- 1Laboratório de Bacteriologia, Instituto Butantan, São Paulo, Brazil
- 2Departamento de Microbiologia, Instituto de Ciências Biomédicas, Universidade de São Paulo, São Paulo, Brazil
- 3Laboratório de Imunobiologia de Transplantes, Departamento de Imunologia, Instituto de Ciências Biomédicas, Universidade de São Paulo, São Paulo, Brazil
- 4Disciplina de Nefrologia, Departamento de Medicina, Universidade Federal de São Paulo, São Paulo, Brazil
Lipids are a big family of molecules with a vast number of functions in the cell membranes, within the cytoplasm, and extracellularly. Lipid droplets (LDs) are the most common storage organelles and are present in almost every tissue type in the body. They also have structural functions serving as building blocks of cellular membranes and may be precursors of other molecules such as hormones, and lipoproteins, and as messengers in signal transduction. Fatty acids (FAs), such as sterol esters and triacylglycerols, are stored in LDs and are used in β-oxidation as fuel for tricarboxylic acid cycle (TCA) and adenosine triphosphate (ATP) generation. FA uptake and entrance in the cytoplasm are mediated by membrane receptors. After a cytoplasmic round of α- and β-oxidation, FAs are guided into the mitochondrial matrix by the L-carnitine shuttle system, where they are fully metabolized, and enter the TCA cycle. Pathogen infections may lead to impaired lipid metabolism, usage of membrane phospholipids, and LD accumulation in the cytoplasm of infected cells. Otherwise, bacterial pathogens may use lipid metabolism as a carbon source, thus altering the reactions and leading to cellular and organelles malfunctioning. This review aims to describe cellular lipid metabolism and alterations that occur upon infections.
1 Introduction
Lipids are usually used as energy-storage depots, structural building blocks, and as second messengers in signaling cascades. They accumulate in lipid-storage specialized tissues, such as the adipose one, but are present in almost every cell type due to their importance as energy and carbon skeleton sources, and as hormone precursors. Through β-oxidation, a process in which a series of enzymes oxidize long, medium, or short FAs, cells yield much more ATP than using glucose or glycogen as primary sources. High energy-demanding cells, such as tubular epithelial cells, colonocytes, and myocytes, often use this energy pathway as their main metabolic pathway. Perturbation on lipid metabolism and its enzymes, as well as dietary overload and obesity, lead to cytoplasmic lipid accumulation and, consequently, lipotoxicity (Kang et al., 2015; Adeva-Andany et al., 2019; Cockcroft, 2021).
In general, lipids are a very diverse group of molecules, but with one characteristic in common: insolubility in water. Storage lipids are often fats, and their derivatives are FAs. They are composed of hydrocarbons, with a carbon skeleton, and may be branched or not. Unsaturation is characterized by a double bond between two carbon atoms, in a cis or trans configuration. Oxidation of FAs is a very exergonic reaction, thus producing a lot of energy that is converted into ATP in the mitochondrion (Cockcroft, 2021).
FAs uptake is mediated by three main transporters, CD36 or fatty acid translocase (FAT), fatty acid binding protein (FABP), and fatty acid transport protein (FATP). After the entrance into the cell cytoplasm, very long- and long-chain FAs are initially α- and β-oxidized in peroxisomes, and their metabolites, together with medium-chain FAs enter mitochondria through the L-carnitine shuttle system to start a new round of β-oxidation. At the end, acetyl-CoA is generated and enters the TCA cycle (Mannaerts et al., 2000; Luiken et al., 2002; Jansen and Wanders, 2006; Storch and McDermott, 2009; Schwenk et al., 2010; Anderson and Stahl, 2013; Melton et al., 2013).
Phospholipids are the major components of biological membranes and are composed of two hydrophobic fatty acyl chains and one hydrophilic head group. The fatty acyl chains are responsible for biological and physical properties affecting all biological processes that occur in the inner and outer membrane leaflets. Alterations in the fatty acyl chains are orchestrated by reacylation and deacylation, known as the Land’s cycle (Van Meer et al., 2008; Wang and Tontonoz, 2019; Cockcroft, 2021). Perturbance of phospholipid content in the cell membrane may be involved in the pathogenesis of some diseases, including bacterial infections.
Intracellular pathogens have well-defined mechanisms to exploit the host’s cell lipid metabolism and use those molecules as an energy source for their metabolic processes. In extracellular pathogens, lipid metabolism impairment and metabolism exploitation are still not completely elucidated (Walpole et al., 2018; Libbing et al., 2019).
Besides lipids’ roles in disease pathogenesis, there is a crescent body of information outlining lipid droplets (LDs) as a hub that integrates metabolic and inflammatory responses to face infections (Monson et al., 2021b). In viral infections, LDs are used as the site of viral replication, since it is partially protected against the machinery of pathogen killing. They also use the lipid content from LDs to build up their capsids (Samsa et al., 2009; van der Meer-Janssen et al., 2010; Farías et al., 2022). Furthermore, foamy macrophages, a hallmark of tuberculous lesions, are generated after Mycobacterium species induction of LDs formation (Agarwal et al., 2021).
This review aims to describe lipid metabolism and exploitation of lipids, lipid metabolism, and cell membrane phospholipids by pathogens, as well as their consequences to cells and organisms.
2 Lipid metabolism
Lipids are molecules composed of a hydrocarbon chain, branched or not, with a carboxyl-terminal group. As mentioned above, unsaturated FAs have a double bond between two carbons in the cis or trans position. Saturated FAs have linear chains with no double bonds along them, that may be as small as those with two carbons (acetic acid) or as large as those with 26 carbons (cerotate) (Adeva-Andany et al., 2019).
Lipid metabolism is a very complex process that involves both anabolic and catabolic reactions. The process starts with FA degradation and absorption by the gastrointestinal tract and the interaction of lipids with the gut microbiome. As this particular subject is beyond the scope of this review, the interested reader may search for additional information, well provided by Schoeler and Caesar (2019), who extensively discuss this aspect of lipid metabolism. Here we will focus on intracellular lipid metabolism and its importance for maintenance of the cellular functioning.
CD36, also known as fatty acid translocase, is a receptor of the scavenger receptor family that mediates the uptake of FAs from the extra- to the intracellular milieu (Luiken et al., 2002; Pepino et al., 2014; Hou et al., 2015; Hua et al., 2015; Yang et al., 2017; Jay and Hamilton, 2018). It is an integral membrane protein, highly glycosylated, and present in erythrocytes, platelets, immune cells, adipocytes, enterocytes, and epithelial cells (Pepino et al., 2014). Although it has a well-described function as a FA transporter, CD36 is also involved in the recognition of other molecules, such as thrombospondin, collagen, Plasmodium-infected erythrocytes, and oxidized-LDL (Nicholson et al., 2001; Silverstein and Febbraio, 2010; Jay and Hamilton, 2018; Maréchal et al., 2018; Pombinho et al., 2018).
It is known that CD36 production is augmented during acute and chronic kidney diseases, which is associated with epithelial-to-mesenchymal transition (EMT) and inflammation in animal and cellular models of kidney injury (Hou et al., 2015; Hua et al., 2015; Yang et al., 2017). Endothelial cells are also prone to undergo endothelial-to-mesenchymal transition after infection or disturbance of cell functioning. In primary endothelial cells infected by Leptospira interrogans CD36 is overexpressed in the cell membrane, as evidenced by immunofluorescence microscopy (Sato and Coburn, 2017).
Treatment of bovine mammary epithelial cells with palmitate leads to CD36 gene overexpression, in a dose-dependent manner. Cells treated with as much as 600 µM of palmitate have CD36 gene expression augmented at almost 40-fold compared to those without any treatment, which highlights the importance of this receptor in lipid uptake, and how lipid intake may influence its concentration in the cell membrane (Qi et al., 2014).
Other receptors are involved in lipid uptake to the cytoplasm, such as fatty acid transporter proteins 1 to 6 (FATP 1-6) and fatty acids binding proteins (FABPs) (Melton et al., 2013). FATPs are also integral membranes with different roles, according to each type. Collectively they are described as membrane-bound proteins that act in the uptake of long-chain FAs and in the activation of FAs, as the first step of β-oxidation, i.e., acylation, of very long-chain FAs (DiRusso et al., 2005; Anderson and Stahl, 2013; Melton et al., 2013).
FABPs have a high affinity to long-chain FAs and bind both saturated and unsaturated FAs (Storch and McDermott, 2009). There are multiple FABPs functioning similarly, and the affinity to a given FA increases as hydrophobicity rises. There are 9 different FABP types described to date, known as FABP 1-9 (Ono, 2005; Li et al., 2020). Different isoforms are named after the main organ where they were described (Furuhashi, 2019). These proteins, also characterized as chaperones, exert functions in FA transport and in intracellular communication (Gorbenko et al., 2010).
Besides being internalized by receptors, endocytosis is also used as a means of transporting lipids into the cell cytoplasm. The most well-studied forms of general endocytosis are the ones mediated by clathrin and caveolin (Johannes et al., 2016; Kaksonen and Roux, 2018; Kostarnoy et al., 2022; Abdulova et al., 2023; Liu et al., 2023; Tang et al., 2023; Yu and Yoshimura, 2023). More recently, a new mechanism of lipid transport and uptake, named Mincle-mediated endocytosis (MiMe) was described. The Mincle receptor was first reported as a pattern recognition receptor (PRR) and plays a role in triggering inflammatory responses after the recognition of fungal and bacterial lipids. However, it also mediates lipid uptake, as the knockout of Mincle leads to a pronounced decrease in lipid internalization by brain vessel endothelial cells.
Free FAs, or albumin-bound FAs are present in the bloodstream and enter the cells by their transporters, as described above. After their entry into the cytoplasm, FAs are activated by the enzyme fatty acyl CoA synthetase that catalyzes the binding of an Acyl-CoA to each acetyl moiety. Very long-chain FAs must be shortened by peroxisomal α-oxidation before entering mitochondria (Adeva-Andany et al., 2019).
Carnitine palmitoyl transferase 1 (CPT1) then catalyzes the transfer of acylcarnitine to the inner mitochondrial membrane. This process occurs with the acyl groups being transferred from Coenzyme A (CoA) to L-carnitine, generating the acylcarnitine esters that are allowed to pass through the mitochondrial outer membrane. Carnitine acyl-carnitine translocase (CACT) transports the acyl-carnitine esters into the mitochondrial matrix in exchange for a free carnitine. Finally, Carnitine palmitoyl transferase 2 (CPT2) catalyzes fatty acyl-CoA esters import into the mitochondria, transferring the acyl groups from the acyl-carnitine esters, generating acyl-CoA esters (Adeva-Andany et al., 2019).
In the mitochondrial matrix, Acyl-CoA is cleaved from FA-Acyl-CoA by β-oxidation and enters the TCA cycle to generate ATP (Berndt et al., 2019; Gewin, 2021). The whole β-oxidation process is catalyzed by only four different enzymes, e.g., Acyl-CoA dehydrogenase, Enoyl-CoA hydratase, 3-L-hydroxyacyl-CoA dehydrogenase, and β-ketothiolase. After the four rounds, there is generation of an acetyl-CoA that enters the TCA pathway, and an Acyl-CoA ester, with two carbons less. These iterative rounds keep happening until total Acyl-CoA esters are consumed (Adeva-Andany et al., 2019).
Conversely, acetyl-CoAs that are produced in the mitochondria and are not used in TCA are exported to the cytosol, and back to the ER, where they are used in lipogenesis, contributing to the biosynthesis of neutral lipids and phospholipids (Wältermann et al., 2007; Turchetto-Zolet et al., 2011; Hsu et al., 2023) De novo lipid synthesis or lipid biogenesis is governed by the mTORC1 signaling pathway (Düvel et al., 2010; Li et al., 2010; Peterson et al., 2011; Owen et al., 2012; Han et al., 2015; Lee et al., 2017), which is responsible for lipogenic enzyme production by SREBP 1 and 2 induction or reduction of their inhibitors (Düvel et al., 2010; Li et al., 2010; Peterson et al., 2011; Owen et al., 2012; Han et al., 2015). Another important role of the mTORC pathway is mediated by SRKP2 that induces more efficient mRNA splicing of lipogenic enzymes, thus enhancing de novo lipid synthesis (Lee et al., 2017).
In this way, lipids are produced after the generation of Acetyl-CoA, in the TCA cycle, from either citrate or acetate by ATP citrate lyase (ACLY) or acyl-CoA synthetase short-chain (ACSS). Fatty acid synthesis then takes place by using Acetyl-CoA and Malonyl-CoA, catalyzed by Fatty Acid Synthetase. The addition of more acetyl-CoA will lead to the formation of a palmitate molecule which is then used to generate longer FAs by elongation processes, unsaturated FAs catalyzed by stearoyl-CoA desnaturase 1 (SCD1), and finally phospholipids and signaling lipids (Lee et al., 2017). Although produced in the mitochondria, the cytosolic acetyl-CoA pool is used by lipogenic enzymes for FA biosynthesis. In vitro, cells under glucose restriction use more acetyl-L-carnitine to generate acetyl-CoA and consequently, fatty acids. Therefore, L-carnitine not only participates in the L-carnitine shuttle but also represents a carbon source to cells under physiological or hypoglycemic environment (Hsu et al., 2023).
Other important mechanism involved in cell homeostasis and pathogen response is macroautophagy, or simply autophagy. During this process, cells engulf and destroy organelles to maintain proper functioning. The phagophore, a double-membrane vacuole, is responsible for engulfing the organelles and fusing them with lysosomes where hydrolysis occurs. The formation of the phagophore is mediated by membrane donation from healthy organelles, but also by de novo synthesis of FAs in the ER, catalyzed by long-chain Acyl-CoA synthetase (ACS), in particular by the enzyme Faa1 (Schütter et al., 2020).
Triacylglycerol, a glycerolipid, is used as storage and is composed of three FAs attached to the three hydroxyl groups of the glycerol backbone (Cockcroft, 2021). Cell membranes are composed of three main lipids: glycerophospholipids, sphingolipids, and sterols, such as cholesterol. Mammalian cells synthesize and yield phospholipids that are not present in prokaryotes, such as phosphatidylserine, phosphatidylcholine, phosphatidylinositol, phosphatidylethanolamine, and phosphatidylglycerol. The percentage of each molecule varies according to the cell type and environment (Wang and Tontonoz, 2019; Cockcroft, 2021).
Neutral lipids are synthesized in the ER mainly as triacylglycerol and are stored in LDs. The most important enzymes, that are rate limiting in the synthesis of triacylglycerol, are acyl-CoA diacylglycerol acyltransferase (DGAT) 1 and 2, responsible for catalyzing the acylation of sn-1,2-diacylglycerol, using acetyl-CoA as a substrate. Evolutionary studies have shown that these enzymes are conserved in all eukaryotes, despite molecular and genetic differences (Turchetto-Zolet et al., 2011). Inhibition of DGAT 1 and 2 leads to decreased LD formation, and to impaired viral replication and bacterial survival during infections (Monson et al., 2021a; Yuan et al., 2021; Kiarely Souza et al., 2022; Dias et al., 2023).
Membrane phospholipid synthesis occurs in the ER. Phosphatidic acid (PA) is used as the precursor of most of the other phospholipids in the host’s cells. A two-round acylation of glycerol-3-phosphate is mediated by the enzymes Glycerol-3-Phosphate Acyltransferase (GPAT) and Lyso-PA Acyltransferase (LPAAT), with PA as an end product. From PA, other phospholipids, such as phosphatidylcholine, phosphatidylethanolamine, and phosphatidylserine are produced. Phosphatidylglycerol and cardiolipin are synthesized in the mitochondria. After their synthesis, phospholipids are addressed to the cytoplasmic and other membranes in the cell and are integrated into them (Van Meer et al., 2008; Cockcroft, 2021).
3 Lipid droplets and their roles in physiology and pathology
LDs are important organelles for cellular functioning and are constituted by high hydrophobic neutral FAs, within an aqueous environment. LDs are present in almost every cell in the organism and are responsible for FAs and specific protein storage, besides taking part in other complex processes, such as viral replication (Walther and Farese, 2012).
A family of proteins named perilipin (PLINs) is involved in lipid uptake, coating, and addressing LDs inside cells (Frank et al., 2015; Najt et al., 2022). Perilipin 1 was the first perilipin described (Greenberg et al., 1991) and after its discovery, the study of LDs, their lipidomic and proteomics, and the role of LDs in health and disease have increased dramatically, expanding the LD biology field (Kobayashi et al., 2008; Kuerschner et al., 2008; García-Arcos et al., 2010; Turchetto-Zolet et al., 2011; Hsieh et al., 2012; Lin et al., 2014; Markgraf et al., 2014; Kamili et al., 2015; Li et al., 2015; Viktorova et al., 2018; Freyre et al., 2019; Guyard et al., 2022; Kim et al., 2022; Oluwadare et al., 2022).
Assembly of LDs in the cytoplasm is thought to occur in very close proximity to the ER, but the exact mechanism by which those organelles are formed is still not completely understood. It has been shown that ER, mitochondria, and LDs are in close contact during LD formation and that this contact is mediated by two proteins called oxysterol binding protein (OSBP)-related protein (ORP) 5 and ORP8 (Guyard et al., 2022). Besides those, PLIN2 is involved in FA uptake and in addressing it in the forming LD (Hsieh et al., 2012; Najt et al., 2022). Other protein that may be involved in the inflammatory response and LD formation is the cell death-inducing DFF45-like effector b (Cideb). Mice lacking this protein are more prone to developing severe DSS-induced colitis and even more severe DSS-induced colitis if they undergo a high-fat diet (Sun et al., 2017). After the formation of a LD, enlargement is achieved by the fusion of multiple droplets. Besides the phospholipid monolayer, that separates LDs from the aqueous environment of the cytoplasm, there are also specific proteins attached to or in close relation with the LD’s phospholipid monolayer (Walther and Farese, 2012).
Usage (lipolysis) or storage (lipogenesis) of lipid content from LDs are very strictly regulated. Insulin and glucagon participate in this regulation, as they are major regulators of metabolism (Walther and Farese, 2012). Within the cell, the mechanism of new LD formation is still under debate, but the production of triacylglycerols and sterol ester by enzymes localized in the ER and the budding of the nascent LD from the ER membrane is one of the proposed mechanisms. Otherwise, usage of lipid content from LDs is mediated by lipases, such as the Adipose Triacylglycerol Lipase (ATGL) that converts triacylglycerol to diglyceride, and the Hormone-sensitive Lipase (HSL) that breaks diglyceride into monoglyceride (Walther and Farese, 2012; Monson et al., 2021b). The involvement of other cellular mechanisms in lipid metabolism has been described, though yet not fully understood (Singh et al., 2009; Yan et al., 2018; Jarc and Petan, 2019).
Autophagy seems to control LD digestion and lipolysis. Inhibition of autophagy in hepatocytes by knocking down the Atg5 gene leads to an augmentation of the number and size of LDs when cells are treated with oleate overload compared to cells in resting conditions. Lipid accumulation in those cells is probably mediated by autophagy inhibition, leading to impaired lipolysis (Singh et al., 2009).
Interferons play a specific role in the generation of LDs, especially during viral infection. Interferon-stimulated genes (ISG) encode some important proteins that act as inhibitors of LD formation (Helbig et al., 2013; Peña Cárcamo et al., 2018; Bosch et al., 2020; An et al., 2022). One of those proteins is the Sterile Alpha Motif and Histidine-aspartate Domain containing protein 1 (SAMHD1). In an HCV infection model, cells that overexpress this protein had 50% less LDs compared to the control and, consequently, produced less virus. On the contrary, the knockdown of the SAMHD1 led to an increase in HCV core protein detection (An et al., 2022). Other ISG that influences LD formation is Viperin. In homeostatic conditions this protein is localized in the ER and is transported to LDs. Viperin also exerts an antiviral role, during infection by Junín mammarenovirus (Peña Cárcamo et al., 2018).
LDs are also the site of eicosanoid production since they are a reservoir of arachidonic acid (Moreira et al., 2009). In Caco2 cells, the presence of cyclooxygenase-2 (COX-2) and prostaglandin E2 (PGE) was described and correlated to inflammation during colon cancer (Accioly et al., 2008). In oleic acid-treated IEC-6 cells, the formation of new LDs overlaps the compartmentalization of cytosolic PLA2, with production and storage of PGE2 within the LDs (Moreira et al., 2009). In the HL-60-derived neutrophils, treatment with Porphyromonas gengivalis led to an increased number of LDs, with a concomitant increase in Perilipin 3 protein. Interestingly, there is also an increase in PGE2 production and release, which demonstrates that there is a relation between the production of LDs and its proteins, and the production and release of eicosanoids (Nose et al., 2013).
3.1 The role of LDs in viral infection
Viral infection is known to induce LD accumulation in the cell cytoplasm. Both Dengue and Zika viruses were shown to induce LD accumulation in vertebrate and invertebrate hosts. Hepatitis C, Herpes simplex-1, Influenza-A, and SARS-CoV-2 also induce LDs in human and mouse models. Viruses use LDs to evade the host´s innate immune system to replicate and form new viral particles (Barba et al., 1997; Samsa et al., 2009; Barletta et al., 2016; Episcopio et al., 2019; da Silva Gomes Dias et al., 2020; Monson et al., 2021a).
SARS-CoV-2, the agent of COVID-19, uses cells’ machinery to induce the production of triacylglycerol and, consequently, LD formation. Those are used by the virus to guarantee a high rate of virion replication. The virus nucleocapsid protein activates DGAT1 and 2 expression, and adipocyte differentiation-related protein (ADRP) is also overexpressed, leading to the formation of more LDs. DGAT inhibition by silencing RNA or pharmacologically, by xanthohumol, leads to lower yields of virus in vitro and a better response to infection in vivo, respectively (da Silva Gomes Dias et al., 2020; Yuan et al., 2021). Another viral protein that is involved in the accumulation of LDs during COVID-19 is ORF3a. The protein itself is capable of inducing LD accumulation by impeding autophagy flux in the cells. Mutations in the ORF3a protein, such as T223I and S171L abrogated induction of LDs and led to deficient replication and lower virus yield in vitro (Wang et al., 2023).
Flaviviruses, such as Zika virus and Dengue virus, are widely known to use cellular lipids to replicate and induce the host’s inflammatory response. Zika virus induces LD formation during the early stage of infection and depletes LD storage during late-stage infection. Inhibition of autophagy in Zika virus-infected MDCK cells leads to LD content reduction and, consequently, viral replication reduction. There is also evidence that DGAT-1 blockage in neural cells and in mice reduces viral replication by blocking LD formation in those cells (Ambroggio et al., 2021; Monson et al., 2021a; Dias et al., 2023; Stoyanova et al., 2023).
As mentioned above, viral infection leads to transient LD induction, and type I- and III-interferon (IFN) mRNA levels are also elevated during the early stages of infection. Both LD and IFN levels increase as early as 2 hours post-infection for LDs and 8 hours post-infection for IFN, remain elevated during the next 48 hours and return to baseline at 72 hours post-infection (Monson et al., 2021a).
3.2 Lipids and bacterial infection
Intracellular bacteria developed a wide range of mechanisms that allow them to invade and multiply inside the host’s cells, such as specialized phagocytes, or other cell types including epithelial or adipose cells. Those mechanisms include avoidance of lysosome fusion to phagosomes, and the ability of those pathogens to survive and grow inside phagocytic vacuoles. After entry into cells, those pathogens rely only on the host’s metabolism to obtain the energy necessary for survival and growth. Subversion of cell lipid metabolism, and the ability of the pathogen to use lipids from the hosts as building blocks and energy sources is one of the mechanisms that allow intracellular persistence (Gago et al., 2018; Walenna et al., 2018; Walpole et al., 2018; Libbing et al., 2019).
By exploiting the role of FABPs in controlling fat metabolism to obtain nutrients from host cells, intracellular microorganisms, such as Chlamydia pneumoniae, hijack FABP4 and induce fatty acid oxidation (FAO) to obtain ATP generated by the host cells, consequently reducing the number and size of LDs inside adipocytes and releasing high amounts of glycerol and FAs. Thus, the use of cellular FAO to fuel bacterial TCA allows replication, growth, and persistence inside the host’s cells (Walenna et al., 2018).
Salmonella Typhimurium, a facultative intracellular bacterium, induces LD biogenesis as early as 1-hour post-infection in macrophages. Differently, Escherichia coli and dead S. Typhimurium are not able to cause LD accumulation. This capacity to induce early LD accumulation is dependent on the type III secretion system and contributes to intracellular bacterial survival and proinflammatory response (Kiarely Souza et al., 2022).
The foamy macrophage is a hallmark of tuberculous lesions. Those are macrophages with extreme LD accumulation. Different from what was first described, IFN-γ induces LD formation in macrophages during Mycobacterium tuberculosis infection as a host response to infection, and, surprisingly, M. tuberculosis is not able to acquire lipids from IFN-γ induced LDs. Thus, they are not a lipid source for the bacteria inside macrophages (Knight et al., 2018). Although the use of lipids from the host’s LD may not seem indispensable for M. tuberculosis growth, in vitro infection of macrophages by the vaccine strain bacillus Calmette-Guérin induces LD formation by TLR2 and leads to PGE2 production and the presence of cyclooxygenase-2 inside the LDs (D’avila et al., 2006; Almeida et al., 2014). Given the participation of macrophages in the response to infection and the ability of M. tuberculosis to trigger the formation of LDs inside those cells, it has become clearer that LDs are not only used by pathogens as a carbon source but are also an innate immune response to infection (D’avila et al., 2006; Almeida et al., 2014; Kumar et al., 2020; Kiarely Souza et al., 2022).
Since extracellular bacteria do not invade cells during infection, they evolved to exploit and use cells’ lipid storages from different locations, but also from LDs (Plotkowski et al., 2008). A brief explanation of FA transport to mitochondria, and how pathogens may interfere with lipid metabolism and cell membrane phospholipids is depicted in Figure 1.
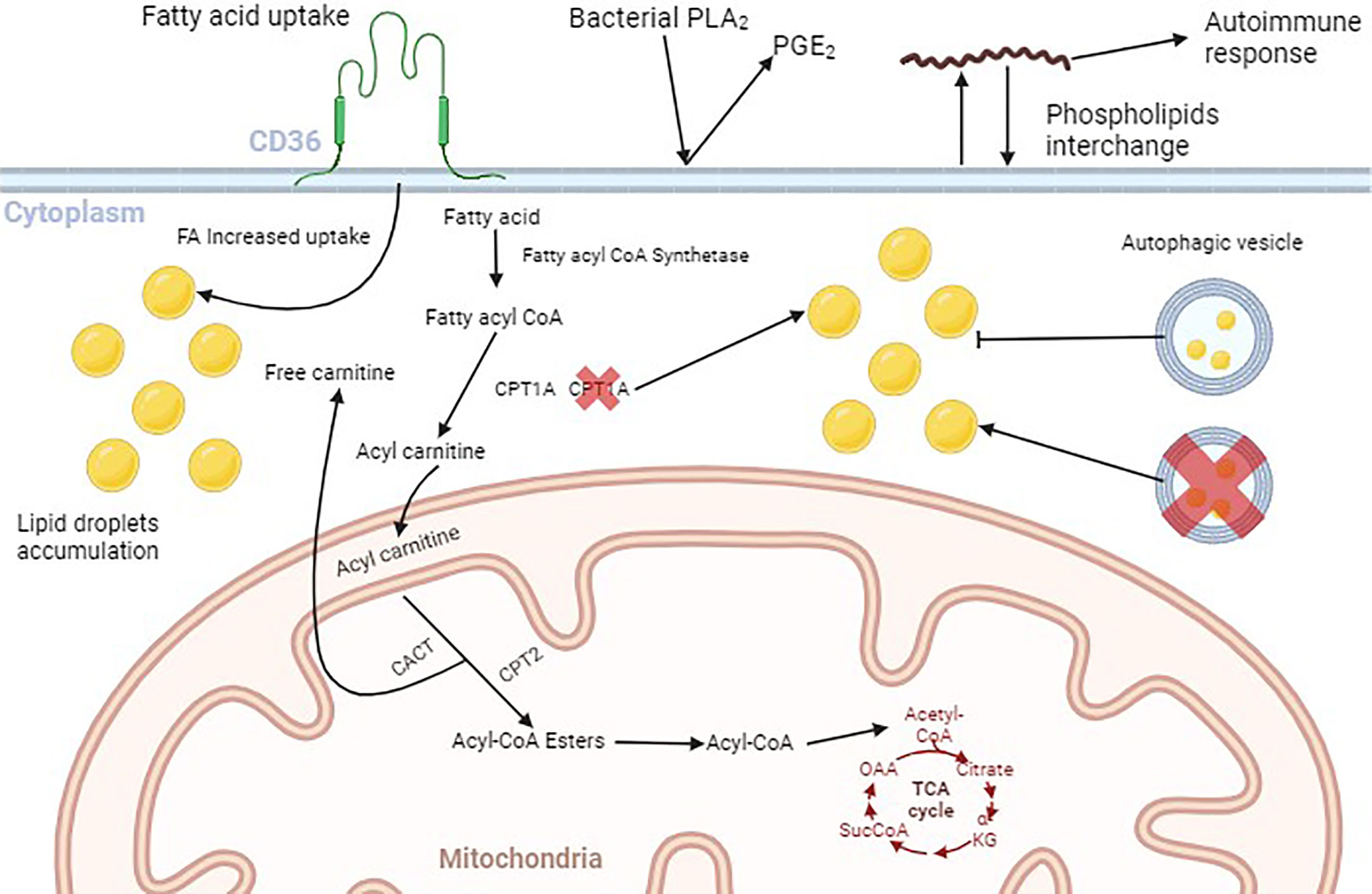
Figure 1 Schematic illustration of lipid fate within the cell. Fatty acids (FAs) bind to their membrane receptors and are translocated to the cytoplasm mediated by the FAs transporters (CD36, FABP, and FATP), where they are chemically modified. First, fatty acyl CoA synthetase adds an acyl CoA moiety to the FA. Then, Carnitine Palmitoyl Transferase 1A (CPT1A) converts the Fatty acyl CoA to Acyl carnitine and facilitates its passage through the mitochondrial outer membrane. Acyl carnitine enters the mitochondrial matrix in exchange for one free carnitine, mediated by Carnitine Acyl Carnitine Transferase (CACT). Carnitine Palmitoyl Transferase 2 (CPTA2) converts acylcarnitine to Acyl-CoA esters that are oxidized to Acyl-CoA that enters the Tricarboxylic Acid Cycle. The increase in the amount of membrane fatty acids receptors leads to the accumulation of cytoplasmic FAs, that are stored as lipid droplets (LDs). Inhibition of the autophagic flux is also responsible for LDs cytoplasmic accumulation. Extracellular pathogens such as P. aeruginosa produce enzymes like Phospholipase A2 (PLA2) that convert membrane phospholipids into Prostaglandin E2 (PGE2). Also, the spirochete B. burgdorferi can incorporate membrane phospholipids into their own membrane and then, reincorporate them into the cell membrane, leading to the production of antibodies underlying autoimmune responses. Figure generated by BioRender.com.
Extracellular bacteria do not invade cells to escape immune responses or to obtain carbon and energy sources. However, they can exploit lipids from cell membranes. There is a growing body of evidence suggesting that extracellular pathogens are able to use host’s membrane phospholipids as a source of FAs to assemble their cell membrane, and also degrade phospholipids leading to the production of proinflammatory cytokines (Libbing et al., 2019). Also, Helicobacter pylori can glycosylate and use the host’s cholesterol to grow and evade the immune system (Wunder et al., 2006). Although these data point to the ability of some bacteria to use hosts’ lipids, the direct use of LDs as well as their induction or inhibition, and their role in direct cell lesions are still being investigated (Wunder et al., 2006; Plotkowski et al., 2008; Dulberger et al., 2020).
Pseudomonas aeruginosa produces a phospholipase-like protease, ExoU, an effector of the type III secretion system that induces the production of eicosanoid-mediated proinflammatory cytokines in an endothelial cell line and in a murine model of infection (Saliba et al., 2005). The presence of ExoU in P. aeruginosa isolates is related to antimicrobial resistance and the occurrence of tissue necrosis (Sharma et al., 2014; Subedi et al., 2018; Springer et al., 2019; Bagayoko et al., 2021; Deruelle et al., 2021). Although related to bacterial virulence, the protease needs a host chaperone to bind to the cell membrane and to induce cell rupture and inflammation. The DNAJC5 chaperone may be one of the chaperones involved in the process since human DNAJC5-deficient cells and Drosophila flies with the DNAJC5 orthologue knocked down are resistant to the necrosis effects caused by ExoU protease (Deruelle et al., 2021). In vitro studies showed that ExoU may interact with phosphatidylinositol-containing lipids and cleave them. Since cell membranes are composed of phosphatidylinositol-containing lipids, their composition may target it to ExoU action, leading to cell membrane rupture and tissue necrosis (Springer et al., 2019; Deruelle et al., 2021).
Another important enzyme produced by P. aeruginosa and secreted by its type V secretion system is PlpD, which displays a phospholipase A1 activity and has a catalytic domain that is quite similar to ExoU. PlpD is also able to bind mainly to phosphatidylinositol, but also to phosphatidic acid, phosphatidylserine, and phosphatidylglycerol (Da Mata Madeira et al., 2016). Despite the well-described binding of PlpD to phospholipids, the exact role of this enzyme in phospholipids’ cleavage is not completely understood. In contrast, a direct activity against phospholipids has been described for a PlpD homologue from Fusobacterium nucleatum (Trunk et al., 2019).
Helicobacter pylori infection is associated with gastritis, gastric ulcer, and gastric cancer. As the pathogen does not have any machinery to produce cholesterol, it relies only on the host’s cholesterol which is incorporated onto the bacterial cell membrane. H. pylori can glycosylate host cholesterol before incorporating it, and differences in glycosylation are associated with immune escape. By α-glycosylating cholesterol with the consequent generation of cholesteryl-α-glucosides they maintain themselves attached to the gastric mucosa and subvert neutrophils and macrophages action, delaying phagocytosis (Wunder et al., 2006; Du et al., 2016; Hsu et al., 2021; Qaria et al., 2021).
It has been postulated that H. pylori also excretes a vacuolating toxin, named VacA, which targets lipid rafts and sphingomyelin (SM), and is part of a whole mechanism that allows the bacterium to survive in the epithelium surface and extract nutrients from host cells, especially lipids. The association of multimeric VacA is dependent on low pH and the complex binds to the membrane as 26 nm oligomers. In supported lipid bilayers in vitro, VacA binds to SM plus cholesterol lipid bilayers without a clear lipid domain preference, but it binds to raft-like domains in lipid bilayers made of dioleoylphosphatidylcholine, SM, and cholesterol. Although it targets the above-mentioned lipids, there is a clear preference of VacA for phosphatidylserine in supported lipid bilayers, given its avidly for this phophplipid (Pagliaccia et al., 2000; Geisse et al., 2004; Gupta et al., 2008; Sewald et al., 2008; Gupta et al., 2010; Kim and Blanke, 2012; Raghunathan et al., 2018).
The use of host’s cell membrane lipids by extracellular pathogens is also considered a virulence factor. Borrelia burgdorferi, the causative agent of Lyme disease, exchanges lipids from lipid rafts with HeLa cells, in vitro. After getting cholesterol from the cell membrane, those spirochetes metabolize and integrate it into their cell wall. On the other hand, as they are capable of transferring metabolized cholesterol from their cell wall to the cell membrane, they target the cell to immune system attack (Östberg et al., 2007; Crowley et al., 2013).
The production of IgG anti-mammalian membrane phospholipids has been proposed as a diagnostic tool to follow the efficiency of Lyme disease treatment. Mice produce anti-phosphatidylglycerol, phosphatidylcholine, phosphatidic acid, phosphatidylserine, and phosphatidylethanolamine after infection by Borrelia burgdorferi cultured in a medium that contains those phospholipids. In human sera, the same pattern of IgG production against phospholipids has been described. In brief, these spirochetes are capable of incorporating host phospholipids, and this mechanism is probably related to the occurrence of symptoms related to autoimmunity, thus important to the pathogenesis of the disease (Arora et al., 2022; Gwynne et al., 2022).
Periodontal pathogens, Porphyromonas gingivalis and Fusobacterium nucleatum are phagocytosed and induce the formation of LDs within murine and human macrophage cell lines, i.e., Raw264.7 and THP-1. The proposed mechanism by which those pathogens induce LD accumulation is by disruption of lipid homeostasis and modulation of proteins and enzymes related to cholesterol influx and efflux, but not with the increase of cholesterol esterification. Lipid receptor expression, such as CD36 and FABP4, are also increased during macrophage infection by both pathogens, which can explain the augmented intake of free FAs or oxidized-LDL. These alterations in lipid metabolism are closely related to the formation of foamy macrophages and the occurrence of atherosclerosis and cardiovascular accidents (Kim et al., 2019; Rho et al., 2021).
Increased expression of the FA transporter CD36 is also observed in primary endothelial cell lines after infection by pathogenic leptospires, L. interrogans (Sato and Coburn, 2017). Interestingly, hamsters infected by pathogenic leptospires have circulating foamy macrophages (Putz et al., 2021). The occurrence of foamy macrophages is well described in mycobacterial infection, due to the accumulation of LDs in the cytoplasm of those phagocytes (Agarwal et al., 2021; Laval et al., 2021). We can speculate that this increase may lead to augmented uptake of FAs by the cells, and consequently accumulation of neutral lipids in the cytoplasm. However, the mechanism by which bacteria enhance CD36 and other surface lipid receptor expression is still not completely understood.
The interaction of pathogens with surface receptors and lipid rafts is necessary for adhesion, but also facilitates entrance into the cell, in the case of intracellular pathogens (van der Meer-Janssen et al., 2010; Crowley et al., 2013; Vromman and Subtil, 2014; Du et al., 2016). The close contact between host cells and bacteria also facilitates the interchange of phospholipids, as in the case of B. burgdorferi and H. pylori (Wunder et al., 2006; Crowley et al., 2013; Du et al., 2016; Qaria et al., 2021; Arora et al., 2022; Gwynne et al., 2022).
4 Conclusions
Lipids and lipid metabolism are crucial for normal cell functioning, mainly in cells that rely only on β-oxidation as a source of ATP. Subversion of β-oxidation and of functioning of FAT, FABP, and FATP are used by pathogens to acquire free FAs as carbon sources, for energy, and production of other molecules.
Intracellular pathogens evolved mechanisms to exploit cell FA metabolism and membrane lipids to favor their growth and survival. The strategies to accomplish this fate are well reported in the literature, and there are plenty of studies describing the exact mechanism used by those pathogens.
Considering that extracellular bacteria lack the machinery for lipid metabolism and production of cholesterol, the exploitation of lipids from the host’s cell membrane is used as the source of lipids that will compose the bacterial membrane. A close interaction between host cells and bacteria is needed to allow membrane lipids to interchange, and this interaction is mediated by lipid rafts present in both host cell and bacterial membrane.
To date, only a few works have been published addressing the use of lipids and the exploitation of lipid metabolism by extracellular pathogens. Those scarce, but relevant studies, however, shed light on the role of lipids in the interaction between extracellular pathogens and host cells, and point to the need for more experimental studies to uncover the pathways underlying these processes.
Author contributions
LP wrote the manuscript and conceived the figure. NC and AB revised it critically for intellectual content. All authors contributed to the article and approved the submitted version.
Funding
We thank Fundação Butantan and Fundação de Amparo à Pesquisa do Estado de São Paulo (FAPESP) for the funding, grant # 2021/01122-9, and the Coordenação de Aperfeiçoamento de Pessoal de Nível Superior (CAPES) for the scholarship granted to the first author, PROEX 88887.357170/2019-00.
Conflict of interest
The authors declare that the research was conducted in the absence of any commercial or financial relationships that could be construed as a potential conflict of interest.
Publisher’s note
All claims expressed in this article are solely those of the authors and do not necessarily represent those of their affiliated organizations, or those of the publisher, the editors and the reviewers. Any product that may be evaluated in this article, or claim that may be made by its manufacturer, is not guaranteed or endorsed by the publisher.
References
Abdulova, A., Purelku, M., Sahin, H., Tanrıverdi, G. (2023). Human ovarian granulosa cells use clathrin-mediated endocytosis for LDL uptake: immunocytochemical and electron microscopic study. Ultrastruct Pathol. 47 (4), 241–252. doi: 10.1080/01913123.2023.2200532
Accioly, M. T., Pacheco, P., Maya-Monteiro, C. M., Carrossini, N., Robbs, B. K., Oliveira, S. S., et al. (2008). Lipid bodies are reservoirs of cyclooxygenase-2 and sites of prostaglandin-E2 synthesis in colon cancer cells. Cancer Res. 68 (6), 1732–1740. doi: 10.1158/0008-5472.CAN-07-1999
Adeva-Andany, M. M., Carneiro-Freire, N., Seco-Filgueira, M., Fernández-Fernández, C., Mouriño-Bayolo, D. (2019). Mitochondrial β-oxidation of saturated fatty acids in humans. Mitochondrion 46, 73–90. doi: 10.1016/j.mito.2018.02.009
Agarwal, P., Gordon, S., Martinez, F. O. (2021). Foam cell macrophages in tuberculosis. Front. Immunol. 12 (775326). doi: 10.3389/fimmu.2021.775326
Almeida, P. E., Roque, N. R., Magalhães, K. G., Mattos, K. A., Teixeira, L., Maya-Monteiro, C., et al. (2014). Differential TLR2 downstream signaling regulates lipid metabolism and cytokine production triggered by Mycobacterium bovis BCG infection. Biochim. Biophys. Acta Mol. Cell Biol. Lipids 1841 (1), 97–107. doi: 10.1016/j.bbalip.2013.10.008
Ambroggio, E. E., Navarro, G. S. C., Socas, L. B. P., Bagatolli, L. A., Gamarnik, A. V. (2021). Dengue and Zika virus capsid proteins bind to membranes and self-assemble into liquid droplets with nucleic acids. J. Biol. Chem. 297 (3), 101059. doi: 10.1016/j.jbc.2021.101059
An, N., Ge, Q., Shao, H., Li, Q., Guo, F., Liang, C., et al. (2022). Interferon-inducible SAMHD1 restricts viral replication through downregulation of lipid synthesis. Front. Immunol. 13. doi: 10.3389/fimmu.2022.1007718
Anderson, C. M., Stahl, A. (2013). SLC27 fatty acid transport proteins. Mol. Aspects Med. 34 (2–3), 516–528. doi: 10.1016/j.mam.2012.07.010
Arora, G., Hart, T., Fikrig, E. (2022). Use of host lipids by the Lyme disease spirochete may lead to biomarkers. J. Clin. Invest. 132 (6), e158254. doi: 10.1172/JCI158254
Bagayoko, S., Leon-Icaza, S. A., Pinilla, M., Hessel, A., Santoni, K., Péricat, D., et al. (2021). Host phospholipid peroxidation fuels ExoU-dependent cell necrosis and supports Pseudomonas aeruginosa-driven pathology. PLoS Pathog. 17 (9), e1009927. doi: 10.1371/journal.ppat.1009927
Barba, G., Harper, F., Harada, T., Kohara, M., Goulinet, S., Matsuura, Y., et al. (1997). Hepatitis C virus core protein shows a cytoplasmic localization and associates to cellular lipid storage droplets. Proc. Natl. Acad. Sci. U.S.A. 94 (4), 1200–1205. doi: 10.1073/pnas.94.4.1200
Barletta, A. B. F., Alves, L. R., Nascimento Silva, M. C. L., Sim, S., Dimopoulos, G., Liechocki, S., et al. (2016). Emerging role of lipid droplets in Aedes aEgypti immune response against bacteria and Dengue virus. Sci. Rep. 6, 19928. doi: 10.1038/srep19928
Berndt, N., Eckstein, J., Heucke, N., Gajowski, R., Stockmann, M., Meierhofer, D., et al. (2019). Characterization of lipid and lipid droplet metabolism in human HCC. Cells 8 (5), 512. doi: 10.3390/cells8050512
Bosch, M., Sánchez-Álvarez, M., Fajardo, A., Kapetanovic, R., Steiner, B., Dutra, F., et al. (2020). Mammalian lipid droplets are innate immune hubs integrating cell metabolism and host defense. Science 370 (6514), eaay8085. doi: 10.1126/science.aay8085
Cockcroft, S. (2021). Mammalian lipids: structure, synthesis and function. Essays Biochem. 65, 813–845. doi: 10.1042/EBC20200067
Crowley, J. T., Toledo, A. M., Larocca, T. J., Coleman, J. L., London, E., Benach, J. L. (2013). Lipid exchange between Borrelia burgdorferi and host cells. PLoS Pathog. 9 (1), e1003109. doi: 10.1371/journal.ppat.1003109
Da Mata Madeira, P. V., Zouhir, S., Basso, P., Neves, D., Laubier, A., Salacha, R., et al. (2016). Structural basis of lipid targeting and destruction by the type V secretion system of Pseudomonas aeruginosa. J. Mol. Biol. 428 (9 Pt A), 1790–1803. doi: 10.1016/j.jmb.2016.03.012
da Silva Gomes Dias, S., Soares, V. C., Ferreira, A. C., Sacramento, C. Q., Fintelman-Rodrigues, N., Temerozo, J. R., et al. (2020). Lipid droplets fuel SARS-CoV-2 replication and production of inflammatory mediators. PLoS Pathog. 16 (12), e1009127. doi: 10.1101/2020.08.22.262733
D’avila, H., Melo, R. C. N., Parreira, G. G., Werneck-Barroso, E., Castro-Faria-Neto, H. C., Bozza, P. T. (2006). Mycobacterium bovis bacillus calmette-guérin induces TLR2-mediated formation of lipid bodies: intracellular domains for eicosanoid synthesis in vivo 1. J. Immunol. 176, 3087–3097. doi: 10.4049/jimmunol.176.5.3087
Deruelle, V., Bouillot, S., Job, V., Taillebourg, E., Fauvarque, M. O., Attrée, I., et al. (2021). The bacterial toxin ExoU requires a host trafficking chaperone for transportation and to induce necrosis. Nat. Commun. 12 (1), 4024. doi: 10.1038/s41467-021-24337-9
Dias, S. S. G., Cunha-Fernandes, T., Souza-Moreira, L., Soares, V. C., Lima, G. B., Azevedo-Quintanilha, I. G., et al. (2023). Metabolic reprogramming and lipid droplets are involved in Zika virus replication in neural cells. J. Neuroinflamm. 20 (1), 61. doi: 10.1186/s12974-023-02736-7
DiRusso, C. C., Li, H., Darwis, D., Watkins, P. A., Berger, J., Black, P. N. (2005). Comparative biochemical studies of the murine fatty acid transport proteins (FATP) expressed in yeast. J. Biol. Chem. 280 (17), 16829–16837. doi: 10.1074/jbc.M409598200
Du, S. Y., Wang, H. J., Cheng, H. H., Chen, S., Wang, L. H. C., Wang, W. C. (2016). Cholesterol glucosylation by Helicobacter pylori delays internalization and arrests phagosome maturation in macrophages. J. Microbiol. Immunol. Infect. 49 (5), 636–645. doi: 10.1016/j.jmii.2014.05.011
Dulberger, C. L., Rubin, E. J., Boutte, C. C. (2020). The mycobacterial cell envelope - a moving target. Nat. Rev. Microbiol. 18 (1), 47–59. doi: 10.1038/s41579-019-0273-7
Düvel, K., Yecies, J. L., Menon, S., Raman, P., Lipovsky, A. I., Souza, A. L., et al. (2010). Activation of a metabolic gene regulatory network downstream of mTOR complex 1. Mol. Cell 39 (2), 171–183. doi: 10.1016/j.molcel.2010.06.022
Episcopio, D., Aminov, S., Benjamin, S., Germain, G., Datan, E., Landazuri, J., et al. (2019). Atorvastatin restricts the ability of influenza virus to generate lipid droplets and severely suppresses the replication of the virus. FASEB J. 33 (8), 9516. doi: 10.1096/fj.201900428RR
Farías, M. A., Diethelm-Varela, B., Navarro, A. J., Kalergis, A. M., González, P. A. (2022). Interplay between lipid metabolism, lipid droplets, and DNA virus infections. Cells 11 (14), 2224. doi: 10.3390/cells11142224
Frank, D. N., Bales, E. S., Monks, J., Jackman, M. J., MacLean, P. S., Ir, D., et al. (2015). Perilipin-2 modulates lipid absorption and microbiome responses in the mouse intestine. PLoS One 10 (7), e0131944. doi: 10.1371/journal.pone.0131944
Freyre, C. A. C., Rauher, P. C., Ejsing, C. S., Klemm, R. W. (2019). MIGA2 Links Mitochondria, the ER, and lipid droplets and promotes de novo lipogenesis in adipocytes. Mol. Cell 76 (5), 811–825.e14. doi: 10.1016/j.molcel.2019.09.011
Furuhashi, M. (2019). Fatty acid-binding protein 4 in cardiovascular and metabolic diseases. J. Atheroscler Thromb. 26 (3), 216–232. doi: 10.5551/jat.48710
Gago, G., Diacovich, L., Gramajo, H. (2018). Lipid metabolism and its implication in mycobacteria–host interaction. Curr. Opin. Microbiol. 41, 36–42. doi: 10.1016/j.mib.2017.11.020
García-Arcos, I., González-Kother, P., Aspichueta, P., Rueda, Y., Ochoa, B., Fresnedo, O. (2010). Lipid analysis reveals quiescent and regenerating liver-specific populations of lipid droplets. Lipids 45 (12), 1101–1108. doi: 10.1007/s11745-010-3492-2
Geisse, N. A., Cover, T. L., Henderson, R. M., Edwardson, J. M. (2004). Targeting of Helicobacter pylori vacuolating toxin to lipid raft membrane domains analyzed by atomic force microscopy. Biochem. J. 381, 911–917. doi: 10.1042/BJ20031719
Gewin, L. S. (2021). Sugar or fat? Renal tubular metabolism reviewed in health and disease. Nutrients 13 (5), 1580. doi: 10.3390/nu13051580
Gorbenko, O., Panayotou, G., Zhyvoloup, A., Volkova, D., Gout, I., Filonenko, V. (2010). Identification of novel PTEN-binding partners: PTEN interaction with fatty acid binding protein FABP4. Mol. Cell Biochem. 337 (1–2), 299–305. doi: 10.1007/s11010-009-0312-1
Greenberg, A. S., Egan, J. J., Wek, S. A., Garty, N. B., Joan Blanchette-Mackieej, E., Londos, C. (1991). Perilipin, a major hormonally regulated adipocyte-specific phosphoprotein associated with the periphery of lipid storage droplets*. J. Of Biol. Chem. 266 (17), 11341–11346. doi: 10.1016/S0021-9258(18)99168-4
Gupta, V. R., Patel, H. K., Kostolansky, S. S., Ballivian, R. A., Eichberg, J., Blanke, S. R. (2008). Sphingomyelin functions as a novel receptor for Helicobacter pylori VacA. PloS Pathog. 4 (5), e1000073. doi: 10.1371/journal.ppat.1000073
Gupta, V. R., Wilson, B. A., Blanke, S. R. (2010). Sphingomyelin is important for the cellular entry and intracellular localization of Helicobacter pylori VacA. Cell Microbiol. 12 (10), 1517–1533. doi: 10.1111/j.1462-5822.2010.01487.x
Guyard, V., Monteiro-Cardoso, V. F., Omrane, M., Sauvanet, C., Houcine, A., Boulogne, C., et al. (2022). ORP5 and ORP8 orchestrate lipid droplet biogenesis and maintenance at ER–mitochondria contact sites. J. Cell Biol. 221 (9), e202112107. doi: 10.1083/jcb.202112107
Gwynne, P. J., Clendenen, L. H., Turk, S. P., Marques, A. R., Hu, L. T. (2022). Antiphospholipid autoantibodies in Lyme disease arise after scavenging of host phospholipids by Borrelia burgdorferi. J. Clin. Invest. 132 (6), e152506. doi: 10.1172/JCI152506
Han, J., Li, E., Chen, L., Zhang, Y., Wei, F., Liu, J., et al. (2015). The CREB coactivator CRTC2 controls hepatic lipid metabolism by regulating SREBP1. Nature 524 (7564), 243–246. doi: 10.1038/nature14557
Helbig, K. J., Carr, J. M., Calvert, J. K., Wati, S., Clarke, J. N., Eyre, N. S., et al. (2013). Viperin is induced following dengue virus type-2 (DENV-2) infection and has anti-viral actions requiring the C-terminal end of viperin. PLoS Negl. Trop. Dis. 7 (4), e2178. doi: 10.1371/journal.pntd.0002178
Hou, Y., Wu, M., Wei, J., Ren, Y., Du, C., Wu, H., et al. (2015). CD36 is involved in high glucose-induced epithelial to mesenchymal transition in renal tubular epithelial cells. Biochem. Biophys. Res. Commun. 468 (1–2), 281–286. doi: 10.1016/j.bbrc.2015.10.112
Hsieh, K., Lee, Y. K., Londos, C., Raaka, B. M., Dalen, K. T., Kimmel, A. R. (2012). Perilipin family members preferentially sequester to either triacylglycerol-specific or cholesteryl-esterspecific intracellular lipid storage droplets. J. Cell Sci. 125 (17), 4067–4076. doi: 10.1242/jcs.104943
Hsu, J., Fatuzzo, N., Weng, N., Michno, W., Dong, W., Kienle, M., et al. (2023). Carnitine octanoyltransferase is important for the assimilation of exogenous acetyl-L-carnitine into acetyl-CoA in mammalian cells. J. Biol. Chem. 299 (2), 102848. doi: 10.1016/j.jbc.2022.102848
Hsu, C. Y., Yeh, J. Y., Chen, C. Y., Wu, H. Y., Chiang, M. H., Wu, C. L., et al. (2021). Helicobacter pylori cholesterol-α-glucosyltransferase manipulates cholesterol for bacterial adherence to gastric epithelial cells. Virulence 12 (1), 2341–2351. doi: 10.1080/21505594.2021.1969171
Hua, W., Huang, H. Z., Tan, L. T., Wan, J. M., Gui, H. B., Zhao, L., et al. (2015). CD36 mediated fatty acid-induced podocyte apoptosis via oxidative stress. PLoS One 10 (5), e0127507. doi: 10.1371/journal.pone.0127507
Jansen, G. A., Wanders, R. J. A. (2006). Alpha-oxidation. Biochim. Biophys. Acta Mol. Cell Res. 1763 (12), 1403–1412. doi: 10.1016/j.bbamcr.2006.07.012
Jarc, E., Petan, T. (2019). Lipid droplets and the management of cellular stress. Yale J. Biol. Med. 92 (3), 435–452.
Jay, A. G., Hamilton, J. A. (2018). The enigmatic membrane fatty acid transporter CD36: New insights into fatty acid binding and their effects on uptake of oxidized LDL. Prostaglandins Leukot. Essent. Fatty Acids 138, 64–70. doi: 10.1016/j.plefa.2016.05.005
Johannes, L., Wunder, C., Shafaq-Zadah, M. (2016). Glycolipids and lectins in endocytic uptake processes. J. Mol. Biol. 428, 4792–4818. doi: 10.1016/j.jmb.2016.10.027
Kaksonen, M., Roux, A. (2018). Mechanisms of clathrin-mediated endocytosis. Nat. Rev. Mol. Cell Biol. 19, 313–326. doi: 10.1038/nrm.2017.132
Kamili, A., Roslan, N., Frost, S., Cantrill, L. C., Wang, D., Della-Franca, A., et al. (2015). TPD52 expression increases neutral lipid storage within cultured cells. J. Cell Sci. 128 (17), 3223–3238. doi: 10.1242/jcs.167692
Kang, H. M., Ahn, S. H., Choi, P., Ko, Y. A., Han, S. H., Chinga, F., et al. (2015). Defective fatty acid oxidation in renal tubular epithelial cells has a key role in kidney fibrosis development. Nat. Med. 21 (1), 37–46. doi: 10.1038/nm.3762
Kiarely Souza, E., Pereira-Dutra, F. S., Rajão, M. A., Ferraro-Moreira, F., Goltara-Gomes, T. C., Cunha-Fernandes, T., et al. (2022). Lipid droplet accumulation occurs early following Salmonella infection and contributes to intracellular bacterial survival and replication. Mol. Microbiol. 117 (2), 293–306. doi: 10.1111/mmi.14844
Kim, I. J., Blanke, S. R. (2012). Remodeling the host environment: modulation of the gastric epithelium by the Helicobacter pylori vacuolating toxin (VacA. Front. Cell. infection Microbiol. 2, 37. doi: 10.3389/fcimb.2012.00037
Kim, S., Li, C., Farese, R. V., Walther, T. C., Voth, G. A. (2022). Key factors governing initial stages of lipid droplet formation. J. Phys. Chem. B 126 (2), 453–462. doi: 10.1021/acs.jpcb.1c09683
Kim, D. J., Rho, J. H., Woo, B. H., Joo, J. Y., Lee, J. Y., Song, J. M., et al. (2019). Periodontal pathogens modulate lipid flux via fatty acid binding protein 4. J. Dent. Res. 98 (13), 1511–1520. doi: 10.1177/0022034519880824
Knight, M., Braverman, J., Asfaha, K., Gronert, K., Stanley, S. (2018). Lipid droplet formation in Mycobacterium tuberculosis infected macrophages requires IFN-γ/HIF-1α signaling and supports host defense. PloS Pathog. 14 (1), e1006874. doi: 10.1371/journal.ppat.1006874
Kobayashi, K., Inoguchi, T., Maeda, Y., Nakashima, N., Kuwano, A., Eto, E., et al. (2008). The lack of the C-terminal domain of adipose triglyceride lipase causes neutral lipid storage disease through impaired interactions with lipid droplets. J. Clin. Endocrinol. Metab. 93 (7), 2877–2884. doi: 10.1210/jc.2007-2247
Kostarnoy, A. V., Gancheva, P. G., Kireev, I. I., Soloviev, A. I., Lepenies, B., Kulibin, A. Y., et al. (2022). A mechanism of self-lipid endocytosis mediated by the receptor Mincle. Proc. Natl. Acad. Sci. U S A 119 (30), e2120489119. doi: 10.1073/pnas
Kuerschner, L., Moessinger, C., Thiele, C. (2008). Imaging of lipid biosynthesis: How a neutral lipid enters lipid droplets. Traffic 9 (3), 338–352. doi: 10.1111/j.1600-0854.2007.00689.x
Kumar, M., Majumder, D., Mal, S., Chakraborty, S., Gupta, P., Jana, K., et al. (2020). Activating transcription factor 3 modulates the macrophage immune response to Mycobacterium tuberculosis infection via reciprocal regulation of inflammatory genes and lipid body formation. Cell Microbiol. 22 (3), e13142. doi: 10.1111/cmi.13142
Laval, T., Chaumont, L., Demangel, C. (2021). Not too fat to fight: The emerging role of macrophage fatty acid metabolism in immunity to Mycobacterium tuberculosis. Immunol. Rev. 301 (1), 84–97. doi: 10.1111/imr.12952
Lee, G., Zheng, Y., Cho, S., Jang, C., England, C., Dempsey, J. M., et al. (2017). Post-transcriptional regulation of de novo lipogenesis by mTORC1-S6K1-SRPK2 signaling. Cell 171 (7), 1545–1558.e18. doi: 10.1016/j.cell.2017.10.037
Li, S., Brown, M. S., Goldstein, J. L. (2010). Bifurcation of insulin signaling pathway in rat liver: mTORC1 required for stimulation of lipogenesis, but not inhibition of gluconeogenesis. Proc. Natl. Acad. Sci. U.S.A. 107 (8), 3441–3446. doi: 10.1073/pnas.0914798107
Li, B., Hao, J., Zeng, J., Sauter, E. R. (2020). SnapShot: FABP functions. Cell 182 (4), 1066–1066. doi: 10.1016/j.cell.2020.07.027
Li, D., Song, J. Z., Li, H., Shan, M. H., Liang, Y., Zhu, J., et al. (2015). Storage lipid synthesis is necessary for autophagy induced by nitrogen starvation. FEBS Lett. 589 (2), 269–276. doi: 10.1016/j.febslet.2014.11.050
Libbing, C. L., McDevitt, A. R., Azcueta, R. M. P., Ahila, A., Mulye, M. (2019). Lipid droplets: A significant but understudied contributor of host–bacterial interactions. Cells 8 (4), 354. doi: 10.3390/cells8040354
Lin, P., Chen, X., Moktan, H., Arrese, E. L., Duan, L., Wang, L., et al. (2014). Membrane attachment and structure models of lipid storage droplet protein 1. Biochim. Biophys. Acta Biomembr 1838 (3), 874–881. doi: 10.1016/j.bbamem.2013.12.003
Liu, Y., Zhang, Q., Zou, M., Cui, J., Shi, X., Li, L., et al. (2023). Cell entry of Bovine herpesvirus-1 through clathrin- and caveolin-mediated endocytosis requires activation of PI3K-Akt-NF-κB and Ras-p38 MAPK pathways as well as the interaction of BoHV-1 gD with cellular receptor nectin-1. Vet. Microbiol. 279, 109672. doi: 10.1016/j.vetmic.2023.109672
Luiken, J. J. F. P., Bonen, A., Glatz, J. F. C. (2002). Cellular fatty acid uptake is acutely regulated by membrane-associated fatty acid-binding proteins. Prostaglandins Leukot. Essent. Fatty Acids 67 (2–3), 73–78. doi: 10.1054/plef.2002.0401
Mannaerts, G. P., Van Veldhoven, P. P., Casteels, M. (2000). Peroxisomal lipid degradation via β- and α-oxidation in mammals. Cell Biochem. Biophys. 32 (1), 73–87. doi: 10.1385/CBB:32:1-3:73
Maréchal, L., Laviolette, M., Rodrigue-Way, A., Sow, B., Brochu, M., Caron, V., et al. (2018). The CD36-PPARγ Pathway in metabolic disorders. Int. J. Mol. Sci. 19 (5), 1529. doi: 10.3390/ijms19051529
Markgraf, D. F., Klemm, R. W., Junker, M., Hannibal-Bach, H. K., Ejsing, C. S., Rapoport, T. A. (2014). An ER protein functionally couples neutral lipid metabolism on lipid droplets to membrane lipid synthesis in the ER. Cell Rep. 6 (1), 44–55. doi: 10.1016/j.celrep.2013.11.046
Melton, E. M., Cerny, R. L., Dirusso, C. C., Black, P. N. (2013). Overexpression of human fatty acid transport protein 2/very long chain acyl-CoA synthetase 1 (FATP2/Acsvl1) reveals distinct patterns of trafficking of exogenous fatty acids. Biochem. Biophys. Res. Commun. 440 (4), 743–748. doi: 10.1016/j.bbrc.2013.09.137
Monson, E. A., Crosse, K. M., Duan, M., Chen, W., O’Shea, R. D., Wakim, L. M., et al. (2021a). Intracellular lipid droplet accumulation occurs early following viral infection and is required for an efficient interferon response. Nat. Commun. 12 (1), 4303. doi: 10.1038/s41467-021-24632-5
Monson, E. A., Trenerry, A. M., Laws, J. L., MacKenzie, J. M., Helbig, K. J. (2021b). Lipid droplets and lipid mediators in viral infection and immunity. FEMS Microbiol. Rev. 45 (4), 1–20. doi: 10.1093/femsre/fuaa066
Moreira, L. S., Piva, B., Gentile, L. B., Mesquita-Santos, F. P., D’Avila, H., Maya-Monteiro, C. M., et al. (2009). Cytosolic phospholipase A2-driven PGE2 synthesis within unsaturated fatty acids-induced lipid bodies of epithelial cells. Biochim. Biophys. Acta Mol. Cell Biol. Lipids 1791 (3), 156–165. doi: 10.1016/j.bbalip.2009.01.003
Najt, C. P., Devarajan, M., Mashek, D. G. (2022). Perilipins at a glance. J. Cell Sci. 135 (5), jcs259501. doi: 10.1242/jcs.259501
Nicholson, A. C., Han, J., Febbraio, M., Silversterin, R. L., Hajjar, D. P. (2001). Role of CD36, the macrophage class B scavenger receptor, in atherosclerosis. Ann. N Y Acad. Sci. 947, 224–228. doi: 10.1111/j.1749-6632.2001.tb03944.x
Nose, F., Yamaguchi, T., Kato, R., Aiuchi, T., Obama, T., Hara, S., et al. (2013). Crucial role of perilipin-3 (TIP47) in formation of lipid droplets and PGE2 production in HL-60-derived neutrophils. PloS One 8 (8), e71542. doi: 10.1371/journal.pone.0071542
Oluwadare, J., Cabodevilla, A. G., Son, N. H., Hu, Y., Mullick, A. E., Verano, M., et al. (2022). Blocking lipid uptake pathways does not prevent toxicity in adipose triglyceride lipase (ATGL) deficiency. J. Lipid Res. 63 (11), 100274. doi: 10.1016/j.jlr.2022.100274
Ono, T. (2005). Studies of the FABP family: A retrospective. Mol. Cell Biochem. 277 (1–2), 1–6. doi: 10.1007/s11010-005-4816-z
Östberg, Y., Berg, S., Comstedt, P., Wieslander, Å, Bergström, S. (2007). Functional analysis of a lipid galactosyltransferase synthesizing the major envelope lipid in the Lyme disease spirochete Borrelia burgdorferi. FEMS Microbiol. Lett. 272 (1), 22–29. doi: 10.1111/j.1574-6968.2007.00728.x
Owen, J. L., Zhang, Y., Bae, S. H., Farooqi, M. S., Liang, G., Hammer, R. E., et al. (2012). Insulin stimulation of SREBP-1c processing in transgenic rat hepatocytes requires p70 S6-kinase. Proc. Natl. Acad. Sci. U.S.A. 109 (40), 16184–16189. doi: 10.1073/pnas.1213343109
Pagliaccia, C., Wang, X. M., Tardy, F., Telford, J. L., Ruysschaert, J. M., Cabiaux, V. (2000). Structure and interaction of VacA of Helicobacter pylori with a lipid membrane. Eur. J. Biochem. 267 (1), 104–109. doi: 10.1046/j.1432-1327.2000.00970.x
Peña Cárcamo, J. R., Morell, M. L., Vázquez, C. A., Vatansever, S., Upadhyay, A. S., Överby, A. K., et al. (2018). The interplay between viperin antiviral activity, lipid droplets and Junín mammarenavirus multiplication. Virology 514, 216–229. doi: 10.1016/j.virol.2017.10.012
Pepino, M. Y., Kuda, O., Samovski, D., Abumrad, N. A. (2014). Structure-function of CD36 and importance of fatty acid signal transduction in fat metabolism. Annu. Rev. Nutr. 34, 281–303. doi: 10.1146/annurev-nutr-071812-161220
Peterson, T. R., Sengupta, S. S., Harris, T. E., Carmack, A. E., Kang, S. A., Balderas, E., et al. (2011). MTOR complex 1 regulates lipin 1 localization to control the srebp pathway. Cell 146 (3), 408–420. doi: 10.1016/j.cell.2011.06.034
Plotkowski, M. C., Brandão, B. A., de Assis, M. C., Feliciano, L. F. P., Raymond, B., Freitas, C., et al. (2008). Lipid body mobilization in the ExoU-induced release of inflammatory mediators by airway epithelial cells. Microb. Pathog. 45 (1), 30–37. doi: 10.1016/j.micpath.2008.01.008
Pombinho, R., Sousa, S., Cabanes, D. (2018). Scavenger receptors: promiscuous players during microbial pathogenesis. Crit. Rev. Microbiol. 44 (6), 685–700. doi: 10.1080/1040841X.2018.1493716
Putz, E. J., Andreasen, C. B., Stasko, J. A., Fernandes, L. G. V., Palmer, M. V., Rauh, M. J., et al. (2021). Circulating foamy macrophages in the golden Syrian hamster (Mesocricetus auratus) model of leptospirosis. J. Comp. Pathol. 189, 98–109. doi: 10.1016/j.jcpa.2021.10.004
Qaria, M. A., Qumar, S., Sepe, L. P., Ahmed, N. (2021). Cholesterol glucosylation-based survival strategy in Helicobacter pylori. Helicobacter 26 (2), e12777. doi: 10.1111/hel.12777
Qi, L., Yan, S., Sheng, R., Zhao, Y., Guo, X. (2014). Effects of saturated long-chain fatty acid on mRNA expression of genes associated with milk fat and protein biosynthesis in bovine mammary epithelial cells. Asian-Australas J. Anim. Sci. 27 (3), 414–421. doi: 10.5713/ajas.2013.13499
Raghunathan, K., Foegeding, N. J., Campbell, A. M., Cover, T. L., Ohi, M. D., Kenworthy, A. K. (2018). Determinants of raft partitioning of the helicobacter pylori pore-forming toxin vacA. Infect Immun 86 (5), e00872-17. doi: 10.1128/IAI.00872-17
Rho, J. H., Kim, H. J., Joo, J. Y., Lee, J. Y., Lee, J. H., Park, H. R. (2021). Periodontal pathogens promote foam cell formation by blocking lipid efflux. J. Dent. Res. 100 (12), 1367–1377. doi: 10.1177/00220345211008811
Saliba, A. M., Nascimento, D. O., Silva, M. C. A., Assis, M. C., Gayer, C. R. M., Raymond, B., et al. (2005). Eicosanoid-mediated proinflammatory activity of pseudomonas aeruginosa ExoU. Cell Microbiol. 7 (12), 1811–1822. doi: 10.1111/j.1462-5822.2005.00635.x
Samsa, M. M., Mondotte, J. A., Iglesias, N. G., Assunção-Miranda, I., Barbosa-Lima, G., Da Poian, A. T., et al. (2009). Dengue virus capsid protein usurps lipid droplets for viral particle formation. PLoS Pathog. 5 (10), e1000632. doi: 10.1371/journal.ppat.1000632
Sato, H., Coburn, J. (2017). Leptospira interrogans causes quantitative and morphological disturbances in adherens junctions and other biological groups of proteins in human endothelial cells. PLoS Negl. Trop. Dis. 11 (7), 1–27. doi: 10.1371/journal.pntd.0005830
Schoeler, M., Caesar, R. (2019). Dietary lipids, gut microbiota and lipid metabolism. Rev. Endocr. Metab. Disord. 20 (4), 461–472. doi: 10.1007/s11154-019-09512-0
Schütter, M., Giavalisco, P., Brodesser, S., Graef, M. (2020). Local Fatty Acid Channeling into Phospholipid Synthesis Drives Phagophore Expansion during Autophagy. Cell 180 (1), 135–149.e14. doi: 10.1016/j.cell.2019.12.005
Schwenk, R. W., Holloway, G. P., Luiken, J. J. F. P., Bonen, A., Glatz, J. F. C. (2010). Fatty acid transport across the cell membrane: regulation by fatty acid transporters. Prostaglandins Leukot. Essent. Fatty Acids 82 (4–6), 149–154. doi: 10.1016/j.plefa.2010.02.029
Sewald, X., Fischer, W., Haas, R. (2008). Sticky socks: Helicobacter pylori VacA takes shape. Trends in Microbiol 16 (3), 89–92. doi: 10.1016/j.tim.2008.01.001
Sharma, P., Faridi, F., Mir, I. A., Sharma, S. K. (2014). Characterization of exo-s, exo-u, and alg virulence factors and antimicrobial resistance in Pseudomonas aeruginosa isolated from migratory Egyptian vultures from India. Infect. Ecol. Epidemiol. 4 (1), 24553. doi: 10.3402/iee.v4.24553
Silverstein, R. L., Febbraio, M. (2010). CD36, a scavenger receptor involved in immunity, metabolism, angiogenesis, and behavior NIH public access. Sci. Signal 2 (72), re3. doi: 10.1126/scisignal.272re3
Singh, R., Kaushik, S., Wang, Y., Xiang, Y., Novak, I., Komatsu, M., et al. (2009). Autophagy regulates lipid metabolism. Nature 458 (7242), 1131–1135. doi: 10.1038/nature07976
Springer, T. I., Reid, T. E., Gies, S. L., Feix, J. B. (2019). Interactions of the effector ExoU from pseudomonas aeruginosa with short-chain phosphatidylinositides provide insights into ExoU targeting to host membranes. J. Biol. Chem. 294 (50), 19012–19021. doi: 10.1074/jbc.RA119.010278
Storch, J., McDermott, L. (2009). Structural and functional analysis of fatty acid-binding proteins. J. Lipid Res. 50 (SUPPL.), S126–S131. doi: 10.1194/jlr.R800084-JLR200
Stoyanova, G., Jabeen, S., Landazuri Vinueza, J., Ghosh Roy, S., Lockshin, R. A., Zakeri, Z. (2023). Zika virus triggers autophagy to exploit host lipid metabolism and drive viral replication. Cell Communication Signaling 21 (1), 114. doi: 10.1186/s12964-022-01026-8
Subedi, D., Vijay, A. K., Kohli, G. S., Rice, S. A., Willcox, M. (2018). Association between possession of ExoU and antibiotic resistance in pseudomonas aeruginosa. PloS One 13 (9), e0204936. doi: 10.1371/journal.pone.0204936
Sun, C., Zhao, Y., Gao, X., Yuan, Y., Wang, C., Wang, Y., et al. (2017). Cideb deficiency aggravates dextran sulfate sodium-induced ulcerative colitis in mice by exacerbating the oxidative burden in colonic mucosa. Inflammation Bowel Dis. 23 (8), 1338–1347. doi: 10.1097/MIB.0000000000001196
Tang, X., Xie, Y., Li, G., Niyazbekova, Z., Li, S., Chang, J., et al. (2023). ORFV entry into host cells via clathrin-mediated endocytosis and macropinocytosis. Vet. Microbiol. 284, 109831. doi: 10.1016/j.vetmic.2023.109831
Trunk, T., Casasanta, M. A., Yoo, C. C., Slade, D. J., Leo, J. C. (2019). Comparison of type 5d autotransporter phospholipases demonstrates a correlation between high activity and intracellular pathogenic lifestyle. Biochem. J. 476 (18), 2657–2676. doi: 10.1042/BCJ20190136
Turchetto-Zolet, A. C., Maraschin, F. S., De Morais, G. L., Cagliari, A., Andrade, C. M. B., Margis-Pinheiro, M., et al. (2011). Evolutionary view of acyl-CoA diacylglycerol acyltransferase (DGAT), a key enzyme in neutral lipid biosynthesis. BMC Evol. Biol. 11 (1), 263. doi: 10.1186/1471-2148-11-263
van der Meer-Janssen, Y. P. M., van Galen, J., Batenburg, J. J., Helms, J. B. (2010). Lipids in host-pathogen interactions: pathogens exploit the complexity of the host cell lipidome. Prog. Lipid Res. 49 (1), 1–26. doi: 10.1016/j.plipres.2009.07.003
Van Meer, G., Voelker, D. R., Feigenson, G. W. (2008). Membrane lipids: where they are and how they behave. Nat. Rev. Mol. Cell Biol. 9 (2), 112–124. doi: 10.1038/nrm2330
Viktorova, E. G., Nchoutmboube, J. A., Ford-Siltz, L. A., Iverson, E., Belov, G. A. (2018). Phospholipid synthesis fueled by lipid droplets drives the structural development of poliovirus replication organelles. PloS Pathog. 14 (8), e1007280. doi: 10.1371/journal.ppat.1007280
Vromman, F., Subtil, A. (2014). Exploitation of host lipids by bacteria. Curr. Opin. Microbiol. 17 (1), 38–45. doi: 10.1016/j.mib.2013.11.003
Walenna, N. F., Kurihara, Y., Chou, B., Ishii, K., Soejima, T., Itoh, R., et al. (2018). Chlamydia pneumoniae exploits adipocyte lipid chaperone FABP4 to facilitate fat mobilization and intracellular growth in murine adipocytes. Biochem. Biophys. Res. Commun. 495 (1), 353–359. doi: 10.1016/j.bbrc.2017.11.005
Walpole, G. F. W., Grinstein, S., Westman, J. (2018). The role of lipids in host-pathogen interactions. IUBMB Life 70 (5), 384–392. doi: 10.1002/iub.1737
Wältermann, M., Stöveken, T., Steinbüchel, A. (2007). Key enzymes for biosynthesis of neutral lipid storage compounds in prokaryotes: Properties, function and occurrence of wax ester synthases/acyl-CoA:diacylglycerol acyltransferases. Biochimie 89, 230–242. doi: 10.1016/j.biochi.2006.07.013
Walther, T. C., Farese, R. V. (2012). Lipid droplets and cellular lipid metabolism. Annu. Rev. Biochem. 81, 687–714. doi: 10.1146/annurev-biochem-061009-102430
Wang, W., Qu, Y., Wang, X., Xiao, M. Z. X., Fu, J., Chen, L., et al. (2023). Genetic variety of ORF3a shapes SARS-CoV-2 fitness through modulation of lipid droplet. J. Med. Virol. 95 (3), e28630. doi: 10.1002/jmv.28630
Wang, B., Tontonoz, P. (2019). Phospholipid remodeling in physiology and disease. Annu. Rev. Physiol. Annu. Reviews; 81, 165–188. doi: 10.1146/annurev-physiol-020518-114444
Wunder, C., Churin, Y., Winau, F., Warnecke, D., Vieth, M., Lindner, B., et al. (2006). Cholesterol glucosylation promotes immune evasion by Helicobacter pylori. Nat. Med. 12 (9), 1030–1038. doi: 10.1038/nm1480
Yan, Q., Song, Y., Zhang, L., Chen, Z., Yang, C., Liu, S., et al. (2018). Autophagy activation contributes to lipid accumulation in tubular epithelial cells during kidney fibrosis. Cell Death Discovery 4 (1), 2. doi: 10.1038/s41420-018-0065-2
Yang, X., Okamura, D. M., Lu, X., Chen, Y., Moorhead, J., Varghese, Z., et al. (2017). CD36 in chronic kidney disease: novel insights and therapeutic opportunities. Nat. Rev. Nephrol. 13 (12), 769–781. doi: 10.1038/nrneph.2017.126
Yu, Y., Yoshimura, S. H. (2023). Self-assembly of CIP4 drives actin-mediated asymmetric pit-closing in clathrin-mediated endocytosis. Nat. Commun. [Internet] 14 (1), 4602. doi: 10.1038/s41467-023-40390-y
Keywords: lipid, lipid metabolism, phospholipids, lipid droplets, bacterial and viral infections
Citation: Prado LG, Camara NOS and Barbosa AS (2023) Cell lipid biology in infections: an overview. Front. Cell. Infect. Microbiol. 13:1148383. doi: 10.3389/fcimb.2023.1148383
Received: 20 January 2023; Accepted: 22 September 2023;
Published: 06 October 2023.
Edited by:
Khushboo Borah Slater, University of Surrey, United KingdomReviewed by:
Filipe Pereira-Dutra, Oswaldo Cruz Foundation (Fiocruz), BrazilCopyright © 2023 Prado, Camara and Barbosa. This is an open-access article distributed under the terms of the Creative Commons Attribution License (CC BY). The use, distribution or reproduction in other forums is permitted, provided the original author(s) and the copyright owner(s) are credited and that the original publication in this journal is cited, in accordance with accepted academic practice. No use, distribution or reproduction is permitted which does not comply with these terms.
*Correspondence: Angela Silva Barbosa, YW5nZWxhLmJhcmJvc2FAYnV0YW50YW4uZ292LmJy