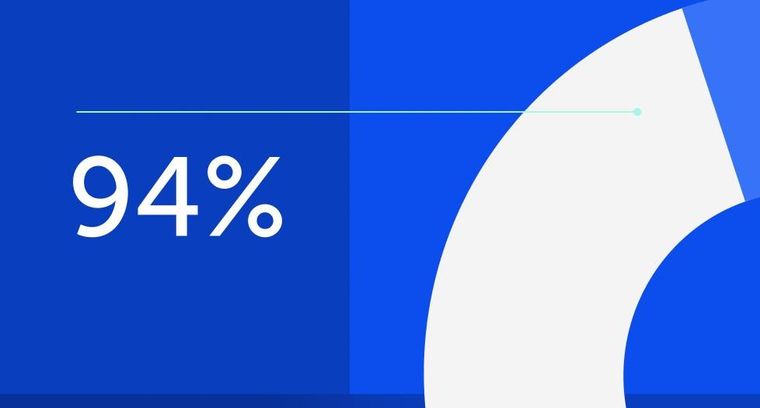
94% of researchers rate our articles as excellent or good
Learn more about the work of our research integrity team to safeguard the quality of each article we publish.
Find out more
REVIEW article
Front. Cell. Infect. Microbiol., 09 March 2023
Sec. Clinical Microbiology
Volume 13 - 2023 | https://doi.org/10.3389/fcimb.2023.1131218
This article is part of the Research TopicAlternative non-pharmacological treatments based on stem cell towards the infection of pathogens and its sequelas.View all 5 articles
Stem cells play a crucial role in re-establishing homeostasis in the body, and the search for mechanisms by which they interact with the host to exert their therapeutic effects remains a key question currently being addressed. Considering their significant regenerative/therapeutic potential, research on mesenchymal stem cells (MSCs) has experienced an unprecedented advance in recent years, becoming the focus of extensive works worldwide to develop cell-based approaches for a variety of diseases. Initial evidence for the effectiveness of MSCs therapy comes from the restoration of dynamic microenvironmental homeostasis and endogenous stem cell function in recipient tissues by systemically delivered MSCs. The specific mechanisms by which the effects are exerted remain to be investigated in depth. Importantly, the profound cell-host interplay leaves persistent therapeutic benefits that remain detectable long after the disappearance of transplanted MSCs. In this review, we summarize recent advances on the role of MSCs in multiple disease models, provide insights into the mechanisms by which MSCs interact with endogenous stem cells to exert therapeutic effects, and refine the interconnections between MSCs and cells fused to damaged sites or differentiated into functional cells early in therapy.
Mesenchymal stem cells (MSCs) are the current focus of extensive works worldwide, directed to elucidate their nature and properties, as well as to develop cell-based therapies for various diseases (Kfoury and Scadden, 2015; Harrell et al., 2019; Lerman, 2021; Zhu et al., 2021; Hoang et al., 2022). Our understanding of the therapeutic potential of MSCs has been promoted by research progresses such as the identification and characterization of MSCs from diverse origins (Darzi et al., 2016; Donders et al., 2016; Lu et al., 2016; Cooper et al., 2020; Medrano-Trochez et al., 2021), recognition of MSC contributions to organismal homeostasis and diseases (Sui B. D et al., 2016; Neri and Borzì, 2020; Sui et al., 2020; Krampera and Le Blanc, 2021; Spallanzani, 2021), the application or intervention of MSCs in tissue engineering and cytotherapy (Akiyama et al., 2012; Cassandras et al., 2020; McNeill et al., 2020), and clarification of transcription factors and signaling pathways capable of controlling the behaviors of MSCs (Feng et al., 2017; Elbaz et al., 2019; Zecchini et al., 2019; Choi et al., 2021).
Further illuminating matters, function and therapeutic efficacy of MSCs are highly regulated by the surrounding niche/microenvironment (Zhu et al., 2016; Mehrbani Azar et al., 2018; Tejero et al., 2019; Gilchrist et al., 2021), and studies on skeletal degenerative and autoimmune conditions have highlighted the essence of cell-host interplay in the forms of cell-cell contact and paracrine secretion in MSC cytotherapy (Liu S. et al., 2015; Kou et al., 2018; Li et al., 2018; Liu et al., 2018; Tejero et al., 2019; Ha et al., 2020). Interestingly, these interactions provide persistent therapeutic benefits that remain detectable long after the disappearance of transplanted MSCs (Liu S. et al., 2015; Ng et al., 2015). Therefore, there is an urgent need for a more complete understanding of the molecular mechanisms and biological processes underlying MSC therapies.
In this review, we summarize recent developments regarding the role of MSCs in a variety of disease models and provide insight into the mechanisms by which MSCs interact with endogenous stem cells to exert therapeutic effects, refining the interconnection between MSCs and cells fused or differentiated into functional cells at the site of damage in the early stages of treatment. This landscape offers a unifying explanation of how the MSC therapy re-establishes the health of the diseased organism across diverse tissues with long-lasting beneficial profiles, shedding light on the future development of cell-free and cell-targeted therapies.
The concept of MSCs originated from seminal studies performed by Friedenstein et al. who confirmed that postnatal mammalian bone marrow (BM) contains a subset of non-hematopoietic stromal cells that are both self-renewing and multipotent. Currently, the MSC concept is referred to as primitive cells capable of adherence, forming fibroblastic colonies and multilineage differentiation when cultured ex vivo (Kfoury and Scadden, 2015). MSCs, other than those derived from the BM (BMMSCs) (Jiang et al., 2002), have been shown to reside in a variety of tissues, such as the adipose (ADSCs) (Zuk et al., 2002), umbilical cord (UCMSCs) (Erices et al., 2000), tendon (TSPCs) (Bi et al., 2007), dental pulp (DPSCs) (Gronthos et al., 2000), periodontal ligament (PDLSCs) (Seo et al., 2004) and even the exfoliated deciduous teeth (SHED) (Miura et al., 2003). The surface profiles of MSCs are still not fully understood; we use a complex of heterogeneous distinct subsets of MSCs, which can be considered as a network of stromal components with interrelated and complementary in vivo capabilities in the maintenance of tissue homeostasis (Kfoury and Scadden, 2015) (Figure 1). Despite recent studies on identifying functional heterogeneity and specific markers of these cells (Chan et al., 2015; Worthley et al., 2015), the ready abilities of isolation, amplification and differentiation have made MSCs an ideal subject for extensive investigation in tissue engineering and regenerative medicine (Kfoury and Scadden, 2015). Furthermore, emerging experimentation elucidating immunomodulation, tissue regeneration, anti-aging ability and in vivo biology of MSCs has prompted their potent applications in cell-based therapy (Yue et al., 2016; Carr et al., 2019; Fu et al., 2019; Yang et al., 2019). Recent experiments with MSCs have been applied to the study and treatment of COVID-19, and the rapid response to emergent diseases is evidence of the promise of MSCs in the treatment of immune and infectious diseases (Li et al., 2020; Meng et al., 2020; Abdelgawad et al., 2021; Shi L. et al., 2021).
Figure 1 The MSC landscape in tissue homeostasis, disease and therapies. The powerful self-renewal and differentiation capabilities, immunomodulatory capacity, regeneration-promoting functions, and paracrine effects of MSCs all play important roles in tissue homeostasis, mesodermal histogenesis, and maintenance of HSC function. MSCs can intervene in disease development and treat related diseases through systemic or local applications.
According to this definition, the basic functional characteristics of MSCs are plastic adherent and clonogenic ex vivo, by which MSCs can be isolated. MSCs induced in conditioned medium for a period of time can be stained with alkaline phosphatase and alizarin red in vitro and express a series of markers of osteogenic differentiation such as alkaline phosphatase (ALP), Runt-related transcription factor 2 (RUNX2), osteocalcin (OCN) and osterix (OSX). In addition, MSCs induced in conditioned medium can be stained with oil red O and microscopically show obvious lipid droplet formation and express markers of lipogenic differentiation such as peroxisome proliferator activated receptor gamma (PPARγ) and lipoprotein lipase (LPL). The self-renewal and multipotency of MSCs were further confirmed in vivo though serial transplantation assays, with labeling of green fluorescent protein (GFP) or surface markers such as Nestin and CD146 , demonstrating the ability to reconstitute heterotopic ossicles when implanted subcutaneously and maintain identical phenotypes when generating secondary MSCs and ossicles. In addition to self-renewal and multipotency, MSCs have been identified as potent immunosuppressors (Bárcia et al., 2015; Yang et al., 2017; Vázquez et al., 2020). They possess the ability to modulate innate immune responses (Jiang et al., 2021; Dave et al., 2022), suppress the proliferation and differentiation of B cells, induce T-cell apoptosis and restore the balance between T-cell subsets, and rescue the onset of inflammation. The immunomodulatory/anti-inflammatory capacity of MSCs has been proven to be particularly important when MSCs treating autoimmune or skeletal degenerative diseases (Csobonyeiova et al., 2017; Jiang et al., 2021; Zhu et al., 2021) upon systemic delivery. Another capacity of MSCs is migration, which enable them to migrate toward damaged tissues during development and after systemic infusion, to differentiate into functional cells that exert a reparative therapeutic effect, or to fuse with cells at the site of damage and then regenerate the damaged tissue (Chen et al., 2016; Liesveld et al., 2020; Wang et al., 2020; Galgaro et al., 2021; Lee et al., 2021; Ma et al., 2021). All these repair processes suggest that MSCs can be mobilized to functional sites for endogenous tissue regeneration and functional remodeling. Recent studies have also shown that MSCs exert therapeutic effects through paracrine effects, such as miRNAs, cytokines and chemokines, which can improve the pathological microenvironment and repair locally damaged tissues; and that mitochondrial transfer mechanisms can provide functional recovery after the repair of mitochondrial dysfunction caused by aging (Babenko et al., 2018). MSCs-derived extracellular vesicles (EVs) have been the focus of recent research, producing membrane-enclosed vesicles in response to external stimuli and playing a critical role in regulating the immune microenvironment, inhibiting inflammatory factor expression and promoting angiogenesis (Babenko et al., 2018).
A conspicuous functional characteristic of MSCs is their reciprocal regulation with the surrounding niche/microenvironment. MSCs reside in a complex architecture composed of neighboring cells and abundant neurovascular bundles (Zhao et al., 2018; Imhof et al., 2020; Wu et al., 2022). MSC behaviors of quiescence and activation of MSCs are tightly controlled by the local niche according to the requirements of the host tissues (Baccin et al., 2020; Hageman et al., 2020). MSCs also accept long-distance regulation by the circulatory microenvironment through soluble factors, such as hormones (for example, estrogen), metabolites (for example, glucose) and inflammatory cytokines (for example, tumor necrosis factor-alpha [TNF-α] and interferon-gamma [IFN-γ]). (Sala et al., 2015; Sui B. D. et al., 2016; Simovic Markovic et al., 2017). MSCs modulate the ambient microenvironmental properties through cell-cell contact and paracrine secretion of various cytokines (Li et al., 2019; Song et al., 2020) and EVs (Liu S. et al., 2015; Hade et al., 2021). MSCs can produce gasotransmitters of nitric oxide (NO) (Ren et al., 2008) and hydrogen sulfide (H2S) (Liu et al., 2014) to create favorable microenvironments through autocrine/paracrine regulatory loops. These mutual communications between MSCs and microenvironments, particularly those connecting MSCs with the host immune systems and release-based interactions, provides crucial mechanisms underlying the therapeutic applications of MSCs (Ansari et al., 2017; Weiss et al., 2019; Planat-Benard et al., 2021; Yao et al., 2021).
Despite the well-documented experimentation describing the functional characteristics of MSCs, including easy access, anti-inflammatory activity mainly in the form of a thirst for damaged cells, immune modulation and regeneration promotion, a fundamental issue concerns the identity and physiological function in vivo (Figure 1). However, studies in this field have been hampered by a lack of MSC-specific antigens that permit both prospective identification and fate mapping. According to the latest statistic, it is estimated that > 9233 clinical trials have been registered and conducted on MSCs, but the lack of adequate standardized methods to assess the main safety issues involved in MSCs, specifically the lack of MSC-specific antigens for prospective identification and fate localization, has hindered their use in clinical settings. Until recently, lineage tracing and ablation studies identified several surface markers representing subsets of MSCs related to their respective nature and niches. In particular, regarding the ability of MSCs to trigger and promote tumorigenesis, although few studies have supported that MSCs are relatively safe for clinical use, the same MSCs applied to other receptors or tissues and organs also require further discussion and study.
The developmental origin and function of MSCs remain an active area of research. Although it was originally believed that MSCs (particularly BMMSCs) are derived from the mesoderm and give rise to mesenchymal cells, such as osteoblasts, adipocytes and chondrocytes (Dennis and Charbord, 2002), evidence has emerged that suggests the existence of different MSC subsets during development and possibly in adults with distinct origins and functions. Dental MSCs are generated from a unique neural crest or glial source in development (Miletich and Sharpe, 2004; Kaukua et al., 2014); most recently, Nestin+ BMMSCs have also been revealed as descendants of the neural crest, and unlike mesoderm-derived MSCs, they maintain hematopoietic stem cells (HSCs) but do not contribute to fetal osteochondrogenesis (Isern et al., 2014). Therefore, as an HSC niche component, MSCs have been detected in major hematopoietic sites during mouse development, such as the aorta-gonad-mesonephros and fetal liver during mid-gestation and in neonatal and adult BM (Mendes et al., 2005). However, the developmental hematopoietic function of MSCs seems to depend on their bone-forming capacity in the BM, where Osx-deficient MSCs fail to form osteoblasts in the metaphyseal area with reduced HSC function (Coşkun et al., 2014). Despite these findings, whether fetal MSCs function differently than postnatal MSCs remain unclear.
The postnatal roles of MSCs in tissue homeostasis is clearly understood. In this regard, BMMSCs have been intensively investigated for their putative contribution to skeletal remodeling (Chan et al., 2015; Worthley et al., 2015) and hematopoiesis (Derecka et al., 2020; Borella et al., 2021; Cai et al., 2022; Schloss et al., 2022). For instance, leptin receptor (Lepr)+ (Yue et al., 2016; Schloss et al., 2022) and Gremlin1+ (Worthley et al., 2015) cells are enriched for osteogenesis with either adipogenesis or chondrogenesis of perivascular BMMSCs in the adult skeletal system, and Nestin+ (Burt et al., 2019; Nobre et al., 2021) and platelet-derived growth factor receptor alpha (PDGFRα)+CD51+ (Lawal et al., 2017; Rux et al., 2017; Mennan et al., 2019) cells have shown co-segregation between colony-forming activity and HSC maintenance activity of BMMSCs. Moreover, dental MSCs have been shown to actively participate in the dynamic turnover of craniofacial bone (Zhao et al., 2015) and dental tissues, as represented by Gli1+ MSC subsets. However, critical questions remain as to whether different MSC markers overlap with each other and how distinct MSC subsets coordinate tissue homeostasis and diseases.
Although specific markers with related physiological function of MSCs in vivo remain to be elucidated, their pathophysiological contributions to diseases, as shown by declined or altered behaviors in situ and ex vivo, have been recognized in skeletal and dental systems. Osteoporosis, the skeletal degenerative disease, is characterized by loss of bone mass with increased marrow adiposity. It has been well documented that BMMSCs suffer from reduced proliferation with a differentiation shift from osteogenesis to adipogenesis in osteopenias of diverse pathologies (Liu et al., 2016; Sui et al., 2016a; Li et al., 2017). These functional impairments of BMMSCs could be attributed to the detrimental effects of diseased microenvironmental factors, such as estrogen deficiency and inflammation status (Chen et al., 2015; Shao et al., 2015). Similar damages to local resident MSCs have also been observed in other inflammatory conditions, e.g. osteoarthritis (Zhen et al., 2013) and periodontitis (Xue et al., 2016). Besides, ablation of Gli1+ cells leads to craniosynostosis and arrest of skull growth, indicating MSCs are indeed indispensible for skeletal homeostasis (Zhao et al., 2015). In addition, MSC aberrations have been revealed as a key pathogenesis in mutant-HSC-driven leukemia, in which BMMSCs could be impaired by neuropathy of the marrow niche to alter their HSC-maintaining secretome (Dong et al., 2016). The above findings further confirm the pathophysiological importance of MSC interactions with microenvironments in tissue homeostasis and diseases.
Given the putative key roles of MSCs in tissue homeostasis, a strategy of in situ regeneration has been proposed to reverse the functional decline of resident MSCs in treating degenerative diseases. Through inhibition of microenvironmental inflammatory impacts, systemically infusion of neutralizing antibodies of either TNF-α or IFN-γ, as well as the non-steroidal anti-inflammatory drug aspirin, has been documented to be sufficient to rescue BMMSC deficiency in osteoporosis (Sala et al., 2015; Liao et al., 2016; Xu et al., 2016; Lu et al., 2017; Simovic Markovic et al., 2017; Chang et al., 2022). Microenvironmental agents for the improvement of MSC function in osteoporosis have also been reported to include the gasotransmitter H2S donor GYY4317 and Insulin-like growth factor 1 (IGF1), together with its binding protein (IGFBP3). More agents have been developed based on mechanistic studies unraveling pharmacological targets in MSC functional regulation, such as the mammalian target of rapamycin (mTOR) signaling inhibitor rapamycin (Chen et al., 2015; Liu et al., 2016), the Notch signaling inhibitor DAPT (Liu S. et al., 2015), the nuclear transcription factor-kappa B (NF-κB) signaling inhibitor PDTC, and the migration stimulator LLP2A-Ale for directing MSCs to bone formation surfaces.
Despite the fact that these pharmacological interventions have proven effective in restoring MSC function in certain disease models, in situ MSC-based regeneration is still in the process of becoming a feasible option, as limitations still exist, including the specificity and sustainability of their therapeutic influences. To date, no MSC-targeted agents have been approved or applied in clinically. Current preclinical and clinical studies have more extensively applied exogenous MSC cytotherapy in harnessing MSCs for therapeutic use, which has been shown to be capable of restoring endogenous MSC function (Liu S. et al., 2015; Chen et al., 2017), as discussed below.
The functional characteristics of MSCs indicate their therapeutic potential. In support of this, research on MSC therapy has experienced unprecedented advances in recent years, becoming the focus of extensive work worldwide to develop approaches for a variety of diseases. In particular, evidence for the potent efficacy of MSC therapy comes from in-depth understanding of the restoration and mechanisms of systemically delivered MSCs in recipient tissue, microenvironmental homeostasis, and endogenous stem cell function. Interestingly, the benefits of MSCs are wide-ranging and remain detectable long after the disappearance of transplanted MSCs (Liu S. et al., 2015; Ng et al., 2015). Accordingly, the current recognition of MSC therapy has advanced from cell-autonomous functional determination to the essence of cell-host interplay (Figure 2).
Figure 2 Cell-host interplay determines efficacy of MSC therapy. Systemic infusion and local transplantation of MSCs can secrete anti-inflammatory factors to target cells through immunomodulatory ability to repair damaged tissues and maintain local tissue immune homeostasis. A variety of cytokines and EV forms of tissue trophic factors can be secreted through the paracrine effect to promote mobilization, proliferation, and anti-apoptosis, to improve the microenvironment of the recipient and inhibit the development and progression of disease.
The landscape of current MSC therapy contains at least two aspects: systemic and locoregional delivery of exogenous MSCs. Among these two application strategies, systemic MSC cytotherapy, primarily through intravenous and intraperitoneal injections, is currently a research hotspot in stem cell therapy, which has been recognized to have therapeutic effects in various diseases. In particular, the immunomodulatory capability of MSCs has made them attractive and potent candidates for autoimmune and inflammatory conditions, in which they can modify the systemic microenvironment further toward a beneficial environment for tissue repair (Shi et al., 2018; Medhat et al., 2019; Borella et al., 2021; Markov et al., 2021). Their ability to suppress immune responses has become the basis for numerous preclinical and clinical studies on a range of systemic conditions and their complications, including systemic lupus erythematosus (SLE), graft versus host disease (GvHD), rheumatoid arthritis (RA) (Liu R. et al., 2015; Gu and Shi, 2016), systemic sclerosis (SSc), inflammatory bowel disease (IBD), type 1 and type 2 diabetes (T1D and T2D), osteoporosis, and osteonecrosis. Furthermore, systemically delivered MSCs benefit locoregional lesions in diverse tissues, such as myocardial infarction (Luo et al., 2017), liver fibrosis, (Watanabe et al., 2019; Shi M. et al., 2021) and renal failure (Yun and Lee, 2019). The promise of MSC cytotherapy in restoring organismal homeostasis has ushered in hundreds of clinical trials that have employed systemic infusion of MSCs.
Locoregional application, has been extensively investigated in regenerative medicine. Studies based on tissue engineering techniques have successfully regenerated/repaired craniofacial (Yu et al., 2021) and long bone defects (Zhao et al., 2021), cartilage (Kangari et al., 2020), functional tooth roots and dental pulp (Xuan et al., 2018; Guo et al., 2021), periodontal structures (Sui et al., 2019), cutaneous wounds (An et al., 2015), infarcted myocardium, neurons and nerves. Furthermore, applications are in continuous progress by optimizing MSC viability with the cell-sheet/cell-aggregate technique (Xuan et al., 2018), improving scaffold materials with nanotechnology (Kuang et al., 2016) or microencapsulation (Moshaverinia et al., 2015), and combining favorable agents for MSC function with preconditioning (Shuai et al., 2016) or co-delivery systems (Moshaverinia et al., 2015). However, a significant challenge in this field is to maintain the viability of implanted MSCs in diseased microenvironments and to maximize their efficacy during the survival period. It has been reported that recipient immune systems, primarily T cells and secreted TNF-α and IFN-γ, remarkably inhibit MSC-mediated tissue regeneration by inducing MSC apoptosis and impairing MSC differentiation (López-García and Castro-Manrreza, 2021; Xie et al., 2021) (Figure 2). Further diminishing the regenerative potential, the detrimental impacts of donor comorbidities regarding aging, inflammation, and hyperglycemia are just beginning to emerge, constituting the main barrier for the application of autologous MSCs. Therefore, further studies are required toward optimizing locoregional application of MSCs, compared to systemic MSC therapy, which demonstrates distinctive advantages of minimum injuries and stable, long-lasting, and wide-ranging beneficial effects.
One of the most profound cell-host interplay in MSC therapy is the re-establishment of the recipient immunological balance, particularly homeostasis among T-cell subsets. Generally, systemic MSC infusion can reduce the number of CD3+ T cells by inhibiting proliferation and inducing apoptosis (Fujii et al., 2018; Sui et al., 2018) while also reducing recipient CD4+ and CD8+ T-cell populations (Wang et al., 2018; Harrell et al., 2021). Specifically, for the CD4+ T-cell subsets, the infused MSCs can suppress pro-inflammatory T helper 1 (Th1) and Th17 cells while promoting anti-inflammatory Th2 and CD4+CD25+Foxp3+ regulatory T cells (Tregs), thus restoring the functional balance (Court et al., 2020). Mechanistically, the infused MSCs exert combined effects by paracrine secretion and cell-cell contact: MSCs secrete monocyte chemotactic protein 1 (MCP-1) to recruit T cells (Liu et al., 2021) and various immunosuppressive cytokines such as NO and indoleamine 2,3-dioxygenase (IDO), and they express FAS ligand (FASL) and bind to FAS on T-cell surfaces to induce apoptosis (Liu et al., 2021). Interestingly, MSCs also express FAS, which plays an important role in regulating MCP-1 secretion to enhance recruitment. Apoptotic T cells can be further engulfed by macrophages and can stimulate macrophage TGF-β production to induce Tregs for immune tolerance. Surprisingly, these effects start as early as 1.5 h post-infusion in mice and last as long as 12–18 months in humans post-infusion. The amazingly persistent efficacy indicates unrecognized mechanisms underlying the long-term functional restoration of each T-cell subset. Similar phenomena were also detected when MSCs maintained macrophage homeostasis by promoting anti-inflammatory M2 polarization and inhibiting pro-inflammatory M1 polarization (Lee et al., 2015; Xie et al., 2016; Pajarinen et al., 2019; Arabpour et al., 2021).
Another example of MSC re-establishment of functional homeostasis is the restored bone remodeling balance observed in systemic MSC therapy. In osteoporotic cytotherapy, the systemic infusion of MSCs rescued the impaired bone formation rate and reduced the stimulated bone resorption rate under diverse pathological conditions (Liu S. et al., 2015; Chen et al., 2017; Sui et al., 2017). The efficacy can be attributed to increased osteoblastogenesis with decreased osteoclastogenesis through indirect mechanisms through immunomodulation (Sui et al., 2017), secretion (Liu S. et al., 2015; Chen et al., 2017), or potential homing (Sui et al., 2016b). Interestingly, skeletal therapeutic effects are also long-lasting (at least 8–12 weeks) (Liu S. et al., 2015; Chen et al., 2017; Sui et al., 2017). The paracrine effects of transplanted MSCs on other types of recipient somatic cells, including cardiac myocytes (Cheng et al., 2020), epithelial cells (Nagaishi et al., 2016), endothelial cells (Lin et al., 2015), fibroblasts (Picke et al., 2018), smooth muscle cells (Cheng et al., 2017), adipocytes (Xie et al., 2016), and neurons (He et al., 2021), have also been reported during MSC application in myocardial infarction, nephropathy, atherosclerosis, scar formation, pulmonary hypertension, insulin resistance, and axon guidance. Collectively, these findings reveal the critical role of cell-host interplay in mediating the wide-ranging and long-lasting efficacy of MSC therapy.
In addition to the functional restorations of recipient cellular components, both systemic and local microenvironments can be modified by MSC therapy toward beneficial circumstances for tissue repair. Depending on their immunomodulatory capacity, infused MSCs exert potent anti-inflammatory effects in the circulation and tissue niches, underlying indirect therapeutic efficacy in inflammation-induced bone and pancreatic islet defects (Jin et al., 2019; Gan et al., 2020; Zhou et al., 2020). The regenerated islet β cells lead to the secondary rescue of hyperglycemia, which is beneficial for addressing diabetic complications. Furthermore, both systemically infused and locoregionally transplanted MSCs secrete numerous tissue trophic factors in the form of cytokines and EVs, which possess various microenvironment-improving effects such as pro-mobilization, pro-proliferation (Deng et al., 2016; Grange et al., 2019; Mathew et al., 2019), anti-apoptosis (Nagaishi et al., 2016; Grange et al., 2019), and pro-/anti-angiogenesis (Todeschi et al., 2015; Zanotti et al., 2016; Xiao et al., 2021), indicating general recovery of diseased recipient microenvironments (Figure 2).
Notably, cell-host interplay indicates reciprocal interactions in that recipient microenvironmental status also greatly influences the therapeutic performance of MSCs. In addition to the pro-inflammatory T cells in recipients that inhibit MSC regeneration through synergistic effects of IFN-γ and TNF-α, crosstalk between transplanted donor MSCs and the recipient immune system also exists in the systemic application of MSCs, but functions distinctively to trigger immunomodulation of exogenous MSCs. In particular, recipient IFN-γ combined with other pro-inflammatory cytokines induce the secretion of chemokines and NO by exogenous MSCs, which recruit and inhibit recipient T cells, respectively. Recipient IFN-γ also elicits the expression and secretion of other immunoregulatory cytokines of donor MSCs such as IDO, thus rescuing the impaired immunosuppressive function of diseased MSCs. Another recipient factor, nevertheless, participates as a regulatory element in the immunosuppressive function, in that the recipient hyperglycemic microenvironment diminishes immunomodulation and therapeutic effects of systemically infused MSCs on osteopenia (Le Blanc et al., 2004; Sui et al., 2017). These findings integrate a previously unrecognized axis into the cell-host interplay in MSC therapy and ravel that the fulfillment of potent therapeutic effects of MSCs requires critical assistance from and a high level of control of recipient microenvironments.
These findings, particularly the long-term restoration of recipient homeostasis, prompt further investigations on the functional recovery of recipient resident stem cells. Because of the increased osteoblastogenesis observed in MSC treating osteoporosis, osteogenic differentiation of recipient BMMSCs has been extensively examined. As expected, rescue of impaired osteogenesis of recipient BMMSCs has been detected in MSC therapies in various murine models, including osteopenia induced by estrogen deficiency (ovariectomy [OVX]), SLE (Liu S. et al., 2015; Ma et al., 2015), and SSc (Chen et al., 2017). Furthermore, recipient BMMSCs exhibited enhanced bone regenerative capability when transplanted ectopically, suggesting correlations with restored bone formation rates in situ (Liu S. et al., 2015; Chen et al., 2017). Recipient BMMSCs also showed stimulated colony-forming capacity after allogeneic MSC infusion (Chen et al., 2017). In addition, exogenous MSC therapy inhibits adipogenesis (Chen et al., 2017) and osteoclastic induction (Ma et al., 2015) of resident MSCs, thereby restoring skeletal homeostasis. Importantly, the functional recovery of recipient BMMSCs persisted for at least 12 weeks post-infusion (Liu S. et al., 2015), again indicating that a single administration of MSCs is capable of maintaining the therapeutic effects for a sustained period of time.
The effects of MSC therapy on the stimulate function of recipient endogenous stem cells have been observed in other systems. Intramuscular injection of prostacyclin-overexpressing MSCs promoted the survival and proliferation of host muscle progenitor cells under hypoxic conditions to show enhanced muscle regeneration in a murine hindlimb ischemia model (Deng et al., 2016). Systemic MSC therapy may also improve pancreatic islet β-cell regeneration to increase insulin production in T1D mice. The subcutaneously transplanted MSCs show long-distance chemotactic and inductive activity on recipient HSCs to form analog BM elements with ectopic hematopoiesis, which can rescue lethally irradiated mice and alleviate aging-related phenotypes in immunocompromised mice. These functional recoveries of endogenous stem cells, together with those observed in BMMSCs, are primarily attributed to the paracrine effects of donor MSCs, rather than their prolonged engraftment in recipient tissues (Liu S. et al., 2015; Deng et al., 2016; Rahmani et al., 2020; Ma et al., 2021; Xiao et al., 2021).
In summary, the above result revealed the extensive efficacy of MSC therapy based on cell-host interplay to trigger intensive restoration of recipient function (Figure 2). These effects, particularly the persistent functional recovery of recipient cells observed in systemic MSC therapy, suggest the existence of critical molecular alterations that mediate the long-term detectable therapeutic benefits.
The promise of stem cell therapy in regenerating damaged tissues and restoring organismal homeostasis in aging and diseases has prompted thousands of clinical trials including > 700 that employ MSCs. This relies on critical molecular mechanisms. Moreover, in the future, specific interaction mechanisms will be based on the paracrine mode of action, and the study of the effects of EVs will become a hot topic in this field.
MSC therapy is a hot topic based on its current translational application and MSCs have recently been listed as promising drugs for the treatment of COVID-19, thus demonstrating their important role in the treatment of inflammatory and immune diseases. However, the feasibility and safety of MSCs have only been tested, and there is a lack of sufficient evidence on their therapeutic efficacy, particularly with regard to the lack of clear evidence to fully characterize their potential therapeutic sequelae. Although some MSCs have been shown to be safe and effective for clinical use, it is uncertain whether this can be extended to other tissues. Their therapeutic risks are mainly focused on their heterogeneity and on the initiation and promotion of tumor production; however exosomes have been shown to circumvent these concerns and are safer to use in clinical regeneration. In conclusion, extensive study is still required before MSCs can be used in a manner and extended to wider clinical applications.
PL designed, drafted and revised the manuscript. YA, TZ critically contributed to the design of the paper for important intellectual content. ST, XH, SL and FF substantially contributed to the conception of the study. JC, KX conceived and supervised the study and revised the paper. All authors contributed to the article and approved the submitted version.
This work was supported by grants from the National Key Research and Development Program of China (2022YFA1104400 to KX and JC) and the National Natural Science Foundation of China (82071075 to KX, and 82100969 to JC).
The authors declare that the research was conducted in the absence of any commercial or financial relationships that could be construed as a potential conflict of interest.
All claims expressed in this article are solely those of the authors and do not necessarily represent those of their affiliated organizations, or those of the publisher, the editors and the reviewers. Any product that may be evaluated in this article, or claim that may be made by its manufacturer, is not guaranteed or endorsed by the publisher.
Abdelgawad, M., Bakry, N. S., Farghali, A. A., Abdel-Latif, A., Lotfy, A. (2021). Mesenchymal stem cell-based therapy and exosomes in COVID-19: current trends and prospects. Stem Cell Res. Ther. 12 (1), 469. doi: 10.1186/s13287-021-02542-z
Akiyama, K., Chen, C., Wang, D., Xu, X., Qu, C., Yamaza, T., et al. (2012). Mesenchymal-stem-cell-induced immunoregulation involves FAS-ligand-/FAS-mediated T cell apoptosis. Cell Stem Cell 10 (5), 544–555. doi: 10.1016/j.stem.2012.03.007
An, Y., Jing, H., Ming, L., Liu, S., Jin, Y. (2015). Bone marrow mesenchymal stem cell aggregate: an optimal cell therapy for full-layer cutaneous wound vascularization and regeneration. Sci. Rep. 5, 17036. doi: 10.1038/srep17036
Ansari, S., Chen, C., Hasani-Sadrabadi, M. M., Yu, B., Zadeh, H. H., Wu, B. M., et al. (2017). Hydrogel elasticity and microarchitecture regulate dental-derived mesenchymal stem cell-host immune system cross-talk. Acta Biomater 60, 181–189. doi: 10.1016/j.actbio.2017.07.017
Arabpour, M., Saghazadeh, A., Rezaei, N. (2021). Anti-inflammatory and M2 macrophage polarization-promoting effect of mesenchymal stem cell-derived exosomes. Int. Immunopharmacol 97, 107823. doi: 10.1016/j.intimp.2021.107823
Babenko, V. A., Silachev, D. N., Popkov, V. A., Zorova, L. D., Pevzner, I. B., Plotnikov, E. Y., et al. (2018). Miro1 enhances mitochondria transfer from multipotent mesenchymal stem cells (MMSC) to neural cells and improves the efficacy of cell recovery. Molecules 23 (3). doi: 10.3390/molecules23030687
Baccin, C., Al-Sabah, J., Velten, L., Helbling, P. M., Grünschläger, F., Hernández-Malmierca, P., et al. (2020). Combined single-cell and spatial transcriptomics reveal the molecular, cellular and spatial bone marrow niche organization. Nat. Cell Biol. 22 (1), 38–48. doi: 10.1038/s41556-019-0439-6
Bárcia, R. N., Santos, J. M., Filipe, M., Teixeira, M., Martins, J. P., Almeida, J., et al. (2015). What makes umbilical cord tissue-derived mesenchymal stromal cells superior immunomodulators when compared to bone marrow derived mesenchymal stromal cells? Stem Cells Int. 2015, 583984. doi: 10.1155/2015/583984
Bi, Y., Ehirchiou, D., Kilts, T. M., Inkson, C. A., Embree, M. C., Sonoyama, W., et al. (2007). Identification of tendon stem/progenitor cells and the role of the extracellular matrix in their niche. Nat. Med. 13 (10), 1219–1227. doi: 10.1038/nm1630
Borella, G., Da Ros, A., Borile, G., Porcù, E., Tregnago, C., Benetton, M., et al. (2021). Targeting the plasticity of mesenchymal stromal cells to reroute the course of acute myeloid leukemia. Blood 138 (7), 557–570. doi: 10.1182/blood.2020009845
Burt, R., Dey, A., Aref, S., Aguiar, M., Akarca, A., Bailey, K., et al. (2019). Activated stromal cells transfer mitochondria to rescue acute lymphoblastic leukemia cells from oxidative stress. Blood 134 (17), 1415–1429. doi: 10.1182/blood.2019001398
Cai, H., Kondo, M., Sandhow, L., Xiao, P., Johansson, A. S., Sasaki, T., et al. (2022). Critical role of Lama4 for hematopoiesis regeneration and acute myeloid leukemia progression. Blood 139 (20), 3040–3057. doi: 10.1182/blood.2021011510
Carr, M. J., Toma, J. S., Johnston, A. P. W., Steadman, P. E., Yuzwa, S. A., Mahmud, N., et al. (2019). Mesenchymal precursor cells in adult nerves contribute to mammalian tissue repair and regeneration. Cell Stem Cell 24 (2), 240–256 e249. doi: 10.1016/j.stem.2018.10.024
Cassandras, M., Wang, C., Kathiriya, J., Tsukui, T., Matatia, P., Matthay, M., et al. (2020). Gli1(+) mesenchymal stromal cells form a pathological niche to promote airway progenitor metaplasia in the fibrotic lung. Nat. Cell Biol. 22 (11), 1295–1306. doi: 10.1038/s41556-020-00591-9
Chan, C. K., Seo, E. Y., Chen, J. Y., Lo, D., McArdle, A., Sinha, R., et al. (2015). Identification and specification of the mouse skeletal stem cell. Cell 160, 1-2, 285–298. doi: 10.1016/j.cell.2014.12.002
Chang, G. R., Lin, W. Y., Fan, H. C., Tu, M. Y., Liu, Y. H., Yen, C. C., et al. (2022). Kefir peptides ameliorate osteoporosis in AKR1A1 knockout mice with vitamin c deficiency by promoting osteoblastogenesis and inhibiting osteoclastogenesis. BioMed. Pharmacother. 156, 113859. doi: 10.1016/j.biopha.2022.113859
Chen, C., Akiyama, K., Wang, D., Xu, X., Li, B., Moshaverinia, A., et al. (2015). mTOR inhibition rescues osteopenia in mice with systemic sclerosis. J. Exp. Med. 212 (1), 73–91. doi: 10.1084/jem.20140643
Chen, N., Sui, B. D., Hu, C. H., Cao, J., Zheng, C. X., Hou, R., et al. (2016). microRNA-21 contributes to orthodontic tooth movement. J. Dent. Res. 95 (12), 1425–1433. doi: 10.1177/0022034516657043
Chen, C., Wang, D., Moshaverinia, A., Liu, D., Kou, X., Yu, W., et al. (2017). Mesenchymal stem cell transplantation in tight-skin mice identifies miR-151-5p as a therapeutic target for systemic sclerosis. Cell Res. 27 (4), 559–577. doi: 10.1038/cr.2017.11
Cheng, H., Chang, S., Xu, R., Chen, L., Song, X., Wu, J., et al. (2020). Hypoxia-challenged MSC-derived exosomes deliver miR-210 to attenuate post-infarction cardiac apoptosis. Stem Cell Res. Ther. 11 (1), 224. doi: 10.1186/s13287-020-01737-0
Cheng, G., Wang, X., Li, Y., He, L. (2017). Let-7a-transfected mesenchymal stem cells ameliorate monocrotaline-induced pulmonary hypertension by suppressing pulmonary artery smooth muscle cell growth through STAT3-BMPR2 signaling. Stem Cell Res. Ther. 8 (1), 34. doi: 10.1186/s13287-017-0480-y
Choi, S., Yu, J., Kim, W., Park, K. S. (2021). N-cadherin mediates the migration of bone marrow-derived mesenchymal stem cells toward breast tumor cells. Theranostics 11 (14), 6786–6799. doi: 10.7150/thno.59703
Cooper, T. T., Sherman, S. E., Bell, G. I., Ma, J., Kuljanin, M., Jose, S. E., et al. (2020). Characterization of a Vimentin(high)/Nestin(high) proteome and tissue regenerative secretome generated by human pancreas-derived mesenchymal stromal cells. Stem Cells 38 (5), 666–682. doi: 10.1002/stem.3143
Coşkun, S., Chao, H., Vasavada, H., Heydari, K., Gonzales, N., Zhou, X., et al. (2014). Development of the fetal bone marrow niche and regulation of HSC quiescence and homing ability by emerging osteolineage cells. Cell Rep. 9 (2), 581–590. doi: 10.1016/j.celrep.2014.09.013
Court, A. C., Le-Gatt, A., Luz-Crawford, P., Parra, E., Aliaga-Tobar, V., Bátiz, L. F., et al. (2020). Mitochondrial transfer from MSCs to T cells induces treg differentiation and restricts inflammatory response. EMBO Rep. 21 (2), e48052. doi: 10.15252/embr.201948052
Csobonyeiova, M., Polak, S., Zamborsky, R., Danisovic, L. (2017). iPS cell technologies and their prospect for bone regeneration and disease modeling: A mini review. J. Adv. Res. 8 (4), 321–327. doi: 10.1016/j.jare.2017.02.004
Darzi, S., Werkmeister, J. A., Deane, J. A., Gargett, C. E. (2016). Identification and characterization of human endometrial mesenchymal Stem/Stromal cells and their potential for cellular therapy. Stem Cells Transl. Med. 5 (9), 1127–1132. doi: 10.5966/sctm.2015-0190
Dave, J. R., Chandekar, S. S., Behera, S., Desai, K. U., Salve, P. M., Sapkal, N. B., et al. (2022). Human gingival mesenchymal stem cells retain their growth and immunomodulatory characteristics independent of donor age. Sci. Adv. 8 (25), eabm6504. doi: 10.1126/sciadv.abm6504
Deng, Y., Yang, Z., Terry, T., Pan, S., Woodside, D. G., Wang, J., et al. (2016). Prostacyclin-producing human mesenchymal cells target H19 lncRNA to augment endogenous progenitor function in hindlimb ischaemia. Nat. Commun. 7, 11276. doi: 10.1038/ncomms11276
Dennis, J. E., Charbord, P. (2002). Origin and differentiation of human and murine stroma. Stem Cells 20 (3), 205–214. doi: 10.1634/stemcells.20-3-205
Derecka, M., Herman, J. S., Cauchy, P., Ramamoorthy, S., Lupar, E., Grün, D., et al. (2020). EBF1-deficient bone marrow stroma elicits persistent changes in HSC potential. Nat. Immunol. 21 (3), 261–273. doi: 10.1038/s41590-020-0595-7
Donders, R., Sanen, K., Paesen, R., Slenders, E., Gyselaers, W., Stinissen, P., et al. (2016). Label-free imaging of umbilical cord tissue morphology and explant-derived cells. Stem Cells Int. 2016, 5457132. doi: 10.1155/2016/5457132
Dong, L., Yu, W. M., Zheng, H., Loh, M. L., Bunting, S. T., Pauly, M., et al. (2016). Leukaemogenic effects of Ptpn11 activating mutations in the stem cell microenvironment. Nature 539 (7628), 304–308. doi: 10.1038/nature20131
Elbaz, E. M., Helmy, H. S., El-Sahar, A. E., Saad, M. A., Sayed, R. H. (2019). Lercanidipine boosts the efficacy of mesenchymal stem cell therapy in 3-NP-induced huntington’s disease model rats via modulation of the calcium/calcineurin/NFATc4 and wnt/β-catenin signalling pathways. Neurochem. Int. 131, 104548. doi: 10.1016/j.neuint.2019.104548
Erices, A., Conget, P., Minguell, J. J. (2000). Mesenchymal progenitor cells in human umbilical cord blood. Br. J. Haematol 109 (1), 235–242. doi: 10.1046/j.1365-2141.2000.01986.x
Feng, J., Jing, J., Li, J., Zhao, H., Punj, V., Zhang, T., et al. (2017). BMP signaling orchestrates a transcriptional network to control the fate of mesenchymal stem cells in mice. Development 144 (14), 2560–2569. doi: 10.1242/dev.150136
Fu, X., Liu, G., Halim, A., Ju, Y., Luo, Q., Song, A. G. (2019). Mesenchymal stem cell migration and tissue repair. Cells 8 (8). doi: 10.3390/cells8080784
Fujii, S., Miura, Y., Fujishiro, A., Shindo, T., Shimazu, Y., Hirai, H., et al. (2018). Graft-Versus-Host disease amelioration by human bone marrow mesenchymal Stromal/Stem cell-derived extracellular vesicles is associated with peripheral preservation of naive T cell populations. Stem Cells 36 (3), 434–445. doi: 10.1002/stem.2759
Galgaro, B. C., Beckenkamp, L. R., van den, M., Nunnenkamp, M., Korb, V. G., Naasani, L. I. S., et al. (2021). The adenosinergic pathway in mesenchymal stem cell fate and functions. Med. Res. Rev. 41 (4), 2316–2349. doi: 10.1002/med.21796
Gan, L., Liu, Y., Cui, D., Pan, Y., Zheng, L., Wan, M. (2020). Dental tissue-derived human mesenchymal stem cells and their potential in therapeutic application. Stem Cells Int. 2020, 8864572. doi: 10.1155/2020/8864572
Gilchrist, A. E., Serrano, J. F., Ngo, M. T., Hrnjak, Z., Kim, S., Harley, B. A. C. (2021). Encapsulation of murine hematopoietic stem and progenitor cells in a thiol-crosslinked maleimide-functionalized gelatin hydrogel. Acta Biomater 131, 138–148. doi: 10.1016/j.actbio.2021.06.028
Grange, C., Skovronova, R., Marabese, F., Bussolati, B. (2019). Stem cell-derived extracellular vesicles and kidney regeneration. Cells 8 (10). doi: 10.3390/cells8101240
Gronthos, S., Mankani, M., Brahim, J., Robey, P. G., Shi, S. (2000). Postnatal human dental pulp stem cells (DPSCs) in vitro and in vivo. Proc. Natl. Acad. Sci. U.S.A. 97 (25), 13625–13630. doi: 10.1073/pnas.240309797
Gu, Y., Shi, S. (2016). Transplantation of gingiva-derived mesenchymal stem cells ameliorates collagen-induced arthritis. Arthritis Res. Ther. 18 (1), 262. doi: 10.1186/s13075-016-1160-5
Guo, H., Li, B., Wu, M., Zhao, W., He, X., Sui, B., et al. (2021). Odontogenesis-related developmental microenvironment facilitates deciduous dental pulp stem cell aggregates to revitalize an avulsed tooth. Biomaterials 279, 121223. doi: 10.1016/j.biomaterials.2021.121223
Ha, D. H., Kim, H. K., Lee, J., Kwon, H. H., Park, G. H., Yang, S. H., et al. (2020). Mesenchymal Stem/Stromal cell-derived exosomes for immunomodulatory therapeutics and skin regeneration. Cells 9 (5). doi: 10.3390/cells9051157
Hade, M. D., Suire, C. N., Suo, Z. (2021). Mesenchymal stem cell-derived exosomes: Applications in regenerative medicine. Cells 10 (8). doi: 10.3390/cells10081959
Hageman, J. H., Heinz, M. C., Kretzschmar, K., van der Vaart, J., Clevers, H., Snippert, H. J. G. (2020). Intestinal regeneration: Regulation by the microenvironment. Dev. Cell 54 (4), 435–446. doi: 10.1016/j.devcel.2020.07.009
Harrell, C. R., Markovic, B. S., Fellabaum, C., Arsenijevic, A., Volarevic, V. (2019). Mesenchymal stem cell-based therapy of osteoarthritis: Current knowledge and future perspectives. BioMed. Pharmacother. 109, 2318–2326. doi: 10.1016/j.biopha.2018.11.099
Harrell, C. R., Volarevic, A., Djonov, V. G., Jovicic, N., Volarevic, V. (2021). Mesenchymal stem cell: A friend or foe in anti-tumor immunity. Int. J. Mol. Sci. 22 (22). doi: 10.3390/ijms222212429
He, J., Zhang, N., Zhu, Y., Jin, R., Wu, F. (2021). MSC spheroids-loaded collagen hydrogels simultaneously promote neuronal differentiation and suppress inflammatory reaction through PI3K-akt signaling pathway. Biomaterials 265, 120448. doi: 10.1016/j.biomaterials.2020.120448
Hoang, D. M., Pham, P. T., Bach, T. Q., Ngo, A. T. L., Nguyen, Q. T., Phan, T. T. K., et al. (2022). Stem cell-based therapy for human diseases. Signal Transduct Target Ther. 7 (1), 272. doi: 10.1038/s41392-022-01134-4
Imhof, T., Balic, A., Heilig, J., Chiquet-Ehrismann, R., Chiquet, M., Niehoff, A., et al. (2020). Pivotal role of tenascin-W (-n) in postnatal incisor growth and periodontal ligament remodeling. Front. Immunol. 11. doi: 10.3389/fimmu.2020.608223
Isern, J., García-García, A., Martín, A. M., Arranz, L., Martín-Pérez, D., Torroja, C., et al. (2014). The neural crest is a source of mesenchymal stem cells with specialized hematopoietic stem cell niche function. Elife 3, e03696. doi: 10.7554/eLife.03696
Jiang, Y., Jahagirdar, B. N., Reinhardt, R. L., Schwartz, R. E., Keene, C. D., Ortiz-Gonzalez, X. R., et al. (2002). Pluripotency of mesenchymal stem cells derived from adult marrow. Nature 418 (6893), 41–49. doi: 10.1038/nature00870
Jiang, Y., Zhang, P., Zhang, X., Lv, L., Zhou, Y. (2021). Advances in mesenchymal stem cell transplantation for the treatment of osteoporosis. Cell Prolif 54 (1), e12956. doi: 10.1111/cpr.12956
Jin, S. S., He, D. Q., Luo, D., Wang, Y., Yu, M., Guan, B., et al. (2019). A biomimetic hierarchical nanointerface orchestrates macrophage polarization and mesenchymal stem cell recruitment to promote endogenous bone regeneration. ACS Nano 13 (6), 6581–6595. doi: 10.1021/acsnano.9b00489
Kangari, P., Talaei-Khozani, T., Razeghian-Jahromi, I., Razmkhah, M. (2020). Mesenchymal stem cells: amazing remedies for bone and cartilage defects. Stem Cell Res. Ther. 11 (1), 492. doi: 10.1186/s13287-020-02001-1
Kaukua, N., Shahidi, M. K., Konstantinidou, C., Dyachuk, V., Kaucka, M., Furlan, A., et al. (2014). Glial origin of mesenchymal stem cells in a tooth model system. Nature 513 (7519), 551–554. doi: 10.1038/nature13536
Kfoury, Y., Scadden, D. T. (2015). Mesenchymal cell contributions to the stem cell niche. Cell Stem Cell 16 (3), 239–253. doi: 10.1016/j.stem.2015.02.019
Kou, X., Xu, X., Chen, C., Sanmillan, M. L., Cai, T., Zhou, Y., et al. (2018). The Fas/Fap-1/Cav-1 complex regulates IL-1RA secretion in mesenchymal stem cells to accelerate wound healing. Sci. Transl. Med. 10 (432). doi: 10.1126/scitranslmed.aai8524
Krampera, M., Le Blanc, K. (2021). Mesenchymal stromal cells: Putative microenvironmental modulators become cell therapy. Cell Stem Cell 28 (10), 1708–1725. doi: 10.1016/j.stem.2021.09.006
Kuang, R., Zhang, Z., Jin, X., Hu, J., Shi, S., Ni, L., et al. (2016). Nanofibrous spongy microspheres for the delivery of hypoxia-primed human dental pulp stem cells to regenerate vascularized dental pulp. Acta Biomater 33, 225–234. doi: 10.1016/j.actbio.2016.01.032
Lawal, R. A., Zhou, X., Batey, K., Hoffman, C. M., Georger, M. A., Radtke, F., et al. (2017). The notch ligand Jagged1 regulates the osteoblastic lineage by maintaining the osteoprogenitor pool. J. Bone Miner Res. 32 (6), 1320–1331. doi: 10.1002/jbmr.3106
Le Blanc, K., Rasmusson, I., Sundberg, B., Götherström, C., Hassan, M., Uzunel, M., et al. (2004). Treatment of severe acute graft-versus-host disease with third party haploidentical mesenchymal stem cells. Lancet 363 (9419), 1439–1441. doi: 10.1016/s0140-6736(04)16104-7
Lee, K. S., Lee, J., Kim, H. K., Yeom, S. H., Woo, C. H., Jung, Y. J., et al. (2021). Extracellular vesicles from adipose tissue-derived stem cells alleviate osteoporosis through osteoprotegerin and miR-21-5p. J. Extracell Vesicles 10 (12), e12152. doi: 10.1002/jev2.12152
Lee, K. C., Lin, H. C., Huang, Y. H., Hung, S. C. (2015). Allo-transplantation of mesenchymal stem cells attenuates hepatic injury through IL1Ra dependent macrophage switch in a mouse model of liver disease. J. Hepatol. 63 (6), 1405–1412. doi: 10.1016/j.jhep.2015.07.035
Lerman, L. O. (2021). Cell-based regenerative medicine for renovascular disease. Trends Mol. Med. 27 (9), 882–894. doi: 10.1016/j.molmed.2021.06.004
Li, X., An, G., Wang, Y., Liang, D., Zhu, Z., Tian, L. (2018). Targeted migration of bone marrow mesenchymal stem cells inhibits silica-induced pulmonary fibrosis in rats. Stem Cell Res. Ther. 9 (1), 335. doi: 10.1186/s13287-018-1083-y
Li, H., Liu, P., Xu, S., Li, Y., Dekker, J. D., Li, B., et al. (2017). FOXP1 controls mesenchymal stem cell commitment and senescence during skeletal aging. J. Clin. Invest. 127 (4), 1241–1253. doi: 10.1172/JCI89511
Li, Z., Niu, S., Guo, B., Gao, T., Wang, L., Wang, Y., et al. (2020). Stem cell therapy for COVID-19, ARDS and pulmonary fibrosis. Cell Prolif 53 (12), e12939. doi: 10.1111/cpr.12939
Li, J. Y., Ren, K. K., Zhang, W. J., Xiao, L., Wu, H. Y., Liu, Q. Y., et al. (2019). Human amniotic mesenchymal stem cells and their paracrine factors promote wound healing by inhibiting heat stress-induced skin cell apoptosis and enhancing their proliferation through activating PI3K/AKT signaling pathway. Stem Cell Res. Ther. 10 (1), 247. doi: 10.1186/s13287-019-1366-y
Liao, L., Su, X., Yang, X., Hu, C., Li, B., Lv, Y., et al. (2016). TNF-α inhibits FoxO1 by upregulating miR-705 to aggravate oxidative damage in bone marrow-derived mesenchymal stem cells during osteoporosis. Stem Cells 34 (4), 1054–1067. doi: 10.1002/stem.2274
Liesveld, J. L., Sharma, N., Aljitawi, O. S. (2020). Stem cell homing: From physiology to therapeutics. Stem Cells 38 (10), 1241–1253. doi: 10.1002/stem.3242
Lin, Y. L., Yet, S. F., Hsu, Y. T., Wang, G. J., Hung, S. C. (2015). Mesenchymal stem cells ameliorate atherosclerotic lesions via restoring endothelial function. Stem Cells Transl. Med. 4 (1), 44–55. doi: 10.5966/sctm.2014-0091
Liu, M., He, J., Zheng, S., Zhang, K., Ouyang, Y., Zhang, Y., et al. (2021). Human umbilical cord mesenchymal stem cells ameliorate acute liver failure by inhibiting apoptosis, inflammation and pyroptosis. Ann. Transl. Med. 9 (21), 1615. doi: 10.21037/atm-21-2885
Liu, D., Kou, X., Chen, C., Liu, S., Liu, Y., Yu, W., et al. (2018). Circulating apoptotic bodies maintain mesenchymal stem cell homeostasis and ameliorate osteopenia via transferring multiple cellular factors. Cell Res. 28 (9), 918–933. doi: 10.1038/s41422-018-0070-2
Liu, Y., Kou, X., Chen, C., Yu, W., Su, Y., Kim, Y., et al. (2016). Chronic high dose alcohol induces osteopenia via activation of mTOR signaling in bone marrow mesenchymal stem cells. Stem Cells 34 (8), 2157–2168. doi: 10.1002/stem.2392
Liu, R., Li, X., Zhang, Z., Zhou, M., Sun, Y., Su, D., et al. (2015). Allogeneic mesenchymal stem cells inhibited T follicular helper cell generation in rheumatoid arthritis. Sci. Rep. 5, 12777. doi: 10.1038/srep12777
Liu, S., Liu, D., Chen, C., Hamamura, K., Moshaverinia, A., Yang, R., et al. (2015). MSC transplantation improves osteopenia via epigenetic regulation of notch signaling in lupus. Cell Metab. 22 (4), 606–618. doi: 10.1016/j.cmet.2015.08.018
Liu, Y., Yang, R., Liu, X., Zhou, Y., Qu, C., Kikuiri, T., et al. (2014). Hydrogen sulfide maintains mesenchymal stem cell function and bone homeostasis via regulation of Ca(2+) channel sulfhydration. Cell Stem Cell 15 (1), 66–78. doi: 10.1016/j.stem.2014.03.005
López-García, L., Castro-Manrreza, M. E. (2021). TNF-α and IFN-γ participate in improving the immunoregulatory capacity of mesenchymal Stem/Stromal cells: Importance of cell-cell contact and extracellular vesicles. Int. J. Mol. Sci. 22 (17). doi: 10.3390/ijms22179531
Lu, S., Ge, M., Zheng, Y., Li, J., Feng, X., Feng, S., et al. (2017). CD106 is a novel mediator of bone marrow mesenchymal stem cells via NF-κB in the bone marrow failure of acquired aplastic anemia. Stem Cell Res. Ther. 8 (1), 178. doi: 10.1186/s13287-017-0620-4
Lu, Z., Wu, M., Zhang, J., Xiong, J., Cheng, J., Shen, W., et al. (2016). Improvement in isolation and identification of mouse oogonial stem cells. Stem Cells Int. 2016, 2749461. doi: 10.1155/2016/2749461
Luo, L., Tang, J., Nishi, K., Yan, C., Dinh, P. U., Cores, J., et al. (2017). Fabrication of synthetic mesenchymal stem cells for the treatment of acute myocardial infarction in mice. Circ. Res. 120 (11), 1768–1775. doi: 10.1161/circresaha.116.310374
Ma, L., Aijima, R., Hoshino, Y., Yamaza, H., Tomoda, E., Tanaka, Y., et al. (2015). Transplantation of mesenchymal stem cells ameliorates secondary osteoporosis through interleukin-17-impaired functions of recipient bone marrow mesenchymal stem cells in MRL/lpr mice. Stem Cell Res. Ther. 6, 104. doi: 10.1186/s13287-015-0091-4
Ma, Z., Zhou, J., Yang, T., Xie, W., Song, G., Song, Z., et al. (2021). Mesenchymal stromal cell therapy for pancreatitis: Progress and challenges. Med. Res. Rev. 41 (4), 2474–2488. doi: 10.1002/med.21801
Markov, A., Thangavelu, L., Aravindhan, S., Zekiy, A. O., Jarahian, M., Chartrand, M. S., et al. (2021). Mesenchymal stem/stromal cells as a valuable source for the treatment of immune-mediated disorders. Stem Cell Res. Ther. 12 (1), 192. doi: 10.1186/s13287-021-02265-1
Mathew, B., Ravindran, S., Liu, X., Torres, L., Chennakesavalu, M., Huang, C. C., et al. (2019). Mesenchymal stem cell-derived extracellular vesicles and retinal ischemia-reperfusion. Biomaterials 197, 146–160. doi: 10.1016/j.biomaterials.2019.01.016
McNeill, E. P., Zeitouni, S., Pan, S., Haskell, A., Cesarek, M., Tahan, D., et al. (2020). Characterization of a pluripotent stem cell-derived matrix with powerful osteoregenerative capabilities. Nat. Commun. 11 (1), 3025. doi: 10.1038/s41467-020-16646-2
Medhat, D., Rodríguez, C. I., Infante, A. (2019). Immunomodulatory effects of MSCs in bone healing. Int. J. Mol. Sci. 20 (21). doi: 10.3390/ijms20215467
Medrano-Trochez, C., Chatterjee, P., Pradhan, P., Stevens, H. Y., Ogle, M. E., Botchwey, E. A., et al. (2021). Single-cell RNA-seq of out-of-thaw mesenchymal stromal cells shows tissue-of-origin differences and inter-donor cell-cycle variations. Stem Cell Res. Ther. 12 (1), 565. doi: 10.1186/s13287-021-02627-9
Mehrbani Azar, Y., Green, R., Niesler, C. U., van de Vyver, M. (2018). Antioxidant preconditioning improves the paracrine responsiveness of mouse bone marrow mesenchymal stem cells to diabetic wound fluid. Stem Cells Dev. 27 (23), 1646–1657. doi: 10.1089/scd.2018.0145
Mendes, S. C., Robin, C., Dzierzak, E. (2005). Mesenchymal progenitor cells localize within hematopoietic sites throughout ontogeny. Development 132 (5), 1127–1136. doi: 10.1242/dev.01615
Meng, F., Xu, R., Wang, S., Xu, Z., Zhang, C., Li, Y., et al. (2020). Human umbilical cord-derived mesenchymal stem cell therapy in patients with COVID-19: a phase 1 clinical trial. Signal Transduct Target Ther. 5 (1), 172. doi: 10.1038/s41392-020-00286-5
Mennan, C., Garcia, J., Roberts, S., Hulme, C., Wright, K. (2019). A comprehensive characterisation of large-scale expanded human bone marrow and umbilical cord mesenchymal stem cells. Stem Cell Res. Ther. 10 (1), 99. doi: 10.1186/s13287-019-1202-4
Miletich, I., Sharpe, P. T. (2004). Neural crest contribution to mammalian tooth formation. Birth Defects Res. C Embryo Today 72 (2), 200–212. doi: 10.1002/bdrc.20012
Miura, M., Gronthos, S., Zhao, M., Lu, B., Fisher, L. W., Robey, P. G., et al. (2003). SHED: stem cells from human exfoliated deciduous teeth. Proc. Natl. Acad. Sci. U.S.A. 100 (10), 5807–5812. doi: 10.1073/pnas.0937635100
Moshaverinia, A., Chen, C., Xu, X., Ansari, S., Zadeh, H. H., Schricker, S. R., et al. (2015). Regulation of the stem cell-host immune system interplay using hydrogel coencapsulation system with an anti-inflammatory drug. Adv. Funct. Mater 25 (15), 2296–2307. doi: 10.1002/adfm.201500055
Nagaishi, K., Mizue, Y., Chikenji, T., Otani, M., Nakano, M., Konari, N., et al. (2016). Mesenchymal stem cell therapy ameliorates diabetic nephropathy via the paracrine effect of renal trophic factors including exosomes. Sci. Rep. 6, 34842. doi: 10.1038/srep34842
Neri, S., Borzì, R. M. (2020). Molecular mechanisms contributing to mesenchymal stromal cell aging. Biomolecules 10 (2). doi: 10.3390/biom10020340
Ng, K. S., Kuncewicz, T. M., Karp, J. M. (2015). Beyond hit-and-Run: Stem cells leave a lasting memory. Cell Metab. 22 (4), 541–543. doi: 10.1016/j.cmet.2015.09.019
Nobre, A. R., Risson, E., Singh, D. K., Di Martino, J. S., Cheung, J. F., Wang, J., et al. (2021). Bone marrow NG2(+)/Nestin(+) mesenchymal stem cells drive DTC dormancy via TGFβ2. Nat. Cancer 2 (3), 327–339. doi: 10.1038/s43018-021-00179-8
Pajarinen, J., Lin, T., Gibon, E., Kohno, Y., Maruyama, M., Nathan, K., et al. (2019). Mesenchymal stem cell-macrophage crosstalk and bone healing. Biomaterials 196, 80–89. doi: 10.1016/j.biomaterials.2017.12.025
Picke, A. K., Campbell, G. M., Blüher, M., Krügel, U., Schmidt, F. N., Tsourdi, E., et al. (2018). Thy-1 (CD90) promotes bone formation and protects against obesity. Sci. Transl. Med. 10 (453). doi: 10.1126/scitranslmed.aao6806
Planat-Benard, V., Varin, A., Casteilla, L. (2021). MSCs and inflammatory cells crosstalk in regenerative medicine: Concerted actions for optimized resolution driven by energy metabolism. Front. Immunol. 12. doi: 10.3389/fimmu.2021.626755
Rahmani, A., Saleki, K., Javanmehr, N., Khodaparast, J., Saadat, P., Nouri, H. R. (2020). Mesenchymal stem cell-derived extracellular vesicle-based therapies protect against coupled degeneration of the central nervous and vascular systems in stroke. Ageing Res. Rev. 62, 101106. doi: 10.1016/j.arr.2020.101106
Ren, G., Zhang, L., Zhao, X., Xu, G., Zhang, Y., Roberts, A. I., et al. (2008). Mesenchymal stem cell-mediated immunosuppression occurs via concerted action of chemokines and nitric oxide. Cell Stem Cell 2 (2), 141–150. doi: 10.1016/j.stem.2007.11.014
Rux, D. R., Song, J. Y., Pineault, K. M., Mandair, G. S., Swinehart, I. T., Schlientz, A. J., et al. (2017). Hox11 function is required for region-specific fracture repair. J. Bone Miner Res. 32 (8), 1750–1760. doi: 10.1002/jbmr.3166
Sala, E., Genua, M., Petti, L., Anselmo, A., Arena, V., Cibella, J., et al. (2015). Mesenchymal stem cells reduce colitis in mice via release of TSG6, independently of their localization to the intestine. Gastroenterology 149 (1), 163–176 e120. doi: 10.1053/j.gastro.2015.03.013
Schloss, M. J., Hulsmans, M., Rohde, D., Lee, I. H., Severe, N., Foy, B. H., et al. (2022). B lymphocyte-derived acetylcholine limits steady-state and emergency hematopoiesis. Nat. Immunol. 23 (4), 605–618. doi: 10.1038/s41590-022-01165-7
Seo, B. M., Miura, M., Gronthos, S., Bartold, P. M., Batouli, S., Brahim, J., et al. (2004). Investigation of multipotent postnatal stem cells from human periodontal ligament. Lancet 364 (9429), 149–155. doi: 10.1016/s0140-6736(04)16627-0
Shao, B., Liao, L., Yu, Y., Shuai, Y., Su, X., Jing, H., et al. (2015). Estrogen preserves fas ligand levels by inhibiting microRNA-181a in bone marrow-derived mesenchymal stem cells to maintain bone remodeling balance. FASEB J. 29 (9), 3935–3944. doi: 10.1096/fj.15-272823
Shi, M., Li, Y. Y., Xu, R. N., Meng, F. P., Yu, S. J., Fu, J. L., et al. (2021). Mesenchymal stem cell therapy in decompensated liver cirrhosis: a long-term follow-up analysis of the randomized controlled clinical trial. Hepatol. Int. 15 (6), 1431–1441. doi: 10.1007/s12072-021-10199-2
Shi, Y., Wang, Y., Li, Q., Liu, K., Hou, J., Shao, C., et al. (2018). Immunoregulatory mechanisms of mesenchymal stem and stromal cells in inflammatory diseases. Nat. Rev. Nephrol. 14 (8), 493–507. doi: 10.1038/s41581-018-0023-5
Shi, L., Wang, L., Xu, R., Zhang, C., Xie, Y., Liu, K., et al. (2021). Mesenchymal stem cell therapy for severe COVID-19. Signal Transduct Target Ther. 6 (1), 339. doi: 10.1038/s41392-021-00754-6
Shuai, Y., Liao, L., Su, X., Yu, Y., Shao, B., Jing, H., et al. (2016). Melatonin treatment improves mesenchymal stem cells therapy by preserving stemness during long-term In vitro expansion. Theranostics 6 (11), 1899–1917. doi: 10.7150/thno.15412
Simovic Markovic, B., Gazdic, M., Arsenijevic, A., Jovicic, N., Jeremic, J., Djonov, V., et al. (2017). Mesenchymal stem cells attenuate cisplatin-induced nephrotoxicity in iNOS-dependent manner. Stem Cells Int. 2017, 1315378. doi: 10.1155/2017/1315378
Song, N., Scholtemeijer, M., Shah, K. (2020). Mesenchymal stem cell immunomodulation: Mechanisms and therapeutic potential. Trends Pharmacol. Sci. 41 (9), 653–664. doi: 10.1016/j.tips.2020.06.009
Spallanzani, R. G. (2021). Visceral adipose tissue mesenchymal stromal cells in the intersection of immunology and metabolism. Am. J. Physiol. Endocrinol. Metab. 320 (3), E512–E519. doi: 10.1152/ajpendo.00341.2020
Sui, B., Chen, C., Kou, X., Li, B., Xuan, K., Shi, S., et al. (2019). Pulp stem cell-mediated functional pulp regeneration. J. Dent. Res. 98 (1), 27–35. doi: 10.1177/0022034518808754
Sui, B. D., Chen, J., Zhang, X. Y., He, T., Zhao, P., Zheng, C. X., et al. (2018). Gender-independent efficacy of mesenchymal stem cell therapy in sex hormone-deficient bone loss via immunosuppression and resident stem cell recovery. Exp. Mol. Med. 50 (12), 1–14. doi: 10.1038/s12276-018-0192-0
Sui, B., Hu, C., Liao, L., Chen, Y., Zhang, X., Fu, X., et al. (2016a). Mesenchymal progenitors in osteopenias of diverse pathologies: differential characteristics in the common shift from osteoblastogenesis to adipogenesis. Sci. Rep. 6, 30186. doi: 10.1038/srep30186
Sui, B., Hu, C., Zhang, X., Zhao, P., He, T., Zhou, C., et al. (2016b). Allogeneic mesenchymal stem cell therapy promotes osteoblastogenesis and prevents glucocorticoid-induced osteoporosis. Stem Cells Transl. Med. 5 (9), 1238–1246. doi: 10.5966/sctm.2015-0347
Sui, B. D., Hu, C. H., Zheng, C. X., Jin, Y. (2016). Microenvironmental views on mesenchymal stem cell differentiation in aging. J. Dent. Res. 95 (12), 1333–1340. doi: 10.1177/0022034516653589
Sui, B. D., Hu, C. H., Zheng, C. X., Shuai, Y., He, X. N., Gao, P. P., et al. (2017). Recipient glycemic micro-environments govern therapeutic effects of mesenchymal stem cell infusion on osteopenia. Theranostics 7 (5), 1225–1244. doi: 10.7150/thno.18181
Sui, B. D., Zheng, C. X., Li, M., Jin, Y., Hu, C. H. (2020). Epigenetic regulation of mesenchymal stem cell homeostasis. Trends Cell Biol. 30 (2), 97–116. doi: 10.1016/j.tcb.2019.11.006
Tejero, R., Huang, Y., Katsyv, I., Kluge, M., Lin, J. Y., Tome-Garcia, J., et al. (2019). Gene signatures of quiescent glioblastoma cells reveal mesenchymal shift and interactions with niche microenvironment. EBioMedicine 42, 252–269. doi: 10.1016/j.ebiom.2019.03.064
Todeschi, M. R., El Backly, R., Capelli, C., Daga, A., Patrone, E., Introna, M., et al. (2015). Transplanted umbilical cord mesenchymal stem cells modify the in vivo microenvironment enhancing angiogenesis and leading to bone regeneration. Stem Cells Dev. 24 (13), 1570–1581. doi: 10.1089/scd.2014.0490
Vázquez, A., Fernández-Sevilla, L. M., Jiménez, E., Pérez-Cabrera, D., Yañez, R., Subiza, J. L., et al. (2020). Involvement of mesenchymal stem cells in oral mucosal bacterial immunotherapy. Front. Immunol. 11. doi: 10.3389/fimmu.2020.567391
Wang, Y., Deng, P., Liu, Y., Wu, Y., Chen, Y., Guo, Y., et al. (2020). Alpha-ketoglutarate ameliorates age-related osteoporosis via regulating histone methylations. Nat. Commun. 11 (1), 5596. doi: 10.1038/s41467-020-19360-1
Wang, L. T., Jiang, S. S., Ting, C. H., Hsu, P. J., Chang, C. C., Sytwu, H. K., et al. (2018). Differentiation of mesenchymal stem cells from human induced pluripotent stem cells results in downregulation of c-myc and DNA replication pathways with immunomodulation toward CD4 and CD8 cells. Stem Cells 36 (6), 903–914. doi: 10.1002/stem.2795
Watanabe, Y., Tsuchiya, A., Seino, S., Kawata, Y., Kojima, Y., Ikarashi, S., et al. (2019). Mesenchymal stem cells and induced bone marrow-derived macrophages synergistically improve liver fibrosis in mice. Stem Cells Transl. Med. 8 (3), 271–284. doi: 10.1002/sctm.18-0105
Weiss, D. J., English, K., Krasnodembskaya, A., Isaza-Correa, J. M., Hawthorne, I. J., Mahon, B. P. (2019). The necrobiology of mesenchymal stromal cells affects therapeutic efficacy. Front. Immunol. 10. doi: 10.3389/fimmu.2019.01228
Worthley, D. L., Churchill, M., Compton, J. T., Tailor, Y., Rao, M., Si, Y., et al. (2015). Gremlin 1 identifies a skeletal stem cell with bone, cartilage, and reticular stromal potential. Cell 160 (1-2), 269–284. doi: 10.1016/j.cell.2014.11.042
Wu, Y., Zhou, X., Yuan, W., Liu, J., Yang, W., Zhu, Y., et al. (2022). Gli1+ mesenchymal stem cells in bone and teeth. Curr. Stem Cell Res. Ther. 17 (6), 494–502. doi: 10.2174/1574888x17666220107102911
Xiao, X., Xu, M., Yu, H., Wang, L., Li, X., Rak, J., et al. (2021). Mesenchymal stem cell-derived small extracellular vesicles mitigate oxidative stress-induced senescence in endothelial cells via regulation of miR-146a/Src. Signal Transduct Target Ther. 6 (1), 354. doi: 10.1038/s41392-021-00765-3
Xie, Z., Hao, H., Tong, C., Cheng, Y., Liu, J., Pang, Y., et al. (2016). Human umbilical cord-derived mesenchymal stem cells elicit macrophages into an anti-inflammatory phenotype to alleviate insulin resistance in type 2 diabetic rats. Stem Cells 34 (3), 627–639. doi: 10.1002/stem.2238
Xie, Z., Yu, W., Zheng, G., Li, J., Cen, S., Ye, G., et al. (2021). TNF-α-mediated m(6)A modification of ELMO1 triggers directional migration of mesenchymal stem cell in ankylosing spondylitis. Nat. Commun. 12 (1), 5373. doi: 10.1038/s41467-021-25710-4
Xu, J., Wang, Y., Li, J., Zhang, X., Geng, Y., Huang, Y., et al. (2016). IL-12p40 impairs mesenchymal stem cell-mediated bone regeneration via CD4(+) T cells. Cell Death Differ 23 (12), 1941–1951. doi: 10.1038/cdd.2016.72
Xuan, K., Li, B., Guo, H., Sun, W., Kou, X., He, X., et al. (2018). Deciduous autologous tooth stem cells regenerate dental pulp after implantation into injured teeth. Sci. Transl. Med. 10 (455). doi: 10.1126/scitranslmed.aaf3227
Xue, P., Li, B., An, Y., Sun, J., He, X., Hou, R., et al. (2016). Decreased MORF leads to prolonged endoplasmic reticulum stress in periodontitis-associated chronic inflammation. Cell Death Differ 23 (11), 1862–1872. doi: 10.1038/cdd.2016.74
Yang, C., Liu, X., Zhao, K., Zhu, Y., Hu, B., Zhou, Y., et al. (2019). miRNA-21 promotes osteogenesis via the PTEN/PI3K/Akt/HIF-1α pathway and enhances bone regeneration in critical size defects. Stem Cell Res. Ther. 10 (1), 65. doi: 10.1186/s13287-019-1168-2
Yang, Q., Nanayakkara, G. K., Drummer, C., Sun, Y., Johnson, C., Cueto, R., et al. (2017). Low-intensity ultrasound-induced anti-inflammatory effects are mediated by several new mechanisms including gene induction, immunosuppressor cell promotion, and enhancement of exosome biogenesis and docking. Front. Physiol. 8. doi: 10.3389/fphys.2017.00818
Yao, M., Cui, B., Zhang, W., Ma, W., Zhao, G., Xing, L. (2021). Exosomal miR-21 secreted by IL-1β-primed-mesenchymal stem cells induces macrophage M2 polarization and ameliorates sepsis. Life Sci. 264, 118658. doi: 10.1016/j.lfs.2020.118658
Yu, M., Ma, L., Yuan, Y., Ye, X., Montagne, A., He, J., et al. (2021). Cranial suture regeneration mitigates skull and neurocognitive defects in craniosynostosis. Cell 184 (1), 243–256 e218. doi: 10.1016/j.cell.2020.11.037
Yue, R., Zhou, B. O., Shimada, I. S., Zhao, Z., Morrison, S. J. (2016). Leptin receptor promotes adipogenesis and reduces osteogenesis by regulating mesenchymal stromal cells in adult bone marrow. Cell Stem Cell 18 (6), 782–796. doi: 10.1016/j.stem.2016.02.015
Yun, C. W., Lee, S. H. (2019). Potential and therapeutic efficacy of cell-based therapy using mesenchymal stem cells for acute/chronic kidney disease. Int. J. Mol. Sci. 20 (7). doi: 10.3390/ijms20071619
Zanotti, L., Angioni, R., Cali, B., Soldani, C., Ploia, C., Moalli, F., et al. (2016). Mouse mesenchymal stem cells inhibit high endothelial cell activation and lymphocyte homing to lymph nodes by releasing TIMP-1. Leukemia 30 (5), 1143–1154. doi: 10.1038/leu.2016.33
Zecchini, S., Giovarelli, M., Perrotta, C., Morisi, F., Touvier, T., Di Renzo, I., et al. (2019). Autophagy controls neonatal myogenesis by regulating the GH-IGF1 system through a NFE2L2- and DDIT3-mediated mechanism. Autophagy 15 (1), 58–77. doi: 10.1080/15548627.2018.1507439
Zhao, H., Feng, J., Ho, T. V., Grimes, W., Urata, M., Chai, Y. (2015). The suture provides a niche for mesenchymal stem cells of craniofacial bones. Nat. Cell Biol. 17 (4), 386–396. doi: 10.1038/ncb3139
Zhao, H., Feng, J., Seidel, K., Shi, S., Klein, O., Sharpe, P., et al. (2018). Secretion of shh by a neurovascular bundle niche supports mesenchymal stem cell homeostasis in the adult mouse incisor. Cell Stem Cell 23 (1), 147. doi: 10.1016/j.stem.2018.05.023
Zhao, L., Zhao, J., Tuo, Z., Ren, G. (2021). Repair of long bone defects of large size using a tissue-engineered periosteum in a rabbit model. J. Mater Sci. Mater Med. 32 (9), 105. doi: 10.1007/s10856-021-06579-7
Zhen, G., Wen, C., Jia, X., Li, Y., Crane, J. L., Mears, S. C., et al (2013). Inhibition of TGF-beta signaling in mesenchymal stem cells of subchondral bone attenuates osteoarthritis. Nat Med 19 (6), 704–712. doi: 10.1038/nm.3143
Zhou, L. L., Liu, W., Wu, Y. M., Sun, W. L., Dörfer, C. E., Fawzy El-Sayed, K. M. (2020). Oral mesenchymal Stem/Progenitor cells: The immunomodulatory masters. Stem Cells Int. 2020, 1327405. doi: 10.1155/2020/1327405
Zhu, Y., Ge, J., Huang, C., Liu, H., Jiang, H. (2021). Application of mesenchymal stem cell therapy for aging frailty: From mechanisms to therapeutics. Theranostics 11 (12), 5675–5685. doi: 10.7150/thno.46436
Zhu, M., Lin, S., Sun, Y., Feng, Q., Li, G., Bian, L. (2016). Hydrogels functionalized with n-cadherin mimetic peptide enhance osteogenesis of hMSCs by emulating the osteogenic niche. Biomaterials 77, 44–52. doi: 10.1016/j.biomaterials.2015.10.072
Keywords: mesenchymal stem cells, cell therapy, cell-host interplay, cell release, microenvironment
Citation: Liu P, An Y, Zhu T, Tang S, Huang X, Li S, Fu F, Chen J and Xuan K (2023) Mesenchymal stem cells: Emerging concepts and recent advances in their roles in organismal homeostasis and therapy. Front. Cell. Infect. Microbiol. 13:1131218. doi: 10.3389/fcimb.2023.1131218
Received: 24 December 2022; Accepted: 03 February 2023;
Published: 09 March 2023.
Edited by:
Wenjia Liu, Xi’an Jiaotong University, ChinaReviewed by:
Xiaoxing Kou, Sun Yat-sen University, ChinaCopyright © 2023 Liu, An, Zhu, Tang, Huang, Li, Fu, Chen and Xuan. This is an open-access article distributed under the terms of the Creative Commons Attribution License (CC BY). The use, distribution or reproduction in other forums is permitted, provided the original author(s) and the copyright owner(s) are credited and that the original publication in this journal is cited, in accordance with accepted academic practice. No use, distribution or reproduction is permitted which does not comply with these terms.
*Correspondence: Ji Chen, amltMTE3Y2pAc29odS5jb20=; Kun Xuan, eHVhbmt1bkBmbW11LmVkdS5jbg==
†These authors have contributed equally to this work and share first authorship
‡These authors have contributed equally to this work and share last authorship
Disclaimer: All claims expressed in this article are solely those of the authors and do not necessarily represent those of their affiliated organizations, or those of the publisher, the editors and the reviewers. Any product that may be evaluated in this article or claim that may be made by its manufacturer is not guaranteed or endorsed by the publisher.
Research integrity at Frontiers
Learn more about the work of our research integrity team to safeguard the quality of each article we publish.