Allison, C., Preston, S., Arandjelovic, P., Hildebrand, J. M., et al. (2018). Necroptotic signaling is primed in mycobacterium tuberculosis-infected macrophages, but its pathophysiological consequence in disease is restricted. Cell Death Differentiation 25, 951–965. doi: 10.1038/s41418-017-0031-1
Tamanai-Shacoori, Z., Le Gall-David, S., Moussouni, F., Sweidan, A., Polard, E., Bousarghin, L., et al. (2022). SARS-CoV-2 and prevotella spp.: friend or foe? a systematic literature review. J. Med. Microbiol. 71(5). doi: 10.1099/jmm.0.001520
Tan, Z. M., Lai, G. P., Pandey, M., Srichana, T., Pichika, M. R., Gorain, B., et al. (2020). Novel approaches for the treatment of pulmonary tuberculosis. Pharmaceutics 12(12), 1196. doi: 10.3390/pharmaceutics12121196
Torrado, E., Cooper, A. M. (2010). IL-17 and Th17 cells in tuberculosis. Cytokine Growth Factor Rev. 21, 455–462. doi: 10.1016/j.cytogfr.2010.10.004
Vancamelbeke, M., Vermeire, S. (2017). The intestinal barrier: a fundamental role in health and disease. Expert Rev. Gastroenterol. Hepatol. 11, 821–834. doi: 10.1080/17474124.2017.1343143
Vandal, O. H., Nathan, C. F., Ehrt, S. (2009). Acid resistance in mycobacterium tuberculosis. J. Bacteriol 191, 4714–4721. doi: 10.1128/JB.00305-09
Vázquez-Pérez, J. A., Carrillo, C. O., Iñiguez-García, M. A., Romero-Espinoza, I., Márquez-García, J. E., Falcón, L. I., et al. (2020). Alveolar microbiota profile in patients with human pulmonary tuberculosis and interstitial pneumonia. Microb. Pathog. 139, 103851. doi: 10.1016/j.micpath.2019.103851
Wang, Y., Deng, Y., Liu, N., Chen, Y., Jiang, Y., Teng, Z., et al. (2022). Alterations in the gut microbiome of individuals with tuberculosis of different disease states. Front. Cell Infect. Microbiol. 12, 836987. doi: 10.3389/fcimb.2022.836987
Wang, S., Yang, L., Hu, H., Lv, L., Ji, Z., Zhao, Y., et al. (2022). Characteristic gut microbiota and metabolic changes in patients with pulmonary tuberculosis. Microb. Biotechnol. 15, 262–275. doi: 10.1111/1751-7915.13761
Wang, B., Yao, M., Lv, L., Ling, Z., Li, L. (2017). The human microbiota in health and disease. Engineering 3, 71–82. doi: 10.1016/J.ENG.2017.01.008
WHO (2021). Global tuberculosis report 2021. World Health Organization. https://www.who.int/publications/i/item/9789240037021
Winglee, K., Eloe-Fadrosh, E., Gupta, S., Guo, H., Fraser, C., Bishai, W. (2014). Aerosol mycobacterium tuberculosis infection causes rapid loss of diversity in gut microbiota. PloS One 9, e97048. doi: 10.1371/journal.pone.0097048
Wipperman, M. F., Fitzgerald, D. W., Juste, M.a.J., Taur, Y., Namasivayam, S., et al. (2017). Antibiotic treatment for tuberculosis induces a profound dysbiosis of the microbiome that persists long after therapy is completed. Sci. Rep. 7, 10767. doi: 10.1038/s41598-017-10346-6
Wlodarska, M., Willing, B., Keeney, K. M., Menendez, A., Bergstrom, K. S., Gill, N., et al. (2011). Antibiotic treatment alters the colonic mucus layer and predisposes the host to exacerbated citrobacter rodentium-induced colitis. Infect. Immun. 79, 1536–1545. doi: 10.1128/IAI.01104-10
Xiao, G., Cai, Z., Guo, Q., Ye, T., Tang, Y., Guan, P., et al. (2022). Insights into the unique lung microbiota profile of pulmonary tuberculosis patients using metagenomic next-generation sequencing. Microbiol. Spectr. 10, e0190121. doi: 10.1128/spectrum.01901-21
Yang, F., Yang, Y., Chen, L., Zhang, Z., Liu, L., Zhang, C., et al. (2022). The gut microbiota mediates protective immunity against tuberculosis via modulation of lncRNA. Gut Microbes 14, 2029997. doi: 10.1080/19490976.2022.2029997
Ye, S., Wang, L., Li, S., Ding, Q., Wang, Y., Wan, X., et al. (2022). The correlation between dysfunctional intestinal flora and pathology feature of patients with pulmonary tuberculosis. Front. Cell Infect. Microbiol. 12, 1090889. doi: 10.3389/fcimb.2022.1090889
Yoon, H., Park, Y. S., Shin, C. M., Kim, N., Lee, D. H. (2022). Gut microbiome in probable intestinal tuberculosis and changes following anti-tuberculosis treatment. Yonsei Med. J. 63, 34–41. doi: 10.3349/ymj.2022.63.1.34
Zafar, H., Saier, M. H., Jr. (2021). Gut bacteroides species in health and disease. Gut Microbes 13, 1–20. doi: 10.1080/19490976.2020.1848158
Zhang, Z., Li, J., Zhang, M., Li, B., Pan, X., Dong, X., et al. (2022). GPR109a regulates phenotypic and functional alterations in macrophages and the progression of type 1 diabetes. Mol. Nutr. Food Res. 66, e2200300. doi: 10.1002/mnfr.202200300
Zhang, M., Shen, L., Zhou, X., Chen, H. (2022). The microbiota of human lung of pulmonary tuberculosis and the alteration caused by anti-tuberculosis drugs. Curr. Microbiol. 79, 321. doi: 10.1007/s00284-022-03019-9
Keywords: gut microbiome, Mycobacterium tuberculosis, Firmicutes, Bacteroidetes, short-chain fatty acids, tuberculosis
Citation: Yu Z, Shen X, Wang A, Hu C and Chen J (2023) The gut microbiome: A line of defense against tuberculosis development. Front. Cell. Infect. Microbiol. 13:1149679. doi: 10.3389/fcimb.2023.1149679
Received: 22 January 2023; Accepted: 29 March 2023;
Published: 18 April 2023.
Edited by:
Susanta Pahari, Texas Biomedical Research Institute, United StatesReviewed by:
Andreas Kupz, James Cook University, AustraliaShikha Negi, Cincinnati Children’s Hospital Medical Center, United States
Copyright © 2023 Yu, Shen, Wang, Hu and Chen. This is an open-access article distributed under the terms of the Creative Commons Attribution License (CC BY). The use, distribution or reproduction in other forums is permitted, provided the original author(s) and the copyright owner(s) are credited and that the original publication in this journal is cited, in accordance with accepted academic practice. No use, distribution or reproduction is permitted which does not comply with these terms.
*Correspondence: Jianyong Chen, Y2p5YWN5NjlAMTYzLmNvbQ==
†These authors have contributed equally to this work