- 1Translational Biology, Medicine, and Health Graduate Program, Virginia Tech, Roanoke, VA, United States
- 2Department of Biomedical Sciences and Pathobiology, Virginia Tech, Virginia-Maryland Regional College of Veterinary Medicine, Blacksburg, VA, United States
- 3Center for Emerging, Zoonotic, and Arthropod-borne Pathogens, Virginia Tech, Blacksburg, VA, United States
Introduction: Flaviviruses like dengue virus (DENV) and Zika virus (ZIKV) are mosquito-borne viruses that cause febrile, hemorrhagic, and neurological diseases in humans, resulting in 400 million infections annually. Due to their co-circulation in many parts of the world, flaviviruses must replicate in the presence of pre-existing adaptive immune responses targeted at serologically closely related pathogens, which can provide protection or enhance disease. However, the impact of pre-existing cross-reactive immunity as a driver of flavivirus evolution, and subsequently the implications on the emergence of immune escape variants, is poorly understood. Therefore, we investigated how replication in the presence of convalescent dengue serum drives ZIKV evolution.
Methods: We used an in vitro directed evolution system, passaging ZIKV in the presence of serum from humans previously infected with DENV (anti-DENV) or serum from DENV-naïve patients (control serum). Following five passages in the presence of serum, we performed next-generation sequencing to identify mutations that arose during passaging. We studied two non-synonymous mutations found in the anti-DENV passaged population (E-V355I and NS1-T139A) by generating individual ZIKV mutants and assessing fitness in mammalian cells and live mosquitoes, as well as their sensitivity to antibody neutralization.
Results and discussion: Both viruses had increased fitness in Vero cells with and without the addition of anti-DENV serum and in human lung epithelial and monocyte cells. In Aedes aegypti mosquitoes—using blood meals with and without anti-DENV serum—the mutant viruses had significantly reduced fitness compared to wild-type ZIKV. These results align with the trade-off hypothesis of constrained mosquito-borne virus evolution. Notably, only the NS1-T139A mutation escaped neutralization, while E-V335I demonstrated enhanced neutralization sensitivity to neutralization by anti-DENV serum, indicating that neutralization escape is not necessary for viruses passaged under cross-reactive immune pressures. Future studies are needed to assess cross-reactive immune selection in humans and relevant animal models or with different flaviviruses.
1 Introduction
Zika virus (ZIKV; Genus Flavivirus; Family Flaviviridae) emerged in the western hemisphere in 2013 (Faria et al., 2016) and is estimated to have caused over 100 million infections by 2018 (Moore et al., 2020). ZIKV causes severe pathologies in neonates, including microcephaly and seizures (Musso et al., 2019). One proposed driver of severe disease is pre-existing immunity against dengue virus (DENV; Genus Flavivirus; Family Flaviviridae) (Castanha et al., 2016; Fowler et al., 2018; Zimmerman et al., 2018; Rathore et al., 2019; Carvalho et al., 2020; Castanha et al., 2020; Katzelnick et al., 2021), four genetically and serologically closely related viruses (Barba-Spaeth et al., 2016). As DENV infects roughly five percent of the global population annually (Bhatt et al., 2013), the likelihood of ZIKV infecting a patient with pre-existing DENV immunity is high (Patterson et al., 2016).While pre-existing cross-reactive DENV immunity from antibodies can be protective (Pedroso et al., 2019; Carvalho et al., 2020; Katzelnick et al., 2021), it can also enhance replication (Castanha et al., 2016; Zimmerman et al., 2018; Castanha et al., 2020) and/or disease (Rathore et al., 2019; Carvalho et al., 2020) upon ZIKV infection. Given that these cross-reactive humoral responses play a significant role in disease, their role in ZIKV evolution should also be examined to more fully understand flavivirus evolution.
Immune-driven evolution occurs when the host immune response neutralizes only a subset of viruses, placing selective pressure on the virus population (Marchi et al., 2021); the surviving viruses—which likely have some resistance to the immune pressure—become founders for a subsequent generation (Coffey et al., 2013; Morris et al., 2020). Evolution driven by antibodies has been described for several viruses, including West Nile virus (Sapkal et al., 2011), Nipah virus (Borisevich et al., 2016), chikungunya virus (Jin et al., 2015), influenza (Lambkin et al., 1994; Cleveland et al., 1997; Ferguson et al., 2003; Doud et al., 2018; Lee et al., 2019), SARS-CoV-2 (Sui et al., 2008; Baum et al., 2020; Haslwanter et al., 2021), and many others (Zhao et al., 2004; Zhao et al., 2006; Gal-Tanamy et al., 2008; Rockx et al., 2010; Anthony et al., 2017; Mishra et al., 2020). While attempts have been made to study the impact of cross-reactive immune-driven evolution in ZIKV, these previous studies use monoclonal antibodies (Keeffe et al., 2018), which are a simplistic model for the complex polyclonal human antibody response or study mutations that were not specific to immune selection(Regla-Nava et al., 2022). It is critical that cross-reactive immune-driven evolution be studied since mutations that arise may have implications for transmission (Liu et al., 2017; Liu et al., 2021a) or disease severity (Yuan et al., 2017; Xia et al., 2018; Shan et al., 2020; Liu et al., 2021a).
To address this gap, we examined the effects of cross-reactive antibody selection by passaging ZIKV in the presence of serum from convalescent dengue patients from the Dominican Republic or control serum from dengue-naïve donors from the United States. After passaging, we sequenced the viral populations using next-generation sequencing (NGS). Compared to the virus passaged in the control serum, the premembrane (prM) region of the anti-DENV serum passaged virus was less divergent from the starting virus and had lower non-synonymous diversity. We then examined the anti-DENV serum passaged virus for enriched mutations and engineered two unique mutations using a reverse genetics system. We assessed the impact of these two mutations, E-V355I and NS1-T139A, on fitness in various mammalian cell lines and Aedes aegypti mosquitoes and their sensitivity to neutralization by anti-DENV immune serum. Notably, both mutations had increased fitness in mammalian cell culture and reduced fitness in live mosquitoes. These results align with the trade-off hypothesis, which states that multi-host viruses that adapt to one host lose fitness in the other hosts (Wilson and Yoshimura, 1994; Kassen, 2002). When their neutralization sensitivity was assessed, NS1-T139A escaped neutralization, but E-V355I was more sensitive to neutralization. These results demonstrate that neutralization escape is not necessary for viruses that have been passaged in cross-reactive immune environments. As a whole, these results suggest that pre-existing immunity may play a significant role in ZIKV evolution.
2 Methods
2.1 Cells and viruses
We obtained Vero cells (CCL-81) and U937-DC-SIGN cells (CRL-3253) from the American Type Culture Collection (ATCC). HEK293A cells were kindly provided by Dr. Jamie Smyth from the Fralin Biomedical Research Institute. A549 cells were kindly provided by Dr. Nisha Duggal from the Virginia-Maryland College of Veterinary Medicine. All cells were maintained at 37°C with 5% CO2. Vero, HEK293A, and A549 cells were cultured in Dulbecco’s modified Eagle’s medium (DMEM) supplemented with 5% fetal bovine serum (FBS), 1% nonessential amino acids, and 0.1% gentamicin. We cultured U937-DC-SIGN cells in Roswell Park Memorial Institute medium (RPMI-1640) supplemented with 2 mM L-glutamine, 5% FBS, 1% nonessential amino acids, 0.1% gentamicin, and 0.05 mM 2-mercaptoethanol. The ZIKV strain used was derived from an infectious clone of strain PRVABC59 (Weger-Lucarelli et al., 2017) and was rescued and passaged once in Vero cells (p1). DENV1 strain R99142 and DENV2 strain PUO-218 were obtained from the CDC. DENV3 strain BC188/97 (NR-3801) and strain DENV4 703-4 (NR-48801) were obtained from the Biodefense and Emerging Infections Research Resources Repository (BEI Resources). West Nile virus (WNV) Kunjin strain SW28919 and yellow fever virus (YFV) strain 17-D were obtained from the University of Texas Medical Branch World Reference Center for Emerging Viruses and Arboviruses.
2.2 Serum sources
All human samples were de-identified by their respective sources prior to purchase. We obtained serum samples from convalescent dengue patients from the Dominican Republic who tested positive for DENV and negative for ZIKV by ELISA via Boca Biolistics, LLC. These samples were referred to as anti-DENV patients A-D. The serum from the four anti-DENV patients was subsequently pooled at equal volumes (hereafter called the anti-DENV pool). Control serum, derived from blood donors from Kentucky, USA, was from Valley Biomedical Products and Services, INC.
2.3 Plaque reduction neutralization test (PRNT)
Serum samples were serially diluted in RPMI-1640 with 10 mM HEPES and 2% FetalPure bovine serum (Genesee Scientific 25-525H), hereafter referred to as viral diluent. Serum samples were mixed with 800 plaque-forming units per mL (PFU/mL) of the virus of interest. The mixture was then incubated at 37°C for one hour, and the virus-serum mixture was used to inoculate wells in a confluent 24-well plate of Vero cells. After a one-hour adsorption period, plaque assay overlay media was added to each well, as previously described (Liu et al., 2021b). We fixed the plates four to seven days later, depending on the virus. We defined the PRNT50 as the highest reciprocal dilution that neutralized the virus by at least 50%.
2.4 Viral passaging
Vero cells were plated to an 80-90% confluency in 24-well plates. On the day of the infection, we mixed ZIKV with an equal volume of the appropriate serum (1:40 dilution of serum). The virus-serum mixtures were incubated for one hour at 37°C and then used to inoculate the Vero cells. After the one-hour adsorption period, the cells were washed in phosphate-buffered saline (PBS); we then added fresh media supplemented with the appropriate human serum. Cells were monitored every 12 hours, and the supernatant was harvested once the cells demonstrated >75% cytopathic effect (CPE), as assessed by visual inspection. Harvested supernatant was stored at -80°C until they were titered plaque assay. This process was repeated for a total of five passages, where the multiplicity of infection (MOI) for each passage was maximized based on the available titer (.01-1). These experiments were performed in triplicate, producing three unique lineages for each serum condition.
2.5 Library preparation and next-generation sequencing (NGS) analysis
We prepared libraries of the unpassaged virus, virus passaged in the anti-DENV pool, and virus passaged in the control serum. To enrich for encapsidated virus and to remove nucleic acids, 120 µL of viral supernatant was mixed with 15 µL of 250 units/mL of Benzonase (Millipore Sigma E1014-5KU) diluted in 10x Benzonase Buffer (200 mM Tris-Cl [pH 7.5], 100 mM NaCl, 20 mM MgCl2) (Depew et al., 2013; Berg et al., 2016; Rodgers et al., 2017) and 15 µL of 250 units/mL of RNAse A (Millipore Sigma 10109142001) (Aryani and Denecke, 2015). Samples were then incubated at 37°C for three hours. RNA was extracted from the samples using the Zymo Quick-RNA Viral Kit (R1035). After extraction, we purified the samples using a 0.8x bead selection with the sparQ PureMag magnetic beads (95196-005) to remove small RNA fragments. First-strand cDNA synthesis was performed using random nonamers and the Maxima H- Reverse Transcriptase kit (EP0751). We synthesized second-strand cDNA using the Q5 High-Fidelity 2X Master Mix (M0492S) (Bates et al., 2021). NGS Libraries were then produced using the sparQ DNA Frag & Library Prep Kit from Quantabio (Cat. 95194-024). Samples were sequenced using 150 bp paired-end reads on the Illumina Novaseq 6000 (Novogene Co., Ltd.). Bioinformatic analysis was performed as previously described (Marano et al., 2022; Roesch et al., 2022). Briefly, adapters and bases with <Q30 were trimmed using Bbduk (Bushnell, 2014); the remaining reads were mapped to the ZIKV reference using the Burrows-Wheeler Aligner (BWA)(Li and Durbin, 2009). Variants were called with LoFreq (Wilm et al., 2012), and new consensus sequences were produced using the Genome Analysis Toolkit (GATK) (McKenna et al., 2010). To perform diversity and selection analysis, we used SNPGenie (Nelson et al., 2015) to calculate the non-synonymous (πN) and synonymous (πS) diversity. To determine the divergence of passaged samples, we generated an all-site VCF file using bcftools (Danecek et al., 2021) and calculated Dxy using pixy (Korunes and Samuk, 2021).
2.6 Generation and rescue of single mutant viruses by bacteria-free cloning
Mutagenic PCR primers were designed in silico to introduce the identified mutations using SnapGene 6.0.2 software (GSL Biotech). We performed PCRs using the SuperFi II Master Mix (Thermo Fisher 12368010), and the products were gel purified using the Machary-Nagel NucleoSpin Gel and PCR Clean-up kit (740609). We assembled the products using the NEB Builder HiFi DNA Assembly Master Mix (E2621L). The assemblies were digested with DpnI (R0176S), Lambda exonuclease (M0262S), and Exonuclease I (M0293S) and amplified using SuperPhi RCA Premix Kit with Random Primers (Evomic Science catalog number PM100) (Bates et al., 2020). As previously described, RCA products were then transfected into HEK293A cells to produce p0 stocks of the virus (Marano et al., 2020; Marano et al., 2022). The p0 stocks were then used to infect Vero cells at an MOI of 0.01 to produce p1 stocks, which were used for all downstream tests. We sequenced the p1stocks of the mutant viruses derived from the anti-DENV passaged population using both Sanger sequencing and next-generation sequencing to confirm the mutation of interest was introduced. The p1 stocks of the mutant viruses derived from the control passaged population were sequenced by Sanger sequencing to confirm the mutation was properly introduced.
2.7 In vitro competition assays
Competition assays were performed similarly to previously described methods (Marano et al., 2022). Briefly, the mutant and wild-type viruses were mixed at a 1:1 PFU ratio to prepare the competition mixes. This mix was then confirmed by plaque assay, and we amplified a PCR amplicon around the mutation site using extraction-free one-step RT-PCR (Genoud et al., 2021) with qScript XLT One-Step RT-PCR Kit (95143-200). We then purified the amplicon and submitted it for Sanger sequencing, and then assessed the ratio of each virus from the resulting chromatograms using QSVanalyzer (Carr et al., 2009). After confirmation, we used the mixes to infect cells at an MOI of 0.01 for each virus. For Vero and A549 cells, virus mixes were added to the cells, and after one-hour adsorption, cells were washed with PBS, and fresh media was added. For the Vero cells with serum supplementation experiments, the mixes were treated identically to the passaging experiments described above. For U937-DC-SIGN cells, we first centrifuged the cells, removed the media, and the cells were resuspended in the diluted virus mix (Fowler et al., 2018). After a two-hour adsorption period, we washed cells with PBS before adding fresh growth media (Fowler et al., 2018). Virus was harvested at 2 (U937-DC-SIGN), 3 (Vero and A549), or 4 (Vero with convalescent DENV serum) days post-infection (dpi). Extraction-free RT-PCR and Sanger sequencing were performed on all samples, and the data was analyzed using QSVanalyzer (Liu et al., 2021a). Relative fitness was calculated as W(t) = F(t)/F(0), where F(t) is defined as the ratio of the mutant virus following the competition and F(0) is defined as the ratio of the mutant virus at baseline (Liu et al., 2021a). We considered the mutant to have increased fitness in the tested environment if W>1. In contrast, we considered the mutant virus to have reduced or no fitness change if W<1 or W=0, respectively.
2.8 Mosquito rearing and in vivo competition assays
We reared Aedes aegypti Poza Rica mosquitoes using previously published methods (Weger-Lucarelli et al., 2016). Briefly, mosquitos were maintained at 28°C with a relative humidity of 75% and a 12:12 (light/dark) photoperiod. During larval stages, mosquitoes were maintained on ground Nishikoi fish food. Adult mosquitoes were given 10% sucrose solution ad libitum via cotton balls. Mosquitoes were separated from the colony 6 – 8 days post eclosion at a 5:1 ratio of females to males into disposable 16 oz containers. Mosquitoes were starved of glucose and water for 24 hours before the infectious blood meal of defibrinated sheep’s blood. Mosquitoes were fed for one hour using the Hemotek membrane feeder system (SP4W1-3). After feeding, mosquitoes were anesthetized at 4°C, and females fed to repletion were separated into a new container. We maintained these mosquitoes for ten days under rearing conditions with sucrose ad libitum. Mosquitoes were then anesthetized using triethylamine, and whole bodies were collected in viral diluent supplemented with 50 µg/mL gentamicin and 2.5 µg/mL of amphotericin B. Samples were processed by adding a single sterile metallic bead per tube, homogenizing the samples at 30 freq/s for 2 min using the Qiagen TissueLyser II (85300), and diluting the samples 1:5 in viral diluent. We used the extraction-free RT-PCR, Sanger sequencing, and QSVanalyzer workflow described above to analyze the samples. The “naïve” blood meal consisted of defibrinated sheep’s blood, 106 PFU/mL of the competition mix, and 0.5 µM ATP. For the “immune” blood meal, we pretreated the virus for one hour by incubating it with anti-DENV serum, but other conditions were unchanged.
2.9 Statistical analysis
Statistical analysis was performed in Prism 9 (GraphPad, San Diego, CA, USA). A two-way ANOVA with Šídák’s correction for multiple comparisons was used to compare the divergence and non-synonymous diversity (πn) within the antibody binding regions of the passaged populations. A two-way ANOVA with Dunnett’s correction for multiple comparisons was used to compare the neutralization of the mutant viruses to the wild type and the growth kinetics of the passaged populations. For in vitro competition assays, a Shapiro-Wilk test for normality was performed to ensure normality, and then a one-sample t-test was performed. For the in vivo competition assays, a Fisher’s exact test was used to determine which species (mutant or WT) would become dominant in a population of mosquitoes.
3 Results
3.1 Donor serum characterization
We first evaluated the serostatus of the donors from the Dominican Republic (referred to as anti-DENV patients A-D) and the US (Kentucky; hereafter referred to as control serum) by performing plaque reduction neutralization tests (PRNTs) against DENV and ZIKV. Donor serum from the United States was used as the control serum because nearly all adults in the Dominican Republic are seropositive for anti-DENV antibodies (Yamashiro et al., 2004). Data are presented as the reciprocal serum dilution which resulted in a 50% reduction in plaques (PRNT50) (Table 1). Since closely related flaviviruses are known to be cross-neutralized by convalescent serum, we defined the infecting virus as having a PRNT50 ≥ 4-fold higher than the other viruses within the group (Sasmono et al., 2018; Sharp et al., 2019). Based on these parameters, we concluded that all anti-DENV donors had a history of DENV infection without prior ZIKV infection. Furthermore, we concluded that the control serum donors had no history of infection with either DENV or ZIKV.
To increase antibody diversity, we pooled the four anti-DENV donors (hereafter referred to as the anti-DENV pool) at equal volumes. We then tested the pool’s neutralization of DENV1-4 and ZIKV, as well as yellow fever virus (YFV) and West Nile virus (WNV) (Table 2). The cross-reactive PRNT50 of the anti-DENV pool against ZIKV was 40, which represents an intermediate between the specific neutralization from the convalescent ZIKV patient (PRNT50 = 320) and the control serum (PRNT50 < 20). Thus, we generated an anti-DENV pool with a history of DENV infection but no previous exposure to ZIKV, WNV, or YFV.
3.2 Passaging virus in the presence of anti-DENV serum
To assess the impact of convalescent dengue serum on ZIKV evolution, we adapted previously reported immune-driven evolution protocols (Borisevich et al., 2016; Lee et al., 2019). Specifically, we mixed ZIKV at a 1:1 ratio by volume with either the anti-DENV pool at the PRNT50 concentration (1:40 dilution) or control serum at the same dilution. After one hour of incubation, we infected Vero cells with the virus-serum mixtures. After adsorption, we washed the cells and maintained them with media supplemented with anti-DENV pool or control serum. We monitored the cells and harvested the supernatant when we observed >75% cytopathic effect (CPE), as determined by visual inspection. We then titered the harvested virus (Figure 1A) and used it for a subsequent passage. By the fifth passage, we observed that the time to produce CPE in the anti-DENV pool passaged virus increased by 1.5 days from the initial passage, while the control serum passaged virus decreased by 0.5 days (Figure 1B). Given this phenotypic change, we next sequenced the virus populations.
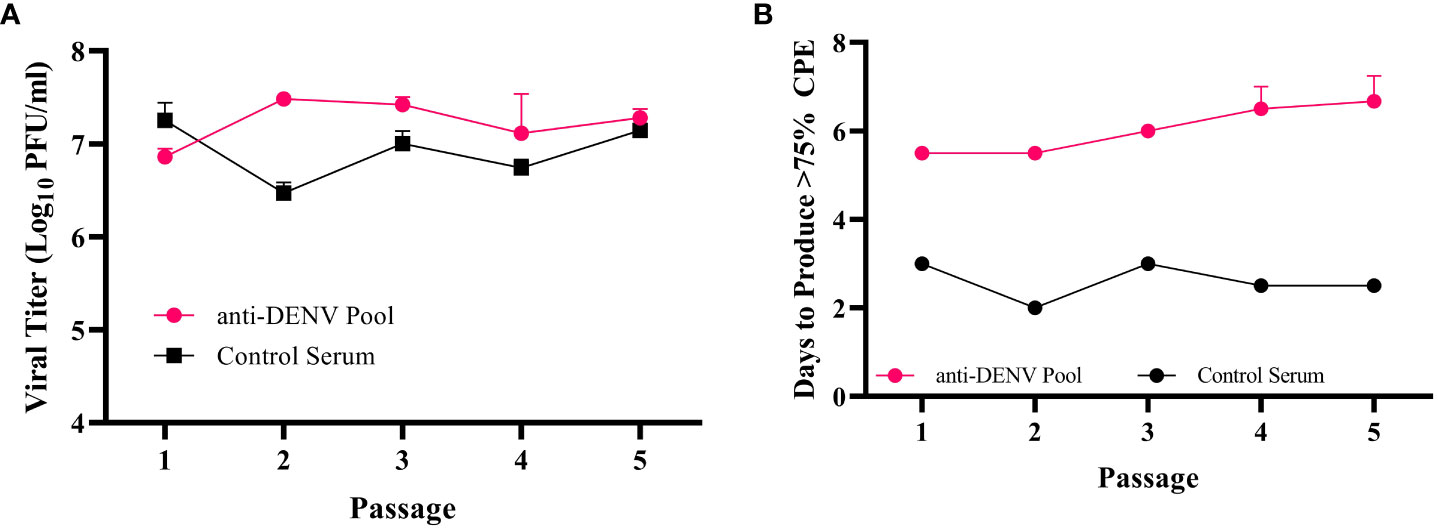
Figure 1 Zika virus (ZIKV) passaging workflow. (A) The post-passage titers of the anti-DENV pool and control serum passaged virus, as measured by plaque assay. Data represent the three independent lineages within each passaging condition (anti-dengue virus (DENV) Pool or Control Serum) (B) During passaging, viral supernatant was harvested when >75% of cells demonstrated cytopathic effects (CPE). The days post-infection where this occurred were recorded for each passage. Data represent the three independent lineages within each passaging condition (anti-dengue virus (DENV) Pool or Control Serum).
3.3 Population divergence, diversity, and mutation selection
We next sought to determine the evolutionary impact of passaging ZIKV in convalescent dengue serum, hypothesizing that the viral populations would differ significantly from the virus passaged in control serum. To this end, we performed Illumina next-generation sequencing (NGS) on viral RNA from the unpassaged virus, the control serum passaged virus, and the anti-DENV pool passaged virus. We included the unpassaged virus to establish an accurate starting consensus sequence. By sequencing the virus passaged in the control serum and the anti-DENV pool, we could differentiate between mutants derived from passaging ZIKV in Vero cells with human sera (control) and the impact of passage in the presence of dengue convalescent serum.
For our analysis, we focused on the regions of known antibody binding: premembrane (prM), envelope (E), and nonstructural protein 1 (NS1) (Vaughan et al., 2010). We examined the average nucleotide distance (divergence) between our unpassaged and passaged viruses (Figure 2A) and the non-synonymous diversity, πn (Figure 2B), within the antibody binding regions. We observed significantly lower divergence (p = 0.043) and non-synonymous diversity (p = 0.001) in the prM region of the anti-DENV pool passaged virus compared to the control serum passaged virus. These results suggest that selection by anti-DENV serum limited the divergence and diversification of the prM protein.
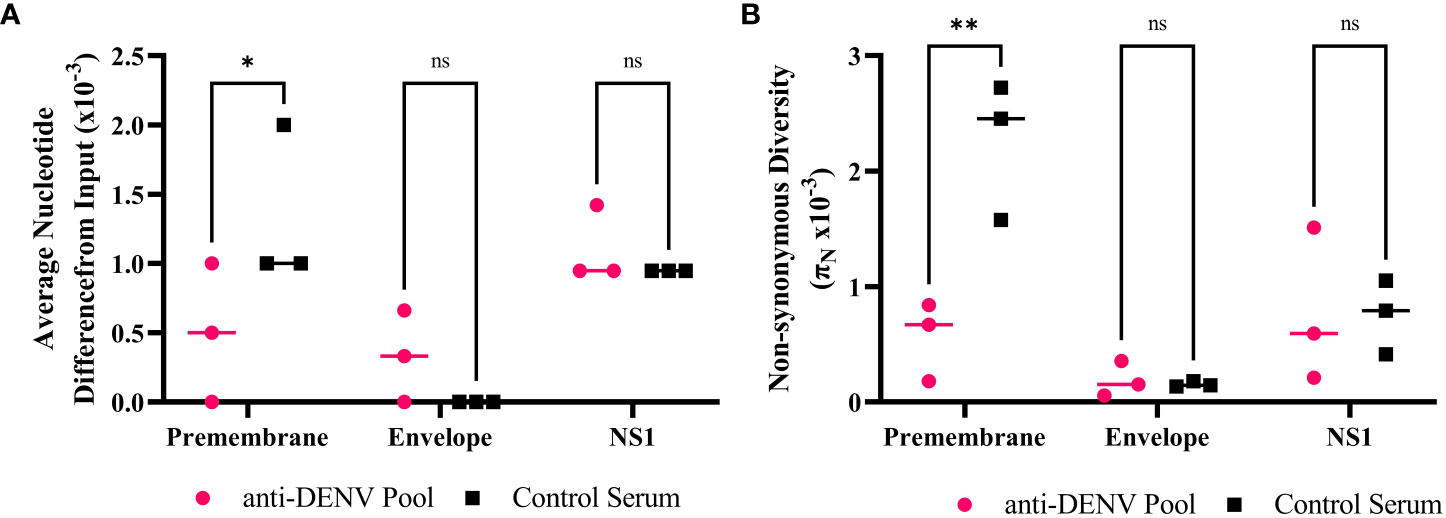
Figure 2 Anti-dengue virus (DENV) immune selection limits Zika virus (ZIKV) divergence and nonsynonymous diversity in the premembrane protein. (A) Divergence within the antibody binding region of passaged samples compared to the input virus. Each point represents the sequencing data from each passage 5 biological replicate. (B) Comparing the πn values at each protein with the antibody binding region of the passaged populations. Each point represents the sequencing data from each passage 5 biological replicate. Statistics were performed using a two-way ANOVA with a Šídák's correction for multiple comparisons (* p = 0.043 ** p = 0.001). NS – nonstructural protein.
We next examined the antibody binding region for single nucleotide variants enriched after passaging in the presence of convalescent dengue serum. To prioritize variants, we used an allelic frequency cutoff of 0.1 as our focus was on higher frequency mutations, which we expected would be more likely to have a phenotypic impact on the virus. Of the 43 mutations identified across the input virus and the passaged populations at an allelic frequency ≥ 0.1 (Supplementary Tables 1-3), five non-synonymous mutations were found exclusively in the anti-DENV pool passaged virus within the antibody binding region and had increased allelic frequency (Table 3). We selected two mutations to construct within the envelope (E), E-V355I, and NS1, NS1-T139A (Figure 3A), regions which have been shown to mediate cell entry (Fontes-Garfias et al., 2017) and alter infectivity in mosquitoes (Liu et al., 2017; Kuo et al., 2020), respectively.
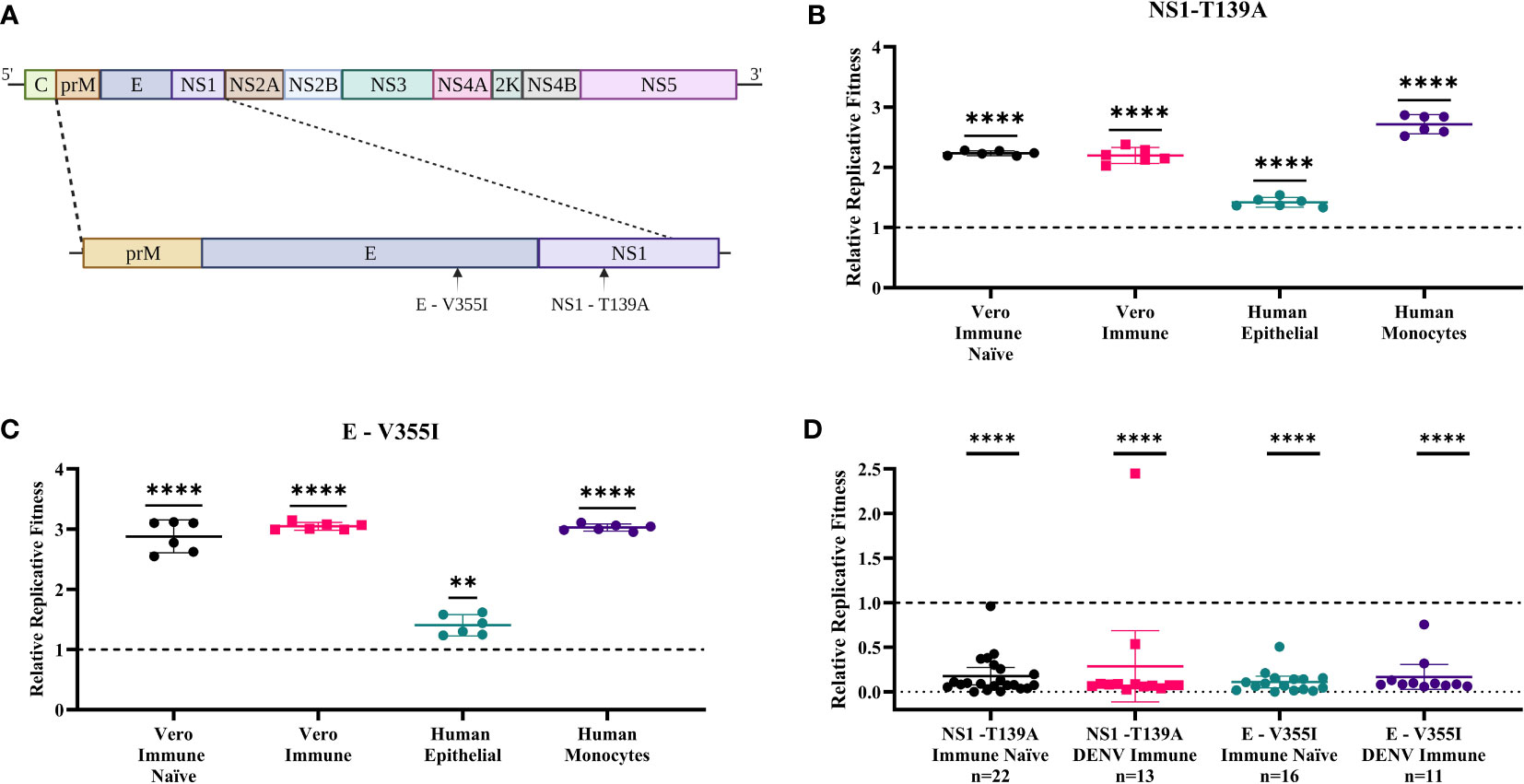
Figure 3 Zika virus (ZIKV) mutants have enhanced fitness in mammalian cells and reduced fitness in mosquitoes. (A) Two mutations were selected (E-V355I and NS1-T139A) to assess replicative fitness. WT virus was mixed with each mutant virus at a 1:1 PFU ratio and used to infect cell lines (MOI=0.01) and mosquitoes (106pfu/ml). Post-infection, virus was harvested and sequenced. The relative fitness was calculated by comparing the proportion of the mutant virus before and after infection. (B, C) In vitro competition assays. B) NS1- T139A and C) E- V355I represent results on cell lines (t-test represented by **** p< .0001 and ** p = .0021). (D) Represent results in mosquitoes. Each data point represents the whole-body homogenate of a single mosquito, and the error bars represent the standard deviation from the mean of pooled fitness across all mosquitoes (N represents the number of mosquitoes) from two independent experiments (Fisher’s exact test represented by **** p < .0001).
3.4 Mutants identified during passage in convalescent dengue serum have increased fitness in mammalian cell culture
To assess the impact of the mutations on replicative fitness, we performed competition assays comparing the mutants to WT ZIKV. We mixed mutant and wild-type viruses at a 1:1 PFU ratio and then used the mixes to infect several cell lines. To calculate the fitness of the mutant virus in each environment, we compared the relative proportion of the mutant virus pre- and post-infection (Liu et al., 2021a; Liu et al., 2022a; Liu et al., 2022b). We tested four cell culture environments: Vero cells without supplementation of anti-DENV serum, representing an immune naïve model (Immune Naïve); Vero cells supplemented with the anti-DENV pool, representing a dengue immune model (Immune); A549 cells representing a human epithelial cell model (Human Epithelial); U937-DC-SIGN cells, representing a human monocyte model (Human Monocyte). These cell lines were selected as they previously were shown to be susceptible to ZIKV infection and represent cell types critical to pathogenesis (Hamel et al., 2015; Khaiboullina et al., 2017; Fowler et al., 2018). The NS1-T139A (Figure 3B) and the E-V355I mutant (Figure 3C) had increased fitness compared to WT ZIKV in all environments tested.
3.5 Mutants identified during passage in convalescent dengue serum have reduced fitness in mosquitoes
Because ZIKV is transmitted between mammalian and insect hosts, it is critical to test the fitness of these immune-selected mutants in mosquitoes to assess the likelihood that these mutations would be maintained in nature. To that end, we fed Aedes aegypti an artificial blood meal containing the WT/mutant competition mixes used in the previous studies. To mimic the impact of a prior DENV infection, we also performed an experiment where we pretreated the virus with the anti-DENV serum pool before the blood meal. We then collected mosquitoes at ten days post-feeding, and whole-body homogenates were tested to determine the proportion of each virus compared to the original blood meal (Figure 3D). Regardless of host immune status, the mutant viruses had significantly reduced fitness compared to WT ZIKV in mosquitoes (p<.0001).
3.6 Neutralization escape is not required for fitness enhancement during cross-reactive immune selection
To understand the impact of immune selection on neutralization sensitivity, we performed PRNTs on the ZIKV mutants using the anti-DENV pool (Figure 4). The anti-DENV pool neutralized wild-type ZIKV at a PRNT50 of 40, similar to what we found previously (Table 2). The mutants showed polarized neutralization susceptibilities; specifically, the anti-DENV pool no longer neutralized NS1-T139A (PRNT50 <20), indicative of escape, while E-V355I was slightly more sensitive to neutralization than the WT (PRNT50 = 80 p <.0001). When we examined the neutralization sensitivity of the populations from which these mutants were derived, we saw neither escape nor sensitization phenotypes (Figure 5A). These results suggest that cross-reactive antibody selection can generate mutants with enhanced sensitivity (E-V355I) and reduced sensitivity (NS1-T139A) to antibody selection and that neutralization escape is not necessary for fitness enhancement during cross-reactive immune selection. To further contextualize the results, we generated two mutants from mutations identified in the control population (Supplemental Table 3): prM-S109P and prM-M159V. These mutations were selected because we observed higher genetic divergence and diversity in the prM protein of the control population compared to the anti-DENV pool population (Figures 2A, B). Both mutants from the control serum passaged population showed greatly enhanced sensitivity to neutralization by the anti-DENV pool (PRNT50 > 640). This behavior was also observed in the passaged populations that these mutants were derived from (Figure 5A). These results indicate that passaging in anti-DENV serum protected ZIKV from taking on these extreme neutralization phenotypes. When we assessed the replication of the passaged populations in Vero cells, we observed that the control serum passaged virus had increased replicative fitness (Figure 5B). In contrast, when we tested the passaged populations in Vero cells supplemented with the anti-DENV pool, we observed that the control serum passaged virus did not replicate (Figure 5C). The phenotypes observed for both NS1-T139A or E-V355I were not reflected at the population level (Figures 5A–C).
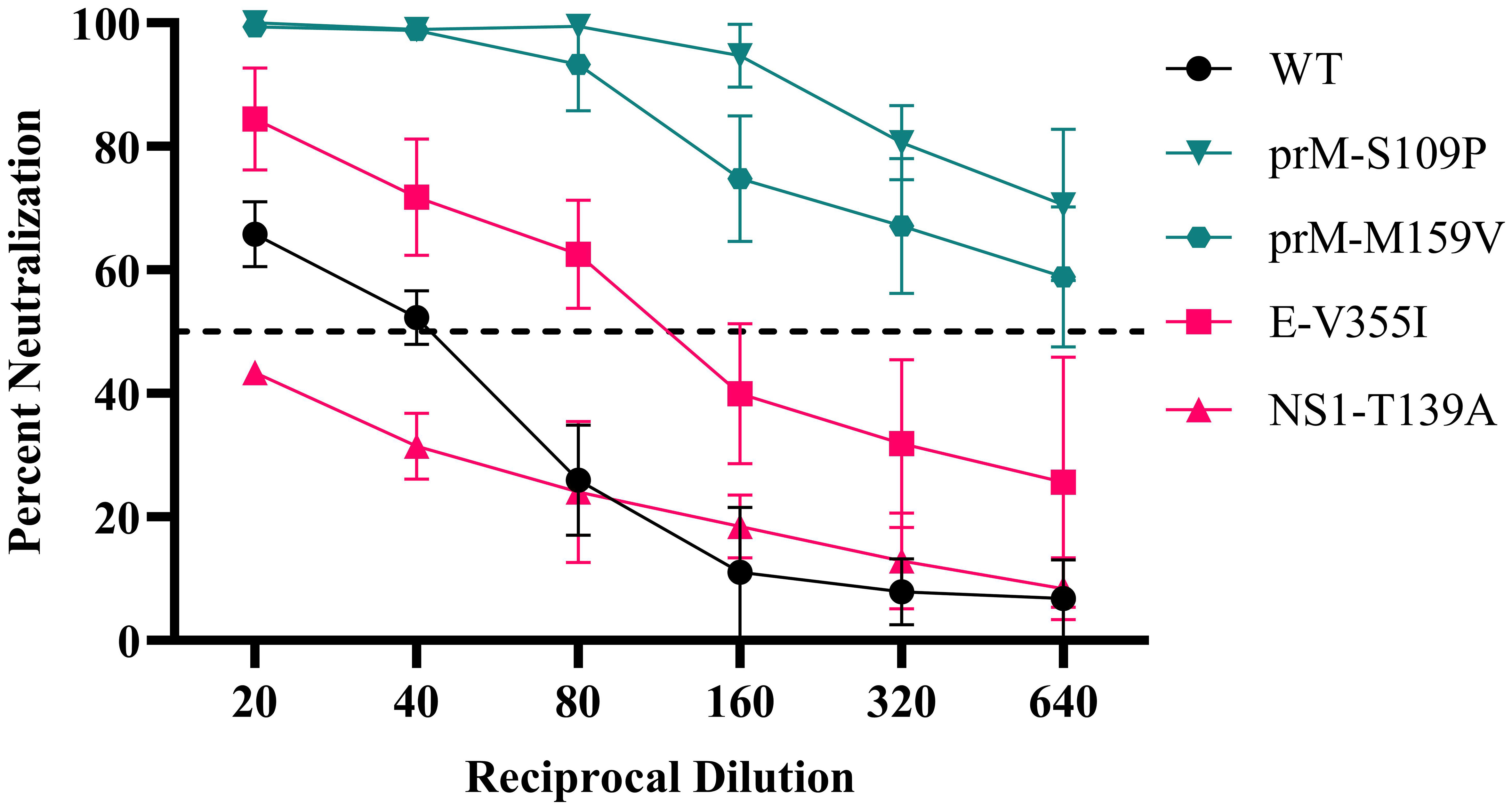
Figure 4 ZIKV mutant neutralization sensitivity. anti-DENV pool were serially diluted and mixed with WT ZIKV (black line), mutants from the anti-DENV pool population (red lines), or mutants identified from the Control Serum-passaged population (green lines). Data are from two biological replicates, each with three technical replicates, and the error bars represent the standard deviation from the mean. The dotted line represents the limit of detection.
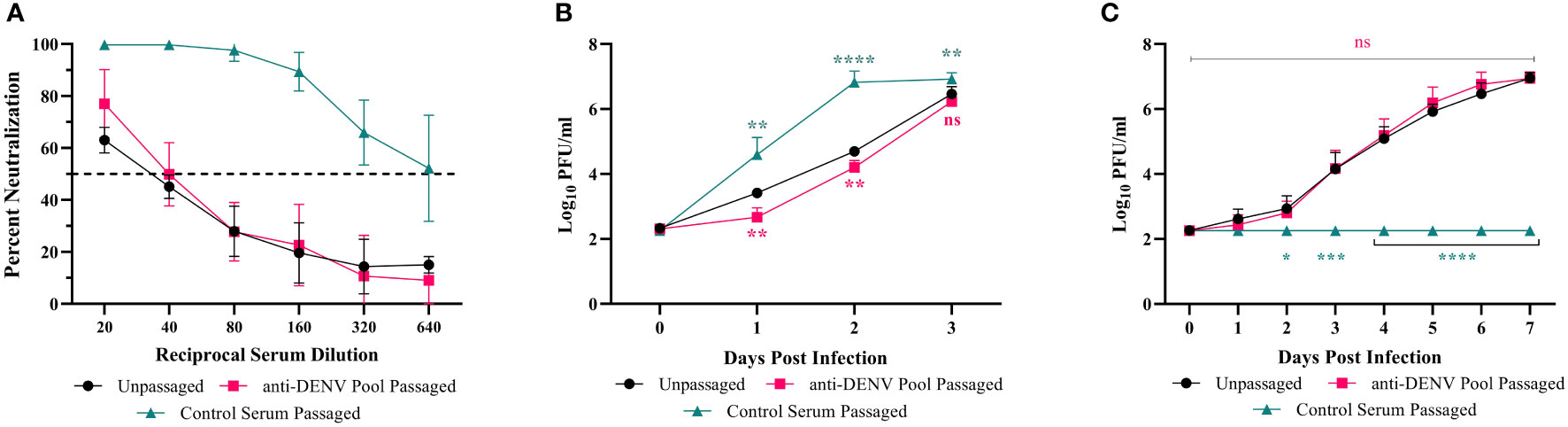
Figure 5 Neutralization dynamics and replicative fitness of passaged populations. WT ZIKV (black line), passage 5 of the anti-DENV pool passaged population (red lines), or passage 5 of the Control Serum-passaged population (green lines) were tested for neutralization sensitivity and replication. Data are from two biological replicates, each with three technical replicates, and the error bars represent the standard deviation from the mean. (A) The anti-DENV serum pool was serially diluted and mixed with 800 PFU/mL of each virus, and percent neutralization was recorded at each dilution. (B, C) Vero cells were either infected at an MOI of .01 with untreated virus (B) or virus incubated with the anti-DENV pool for 1 hour. Statistics were performed using a two-way ANOVA with a Dunnett’s correction for multiple comparisons comparing the passaged viruses to the unpassaged virus (ns - nonsignificant, * - p = 0.0139, ** - p = 0.001, *** - p = 0.005, **** - p < 0.0001).
4 Discussion
The recent ZIKV outbreak in the Americas was associated with several adaptive mutations that potentially facilitated its rapid emergence (Liu et al., 2017; Yuan et al., 2017; Xia et al., 2018; Shan et al., 2020; Liu et al., 2021a), though the driver of these mutations is unknown. One potential driver of ZIKV evolution is selection mediated by pre-existing adaptive immune responses to closely related flaviviruses such as DENV. While immune-driven evolution has been well-characterized for other viruses (Lambkin et al., 1994; Cleveland et al., 1997; Ferguson et al., 2003; Zhao et al., 2004; Zhao et al., 2006; Gal-Tanamy et al., 2008; Sui et al., 2008; Jin et al., 2015; Borisevich et al., 2016; Anthony et al., 2017; Doud et al., 2018; Lee et al., 2019; Mishra et al., 2020), previous studies with ZIKV have some limitations. Regla-Nava et al. sought to examine how pre-existing immunity to DENV affected the evolution and pathology of ZIKV (Regla-Nava et al., 2022). Using a passaging system in which they oscillated ZIKV infection between mosquito cells and dengue-naïve or dengue-immune mice, they identified a mutant, NS2B I39V, that appeared to escape pre-existing dengue immunity, and increased viral loads, adult and fetal mortality, and mosquito infection (Regla-Nava et al., 2022). However, NS2B I39V occurred in both the experimental and control populations; therefore, the mutation was likely not driven by immune pressure. Interestingly, we also found NS2B I39V in our anti-DENV passaged population (Supplemental Table 2). Thus, there remained a gap in understanding the specific impact of cross-reactive immune selection on ZIKV evolution. To fill this gap, we passaged ZIKV in the presence of serum from convalescent dengue patients or dengue naïve serum and then studied the evolutionary and fitness consequences on the virus. Within the population passaged in the anti-DENV pool, we identified two mutations within the dominant antibody binding region, NS1-T139A and E-V355I. Of note, ZIKV E-355I has been previously isolated from a human in Brazil (NCBI Accession OL423668) (Hadfield et al., 2018). While there is a single report of a DENV4 isolate containing E-355I (NCBI Accession KT276273), NS1-T139A has not previously been detected in either ZIKV or DENV (Hadfield et al., 2018). When the mutants were assessed, they demonstrated altered sensitivity to antibody neutralization, increased fitness in mammalian cells, and reduced fitness in Aedes aegypti mosquitoes. While these findings did not support our initial hypothesis regarding only generating escape mutants, they did align with our hypothesis regarding fitness trade-offs.
Immune selection provides a complex pressure as there is both the enrichment for escape mutations (diversifying selection) within a population resistant to neutralization (Lambkin et al., 1994; Cleveland et al., 1997; Ferguson et al., 2003; Zhao et al., 2004; Zhao et al., 2006; Gal-Tanamy et al., 2008; Rockx et al., 2010; Sapkal et al., 2011; Jin et al., 2015; Borisevich et al., 2016; Anthony et al., 2017; Doud et al., 2018; Lee et al., 2019) and the removal of genotypes sensitive to neutralization (purifying selection) (Hu et al., 2021). Two critical aspects of this selection are genetic diversity (Lee et al., 2015) and divergence (MacLean et al., 2021): we observed higher genetic divergence and diversity within the prM protein in the control serum-passaged population compared to the virus passaged in the anti-DENV pool. Our results suggest that anti-DENV serum likely tempered the ability of ZIKV to diverge during passaging. When we generated two mutants found in the control population, prM-S109P and prM-M159V, we observed that both were highly sensitive to neutralization by the anti-DENV pool, suggesting that the prM protein may be important for ZIKV neutralization by dengue convalescent serum.
Given previous results, we hypothesized that immune-driven evolution would generate neutralization escape mutants (Zhao et al., 2004; Borisevich et al., 2016; Lee et al., 2019), as we observed with NS1-T139A; in contrast, E-V355I was more sensitive to neutralization by the anti-DENV pool than WT ZIKV. The overall fitness benefit conferred by the E- V355I mutation in all mammalian environments tested (Figure 4) may explain this paradox as suggested by Blish et al. for immune selected gp41 mutants of HIV(Blish et al., 2008). The escape phenotype produced by a nonstructural protein mutant, NS1-T139A, may also appear unexpected since nonstructural proteins are generally not found extracellularly. NS1, however, exists as an intracellular monomer, a membrane-bound dimer, and an extracellular hexamer (Rastogi et al., 2016). During infection, secreted NS1 disrupts endothelial junctions, resulting in tissue permeabilization and vascular leakage (Puerta-Guardo et al., 2019; Puerta-Guardo et al., 2022), so neutralizing NS1 may be an important protective strategy. Indeed, passive transfer studies using serum from mice vaccinated with NS1 protected against a lethal ZIKV challenge (Bailey et al., 2019). These results further demonstrate NS1’s importance as a target for antibody selection. NS1-T139A also increased fitness in vitro in mammalian cells, possibly due to its ability to “prime” cells for infection, resulting in increased viral replication (Alcon-LePoder et al., 2005; Avirutnan et al., 2007; Crook et al., 2014).
While the mutations identified following passage in the presence of anti-DENV serum enhanced fitness in mammalian cell culture, they significantly reduced fitness in live mosquitoes. These results align with the trade-off hypothesis (Wilson and Yoshimura, 1994; Kassen, 2002), which states that as an arthropod-borne virus, or any virus in a multi-host system, adapts to one of its hosts, it may lose fitness in the other host(s) (Wilson and Yoshimura, 1994; Kassen, 2002). These data suggest that while mutants arise following exposure to cross-reactive antibodies in a DENV-immune person, if wild-type virus remains within the population, they might be removed from the population upon mosquito infection, thereby limiting the mutant’s spread.
Limitations of the study: We used only a single post-epidemic strain of ZIKV and convalescent serum from the Dominican Republic. We may have observed different results using different viruses or serum samples. Furthermore, by pooling samples by neutralization strength instead of by equal volumes, therefore preventing a given serum sample from overpowering the pool, we may have also observed different results. We focused only on humoral immunity generated from natural infection. However, cross-reactive T cells, which have been shown to play a role in protection against ZIKV (Regla-Nava et al., 2018) and to drive the evolution of other viruses (Ciurea et al., 2001; Cao et al., 2003; Bowen and Walker, 2005; Zompi et al., 2012; Agerer et al., 2020), should also be examined as a driver of ZIKV evolution. Our passaging approach involved only mammalian cells, representing the multiple rounds of replication within a single host; however, an alternating passaging system between mosquito and mammalian cells could also have been used to mimic the natural transmission cycle (Deardorff et al., 2011; Talavera-Aguilar et al., 2021; Regla-Nava et al., 2022). Finally, we generated only single mutants; however, we could have engineered mutant viruses with multiple mutations to assess potential epistatic interactions, which would explain the differences observed between the mutant viruses and the populations (Ferreira et al., 2021).
Conclusions: We demonstrated that cross-reactive selection in the presence of anti-DENV antibodies alters ZIKV evolution and fitness. Specifically, we found that passaging ZIKV in mammalian cells with anti-DENV antibodies results in the generation of mutants with altered sensitivity to neutralization. Notably, passaging ZIKV in the presence of anti-DENV serum constrained the evolution of the virus population compared to control populations, which became highly sensitive to neutralization and more fit in Vero cells. However, the mutants from populations passaged in the presence of anti-DENV serum had increased fitness in mammalian cells. This is possibly due to epistatic interactions reducing fitness. We observed a significant reduction in fitness for both mutants in mosquitoes, consistent with the trade-off hypothesis. These results improve our understanding of the drivers of ZIKV evolution and suggest that future work is needed to more fully dissect the evolutionary implications of inter-flavivirus immune interactions, including inter-serotype interactions with DENV and viruses within the Japanese encephalitis complex [As reviewed (Rathore and St. John, 2020)].
Data availability statement
The datasets presented in this study can be found in online repositories. The names of the repository/repositories and accession number(s) can be found in the article/Supplementary Material.
Author contributions
Conceptualization, JW-L; methodology, JM and JW-L; cloning, JM; characterization, JM; formal analysis, JM and JW-L; validation, JM; writing—original draft preparation, JM; writing—review and editing, JM and JW-L; visualization, JM and JW-L. All authors contributed to the article and approved the submitted version.
Funding
This work was supported by seed grant funding from Virginia Tech’s Center for Emerging and Zoonotic Pathogens (CeZAP) and Virginia College of Osteopathic Medicine (VCOM) One Health seed funds awarded to JW-L.
Acknowledgments
Figure 3A was generated using BioRender. The Virginia Tech Genomic Sequencing Center performed Sanger sequencing. The Center for Biostatistics and Health Data Science provided statistical support. We thank Christina Chuong and Kelsey Marano for editing the manuscript.
Conflict of interest
The authors declare that the research was conducted in the absence of any commercial or financial relationships that could be construed as a potential conflict of interest.
Publisher’s note
All claims expressed in this article are solely those of the authors and do not necessarily represent those of their affiliated organizations, or those of the publisher, the editors and the reviewers. Any product that may be evaluated in this article, or claim that may be made by its manufacturer, is not guaranteed or endorsed by the publisher.
Supplementary material
The Supplementary Material for this article can be found online at: https://www.frontiersin.org/articles/10.3389/fcimb.2023.1130749/full#supplementary-material
References
Agerer, B., Koblischke, M., Gudipati, V., Smyth, M., Popa, A., Genger, J.-W., et al. (2020). SARS-CoV-2 mutations in MHC-I-restricted epitopes evade CD8+ T cell responses. Science Immunology 6 (57), eabg6461. doi: 10.1126/sciimmunol.abg6461
Alcon-LePoder, S., Drouet, M.-T., Roux, P., Frenkiel, M.-P., Arborio, M., Durand-Schneider, A.-M., et al. (2005). The secreted form of dengue virus nonstructural protein NS1 is endocytosed by hepatocytes and accumulates in late endosomes: Implications for viral infectivity. J. Virol. 79 (17), 11403–11411. doi: 10.1128/JVI.79.17.11403-11411.2005
Anthony, C., York, T., Bekker, V., Matten, D., Selhorst, P., Ferreria, R.-C., et al. (2017). Cooperation between strain-specific and broadly neutralizing responses limited viral escape and prolonged the exposure of the broadly neutralizing epitope. J. Virol. 91 (18), e00828–e00817. doi: 10.1128/jvi.00828-17
Aryani, A., Denecke, B. (2015). In vitro application of ribonucleases: Comparison of the effects on mRNA and miRNA stability. BMC Research Notes 8 (1), 164. doi: 10.1186/s13104-015-1114-z
Avirutnan, P., Zhang, L., Punyadee, N., Manuyakorn, A., Puttikhunt, C., Kasinrerk, W., et al. (2007). Secreted NS1 of dengue virus attaches to the surface of cells via interactions with heparan sulfate and chondroitin sulfate e. PLoS Pathog. 3 (11), e183. doi: 10.1371/journal.ppat.0030183
Bailey, M. J., Broecker, F., Duehr, J., Arumemi, F., Krammer, F., Palese, P., et al. (2019). Antibodies elicited by an NS1-based vaccine protect mice against zika virus. mBio 10 (2). doi: 10.1128/mBio.02861-18
Barba-Spaeth, G., Dejnirattisai, W., Rouvinski, A., Vaney, M.-C., Medits, I., Sharma, A., et al. (2016). Structural basis of potent zika–dengue virus antibody cross-neutralization. Nature 536 (7614), 48–53. doi: 10.1038/nature18938
Bates, T. A., Chuong, C., Hawks, S. A., Rai, P., Duggal, N. K., Weger-Lucarelli, J (2021). Development and characterization of infectious clones of two strains of usutu virus. Virology 554, 28–36. doi: 10.1016/j.virol.2020.12.004
Bates, T.A., Chuong, C., Hawks, S.A., Rai, P., Duggal, N.K., Weger-Lucarelli, J.. (2020). Development and characterization of infectious clones of two strains of usutu virus. Virology 554, 28–36. doi: 10.1016/j.virol.2020.12.004
Baum, A., Fulton, B. O., Wloga, E., Copin, R., Pascal, K. E., Russo, V., et al. (2020). Antibody cocktail to SARS-CoV-2 spike protein prevents rapid mutational escape seen with individual antibodies. Science 369 (6506), 1014–1018. doi: 10.1126/science.abd0831
Berg, M. G., Yamaguchi, J., Alessandri-Gradt, E., Tell, R. W., Plantier, J.-C., Brennan, C. A. (2016). A pan-HIV strategy for complete genome sequencing. J. Clin. Microbiol. 54 (4), 868–882. doi: 10.1128/jcm.02479-15
Bhatt, S., Gething, P. W., Brady, O. J., Messina, J. P., Farlow, A. W., Moyes, C. L., et al. (2013). The global distribution and burden of dengue. Nature 496 (7446), 504–507. doi: 10.1038/nature12060
Blish, C. A., Nguyen, M.-A., Overbaugh, J. (2008). Enhancing exposure of HIV-1 neutralization epitopes through mutations in gp41. PLoS Med. 5 (1), e9. doi: 10.1371/journal.pmed.0050009
Borisevich, V., Lee, B., Hickey, A., Debuysscher, B., Broder, C. C., Feldmann, H., et al. (2016). Escape from monoclonal antibody neutralization affects henipavirus fitness In vitro and In vivo. J Infect Dis 213, 3, 448–455. doi: 10.1093/infdis/jiv449
Bowen, D. G., Walker, C. M. (2005). Mutational escape from CD8+ T cell immunity. J. Exp. Med. 201 (11), 1709–1714. doi: 10.1084/jem.20050808
Bushnell, B. (2014). BBMap: A fast, accurate, splice-aware aligner (Berkeley, CA (United States: Lawrence Berkeley National Lab. (LBNL).
Cao, J., McNevin, J., Malhotra, U., McElrath, M. J. (2003). Evolution of CD8+T cell immunity and viral escape following acute HIV-1 infection. J. Immunol. 171 (7), 3837–3846. doi: 10.4049/jimmunol.171.7.3837
Carr, I. M., Robinson, J. I., Dimitriou, R., Markham, A. F., Morgan, A. W., Bonthron, D. T. (2009). Inferring relative proportions of DNA variants from sequencing electropherograms. Bioinformatics 25 (24), 3244–3250. doi: 10.1093/bioinformatics/btp583
Carvalho, M. S., Freitas, L. P., Cruz, O. G., Brasil, P., Bastos, L. S. (2020). Association of past dengue fever epidemics with the risk of zika microcephaly at the population level in Brazil. Sci. Rep. 10 (1), 1752. doi: 10.1038/s41598-020-58407-7
Castanha, P. M. S., Erdos, G., Watkins, S. C., Falo, L. D., Jr., Marques, E. T. A., Barratt-Boyes, S. M. (2020). Reciprocal immune enhancement of dengue and zika virus infection in human skin. JCI Insight 5 (3). doi: 10.1172/jci.insight.133653
Castanha, P. M., Nascimento, E. J. M., Cynthia, B., Cordeiro, M. T., De Carvalho, O. V., De Mendonça, L. R., et al. (2016). Dengue virus (DENV)-specific antibodies enhance Brazilian zika virus (ZIKV) infection. J. Infect. Dis., jiw638. doi: 10.1093/infdis/jiw638
Ciurea, A., Hunziker, L., Martinic, M. M. A., Oxenius, A., Hengartner, H., Zinkernagel, R. M. (2001). CD4+ t-cell–epitope escape mutant virus selected in vivo. Nat. Med. 7 (7), 795–800. doi: 10.1038/89915
Cleveland, S. M., Taylor, H. P., Dimmock, N. J. (1997). Selection of neutralizing antibody escape mutants with type a influenza virus HA-specific polyclonal antisera: Possible significance for antigenic drift. Epidemiol. Infection 118 (2), 149–154. doi: 10.1017/s0950268896007303
Coffey, L. L., Forrester, N., Tsetsarkin, K., Vasilakis, N., Weaver, S. C. (2013). Factors shaping the adaptive landscape for arboviruses: Implications for the emergence of disease. Future Microbiol 8, 2, 155–176. doi: 10.2217/fmb.12.139
Crook, K. R., Miller-Kittrell, M., Morrison, C. R., Scholle, F. (2014). Modulation of innate immune signaling by the secreted form of the West Nile virus NS1 glycoprotein. Virology 458-459, 172–182. doi: 10.1016/j.virol.2014.04.036
Danecek, P., Bonfield, J. K., Liddle, J., Marshall, J., Ohan, V., Pollard, M. O., et al. (2021). Twelve years of SAMtools and BCFtools. Gigascience 10 (2). doi: 10.1093/gigascience/giab008
Deardorff, E. R., Fitzpatrick, K. A., Jerzak, G. V. S., Shi, P.-Y., Kramer, L. D., Ebel, G. D. (2011). West Nile Virus experimental evolution in vivo and the trade-off hypothesis. PLoS Pathog. 7 (11), e1002335. doi: 10.1371/journal.ppat.1002335
Depew, J., Zhou, B., McCorrison, J. M., Wentworth, D. E., Purushe, J., Koroleva, G., et al. (2013). Sequencing viral genomes from a single isolated plaque. Virology Journal 10, 1, 181. doi: 10.1186/1743-422x-10-181
Doud, M. B., Lee, J. M., Bloom, J. D. (2018). How single mutations affect viral escape from broad and narrow antibodies to H1 influenza hemagglutinin. Nat. Commun. 9 (1), 1386. doi: 10.1038/s41467-018-03665-3
Faria, N. R., Azevedo, R., Kraemer, M. U. G., Souza, R., Cunha, M. S., Hill, S. C., et al. (2016). Zika virus in the americas: Early epidemiological and genetic findings. Science 352 (6283), 345–349. doi: 10.1126/science.aaf5036
Ferguson, N. M., Galvani, A. P., Bush, R. M. (2003). Ecological and immunological determinants of influenza evolution. Nature 422 (6930), 428–433. doi: 10.1038/nature01509
Ferreira, I. A. T. M., Kemp, S. A., Datir, R., Saito, A., Meng, B., Rakshit, P., et al. (2021). SARS-CoV-2 B.1.617 mutations L452R and E484Q are not synergistic for antibody evasion. J. Infect. Dis. 224 (6), 989–994. doi: 10.1093/infdis/jiab368
Fontes-Garfias, C. R., Shan, C., Luo, H., Muruato, A. E., Medeiros, D. B. A., Mays, E., et al. (2017). Functional analysis of glycosylation of zika virus envelope protein. Cell Rep. 21 (5), 1180–1190. doi: 10.1016/j.celrep.2017.10.016
Fowler, A. M., Tang, W. W., Young, M. P., Mamidi, A., Viramontes, K. M., McCauley, M. D., et al. (2018). Maternally acquired zika antibodies enhance dengue disease severity in mice. Cell Host Microbe 24 (5), 743–750.e745. doi: 10.1016/j.chom.2018.09.015
Gal-Tanamy, M., Keck, Z.-Y., Yi, M., McKeating, J. A., Patel, A. H., Foung, S. K. H., et al. (2008). In vitro selection of a neutralization-resistant hepatitis c virus escape mutant. Proc. Natl. Acad. Sci. 105 (49), 19450–19455. doi: 10.1073/pnas.0809879105
Genoud, V., Stortz, M., Waisman, A., Berardino, B. G., Verneri, P., Dansey, V., et al. (2021). Extraction-free protocol combining proteinase K and heat inactivation for detection of SARS-CoV-2 by RT-qPCR. PloS One 16 (2), e0247792. doi: 10.1371/journal.pone.0247792
Hadfield, J., Megill, C., Bell, S. M., Huddleston, J., Potter, B., Callender, C., et al. (2018). Nextstrain: real-time tracking of pathogen evolution. Bioinformatics 34 (23), 4121–4123. doi: 10.1093/bioinformatics/bty407
Hamel, R., Dejarnac, O., Wichit, S., Ekchariyawat, P., Neyret, A., Luplertlop, N., et al. (2015). Biology of zika virus infection in human skin cells. J. Virol. 89 (17), 8880–8896. doi: 10.1128/jvi.00354-15
Haslwanter, D., Dieterle, M. E., Wec, A. Z., O’Brien, C. M., Sakharkar, M., Florez, C., et al. (2021). A combination of receptor-binding domain and n-terminal domain neutralizing antibodies limits the generation of SARS-CoV-2 spike neutralization-escape mutants. mBio 12 (5), e02473–e02421. doi: 10.1128/mBio.02473-21
Hu, Y., Zou, S., Wang, Z., Liu, Y., Ren, L., Hao, Y., et al. (2021). Virus evolution and neutralization sensitivity in an HIV-1 subtype b' infected plasma donor with broadly neutralizing activity. Vaccines 9 (4). doi: 10.3390/vaccines9040311
Jin, J., Liss, N., Chen, D.-H., Liao, M., Fox, J., Shimak, R., et al. (2015). Neutralizing monoclonal antibodies block chikungunya virus entry and release by targeting an epitope critical to viral pathogenesis. Cell Rep. 13 (11), 2553–2564. doi: 10.1016/j.celrep.2015.11.043
Kassen, R. (2002). The experimental evolution of specialists, generalists, and the maintenance of diversity. J. Evolutionary Biol. 15 (2), 173–190. doi: 10.1046/j.1420-9101.2002.00377.x
Katzelnick, L. C., Zambrana, J. V., Elizondo, D., Collado, D., Garcia, N., Arguello, S., et al. (2021). Dengue and zika virus infections in children elicit cross-reactive protective and enhancing antibodies that persist long term. Sci. Transl. Med. 13 (614), eabg9478. doi: 10.1126/scitranslmed.abg9478
Keeffe, J. R., Van Rompay, K. K. A., Olsen, P. C., Wang, Q., Gazumyan, A., Azzopardi, S. A., et al. (2018). A combination of two human monoclonal antibodies prevents zika virus escape mutations in non-human primates. Cell Rep. 25 (6), 1385–1394.e1387. doi: 10.1016/j.celrep.2018.10.031
Khaiboullina, S. F., Uppal, T., Sarkar, R., Gorzalski, A., St Jeor, S., Verma, S. C. (2017). ZIKV infection regulates inflammasomes pathway for replication in monocytes. Sci. Rep. 7 (1), 16050. doi: 10.1038/s41598-017-16072-3
Korunes, K. L., Samuk, K. (2021). Pixy: Unbiased estimation of nucleotide diversity and divergence in the presence of missing data. Mol. Ecol. Resour. 21 (4), 1359–1368. doi: 10.1111/1755-0998.13326
Kuo, L., Jaeger, A. S., Banker, E. M., Bialosuknia, S. M., Mathias, N., Payne, A. F., et al. (2020). Reversion to ancestral zika virus NS1 residues increases competence of aedes albopictus. PLoS Pathog. 16 (10), e1008951. doi: 10.1371/journal.ppat.1008951
Lambkin, R., McLain, L., Jones, S. E., Aldridge, S. L., Dimmock, N. J. (1994). Neutralization escape mutants of type a influenza virus are readily selected by antisera from mice immunized with whole virus: a possible mechanism for antigenic drift. J. Gen. Virol. 75 (12), 3493–3502. doi: 10.1099/0022-1317-75-12-3493
Lee, A. J., Das, S. R., Wang, W., Fitzgerald, T., Pickett, B. E., Aevermann, B. D., et al. (2015). Diversifying selection analysis predicts antigenic evolution of 2009 pandemic H1N1 influenza a virus in humans. J. Virol. 89 (10), 5427–5440. doi: 10.1128/jvi.03636-14
Lee, J. M., Eguia, R., Zost, S. J., Choudhary, S., Wilson, P. C., Bedford, T., et al. (2019). Mapping person-to-person variation in viral mutations that escape polyclonal serum targeting influenza hemagglutinin. eLife 8. doi: 10.7554/elife.49324
Li, H., Durbin, R. (2009). Fast and accurate short read alignment with burrows-wheeler transform. Bioinformatics 25 (14), 1754–1760. doi: 10.1093/bioinformatics/btp324
Liu, Y., Liu, J., Du, S., Shan, C., Nie, K., Zhang, R., et al. (2017). Evolutionary enhancement of zika virus infectivity in aedes aegypti mosquitoes. Nature 545 (7655), 482–486. doi: 10.1038/nature22365
Liu, Y., Liu, J., Johnson, B. A., Xia, H., Ku, Z., Schindewolf, C., et al. (2022a). Delta spike P681R mutation enhances SARS-CoV-2 fitness over alpha variant. Cell Rep. 39 (7), 110829. doi: 10.1016/j.celrep.2022.110829
Liu, Y., Liu, J., Plante, K. S., Plante, J. A., Xie, X., Zhang, X., et al. (2022b). The N501Y spike substitution enhances SARS-CoV-2 infection and transmission. Nature 602 (7896), 294–299. doi: 10.1038/s41586-021-04245-0
Liu, J., Liu, Y., Shan, C., Nunes, B. T. D., Yun, R., Haller, S. L., et al. (2021a). Role of mutational reversions and fitness restoration in zika virus spread to the americas. Nat. Commun. 12 (1), 595. doi: 10.1038/s41467-020-20747-3
Liu, J. L., Webb, E. M., Zabetakis, D., Burke, C. W., Gardner, C. L., Glass, P. J., et al. (2021b). Stabilization of a broadly neutralizing anti-chikungunya virus single domain antibody. Front. Med. (Lausanne) 8. doi: 10.3389/fmed.2021.626028
MacLean, O. A., Lytras, S., Weaver, S., Singer, J. B., Boni, M. F., Lemey, P., et al. (2021). Natural selection in the evolution of SARS-CoV-2 in bats created a generalist virus and highly capable human pathogen. PloS Biol. 19 (3), e3001115. doi: 10.1371/journal.pbio.3001115
Marano, J., Cereghino, C., Finkielstein, C., Weger-Lucarelli, J. (2022). An in vitro workflow to create and modify novel infectious clones using replication cycle reaction (Research Square).
Marano, J. M., Chuong, C., Weger-Lucarelli, J. (2020). Rolling circle amplification: A high fidelity and efficient alternative to plasmid preparation for the rescue of infectious clones. Virology 551, 58–63. doi: 10.1016/j.virol.2020.08.016
Marchi, J., Lässig, M., Walczak, A. M., Mora, T. (2021). Antigenic waves of virus–immune coevolution. Proc. Natl. Acad. Sci. 118 (27), e2103398118. doi: 10.1073/pnas.2103398118
McKenna, A., Hanna, M., Banks, E., Sivachenko, A., Cibulskis, K., Kernytsky, A., et al. (2010). The genome analysis toolkit: A MapReduce framework for analyzing next-generation DNA sequencing data. Genome Res. 20 (9), 1297–1303. doi: 10.1101/gr.107524.110
Mishra, N., Sharma, S., Dobhal, A., Kumar, S., Chawla, H., Singh, R., et al. (2020). Broadly neutralizing plasma antibodies effective against autologous circulating viruses in infants with multivariant HIV-1 infection. Nat. Commun. 11 (1), 4409. doi: 10.1038/s41467-020-18225-x
Moore, S. M., Oidtman, R. J., Soda, K. J., Siraj, A. S., Reiner, R. C., Jr., Barker, C. M., et al. (2020). Leveraging multiple data types to estimate the size of the zika epidemic in the americas. PloS Negl. Trop. Dis. 14 (9), e0008640. doi: 10.1371/journal.pntd.0008640
Morris, D. H., Petrova, V. N., Rossine, F. W., Parker, E., Grenfell, B. T., Neher, R. A., et al. (2020). Asynchrony between virus diversity and antibody selection limits influenza virus evolution. eLife 9, e62105. doi: 10.7554/eLife.62105
Musso, D., Ko, A. I., Baud, D. (2019). Zika virus infection — after the pandemic. New Engl. J. Med. 381 (15), 1444–1457. doi: 10.1056/NEJMra1808246
Nelson, C. W., Moncla, L. H., Hughes, A. L. (2015). SNPGenie: Estimating evolutionary parameters to detect natural selection using pooled next-generation sequencing data. Bioinformatics 31 (22), 3709–3711. doi: 10.1093/bioinformatics/btv449
Patterson, J., Sammon, M., Garg, M. (2016). Dengue, zika and chikungunya: Emerging arboviruses in the new world. West J. Emerg. Med. 17 (6), 671–679. doi: 10.5811/westjem.2016.9.30904
Pedroso, C., Fischer, C., Feldmann, M., Sarno, M., Luz, E., Moreira-Soto, A., et al. (2019). Cross-protection of dengue virus infection against congenital zika syndrome, northeastern Brazil. Emerg. Infect. Dis. 25 (8), 1485–1493. doi: 10.3201/eid2508.190113
Puerta-Guardo, H., Biering, S. B., de Sousa, F. T. G., Shu, J., Glasner, D. R., Li, J., et al. (2022). Flavivirus NS1 triggers tissue-specific disassembly of intercellular junctions leading to barrier dysfunction and vascular leak in a GSK-3β-Dependent manner. Pathogens 11 (6), 1598–1613.e1598. doi: 10.3390/pathogens11060615
Puerta-Guardo, H., Glasner, D. R., Espinosa, D. A., Biering, S. B., Patana, M., Ratnasiri, K., et al. (2019). Flavivirus NS1 triggers tissue-specific vascular endothelial dysfunction reflecting disease tropism. Cell Rep. 26 (6), 1598–1613.e1598. doi: 10.1016/j.celrep.2019.01.036
Rastogi, M., Sharma, N., Singh, S. K. (2016). Flavivirus NS1: A multifaceted enigmatic viral protein. Virol. J. 13, 131. doi: 10.1186/s12985-016-0590-7
Rathore, A. P. S., Saron, W. A. A., Lim, T., Jahan, N., St. John, A. L. (2019). Maternal immunity and antibodies to dengue virus promote infection and zika virus–induced microcephaly in fetuses. Sci. Adv. 5 (2), eaav3208. doi: 10.1126/sciadv.aav3208
Rathore, A. P. S., St. John, A. L. (2020). Cross-reactive immunity among flaviviruses. Front. Immunol. 11. doi: 10.3389/fimmu.2020.00334
Regla-Nava, J. A., Elong Ngono, A., Viramontes, K. M., Huynh, A.-T., Wang, Y.-T., Nguyen, A.-V. T., et al. (2018). Cross-reactive dengue virus-specific CD8+ T cells protect against zika virus during pregnancy. Nat. Commun. 9 (1), 3042. doi: 10.1038/s41467-018-05458-0
Regla-Nava, J. A., Wang, Y.-T., Fontes-Garfias, C. R., Liu, Y., Syed, T., Susantono, M., et al. (2022). A zika virus mutation enhances transmission potential and confers escape from protective dengue virus immunity. Cell Rep. 39 (2), 110655. doi: 10.1016/j.celrep.2022.110655
Rockx, B., Donaldson, E., Frieman, M., Sheahan, T., Corti, D., Lanzavecchia, A., et al. (2010). Escape from human monoclonal antibody neutralization affects in vitro and in vivo fitness of severe acute respiratory syndrome coronavirus. J. Infect. Dis. 201 (6), 946–955. doi: 10.1086/651022
Rodgers, M. A., Wilkerson, E., Vallari, A., McArthur, C., Sthreshley, L., Brennan, C. A., et al. (2017). Sensitive next generation sequencing method reveals deep genetic diversity of HIV-1 in the democratic republic of the Congo. J Virol 91 (6). doi: 10.1128/jvi.01841-16
Roesch, F., Cereghino, C., Carrau, L., Hardy, A., Ribeiro-Filho, H., Lacritick, A. H., et al. (2022). The E2 glycoprotein holds key residues for mayaro virus adaptation to the urban. Aedes aegypti mosquito. bioRxiv 2022, 2004.2005.487100. doi: 10.1101/2022.04.05.487100
Sapkal, G. N., Harini, S., Ayachit, V. M., Fulmali, P. V., Mahamuni, S. A., Bondre, V. P., et al. (2011). Neutralization escape variant of West Nile virus associated with altered peripheral pathogenicity and differential cytokine profile. Virus Res. 158 (1-2), 130–139. doi: 10.1016/j.virusres.2011.03.023
Sasmono, R. T., Taurel, A.-F., Prayitno, A., Sitompul, H., Yohan, B., Hayati, R. F., et al. (2018). Dengue virus serotype distribution based on serological evidence in pediatric urban population in Indonesia. PLoS Negl. Trop. Dis. 12 (6), e0006616. doi: 10.1371/journal.pntd.0006616
Shan, C., Xia, H., Haller, S. L., Azar, S. R., Liu, Y., Liu, J., et al. (2020). A zika virus envelope mutation preceding the 2015 epidemic enhances virulence and fitness for transmission. Proc. Natl. Acad. Sci. U.S.A. 117 (33), 20190–20197. doi: 10.1073/pnas.2005722117
Sharp, T. M., Fischer, M., Muñoz-Jordán, J. L., Paz-Bailey, G., Staples, J. E., Gregory, C. J., et al. (2019). Dengue and zika virus diagnostic testing for patients with a clinically compatible illness and risk for infection with both viruses. MMWR. Recommendations Rep. 68 (1), 1–10. doi: 10.15585/mmwr.rr6801a1
Sui, J., Aird, D. R., Tamin, A., Murakami, A., Yan, M., Yammanuru, A., et al. (2008). Broadening of neutralization activity to directly block a dominant antibody-driven SARS-coronavirus evolution pathway. PloS Pathog. 4 (11), e1000197. doi: 10.1371/journal.ppat.1000197
Talavera-Aguilar, L. G., Murrieta, R. A., Kiem, S., Cetina-Trejo, R. C., Baak-Baak, C. M., Ebel, G. D., et al. (2021). Infection, dissemination, and transmission efficiencies of zika virus in aedes aegypti after serial passage in mosquito or mammalian cell lines or alternating passage in both cell types. Parasites Vectors 14 (1), 261. doi: 10.1186/s13071-021-04726-1
Vaughan, K., Greenbaum, J., Blythe, M., Peters, B., Sette, A. (2010). Meta-analysis of all immune epitope data in theFlavivirusGenus: Inventory of current immune epitope data status in the context of virus immunity and immunopathology. Viral Immunol. 23 (3), 259–284. doi: 10.1089/vim.2010.0006
Weger-Lucarelli, J., Duggal, N. K., Bullard-Feibelman, K., Veselinovic, M., Romo, H., Nguyen, C., et al. (2017). Development and characterization of recombinant virus generated from a new world zika virus infectious clone. J. Virol. 91 (1), JVI.01765–01716. doi: 10.1128/jvi.01765-16
Weger-Lucarelli, J., Rückert, C., Chotiwan, N., Nguyen, C., Garcia Luna, S. M., Fauver, J. R., et al. (2016). Vector competence of American mosquitoes for three strains of zika virus. PLoS Negl. Trop. Dis. 10 (10), e0005101. doi: 10.1371/journal.pntd.0005101
Wilm, A., Aw, P. P., Bertrand, D., Yeo, G. H., Ong, S. H., Wong, C. H., et al. (2012). LoFreq: A sequence-quality aware, ultra-sensitive variant caller for uncovering cell-population heterogeneity from high-throughput sequencing datasets. Nucleic Acids Res. 40 (22), 11189–11201. doi: 10.1093/nar/gks918
Wilson, D. S., Yoshimura, J. (1994). On the coexistence of specialists and generalists. Am. Nat. 144 (4), 692–707. doi: 10.1086/285702
Xia, H., Luo, H., Shan, C., Muruato, A. E., Nunes, B. T. D., Medeiros, D. B. A., et al. (2018). An evolutionary NS1 mutation enhances zika virus evasion of host interferon induction. Nat. Commun. 9 (1), 414. doi: 10.1038/s41467-017-02816-2
Yamashiro, T., Vardez, S., Nishizono, A., Petit, A., Taveras, D., Cesin, A. J., et al. (2004). SEROPREVALENCE OF IgG SPECIFIC FOR DENGUE VIRUS AMONG ADULTS AND CHILDREN IN SANTO DOMINGO, DOMINICAN REPUBLIC. Am. J. Trop. Med. Hygiene 71 (2), 138–143. doi: 10.4269/ajtmh.2004.71.138
Yuan, L., Huang, X.-Y., Liu, Z.-Y., Zhang, F., Zhu, X.-L., Yu, J.-Y., et al. (2017). A single mutation in the prM protein of zika virus contributes to fetal microcephaly. Science 358 (6365), 933–936. doi: 10.1126/science.aam7120
Zhao, X., Chen, F.-P., Sullender, W. M. (2004). Respiratory syncytial virus escape mutant derived in vitro resists palivizumab prophylaxis in cotton rats. Virology 318 (2), 608–612. doi: 10.1016/j.virol.2003.10.018
Zhao, X., Liu, E., Chen, F.-P., Sullender, W. M. (2006). In vitro and In vivo fitness of respiratory syncytial virus monoclonal antibody escape mutants. J. Virol. 80 (23), 11651–11657. doi: 10.1128/jvi.01387-06
Zimmerman, M. G., Quicke, K. M., O'Neal, J. T., Arora, N., Machiah, D., Priyamvada, L., et al. (2018). Cross-reactive dengue virus antibodies augment zika virus infection of human placental macrophages. Cell Host Microbe 24 (5), 731–742.e736. doi: 10.1016/j.chom.2018.10.008
Keywords: evolution, flaviviruses, cross-reactive immunity, Zika virus (ZIKV), dengue virus (DENV), trade-off hypothesis
Citation: Marano JM and Weger-Lucarelli J (2023) Replication in the presence of dengue convalescent serum impacts Zika virus neutralization sensitivity and fitness. Front. Cell. Infect. Microbiol. 13:1130749. doi: 10.3389/fcimb.2023.1130749
Received: 23 December 2022; Accepted: 13 February 2023;
Published: 09 March 2023.
Edited by:
Hongguang Ren, Institute of Biotechnology (CAAS), ChinaReviewed by:
Jaturong Sewatanon, Mahidol University, ThailandVida Liliana Hodara, Texas Biomedical Research Institute, United States
Hui Zhao, Beijing Institute of Microbiology and Epidemiology, China
Copyright © 2023 Marano and Weger-Lucarelli. This is an open-access article distributed under the terms of the Creative Commons Attribution License (CC BY). The use, distribution or reproduction in other forums is permitted, provided the original author(s) and the copyright owner(s) are credited and that the original publication in this journal is cited, in accordance with accepted academic practice. No use, distribution or reproduction is permitted which does not comply with these terms.
*Correspondence: James Weger-Lucarelli, d2VnZXJAdnQuZWR1