- 1Center for Vector-borne Infectious Diseases, Dept. of Microbiology, Immunology and Pathology, Colorado State University, Fort Collins, CO, United States
- 2Chakri Naruebodindra Medical Institute, Faculty of Medicine Ramathibodi Hospital, Mahidol University, Samut Prakan, Thailand
Among many medically important pathogens, arboviruses like dengue, Zika and chikungunya cause severe health and economic burdens especially in developing countries. These viruses are primarily vectored by mosquitoes. Having surmounted geographical barriers and threat of control strategies, these vectors continue to conquer many areas of the globe exposing more than half of the world’s population to these viruses. Unfortunately, no medical interventions have been capable so far to produce successful vaccines or antivirals against many of these viruses. Thus, vector control remains the fundamental strategy to prevent disease transmission. The long-established understanding regarding the replication of these viruses is that they reshape both human and mosquito host cellular membranes upon infection for their replicative benefit. This leads to or is a result of significant alterations in lipid metabolism. Metabolism involves complex chemical reactions in the body that are essential for general physiological functions and survival of an organism. Finely tuned metabolic homeostases are maintained in healthy organisms. However, a simple stimulus like a viral infection can alter this homeostatic landscape driving considerable phenotypic change. Better comprehension of these mechanisms can serve as innovative control strategies against these vectors and viruses. Here, we review the metabolic basis of fundamental mosquito biology and virus-vector interactions. The cited work provides compelling evidence that targeting metabolism can be a paradigm shift and provide potent tools for vector control as well as tools to answer many unresolved questions and gaps in the field of arbovirology.
1 Introduction
1.1 Arboviruses are a significant disease burden to human populations
Arboviral infections are becoming increasingly aggressive on a global scale due to climate change, global travel and the development of insecticide resistance in vectors. They are vectored by mosquitoes, ticks, sandflies or biting midges. Among these, mosquito-borne viruses contribute heavily to the disease burden in human populations especially in developing countries (Franklinos et al., 2019). Flaviviruses such as dengue, Zika and West Nile and alphaviruses such as chikungunya are amongst the most common mosquito-borne viruses causing human disease. Dengue viruses (DENVs) are responsible for ~400 million infections each year and more than a quarter of the global population lives in endemic areas (Bhatt et al., 2013). Zika virus (ZIKV) has caused severe epidemics and according to the World Health Organization (WHO), a total of 86 countries and territories have reported ZIKV cases to date (World Health Organization, 2022). In addition to being transmitted by the mosquito vector, ZIKV can be transmitted from mother to fetus during pregnancy, through sexual contact, blood transfusions and organ transplants thus widening its transmission capacity. It is estimated that during the last epidemic in 2015-2016, approximately 1.5 million people were infected by ZIKV in Brazil with over 3,500 microcephaly cases reported (European Center for Disease Prevention and Control, 2015). West Nile virus (WNV) is another important agent causing disease in both humans and horses and is the most common etiological agent of viral encephalitis (Chancey et al., 2015). There is currently no vaccine available for WNV. Besides these flaviviruses, chikungunya virus (CHIKV) is an alphavirus that has caused severe outbreaks in Asia, Africa, Americas, and Europe making it a public health concern globally (World Health Organization, 2020). As of October 2022, nearly 3,400,000 chikungunya cases and 70 deaths have been reported globally with Brazil having the most cases (European Center for Disease Prevention and Control, 2022). During the massive outbreak in 2005-2006 in La Reunion Island, the virus acquired the ability to transmit via its secondary vector, Aedes albopictus due to an amino acid change in the E1 glycoproteinintheEast-
Central-South African genotype of the virus. In addition, other mutations in the E1 and E2 glycoproteins have further increased mosquito infectivity of the virus (Tsetsarkin et al., 2007).
1.2 Mosquitoes are the primary vector of medically relevant arboviruses
Ae. aegypti (Diptera: Culicidae) is the major mosquito vector that transmits the viruses discussed above. These mosquitoes inhabit various regions of the world including both tropical and subtropical areas across several continents including Asia, Africa, North and South America, Europe and Australia (Kraemer et al., 2015). Since they are anthropophilic, these mosquitoes are well adapted to rapid urbanization and prefer artificial water containers for egg laying (Scott and Takken, 2012; Kraemer et al., 2015). Interestingly, the geographical distribution of the mosquito vector is temperature dependent (Brady et al., 2012; Brady et al., 2014; Kraemer et al., 2015) and it has been predicted that the mosquito habitats will be expanded to currently more temperate regions due to climate change (Khormi and Kumar, 2014; Mweya et al., 2016). Therefore, it is anticipated that even larger human populations will be exposed to these disease carrying vectors in the future.
1.3 Metabolism is at the forefront of mosquito development and biology
Living organisms are vastly diverse. Every organism has signature characteristics in morphology, anatomy and physiology. Further, there is significant diversity in factors such as behavior and ecology. On the contrary, organisms are also remarkably analogous to each other based on fundamental traits at the molecular level. These similarities are basically mirrored in metabolism and biochemical mechanisms of inheritance (Robert Burger et al., 2021). Metabolites are universal molecules that do not vary across species or ecological barriers. These molecules are reflective of the output of genetic expression (DNA/RNA/protein interactions). Metabolic adaptations occur within an organism when in need of maintaining energy homeostasis and development under different environmental stimuli (Koyama et al., 2020). Therefore, metabolism is intimately associated with the biology of an organism. Investigating mosquito metabolism can provide the blueprint of a mosquito’s response to stimuli such as a viral infection, the changing microbiome, insecticide resistance and environmental changes. Importantly, metabolites can be traced back to identify the genotype of a particular phenotype helping to understand the molecular basis of biochemical responses.
Lipid metabolism in mosquitoes has been studied since the mid-1900s. Due to the lack of advanced technologies, these studies focused on the response of mosquito lipids to different diets, the conversion of food to fat, storage of fat in the fat body of the insects, the utilization of fat for energy during flight, metamorphosis, starvation, and the deposition of fat for oogenesis. The recent availability of the genome sequences of certain mosquitoes, advanced molecular biology techniques and the advent of systems biology approaches especially techniques in metabolomics have helped us understand lipid metabolism at a molecular level in mosquitoes as well as other insects. The first half of this review will focus on the findings from the late 1900s to the early 2000s, on the utilization of lipids in several physiological processes of mosquitoes. The second half of this review will focus on the discovery (or re-discovery) of mosquito lipids from the molecular biology/omics era.
2 The mosquito life cycle is intimately associated with metabolic processes
The mosquito life cycle has four major stages (Figure 1A, 1-4): eggs, larvae, pupae and adults. Each stage has a different morphology, habitat, behavior, food source, and thus is exposed to different metabolic sources driving differential usage of metabolites. Additionally, each stage of the mosquito life cycle is regulated to provide optimum resources for the next developmental phase. Therefore, the conditions faced by the immature phases of the mosquito drive reproductive success, longevity, and vector competence of the adult mosquito. Communication between different metabolic pathways that are active at each life stage may be key to this nutritional continuity.
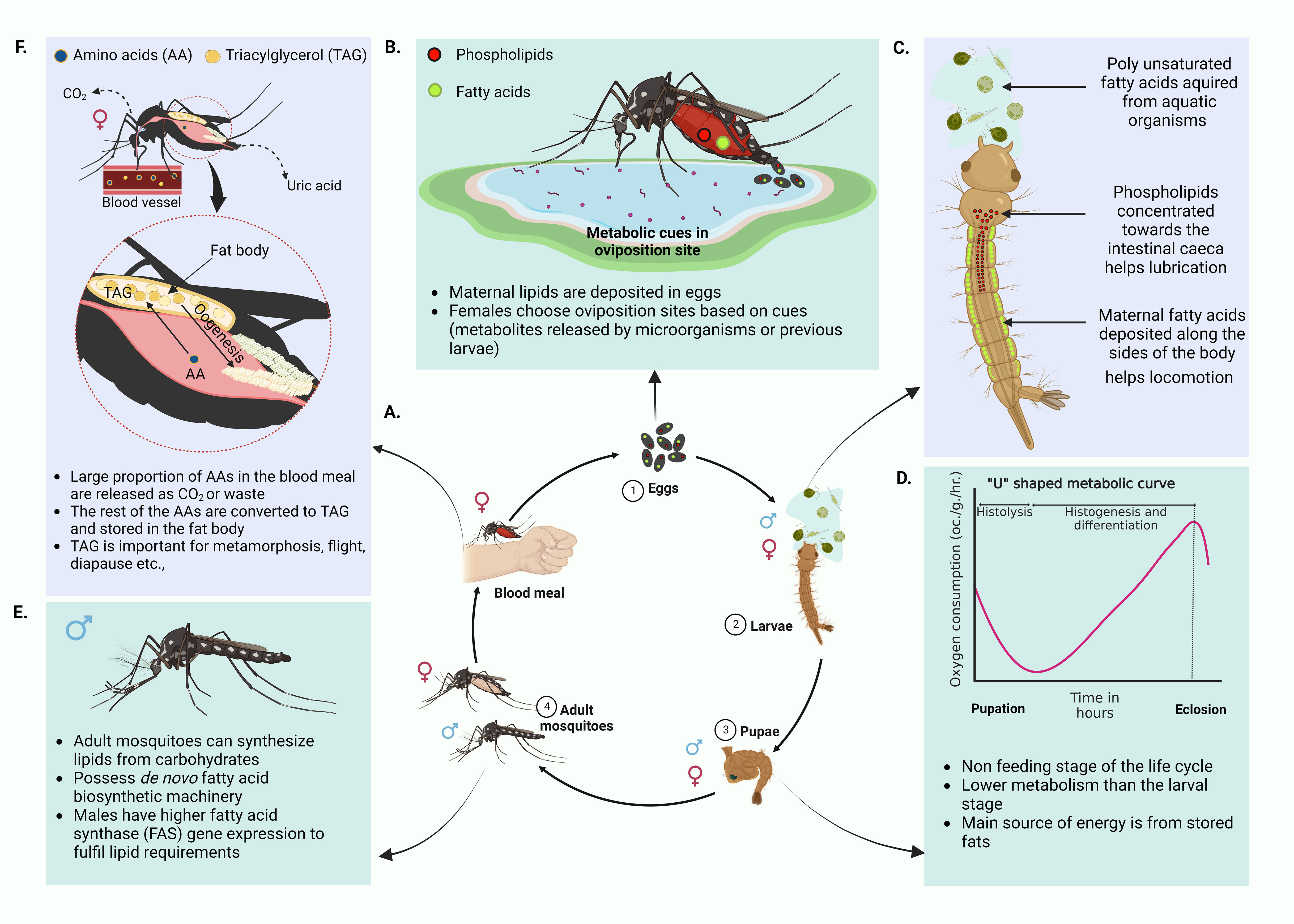
Figure 1 Mosquito life cycle and related metabolism. (A) Life cycle of the mosquito. Four major stages of the mosquito life cycle, eggs, larvae, pupae and adults. Male mosquitoes feed on nectar and other sugar sources whereas the female mosquitoes require a vertebrate blood meal for egg maturation. (B) Deposition of maternal lipids in eggs and selection of oviposition sites. Majority of lipids in mosquito eggs come from maternal lipid deposits (Ziegler, 1997; Canavoso et al., 2001; Ziegler and Ibrahim, 2001; Atella and Shahabuddin, 2002; Sushchik et al., 2013). These lipids are generated post blood meal. Only a small proportion of egg lipids represent lipids generated from a sugar meal. Maternal lipid deposits aid the survival of the neonate larvae. Female mosquitoes exploit a range of environmental cues, especially metabolites in the habitats to select good oviposition sites (Perry and Fay, 1967; Ikeshoji et al., 1975; Ikeshoji et al., 1979; Bentley and Day, 1989; Davis, 1976) (C) Differential distribution of maternal lipids in neonate larvae. Maternal lipid distribution commences at the embryonic stages. Maternal phospholipids concentrated towards the intestinal caeca of the larvae assists lubrication and assimilation of nutrients from the diet. Maternal fatty acids are deposited along the sides of the body, especially in association with muscles. These help the neonate larvae locomote in search of food (Atella and Shahabuddin, 2002; Sushchik et al., 2013). (D) “U” shaped metabolic curve of pupal stage. During this non feeding life stage, pupae use energy mainly from stored fats. If metabolic rate is measured with respect to rate of oxygen consumption, pupae show the least metabolic rate and have a characteristic “U” shaped curve (Chapman, 2013). (E) Adult mosquito biology. Both male and female mosquitoes can synthesize lipids from sugar meals. Females can do this faster than males. They also have de novo fatty acid biosynthetic machinery. Males have more active fatty acid synthase gene expression. This is reflective of dependence on de novo fatty acid synthesis to fulfill lipid requirements that are not fulfilled by the high sugar diet (Jenkin et al., 1975; Sushchik et al., 2013; Chotiwan et al., 2022) (F) Utilization of amino acids in the blood meal by female mosquitoes. A major proportion of the blood meal amino acids are oxidized to CO2 or excreted as uric acid while the remaining portion is converted to triglycerides (TAGs) and stored in the mosquito fat body (Lehane, 1991; Zhou et al., 2004a; Horvath et al., 2021). Deposited TAGs are used in oogenesis, metamorphosis, prolonged flight and other physiological processes. (Created with BioRender.com).
Energy and nutritional requirements of each life stage depend on the acquired meal which provides a unique repertoire of nutrients. As reviewed by Rivera-Pérez, Clifton and Noriega, 2017 nutritional requirements of a mosquito can be classified into two groups, macronutrients (Carbohydrates, fatty acids and amino acids) and micronutrients (vitamins, salts, sterols and metals) (Rivera-Pérez et al., 2017). In general, adult mosquitoes demand more energy for active flight and reproduction (Nayar and Van Handel, 1971). Lipids serve as the ideal energy source due to their high caloric value per amount of substrate as well as the ease of storage as anhydrous triglycerides (Downer and Matthews, 1976). Further, lipid metabolism plays a vital role in mosquito vitellogenesis and egg generation. Female mosquitoes transfer a major portion of lipids acquired via a sugar meal (prior to a blood meal) into the ovaries (Briegel et al., 2002). Due to these reasons, lipid metabolism plays a key role in efficient nutrient utilization in mosquitoes.
2.1 Eggs
Eggs are laid in water (oviposition). Being anautogenous insects (ex: Ae. aegypti), female mosquitoes require a vertebrate blood meal in order to produce eggs (Clements, 1992). Studies on eggs of other insects like Manduca sexta (tobacco hornworm) have shown that approximately 40% the dry mass of eggs represents lipids. Most of these lipids are acquired through maternal depositions and only 1% is generated in the egg (Canavoso et al., 2001). Provided that lipids have various functions, distribution of lipids in suitable tissues in the developing embryo is important for the emerging neonates (Atella and Shahabuddin, 2002).
After consumption of an adequate blood meal to facilitate ovarian development, female mosquitoes engage in the quest of finding suitable oviposition sites. Different mosquito species have varying preferences for oviposition sites. Ae. aegypti mosquitoes prefer freshwater habitats for egg laying while some species of Culex lay eggs in a wide range of sites from salt marshes to artificial containers. As reviewed by Bentley and Day, mosquitoes select their oviposition sites based on chemical and physiological cues at the site (Figure 1B) (Bentley and Day, 1989). Experiments on gravid colony Ae. aegypti mosquitoes have reported olfactory responses to fatty acid esters (Perry and Fay, 1967). A different study (Davis, 1976) reported one of the fatty acid esters, methyl propionate, as an active chemoattractant in oviposition. Additional studies have reported the influence of metabolites such as 7,11-dimethyloctadecane produced by the bacterium Pseudomonas aeruginosa as an oviposition attractant (Ikeshoji et al., 1975; Ikeshoji et al., 1979). According to a review by Bentley et al., there are multiple cues that are either of larval, pupal or adult origin that influence oviposition site selection in mosquitoes. These could be metabolites released from the previous life stages signaling the newly gravid mosquitoes the suitability of the site for safe oviposition (Bentley and Day, 1989).
2.2 Larvae
Eggs hatch to produce larvae that undergo four instar stages before developing into a non-feeding pupa. Neonate larvae acquire most of the lipids from the mother through the maternal deposition of lipids in eggs (Ziegler, 1997; Ziegler and Ibrahim, 2001; Atella and Shahabuddin, 2002). As discussed previously, there is a considerable functional variability between lipids. Sequestering appropriate lipids in suitable sites in the embryo is critical for the health of neonates (Atella and Shahabuddin, 2002). Using fluorescently labeled fatty acids and phospholipids, Atella and Shahabuddin were able to track the distribution of maternal lipids in developing mosquito eggs and larvae. They found that fatty acids were distributed along the sides of the larval body especially where the muscles are located, while phospholipids aggregated along the intestinal gastric caeca (Figure 1C) (Atella and Shahabuddin, 2002). The authors justify this distribution mentioning the different functions owned by the lipids. Fatty acids deposited alongside the larvae body, especially in association with muscles are assumed to provide energy to support locomotion and rapid movements of newly emerged larvae to find food. Maternal phospholipids that are accumulated in the motile gastric caeca secrete lubricants into the lumen of the gut. These are possibly aiding the neonate larvae in assimilating ingested food. Larvae further acquire lipids, especially the essential polyunsaturated fatty acids, from aquatic food sources such as diatoms and algae (Sushchik et al., 2013). These lipids are required for the proper functioning of innate immunity, developmental processes, and the ability to fly in their adult stage (Dadd and Kleinjan, 1979; Dadd et al., 1987; Stanley and Miller, 2006). Fatty acids that are acquired during larval stages are transferred to the adult stages. However, during metamorphosis, fatty acid conversions occur where eicasopentanoic acid (EPA) and Arachidonic acid (AA) are transferred to the adult mosquito from the triacylglycerol (TAG) stores of the larvae to generate more polar lipids (Sushchik et al., 2013). Further, studies have shown how larval diet can alter vector competence in the adult Ae. aegypti mosquito (Nasci and Mitchell, 1994; Muturi et al., 2011). Female Ae. aegypti mosquitoes developing from larvae that are fed with a high nutrient diet have presented larger body size which is related with a greater metabolic reserve (Briegel, 1990). The feeding success of these female mosquitoes on vertebrate hosts is also significantly greater. This suggests that better nutrition at larval stages can impact the adult vectorial capacity. Moreover, studies by Silva et al., 2021 has shown how higher larval rearing densities can elevate stored TAG levels within adult mosquitoes and also influence the size and fecundity of the mosquito (Silva et al., 2021).
2.3 Pupae
The pupae stage is a non-feeding period solely relying on energy stored at the larval stage. These energy reserves thus determine the ability of a newly emerged adult to survive, reproduce and transmit disease. If metabolic rate is determined by the rate of oxygen consumption of the organism, pupae show the least rate in comparison to larvae and the adult stages (Chapman, 2013). However, this metabolic rate fluctuates with time where it is initially high and then drops before it rises again by the time of eclosion (Chapman, 2013). The trend of metabolic variation is known to have a characteristic ‘U’ curve indicating the fall of metabolic rate initially at histolysis followed by an increase at histogenesis and differentiation (Figure 1D). Interestingly, the main source of energy is gained via fats (supplemented with a smaller portion of carbohydrates) during the pupal period (Chapman, 2013).
2.4 Adult mosquitoes
Adult mosquitoes are able to synthesize lipids from carbohydrate (sugar) meals (Ziegler and Ibrahim, 2001). Both males and females possess de novo fatty acid biosynthesis machinery, such as fatty acid synthase (FAS) and Δ-9 fatty acid desaturase enzymes, but males have higher FAS gene expression than females to fulfil lipid requirements (Figure 1E) (Jenkin et al., 1975; Sushchik et al., 2013; Chotiwan et al., 2022). By feeding on sugar meals alone, the females are capable of increasing their lipid content up to 300µg within 5 days (Ziegler and Ibrahim, 2001).
A blood meal taken by a female mosquito can induce a pronounced metabolic change in its physiological status (Figure 2A). This phenomena is known as a ‘ metabolic switch’ (Das De et al., 2022). Das De et al. compared the transcriptome of sugar-fed and blood-fed mosquitos using RNA-seq and showed that feeding blood (a high protein diet), induced expression of transcripts in the brain that are related to mitochondrial function and energy metabolism (Figure 2B) (Das De et al., 2022). Blood meals can also serve as an indirect source for lipids. In blood, lipids compose only about 4% of the nutrients (Lehane, 1991). Although no direct evidence has shown that the mosquito midgut epithelium can directly absorb lipids from the blood meal, increases in the expression of the genes that encode the proteins that absorb lipids from food, fatty acid binding protein and long chain fatty acid transport protein, have been reported (Sanders et al., 2003). The other 95% of nutrients in the blood meal are protein (Lehane, 1991). Using [14C]-labeled protein meals, Zhou et. al., have shown that approximately 30% of blood meal amino acids were oxidized to CO2 or excreted as waste (Figure 1F) (Zhou et al., 2004a). To determine how female Ae. aegypti mosquitoes detoxify ammonia that is generated during the oxidation of amino acids in a blood meal, mosquitoes were fed with labeled 15NH4Cl. The labeled 15N was traced in the whole mosquito body using electrospray ionization (ESI)-mass spectrometry and stable label isotope tracing (Horvath et al., 2021). The study showed that 15N was rapidly incorporated into glutamine (Gln) via glutamine synthase (GS) and with the aid of other enzymes, additional N-containing metabolites were generated in the mosquito (Horvath et al., 2021). However, 16% of the meal was converted to TAG, the storage lipid (Zhou et al., 2004a). The expression of several genes involved in lipid synthesis also increased after the blood meal was taken (Sanders et al., 2003). This finding provides more evidence that the blood meal can serve as a source of lipid reserves in mosquitoes. The reserve lipids are required for several physiological processes, such as oogenesis, metamorphosis, diapause, and prolonged flight (Zhou and Miesfeld, 2009; Arrese and Soulages, 2010; Sushchik et al., 2013). They also serve as a source for fatty acids which are precursors for synthesizing eicosanoids, pheromones, glycerophospholipids (GPs) and wax (Arrese and Soulages, 2010).
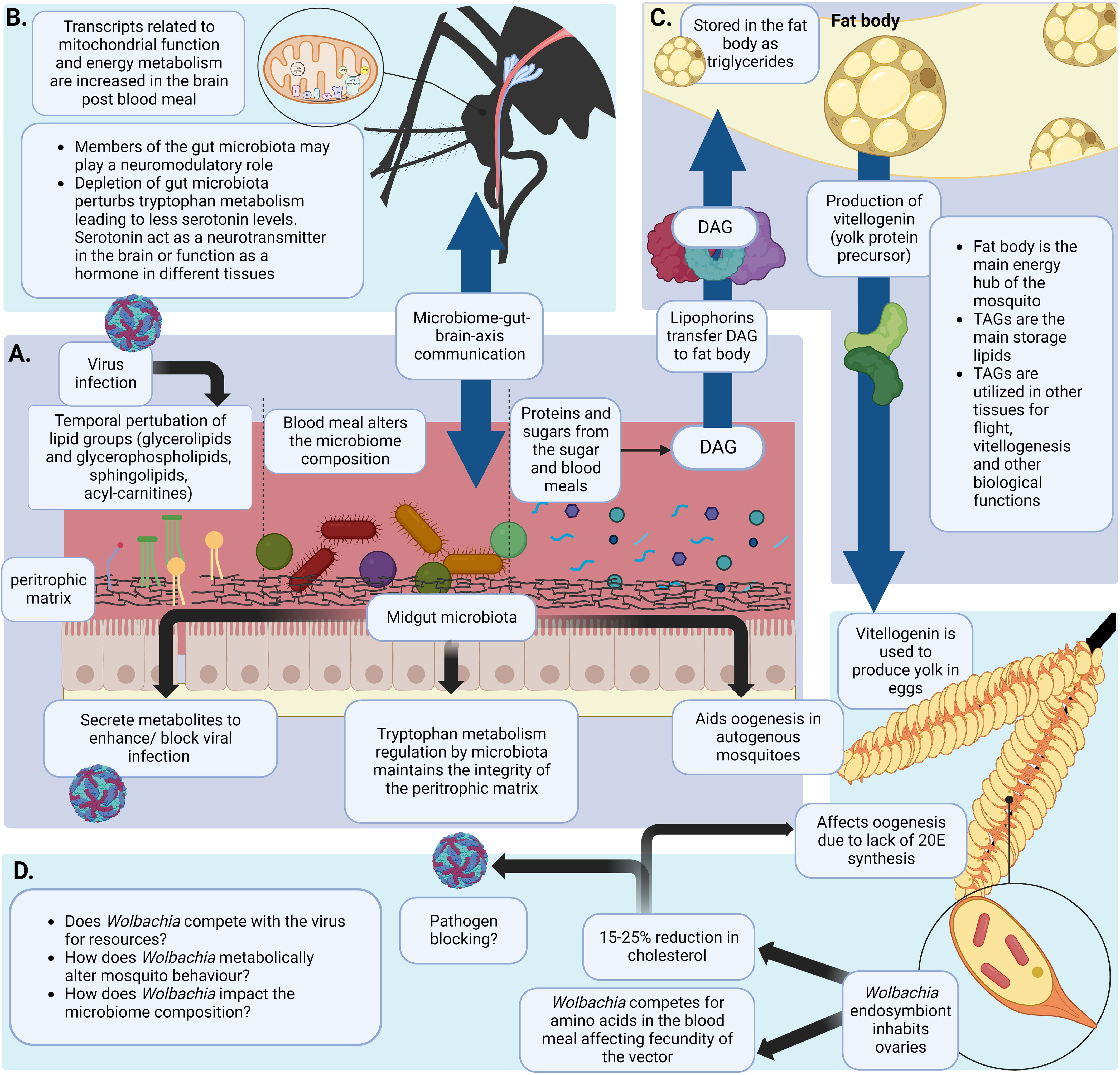
Figure 2 Blood meal induced metabolic changes in the mosquito. (A) The mosquito midgut acts as a major hub in metabolism related processes. It has a unique structure and a metabolic landscape that can be altered due to the blood meal. A virus entering the midgut via a blood meal and establishing infection can temporally alter the lipid landscape of the midgut (Chotiwan et al., 2018). The blood meal can also alter the gut microbiota diversity and composition. Gut microbiota are involved in metabolic processes like maintaining the integrity of the peritrophic matrix by regulating tryptophan metabolism. This is important to prevent Plasmodium spp. Infections (Okech et al., 2006; Lima et al., 2012; Feng et al., 2021; Bottino-Rojas et al., 2022). Microbiota also plays a role in enhancing/blocking viral infections by secreting metabolites or altering metabolic homeostasis (Apte-Deshpande et al., 2012; Ramirez et al., 2012; Apte-Deshpande et al., 2014; Ramirez et al., 2014). Nutritional resources required for egg production by autogenous mosquitoes are fulfilled by gut microbiota of the mosquito (Coon et al., 2016) (B) Intake of a protein rich blood meal activates the ‘metabolic switch’ in female mosquitoes. Following a blood meal brain transcripts related to energy metabolism and mitochondrial function were observed to be increased (Das De et al., 2022). Gut microbiome plays a significant role in gut-brain-axis of communication (Das De et al., 2022) (C) Proteins and sugars ingested in a blood and/or a sugar meal are digested and transported to the fat body of the mosquito by lipophorins. Yolk protein precursor-vitellogenin is produced using stored TAGs in the fat body. These proteins are transported to the ovaries for oogenesis (Ziegler and Vanantwerpen, 2006; Arrese and Soulages, 2010; Gabrieli et al., 2014; Wang et al., 2017) (D) Wolbachia is an endosymbiont mainly inhabiting the ovaries of the mosquito (Hilgenboecker et al., 2008; Zug and Hammerstein, 2012). Wolbachia is known to deplete cholesterol in the ovaries which is a precursor for 20E synthesis. This detrimentally affects oogenesis of the mosquito. Moreover, Wolbachia is shown to compete with the mosquito vector for amino acids ultimately affecting fecundity of the mosquito (248,25o,252). (Created with BioRender.com).
2.5 Lipid storage and mobilization
In the adult mosquito, the fat body is the central location for lipid synthesis, storage and degradation for energy production (Figure 2C) (Arrese and Soulages, 2010). It is an organ composed of loose tissues distributed throughout the insect body, lining the underneath of the cuticle and surrounding the gut and reproductive tissues (Dettloff et al., 2001). The majority of the cells in the fat body are adipocytes. These cells contain numerous lipid droplets which serve as the center of cellular lipid storage and energy metabolism (Olofsson et al., 2009). More than 50% of the dry weight of the fat body are lipids (Ziegler, 1991). The fat body also stores carbohydrates in the form of glycogen which constitutes about 25% of the dry weight. The rest of the carbohydrates (> 50% of intake glucose in Ae. aegypti) are oxidized or converted to lipids (Ziegler, 1991; Zhou et al., 2004b). This organ also serves as a source for synthesizing most of the hemolymph proteins. These proteins include lipophorin, the protein that is responsible for transporting lipids between cells or tissues, and vitellogenin, the protein that is required for egg maturation during oogenesis (Ziegler and Vanantwerpen, 2006).
Nutrients that are absorbed in the gut are transported to the fat body and converted to glycogen and lipids (Arrese and Soulages, 2010). Muscle cells only contain a small amount of energy reserves. As a result, the energy required for prolonged flight is provided by the fat body (Kaufmann and Brown, 2008). Less than 1% of lipids in eggs are locally synthesized. More than 80% of lipids in eggs are transferred from the fat body (Figures 2C, D) (Ziegler, 1997; Ziegler and Ibrahim, 2001).
Lipophorin is the main hemolymph lipoprotein. It plays a role as a reusable shuttle transporting lipids between tissues (Ziegler and Vanantwerpen, 2006). Similar to human high-density lipoprotein (HDL) and low-density lipoprotein (LDL), there are high- and low-density lipophorins (HDLp and LDLp) in insects. LDLp contains up to 63% of the lipids, while HDLp contains 30-50% of the lipids (Beenakkers et al., 1985). Apolipophorin I and II are integral components of the lipophorin particles whereas Apolipophorin III is transiently associated with the lipophorin particle (Van der Horst and Ryan, 2017). Lipophorins in most insects are enriched in diacylglycerol (DAG). However, lipophorins in mosquitoes and some other dipterans, but not Drosophila melanogaster (D. melanogaster) are enriched in triacylglycerol (Pennington et al., 1996; Pennington and Wells, 2002). The mechanism/s of lipid uptake from lipophorins into the oocytes are still unclear. Both receptor-mediated endocytosis of the intact lipoprotein particles and extracellular hydrolysis of lipids from the lipoprotein core have been observed (Ziegler and Vanantwerpen, 2006).
2.6 The gonadotropic cycle
Female mosquitoes require a considerable amount of energy and intense metabolic support during reproduction. The gonadotrophic cycle (egg production cycle) of an Ae. aegypti female is regulated by altering titers of two major hormones, juvenile hormone (JH) and a steroid hormone called 20-hydroxyecdysone (20E) (Attardo et al., 2005; Roy et al., 2015). The gonadotropic cycle has two phases. In the first phase, the posteclosion (PE) phase, JH regulates the development of the mosquito which drives physiological functions related to egg maturation and blood digestion. The female mosquito is physiologically prepared for blood meal digestion and egg maturation by this hormone (Wang et al., 2017; Ling and Raikhel, 2021). The fat body and ovaries need to be exposed to JH in order for the synthesis and accumulation of yolk protein precursor vitellogenin (Vg) (Gwadz and Spielman, 1973) following PE period (previtellogenic maturation), the vitellogenic phase starts with the mosquito taking a blood meal. During this post blood meal phase (PBM), the titers of JH are reduced while 20E titers are increased (Arrese and Soulages, 2010). Cholesterol ingested in a blood meal acts as a precursor of 20E synthesis (Clayton, 1964; Ekoka et al., 2021). Cholesterol stored in the prothoracic glands of the mosquito larvae and pupae can also be used to synthesize 20E (Jenkins et al., 1992). 20E regulates and supports blood meal digestion and egg development in female mosquitoes (Gabrieli et al., 2014; Wang et al., 2017). Interestingly, pathways related to carbohydrate metabolism were shown to be upregulated during the peak of 20E synthesis in females (18-24hPBM) (Hou et al., 2015). Later, Dong et al., have showed that 20E regulated the carbohydrate metabolism through a nuclear transcription factor HR38 (Dong et al., 2018). Additionally, studies by Hou et al. have demonstrated how major carbohydrate metabolic pathways (glycolysis, glycogen and sugar metabolism and the citrate cycle) were considerably downregulated in the mosquito fat body at PE (Hou et al., 2015). However, these pathways were upregulated at the PBM stage. In addition, TAG levels were also decreased at PE but elevated PBM.
Lipids that are synthesized in the fat body are transported and deposited in eggs. These lipids contribute to about 35% of the weight of Ae. aegypti oocytes (Troy et al., 1975). It should be noted that lipids that are synthesized from carbohydrate meals are not sufficient to trigger the maturation of oocytes. Blood meals, or to be specific, amino acids in the meal, are needed to trigger the release of vitellogenin stimulating hormone in the ovaries to initiate the maturation process of the oocytes (Hagedorn et al., 1979). Accumulation of lipids in the oocytes starts only after a blood meal is taken (Troy et al., 1975). Although ovaries are capable of synthesizing complex lipids, especially GPs, less than 1% of locally synthesized lipids were found in the egg (Ziegler and Vanantwerpen, 2006). Using radioactively labeled lipids, Ziegler et. al., found that the majority of the lipids in eggs were TAG that was transferred from the fat body (Ziegler, 1997; Ziegler and Ibrahim, 2001; Sanders et al., 2003).
Ae. aegypti possess a mechanism to maintain metabolic homeostasis during the gonotrophic cycle. Zhou et al., did not observe differences in lipid and protein content and the number of eggs laid from females that underwent starvation before a blood meal (Zhou et al., 2004b). However, they observed significantly lower lipid and glycogen content in the mother after the eggs were laid. This indicates a trade-off between fecundity of the mother and the quality of the eggs. Although a significant portion of lipids accumulating in the oocytes from the first gonotrophic cycle comes from larval food and pre-existing maternal stores (Zhou et al., 2004b), the ability to de novo synthesize fatty acids is still important to produce viable eggs. Transient knockdown (KD) of two key enzymes in the de novo fatty acid biosynthesis pathway, acetyl-CoA carboxylase (ACC) and fatty acid synthase (FAS), caused a significantly lower number of eggs in the first gonotrophic cycle (Alabaster et al., 2011). Eggs that were produced from ACC-deficient mosquitoes also lacked eggshells and were nonviable.
3 Mosquito immunity and metabolism
Mosquitoes mostly depend on innate immunity. Additionally, innate immune priming in mosquitoes can lead to memory like responses in mosquitoes. Mosquitoes release lipoxin/lipocalin complex as a result of immune priming (Ramirez et al., 2015). Since they are constantly exposed to a variety of microorganisms in varying habitats as well as blood meal sources, the mosquito innate immune system is well adapted to initiate a strong immune response against these foreign entities. Three major immune signaling pathways have been identified in mosquitoes; the Toll, Immune Deficiency (IMD) and Janus/kinase and signal transducers and activators of transcription (JAK-STAT). Besides these immune signaling pathways, the RNA interference (RNAi) pathway also plays an important role during antiviral defense although it is not considered as a classical immune signaling pathway.
Several studies in Drosophila melanogaster provide evidence on how metabolism and immunity are related in the fly. Activation of the IMD pathway in the fat body of D. melanogaster is associated with modifications in host metabolism. In a transcriptional analysis in Drosophila, activation of IMD pathway resulted in changes in expression of metabolism related genes of the fly. For example, genes responsible for the insulin signaling pathway and TOR (Target of Rapamycin) that responds to constant environmental changes and maintains energy, growth and developmental homeostasis of the fly were observed to decrease. These observations were further strengthened by reduction of expression of enzymes responsible for key metabolic functions including glycolysis and the TCA cycle, ATP generation by mitochondria and fatty acid β-oxidation (Davoodi et al., 2019). Under persistent IMD activation, the fly undergoes depleted fat reserves, hyperglycemia and impaired development (Davoodi et al., 2019). Further, IMD mutants showed hyperlipidemia, impaired insulin signaling and compromised glucose tolerance. Following these observations, the authors hypothesized that loss of metabolic regulation hindered the mounting of immune responses against microbial infections in the fly (Davoodi et al., 2019). Similarly, Martínez et al. has reported how TAG is diminished at the tissue level when the Toll signaling pathway is activated in the larval fat body of Drosophila (Martínez et al., 2020). The study also describes how enzymes of the Kennedy pathway, responsible for phosphatidylcholine and phosphatidylethanolamine homeostasis were increased upon activation of Toll signaling. In addition, transmission electron microscopy observations depicted how Toll signaling activation resulted in expansion of the endoplasmic reticulum (ER) volume in fat body cells. These observations provide compelling evidence that the metabolic landscape is intimately associated with immune signaling (Martínez et al., 2020).
Eicosanoids are fatty acid derivatives that can act as immunomodulatory molecules. They are mostly oxygenated metabolites of three C20 polyunsaturated fatty acids including arachidonic acid (20:4n-6), dihomo-gamma-linolenic acid (20:3n-6) and eicosapentanoic acid (20:5n-3). Eicosanoids are composed of 3 major groups of metabolites: prostaglandins, lipoxygenase metabolites, and epoxyeicosatrienoic acids (Stillwell, 2016). In insects, eicosanoids are known to mediate phagocytosis, micro aggregation, nodulation and encapsulation of invading microbes and metazoans (Stanley and Miller, 2006; Stanley and Shapiro, 2007). Since mosquitoes are unable to synthesize C20 polyunsaturated fatty acids, they require these fatty acids from diets (Blomquist et al., 1991; Sushchik et al., 2013).
All three groups of eicosanoid metabolites are found in mosquitoes (Petzel and Stanley-Samuelson, 1992; Ramirez et al., 2015; Xu et al., 2015). In Anopheles gambiae lipoxin A4 was found to be induced against the invasion of Plasmodium ookinetes in the midgut (Ramirez et al., 2015). The role of eicosanoids in mosquitoes against virus infection has only been reported in C6/36 cells (Ae. albopictus cells). Prostaglandin A1 was found to inhibit the replication of vesicular stomatitis virus in a dose-dependent manner (Burlandy et al., 2004). The role of eicosanoid metabolites in DENV infection of mosquitoes is still unknown. Chotiwan et al, 2018 observed that Prostaglandin A2 and D2 were upregulated in DENV2 infected Ae. aegypti midguts during early replication time points. Interestingly, DENV infection in human (Huh7) and dendritic cells induced the expression of cyclooxygenase-2 (COX-2), the enzyme that produces prostaglandin E2 (Wu et al., 2009; Lin et al., 2017). The production of prostaglandin E2 in infected cells was also enhanced and promoted migration of DENV infected dendritic cells from the upper to the lower chamber in culture (Wu et al., 2009). Mice that were treated with COX-2 inhibitor were protected from DENV infection (Lin et al., 2017). The role of prostaglandins in DENV infection in the mosquito remains to be investigated.
Autophagy is a cellular mechanism that removes unwanted debris and damaged organelles from a system. This process facilitates recycling of material as well as regeneration of newer cells. Another function of autophagy is intracellular pathogen clearance (Deretic and Levine, 2009). In insects, autophagy is important during metamorphosis, development, response to starvation as well as defense against pathogens (Tian et al., 2013; Romanelli et al., 2016; Tettamanti and Casartelli, 2019). However, viruses like DENV can seize this cellular mechanism to boost replication. Although the core mechanism is not well understood, experimental data reveals that autophagy induced by DENV modifies cellular lipid metabolism (Heaton et al., 2010).
Immunometabolism is a rapidly evolving discipline that investigates the relationship between metabolic homeostasis and immunity during infection. There is ample evidence to strengthen the argument of metabolic pathways being closely associated with cellular immune signaling pathways. However, immunometabolism is not well explored in arboviral vectors. There is a necessity to understand the mechanisms underlying the crosstalk between immune responses and cellular metabolic homeostasis. Such studies would provide a better understanding of the choke points that can be employed in pathogen blocking and vector control.
4 Metabolic processes are associated with senescence in mosquitoes
The normal life span of a wild mosquito can vary from approximately 10 to 60 days. Males have a shorter life span of nearly 10 days while females live longer for approximately 60 days (Ehrlich, 2022). However, these periods critically depend on environmental conditions such as temperature, humidity, and the availability of blood meals for females. As with any other organism, aging affects multiple physiological processes in mosquitoes. Digestion, mating, reproduction, flight and immunity are among some of the traits that are altered due to aging (Edman, 1970; Christensen et al., 1986; Hillyer et al., 2004; King and Hillyer, 2013; Sawadogo et al., 2013). In addition, aging can have a critical impact on the vectorial capacity of a mosquito. Any pathogen that is vectored by a mosquito needs to complete an extrinsic incubation period (EIP) prior to being transmitted (Cook et al., 2008). This EIP allows the pathogen to amplify within the vector. The inability of a mosquito to survive until the pathogen completes the EIP, renders a discontinuation in the transmission cycle. It is also important to note that with aging, immune responses of the vector might weaken thereby influencing vector competence (Boëte and Koella, 2003). Besides these alterations, metabolism of the mosquito is also prone to change since it is closely associated with the physiology of the organism. Discussed here are some of the key studies based on mosquito development, aging, and related metabolism.
4.1 Fatty acid synthesis
As discussed in this review, fatty acids are a group of vital lipids in mosquitoes serving as structural components in cellular membranes, energy homeostasis, signaling, innate immunity and reproduction. Fatty acid synthesis is conducted by a multifunctional enzyme complex called the fatty acid synthase complex (FAS) (Maier et al., 2008). In Ae. aegypti several paralogues of FAS were found (Chotiwan et al., 2022). The study investigated the dynamic expression of FAS genes in relation to developmental stages. Larval and pupal stages showed negligible FAS expression in comparison to adult stages (Chotiwan et al., 2022). This is consistent with the fact that larvae and pupae utilize maternal lipids deposited in the eggs and do not need to synthesize fatty acids in early life stages. All FAS genes except one isoform were highly expressed in adult male mosquitoes in comparison to other life stages. Since male mosquitoes do not feed on blood, they solely depend on nutrients taken up in a plant meal (nectar) and need FAS function to fulfil their lipid requirements (Chotiwan et al., 2022).
4.2 Glycogenesis and lipogenesis
Sugars obtained in the diet of mosquitoes are partially hydrolyzed by enzymes in the saliva when stored in the crop. During this temporal storage, salivary enzymes partially hydrolyze the ingested sugars to produce hexoses. Clements describes how these end products are utilized in the synthesis of glycogen (glycogenesis), fatty acids and triglycerides (lipogenesis) in proportions that are species and life stage specific (Clements, 1992). Several studies have also extensively investigated adult energy metabolism in multiple mosquito species and reported age specific trends of glycogenesis and lipogenesis (Briegel, 1990; Briegel and Timmermann, 2001; Ziegler and Ibrahim, 2001). Briegel et al., and Briegel and Timmermann investigated the accumulation of glycogen during the first week and lipids during the first two weeks in the adult life stages of Ae. aegypti and Ae. albopictus mosquitoes (Briegel, 1990; Briegel and Timmermann, 2001). In similar studies in Culex tarsalis, carbohydrates and lipids followed the same trend as in other mosquito species. However, lipid synthesis was more rapid than carbohydrate synthesis in C. tarsalis (Gray and Bradley, 2003). Further, C. tarsalis mosquitoes exhibited higher lipid storage trends in young adults in comparison to Ae. aegypti mosquitoes. These trends could be accounting for the autogenous potential of the Culex species which imply that the adults do not need a blood meal to lay eggs but can utilize lipid reserves (Gray and Bradley, 2003).
4.3 Glutathione Metabolism
Glutathione (GSH) is an important molecule for insects. This enzyme plays a critical role in a number of biosynthetic and detoxification reactions (Forman et al., 2009). Glutathione transferases (GST) are also important enzymes that play a role in detoxification of substances that can be both endogenous or xenobiotic. In insects, these enzymes are known to play a role in insecticide resistance (Enayati et al., 2005). Besides these functions, studies also discuss the alteration of GSH during aging of Ae. aegypti mosquitoes (Hazelton and Lang, 1983). The GSH biosynthesis rate was observed to be distinctly reduced in aging adult mosquitoes. Further, a considerable decrease in biosynthetic rates were observed during senescence of the mosquitoes (Hazelton and Lang, 1983). Impaired GSH biosynthesis leading to low levels of GSH implies that regular cellular functions of GSH such as detoxification of peroxides and xenobiotics will be impaired ultimately leading to tissue damage and death.
Vector control programs take the average age of a mosquito population as a crucial determinant of vectorial capacity and potential of disease transmission (Johnson et al., 2020). This makes mosquito age grading important in vector control. The technique in current use for age grading is the Detinova parity method that assesses the age of a female mosquito by taking the changes in ovary appearance into account (Gray et al., 2022). In addition, novel tools like surface – enhanced Raman spectroscopy (SERS) is currently being studied as a potential mosquito age grading technique (Wang D. et al., 2022). In a study by Wang et al., where age of a mosquito is determined by both SERS and Infrared spectroscopy states that key biological molecules are altered in a mosquito with age which subsequently alter the spectra obtained by either of the mentioned methods (Wang D. et al., 2022). However, in the field, there is a lack of capacity to accurately determine the age of a mosquito caught in the wild. Often the techniques used are impractical or unreliable (Johnson et al., 2020). Therefore, understanding metabolic changes occurring with aging in mosquitoes can be used to develop biomarker point-of-use tests for age grading as well as vector control (Iovinella et al., 2015).
5 Virus infection modulates metabolism in the mosquito
5.1 Barriers to infection
When a mosquito takes a viremic blood meal, the virus particles have to pass through several physical barriers in the mosquito in order to establish a successful infection, disseminate through the mosquito and be transmitted to a human host (Bosio et al., 2000). Infection of the midgut epithelium is the first barrier to infection. Presence of DENV, serotype 2 (DENV2) in the midgut tissue can be detected with the 3H5 monoclonal antibody as early as 2 days after the infectious blood meal was taken (Salazar et al., 2007). Staining at this early stage shows infected foci, indicating that the infection spreads laterally from the initial infected epithelial cells to the neighboring cells and eventually throughout the midgut (Salazar et al., 2007).
Upon successful infection of midgut epithelial cells, the virus must pass through the midgut escape barrier and continue to replicate in other tissues. Studies using electron-microscopy have shown that flaviviruses such as WNV and St. Louis encephalitis virus, escape from the midgut to the secondary tissues by passing through the basal lamina, the layer of extracellular matrix surrounding the midgut (Whitfield et al., 1973; Girard et al., 2005). Interestingly, a second non-infectious blood meal ingested by the mosquito enhances viral escape due to micro-perforations in the mid-gut (Armstrong et al., 2020) A study on DENV2 tropism in Ae. aegypti detected viral antigen in the trachea from the abdominal areas, suggesting that the trachea may also serve as an escape route for the virus from the midgut (Salazar et al., 2007). Following escape of the midgut barrier, the virus must replicate and amplify the infection in secondary tissues. Each of these tissues presents infection and escape barriers. A study has shown that DENV2 replicates in the fat body, hemocytes, nerve tissues, ommatidia of the compound eyes, esophagus, hindgut, cardia, trachea and Malpighian tubules (Salazar et al., 2007). Unlike WNV, DENV2 was not found to infect muscles (Girard et al., 2005; Salazar et al., 2007). Efficacious pass-through these barriers allows the virus to infect the salivary glands where the virus can be shed in the saliva when the next blood meal is taken to transmit to another host (Bosio et al., 2000).
5.2 Infection induced membrane rearrangements
In order for arboviruses to better survive in nature, they must evolve to survive in the invertebrate vector as well as the vertebrate host (Rückert and Ebel, 2018). Flaviviruses infect and rearrange the membrane architecture in their arthropod host cells like that observed in infected human cells (Girard et al., 2005; Welsch et al., 2009; Gillespie et al., 2010; Offerdahl et al., 2012; Junjhon et al., 2014; da Encarnação Sá-Guimarães et al., 2021; Mazeaud et al., 2021). Interestingly, these virus-induced membrane structures are morphologically and functionally conserved between these evolutionary distant hosts. These structures are summarized in Table 1. They are mostly endoplasmic reticulum (ER)-derived and include i) vesicles (Ve), the circular vesicular structures that house the viral replication complex, ii) vesicle packet (Vp), the larger vesicles that surround Ve, iii) convoluted membranes (CM), the site of viral protein translation and processing, and iv) tubular structures (T) with unknown function. In C6/36 cells infected with DENV2, Junjhon et al. observed Vp, Ve and T, with the number of Ve increasing with viral RNA copy number indicating a linear correlation between membrane structures and viral RNA replication (Junjhon et al., 2014). However, in contrast to DENV2 infected human Huh7 cells, CM were not found in infected C6/36 cells (Junjhon et al., 2014).
Similar vesicular structures were observed in mosquito cell lines infected with other flaviviruses. Electron micrographs revealed the induction of similar vesicular structures in Ae. albopictus cells infected with Kunjin virus and Ae. aegypti cells infected with yellow fever virus (Ishak et al., 1988). These membrane-rearrangements were also observed in WNV infected Culex quinquefasciatus mosquito tissues (Girard et al., 2005). The above-mentioned vesicle structures were observed in the midgut epithelium, midgut muscle and salivary gland tissues indicating that this specific membrane architecture was universally induced in both human and mosquito hosts in response to infection with most flaviviruses. In addition to these discussed structures, DENV infection of C6/36 cells was reported to produce exosomes that could infect naive C6/36 cells. Based on these observations, the authors suggested that virus induced exosomes have infectious potential and supports viral dissemination in C6/36 cells (Reyes-Ruiz et al., 2019). These studies on membrane architecture, together with the studies on lipid composition of infected cells, suggest that in addition to rearranging cellular membrane architecture during infection, existing membrane lipids are reorganized (Vial et al., 2020) as well as additional lipids (such as GPs and sphingolipids, SPs) are synthesized and incorporated into these membranes to promote expansion of membrane mass. Lipids with unsaturated fatty acyl chains and cone-shaped lipids such as PE, lysoGPs and ceramides (Cer) were increased likely to provide curvature and membrane-bending capabilities to facilitate the specific architecture required. Essentially, there is a concerted effort (by viral gene products) to alter both lipid metabolism and cellular membrane architecture to acquire an intracellular environment conducive to viral replication (Roosendaal et al., 2006; Miller et al., 2007; Perera and Kuhn, 2008; Perera et al., 2012).
5.3 Lipid metabolism and its impact on viral infection
Several studies to date have highlighted the impact of viral infection on the mosquito metabolic landscape (Table 2) (Perera et al., 2012; Melo et al., 2016). The initial study to utilize metabolomics to profile the metabolic landscape was carried out by Perera et al, on C6/36 Ae. Albopictus cells infected with DENV2. The study highlighted that ~15% of the metabolome was altered by infections of these cells. Lipid changes included those that had the capacity to alter membrane curvature and destabilize architecture, fluidity, and permeability. Specifically, GPs with smaller head groups such as phosphatidylethanolamine (PE) and cone-shaped (ie: ceramide) and inverse-cone shaped lipids (ie: lysophospholipids) were elevated in infected cells, specifically in membranes enriched in the replication complex. They also observed changes in SPs and glycerolipid intermediates such as monoacylglycerols (MAG) and DAG which are bioactive signaling molecules that participate in membrane fusion, fission, and trafficking and capable of enhancing a conducive environment for viral replication (Perera et al., 2012). These studies also demonstrated that like observations in human cells, de novo fatty acid biosynthesis via the enzyme, FAS, was important for viral replication in mosquito cells. Subsequently, a metabolomics study on Zika virus infected C6/36 cells identified 13 similar lipid species as specific biomarkers of infection (Melo et al., 2016). These included several species of SPs, GPs such as phosphatidylcholine (PC), phosphatodylserine (PS) and PE, as well as the bioactive intermediates such as DAG.
Interestingly, in an elegant study using isotopically labelled precursors, Vial et al, determined that at early time points, de novo biosynthesis of aminophospholipids such as PC and PE was actively blocked by DENV infection of Ae. Aegypti (Aag2) cells and instead that existing amino PLs were reorganized into replication complexes (Vial et al., 2020). These studies also demonstrated that in Ae. Aegypti mosquitoes, the rate-limiting enzyme that catalyzes aminoPL biosynthesis, acylglycerolphosphate acyltransferase (AGPAT), was decreased by DENV infection further supporting the hypothesis that aminoPL reorganization rather than de novo biosynthesis was activated during early infection (Vial et al., 2019)
In the adult mosquito, Chotiwan et al. demonstrated how lipid metabolism was temporally altered in infected Ae. aegypti mosquito midguts (the first site of viral replication) during infection with DENV2. In this study, GPs, SPs and fatty acids were significantly elevated and correlated temporally with the development of viral replication in the midgut (Chotiwan et al., 2018). GPs in insects play a critical role in tolerance to environmental changes (Guan et al., 2013). Elevation of glycerolipid intermediates suggested that resources were diverted from energy storage to biosynthesis during infection. Increased acyl-carnitines signaled functional disruptions in mitochondrial activities and energy production. Therefore, this study highlighted that significant metabolic perturbations occurred at early stages of viral replication in the mosquito.
5.3.1 Fatty acids and derivatives
Fatty acids are synthesized via the de novo fatty acid biosynthesis pathway and are precursors that are incorporated into complex lipid molecules. When fatty acids are linked to coenzyme A, they become activated and can be incorporated into complex lipids such as GPs, SPs and glycolipids (GLs) that can serve as structural components in membranes as well as bioactive molecules in cellular signaling. As independent entities, fatty acids and derivatives also have roles in signaling, energy homeostasis and the immune response.
Numerous studies in both mammalian and mosquito systems have shown that de novo fatty acid biosynthesis via FAS activity is a critical function required to support viral replication (Ishak et al., 1988; Roosendaal et al., 2006; Martıń-Acebes et al., 2011; Offerdahl et al., 2012; Nasheri et al., 2013; Tonglunan et al., 2017; Reyes-Ruiz et al., 2019; Vial et al., 2020; Chu et al., 2021; Liu et al., 2021; Mazeaud et al., 2021) Chotiwan, et al., 2018 also showed that numerous putatively identified fatty acids and derivatives were elevated in DENV infected Ae. Aegypti midguts compared to controls. Species such as fatty amides, hydroxy fatty acids, fatty amines, glycosides, dicarboxylic acids, keto fatty acids, eicosanoids and leukotrienes were detected (Chotiwan et al., 2018). Eicosanoids are known to be players of immunity in insects (Stanley and Miller, 2006). Prostaglandin A2, prostaglandin D2 (PGD2), PGD2-dihydroxypropanylamine and thromboxane, eicosanoid subspecies, were elevated in DENV infected mosquito midguts (Chotiwan et al., 2018). These molecules are known to have a potential signaling function in Drosophila (Tortoriello et al., 2013). Unfortunately, unlike in mammalian systems, our current knowledge on these numerous bioactive molecules in the mosquito are limited to detection and quantification following exposure to virus infection. Future studies will need to elucidate the exact mechanisms of how these molecules might function to support or limit viral infection in the mosquito vector.
5.3.2 Acyl-carnitines
Acyl-carnitines are esters of L-carnitine and fatty acids and belong to a large class of metabolites that are also identified as non-protein amino acids. These molecules act as intermediates that shuttle fatty acyl-CoA from the cytoplasm into the mitochondria for β-oxidation and energy production. These are known to be critical regulators of energy conservation in diapausing mosquitoes (Batz and Armbruster, 2018)High resolution liquid chromatography mass spectrometry analysis of DENV2 infected Ae. aegypti midguts have revealed that numerous acyl-carnitines were significantly increased following infection. Interestingly, many of these elevated molecules had medium chain fatty acids (Chotiwan et al., 2018). Medium length fatty acyl chains are generated due to incomplete β-oxidation resulting from mitochondrial overload (Koves et al., 2008). Two hypotheses were presented: i) accumulation of acyl-carnitines during viral infection could be caused by stalling of their transport into the mitochondria resulting in a blockage or inhibition of β-oxidation. This is observed in cells exposed to hypoxia as well as in mammalian systems during DENV infection (Knabb et al., 1986; Kler et al., 1991; El-Bacha et al., 2007; Bakermans et al., 2013; Fontaine et al., 2015). This scenario could result in lipid partitioning and diversion of fatty acyl-CoAs into complex lipids required for virus-induced membrane expansion, at the expense of fatty acid oxidation, ii) Alternately, the accumulation of medium chain length acyl-carnitines could be due to a bottleneck caused by a large proportion entering the mitochondria inducing mitochondrial overload. This results in only a proportion of the molecules being processed via β-oxidation. Future studies will need to explore the molecular mechanisms of these scenarios and determine how mitochondrial energetics relate to viral infection success.
5.3.3 Sphingolipids
SP are critical for structural integrity of cellular membranes. However, they also play critical roles as bioactive signaling molecules involved in stimulating many processes in the cell (Hannun and Obeid, 2008). In insects, the best-known information on SPs is from studies in Drosophila (Acharya and Acharya, 2005). These studies have shown that SPs are involved in the regulation of energy homeostasis, fat body metabolism, phototransduction, brain development and behavior (Acharya et al., 2008; Dasgupta et al., 2009; Bauer, 2010; Kohyama-Koganeya et al., 2011; Kraut, 2011; Chen et al., 2016). The functions of SPs in Ae. species are less well studied (Townsend et al., 1972; Jenkin et al., 1975; McMeans et al., 1975). Studies by our group on Ae. albopictus (Perera et al., 2012) and Ae. aegypti cells and Ae. aegypti mosquitoes (Chotiwan et al., 2018) have revealed a significant perturbation of SPs during infection with dengue viruses with many molecular species elevated and required for infection. Specifically, Chotiwan et al. demonstrated that a central hub in the SP pathway that interconverted dihydroceramide to ceramide was required for the virus life cycle. Additional studies on the impact of Wolbachia on SP metabolism in Ae. aegypti are discussed below.
5.3.4 Cholesterol
Cholesterol was shown to be essential for flavivirus entry, replication and assembly in human cells (Mackenzie et al., 2007; Lee et al., 2008; Rothwell et al., 2009; Carro and Damonte, 2013). Manipulation of cholesterol biosynthesis either by RNA interference (RNAi)-mediated gene silencing of cholesterol biosynthesis genes or using inhibitors of cholesterol biosynthesis enzymes such as lovastatin reduced DENV and WNV replication in human cells (Mackenzie et al., 2007; Rothwell et al., 2009). Intracellular availability of cholesterol was also shown to facilitate successful DENV replication in mosquito cells and mosquito vectors (Tree et al., 2019). Mosquitoes cannot synthesize cholesterol de novo and need to acquire cholesterol exogenously such as from the microbiome or from food (Clayton et al., 1964). As a result, mosquitoes rely on the processes for cellular absorption, trafficking and metabolism of cholesterol. Transcription and protein expression of host factors that are involved in cholesterol trafficking and homeostasis were increased upon DENV infection of Aag2 cells, indicating that these cellular factors were viral agonists (Fu et al., 2015). Sterol carrier protein-2 (SCP-2) is a cytosolic protein involved in cholesterol binding and transport in mammalian cells (Krebs and Lan, 2003; Vyazunova and Lan, 2010). Studies by Fu et. al., found that inhibition of SCP-2 using RNAi mediated gene silencing or the SCP-2 inhibitor (N-(4-{[4-(3,4-dichlorophenyl)-1,3-thiazol-2-yl]amino}phenyl)acetamide hydrobromide) altered the cellular distribution of free cholesterol and also significantly reduced DENV titers in Aag2 cells (Jupatanakul et al., 2014; Fu et al., 2015). Genome-wide transcriptomic analyses of Ae. aegypti (Liverpool strain) revealed that the transcripts of members in the lipid-binding protein gene families, the myeloid differentiation 2-related lipid recognition protein (ML) and Niemann Pick type C1 (NPC1) families, were increased upon DENV infection (Nene et al., 2007; Jupatanakul et al., 2014). These proteins function in cholesterol absorption, trafficking and metabolism in mosquitoes (Jupatanakul et al., 2014). Loss-of-function studies using RNAi mediated gene silencing of these genes reduced DENV infection in the midgut of both lab-adapted and field-derived strains of Ae. aegypti (Jupatanakul et al., 2014). Lastly, Geoghegan et. al., have shown that Wolbachia, an intracellular endosymbiotic bacterium, inhibited DENV in Ae. aegypti cells by perturbing cholesterol trafficking and causing accumulation of cholesterol in lipid droplets (Geoghegan et al., 2017). A compound, 2-hydroxyorioyl-β-cyclodextrin, that restores lysosomal cholesterol accumulation in Niemann-Pick type C disease rescued DENV replication in Wolbachia-infected mosquito cells (Liu, 2012; Geoghegan et al., 2017). In summary, these studies have shown the importance of cholesterol metabolism and intracellular trafficking, which play agonist roles facilitating DENV infection and replication in mosquito vectors.
5.3.5 Lipid droplets and lipid reserves
Lipid droplets (LDs) are ER derived organelles that store neutral lipids like TAGs and sterol esters (Tauchi-Sato et al., 2002). They have a hydrophobic core consisting of these neutral lipids and are surrounded by a phospholipid monolayer associated with a specific repertoire of proteins (Brown, 2001; Bartz et al., 2007; Olzmann and Carvalho, 2019). These proteins have multiple functions and belong to numerous protein families such as enzymes involved in lipid synthesis (Kuerschner et al., 2008; Stone et al., 2009), lipolysis and membrane trafficking and proteins involved in maintaining structural integrity (Brasaemle, 2007; Guo et al., 2009). Recently, LDs were fully entitled as organelles with the primary function of lipid and energy homeostasis (Farese and Walther, 2009). LDs also serve the purpose of shielding the cell from toxic effects of excess lipids by compartmentalization of lipids. Mosquitoes store lipids acquired from the blood meal in lipid droplets (LDs). While LDs are found in almost all tissues in the mosquito, they are enriched in adipocytes in the fat body. These LDs can have dynamic sizes depending on the nutritional and metabolic status of the mosquito (Pinch et al., 2021). Lipids in LDs can be catabolized to generate energy via β-oxidation, provide building blocks for membrane biogenesis and bioactive molecules for signaling. Studies in Drosophila have shown that LDs are also players in intracellular protein metabolism (Cermelli et al., 2006; Farese and Walther, 2009).
TAGs accounts for the most common storage lipid in insects (Arrese and Soulages, 2010). In mosquitoes, TAGs from LDs and other tissues in the fat body are transported between tissues in the rest of the mosquito via lipophorins (Ford and Van Heusden, 1994; Van Heusden et al., 1997; Pennington and Wells, 2002). DAGs are lipolytic products of TAG and are believed to be the major class of transported lipids in most other insects. DAGs serve as intermediates in GP synthesis (van Meer et al., 2008; Sarri et al., 2011) and play a key role as a second messenger that regulates cell proliferation, mitochondrial physiology, apoptosis and survival (Mérida et al., 2008; Lin et al., 2014).
In addition to the above discussed functions, LDs are also known to have a relationship with activating Toll-like receptors (TLR), during DENV infection (Barletta et al., 2016). Barletta et al., have shown that LDs accumulated in Aag2 cells when challenged with DENV or Sindbis virus or a bacterial pathogen (Barletta et al., 2016). Further, LDs were observed to accumulate in midgut cells of mosquitoes as a response to bacterial or viral infection. Interestingly, this accumulation of LDs in the mosquito midgut cells occurred when the native microflora was proliferating after a blood meal (Barletta et al., 2016). As proposed by Barletta et al., buildup of LDs in infected mosquito cells and tissues is suggestive of an immune role played by LDs. Alternately, it could also serve as an energy reserve for the microflora. Additional research needs to be conducted to fully unravel the coupled relationship between insect immune responses and LDs.
Studies have reported increased numbers of LDs in dengue infected cells in response to the virus. This observation was seen in both mammalian cells (BHK, HepG2) and mosquito cells (C6/36 and Aag2) (Samsa et al., 2009). It was proposed that there is a possible crosstalk between viral replication and LD metabolism. The authors demonstrated that DENV replication was disrupted when LD formation was pharmacologically inhibited (Samsa et al., 2009). Concurrently, Heaton and Randall showed that DENV infection induced autophagy to regulate cellular lipid metabolism. This study observed an increase in the number of LDs but they were much smaller in size. The authors hypothesized that LD stored lipids like TAGs were being broken down to free fatty acids that could be shunted to increase cellular β-oxidation and generate more ATP required for virus replication (Heaton and Randall, 2010).
In summary, LDs as metabolic organelles play a critical role in mosquito lipid storage, transport, metabolism and energy homeostasis. Evidence suggest that they may also have a role in immunity that impacts both the microbiome and possibly pathogen transmission. While our knowledge of LDs has been gathered from studying these organelles in mammalian systems, there is a critical gap in our understanding of the molecular mechanisms that drive LD formation, activation and metabolism in relation to the needs or responses of the mosquito to environmental queues, the microbiome and pathogen infection.
6 Vector control, pathogen blocking and metabolism
As discussed above, metabolic homeostasis is important for proper biological functions of an organism. Every stimulus including environmental changes (temperature, humidity), exposure to insecticides, the microbiome including transinfected microbiota (Wolbachia) can alter the metabolic landscape. These altered metabolic landscapes can have significant impact on obligatory pathogens such as viruses that require resources of the host to carry out successful replication. In this section we discuss the metabolic impact of insecticides, insecticide resistance, the microbiome and biocontrol strategies such as Wolbachia on the mosquito and vector competence.
6.1 Insecticide resistance is integrated with metabolic changes in the mosquito
Vector control has been critical in the prevention and control of several vector-borne diseases. The use of insecticides to kill or deter vectors has been the mainstay of vector control globally (van den Berg et al., 2021). Intense insecticide use has selected mechanisms of resistance that are now prevalent in malaria vectors such as Anopheles gambiae, Anopheles sinensis, and Anopheles funestus (Hancock et al., 2018), as well as arbovirus-vectors such as Ae. aegypti and Ae. albopictus (Moyes et al., 2017). Insecticide resistance mechanisms include decreased cuticular penetration of insecticides, increased enzyme metabolism, and decreased sensitivity of insecticide target sites (Oppenoorth, 1984; Scott, 1990). The mechanisms of metabolic resistance will be the focus of this section (Figure 3).
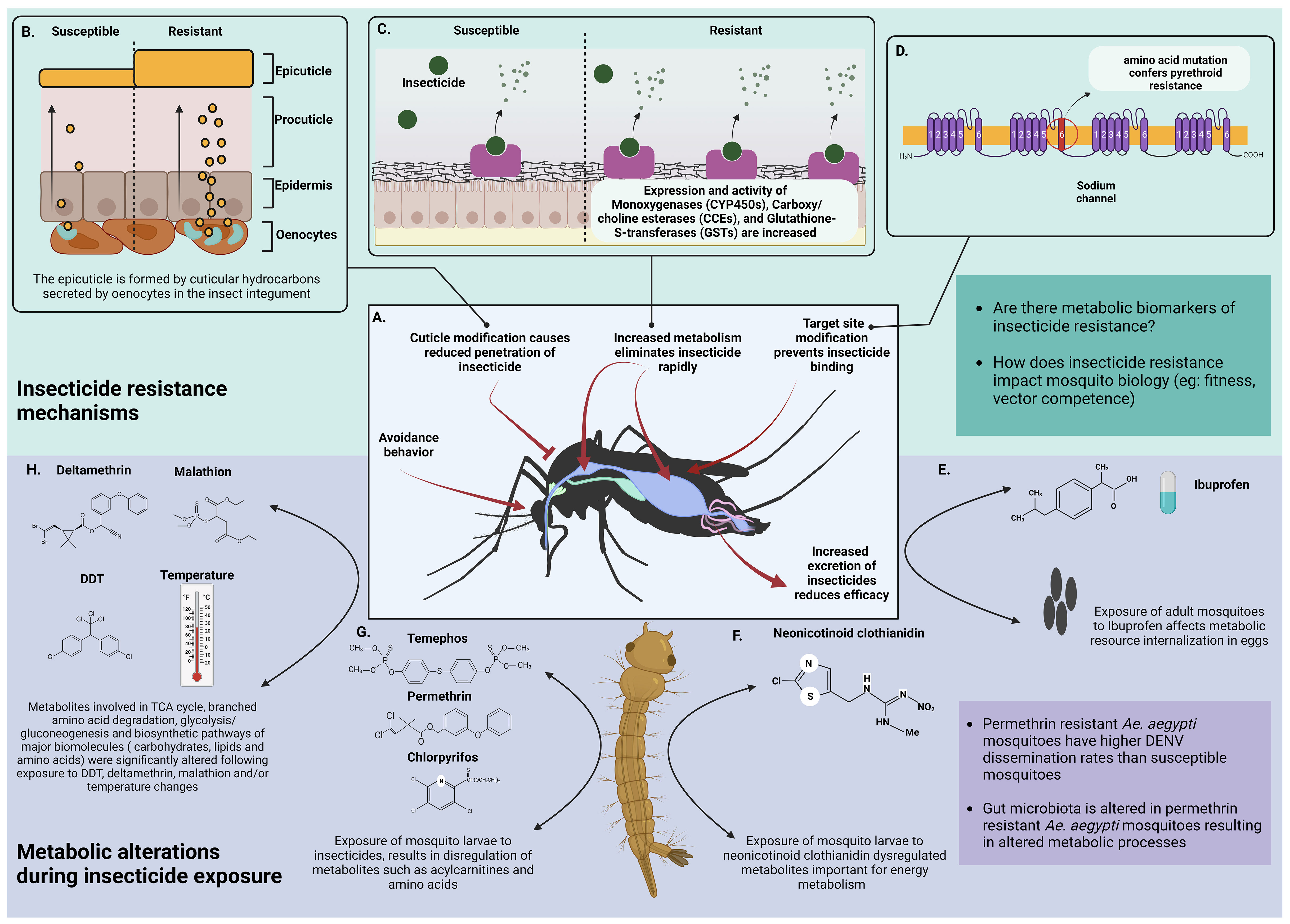
Figure 3 Metabolic basis of insecticide resistance. (A) General strategies of insecticide resistance shown by mosquitoes. (B) Resistance is acquired through modification of the integument by thickening the cuticle (image adapted from Bass et al., 2016) (Bass and Jones, 2016) (C) Insecticide resistant mosquitoes show higher expression and activity of esterases. These enzymes rapidly bind with incoming insecticide molecules and metabolize them thereby inhibiting their activity. The impact of the metabolites following insecticide degradation on mosquito metabolism is unknown. (D) Mosquitoes acquire insecticide resistance by mutating the targeted binding sites (Oppenoorth, 1984; Scott, 1990) (E) Direct exposure of adult mosquitoes or larvae to Ibuprofen (an ubiquitous surface water contaminant) had no direct effect but it dysregulated internalization of metabolic resources like amino acids, carbohydrates, polyols, phosphoric acid and ornithine in F1 progeny (Prud’homme et al., 2018) (F) Culex pipiens larvae exposed to varying concentrations of neonicotinoid clothianidin had differences in metabolites important for energy metabolism (acylcarnitines, GPs and biogenic amine abundance). Higher concentrations of clothianidin reduced acylcarnitines, GPs and biogenic amines after 24 hours of exposure. Low and medium concentrations of clothianidin reduced GPs and amines but increased acylcarnitines indicating that low pesticide doses increased energy requirements of exposed larvae (Russo et al., 2018) (G) Exposure of Culex quinquefasciatus larvae to Temephos, Permethrin and Chlorpyrifos dysregulated acylcarnitines and the amino acid arginine. Arginine levels were increased following exposure to Permethrin and Temephos whereas acylcarnitines responded differently to each insecticide (Martin-Park et al., 2017) (H) Major biological pathways are altered due to exposure of mosquitoes to insecticides such as DDT, deltamethrin and malathion and/or temperature (Singh et al., 2022) Created with BioRender.com.
Metabolic resistance protects against all insecticides used in public health, including pyrethroids, organophosphates, carbamates, and organochlorines (Smith et al., 2016) Insecticide metabolism is conducted by three enzyme families, cytochrome P450 monooxygenases (P450), glutathione transferases (GST), and carboxy/cholinesterases (CCE) (Strode et al., 2008; Feyereisen, 2012). Enhanced metabolism in resistant insects can be caused by gene over-expression (cis/trans regulation and gene amplification) or allelic variation of members of these enzyme families.
Because of the complexity of the enzyme family systems and the difficulty in purifying these enzymes (e.g., substrate overlap, instability, yields), understanding the mechanisms of resistance have proved difficult. The development of molecular and bioinformatics tools enabled the identification of genes and associated regulatory processes in resistant insects resulting in significant progress over the last decade. Several studies have investigated the differential gene expression of CYPs, GSTs, and CCEs in resistant Anopheles, Culex, and Aedes mosquitos (Smith et al., 2016; Moyes et al., 2017; Vontas et al., 2020). However, relatively few detoxification enzymes have been examined in vitro to validate their ability to metabolize insecticides. For example, CYP6P3, CYP6M2, and GSTE2 in An. gambiae have shown to metabolize pyrethroids, DDT, and bendiocarb (Müller et al., 2008; Stevenson et al., 2011; Mitchell et al., 2012; Yunta et al., 2019). Pyrethroids are metabolized by CYP6P9a and CYP6P9b in An. funestus (Riveron et al., 2014) and by CYP9M6, CYP6BB2, CYP9J24, CYP9J26, CYP6J28 and CYP9J32 in Ae. aegypti (Mitchell et al., 2012; Kasai et al., 2014). The role of these enzymes in pyrethroid resistance has been validated in vivo utilizing heterologous expression in Drosophila (Pavlidi et al., 2012; Edi et al., 2014; Reid et al., 2014; Riveron et al., 2014; Ibrahim et al., 2015) or RNA interference (RNAi) technologies in Ae. albopictus (Xu et al., 2018). A GAL4/UAS expression system was recently developed in An. gambiae to confirm in vivo that overexpression of GSTE2 conferred organophosphate and organochlorine resistance, CYP6P3 conferred pyrethroid and carbamate resistance, and CYP6M2 conferred pyrethroid resistance when overexpressed in the same tissues (Adolfi et al., 2019).
Metabolic technologies have recently been utilized to investigate the mosquito’s reaction to insecticides and to find metabolic routes of insecticide detoxification. For example, Prud’homme et al used targeted gas chromatography mass spectrometry (GC/MS) in conjunction with transcriptomics to assess the effect of ibuprofen on Ae. aegypti (Prud’homme et al., 2018). Direct ibuprofen exposure in larvae and adults had no effect on the 53 quantified polar metabolites, but F1 eggs from ibuprofen-exposed parents had lower levels of amino acids, carbohydrates, polyols, phosphoric acid, and ornithine, implying that ibuprofen exposure affected metabolic resource internalization in eggs (Prud’homme et al., 2018).
The profile of 12 amino acids and 31 acylcarnitines in Culex quinquefasciatus larvae treated with chlorpyrifos, temephos, and permethrin was determined using liquid chromatography tandem mass spectrometry (LC-MS/MS) (Martin-Park et al., 2017). Two acylcarnitines (C0 and C2) and the amino acid arginine were shown to be differentially associated with insecticide exposure. C0 concentrations were considerably higher in permethrin-exposed larvae, whereas C2 concentrations increased in permethrin-exposed but dropped in temephos-exposed larvae. Permethrin and temephos exposure enhanced arginine levels in larvae (Martin-Park et al., 2017). Studies have also shown that permethrin resistant mosquitoes have altered gut microbiota in comparison to the wild type and therefore have altered metabolic processes (Muturi et al., 2021).
In a separate study, Culex pipiens larvae subjected to varying concentrations of the neonicotinoid clothianidin showed differences in three groups of metabolites important in energy metabolism, including acylcarnitines, glycerophospholipids (GPs), and biogenic amine abundance (Russo et al., 2018) The unipolar and polar metabolites were quantified using flow injection tandem mass spectrometry (FIA-MS/MS) and LC-MS/MS, respectively. The highest dosage of clothianidin reduced acylcarnitines, GPs, and biogenic amines after 24 hours of exposure. Low and medium amounts reduced GPs and biogenic amines while increasing acylcarnitines. GPs and acylcarnitines were reduced at low and medium concentrations after 48 hours of exposure. These findings imply that low pesticide doses raised the energy requirements of exposed species (Russo et al., 2018).
Nuclear magnetic resonance (NMR) spectroscopy was utilized in Ae. aegypti to discover changes in metabolites caused by temperature and/or exposure to DDT, malathion, and deltamethrin. Metabolites involved in the tricarboxylic acid cycle, branched amino acid degradation, glycolysis/gluconeogenesis, amino acid, lipid and carbohydrate, nucleotide PRPP pathway, and phospholipid metabolism were the most affected (Singh et al., 2022). Seven of the nine discovered compounds were shown to be significantly altered by individual temperature and insecticide exposure. These included pyruvates, maltose, citrate, nicotinate, and -hydroxybutyrate, all of which are components of the glycolysis/gluconeogenesis, tri-carboxylic acid cycle energy producing pathways.
A recent study used LC-MS/MS to compare the metabolites of deltamethrin resistant and susceptible An. sinensis in larva and adult stages. Deltamethrin exposure resulted in the identification of 127 and 168 distinct metabolites in larvae and adults, respectively. Organooxygen compounds, carboxylic acids, GPs, and purine nucleic acids were shown to be different between resistant and susceptible mosquitos. The GPs route was shared by resistant larva and adults, and it may play an essential role in the metabolic deltamethrin detoxification (Li et al., 2022).
Pyrethroid resistance is typically associated with biological fitness costs, including size, longevity, fecundity, and mating behavior (Brito et al., 2013; Smith et al., 2016; Vera-Maloof et al., 2020). Moreover, pyrethroid resistance may influence the mosquito’s inherent permissiveness to viral infection, replication, and transmission. Few studies have reported the association between pyrethroid resistance and Aedes vectorial competence (VC), and the outcomes have been widely inconsistent among virus, mosquito strains and stage of viral infection. Few studies have evaluated the relationship between vector competence (VC) and pyrethroid resistance in laboratory Ae. aegypti (Zhao et al., 2018; Chen et al., 2020; Parker-Crockett et al., 2021; Stephenson et al., 2021; Wang L. et al., 2022) and Ae. albopictus strains (Richards et al., 2017; Chen et al., 2021). The direction of VC response differed among virus, viral replication stage, and mosquito strains. For example, VC for ZIKV (Zhao et al., 2018; Parker-Crockett et al., 2021) and DENV (Chen et al., 2021) was higher in the pyrethroid resistant strain. In contrast, three investigations have shown that the pyrethroid-resistant strains had significantly lower viral infection rates than the susceptible strains (Deng et al., 2021; Stephenson et al., 2021; Wang L. et al., 2022). Interestingly, only one study found that high kdr allele frequencies were associated with lower DENV-infection rates in field populations in Florida (Stephenson et al., 2021). As more studies elucidate the specific interactions between arboviruses and field resistant mosquitoes, understanding the mechanisms of interaction in specific mosquito tissues remains to be explored.
6.2 The gut microbiome influences metabolic homeostasis in the mosquito
Recent studies on the mosquito microbiome have highlighted its critical impact on various stages of the mosquito life cycle including development and reproduction as well as ecological adaptation, pathogen infection, immunity and vector competence (Figure 2A). Increasing evidence suggests a critical metabolic inter-dependency between the microbiome and the mosquito vector that drive the resulting outcomes with detrimental effects if the metabolic relationship is perturbed.
The metabolic impact of the microbiome is evident throughout the life cycle of the mosquito. A comparison of Ae. Aegypti (requires a blood meal for egg production) versus Ae. Atropalpus (does not require a blood meal for egg production) identified that the microbiome played a crucial role in providing the nutritional resources required for Ae. Atropalpus egg production in the absence of a blood meal (Coon et al., 2016). Studies have also shown that bacterial microbiota in aquatic habitats significantly impact larval nutrient acquisition and/or assimilation influencing growth, development, and survival into pupation (Coon et al., 2017; Vogel et al., 2017; Correa et al., 2018; Valzania et al., 2018). These studies compared transcriptional responses in axenic (bacteria free) larvae and larvae containing either native or gnotobiotic (single species) microbiomes and discovered that processes such as amino acid transport, hormonal signaling, and metabolism were differentially expressed, suggesting a key role in larval development and survival. Axenic larvae exhibited delayed development time and stunted growth in comparison to their bacterially colonized counterparts.
A study by Feng et al., demonstrated the influence of gut microbiota of Anopheles stephensi (a potent malaria vector) on tryptophan metabolism. The study reported how elimination of gut microbiota via antibiotics increased accumulation of tryptophan and its metabolites (kynurenine and 3-hydroxykynurenine, 3H-K) in the mosquito with high levels of 3H-K leading to structural impairments in the peritrophic matrix which in turn facilitated Plasmodium berghei infection (Feng et al., 2021). Similar observations were also made by Das de et al (Das De et al., 2022),. Blood meal digestion also increased these specific metabolites in the midgut, and the gut microbiome was critical for the catabolism that maintains normal levels of these metabolites. In a second study, Bottino-Rojas et al., showed that the product of the kynurenine pathway, xanthurenic acid, was critical for controlling levels of microbiota as well as reproduction and survival of the mosquito (Bottino-Rojas et al., 2022). Mutations in orthologs of kynurenine hydroxylase impaired reproduction and survival in Ae. aegypti, An. stephensi and Culex quinquefasciatus as well as disrupted the midgut permeability barrier in An. stephensi. Kynurenine is a tryptophan metabolite. Together these studies suggest that tryptophan catabolism is critical for maintaining the integrity of the peritrophic matrix, preventing infection, maintaining normal levels of the gut microbiome, and ensuring survival and reproduction of the mosquito vector (Okech et al., 2006; Lima et al., 2012; Bottino-Rojas et al., 2022). Interestingly, tryptophan metabolism is a key pathway altered in the human metabolome during severe disease caused by DENV infection. Serotonin (another product of tryptophan metabolism) and kynurenine were identified to show differential abundance in DENV-infected patient serum. Serotonin levels were significantly lower in patients with dengue hemorrhagic fever (DHF) than those in the febrile phase (Cui et al., 2016).
On the other hand, there is evidence to show that metabolism of the mosquito influences the modulation of microbial density in different Ae. aegypti strains, in turn altering vector competence. A study by Short et al., demonstrated how specific metabolic pathways such as the branched chain amino acid (BCAA) degradation pathway can influence mosquito midgut microbiota. When the BCAA degradation pathway was silenced in two mosquito strains with broadly differing gut microbiota, a significant alteration in microbiota composition was observed in both strains. This resulted in levelling the variation between the two microbiomes. The authors hypothesize that variations in amino acid metabolism can be a crucial determinant of microbiota in a vector (Short et al., 2017). In relation to these results, there are previous studies that have shown how alterations of the mosquito midgut microbiota can alter vector competence in Ae. aegypti mosquitoes. An increase in DENV titers was observed in Ae. aegypti mosquitoes after reduction of bacterial loads in the midgut through antibiotic treatment (Xi et al., 2008). Moreover, introduction of multiple bacterial species into a native mosquito gut microbiome has been shown to decrease DENV titers (Ramirez et al., 2012; Ramirez et al., 2014). However, there is also evidence to show how specific midgut bacterial populations can enhance susceptibility of a vector to DENV and CHIKV (Apte-Deshpande et al., 2012; Apte-Deshpande et al., 2014). These studies highlight gaps that remain in our understanding of the mechanisms underlying the microbiome-mosquito-pathogen multipartite interactions that result in the outcomes observed, stimulating further studies.
6.3 Wolbachia as a control strategy impacts mosquito metabolism
As discussed earlier, vector control is an efficient way of preventing the spread of arboviruses. However, during the past two-three decades, the major vectors of these arboviruses have expanded their geographic range and population size (Kraemer et al., 2015). Rapid urbanization, climate changes and development of resistance to insecticides are some of the major factors governing the emergence of many medically important arboviruses. Efficient, vector control strategies are considered necessary to overcome these problems. In the last decade, interventions with biocontrol agents like Wolbachia have revolutionized the field of vector control. Wolbachia is a maternally inherited, endosymbiotic bacterium found naturally in ~ 60% of the insect population (Hilgenboecker et al., 2008; Zug and Hammerstein, 2012). Due to the unique capability of Wolbachia to block many arthropod-borne viruses, it has been widely used for biological control of mosquito borne viruses including dengue, West Nile and Zika (Moreira et al., 2009; Walker et al., 2011; Aliota et al., 2016). Wolbachia is a safe vector control strategy as there is no evidence of impact on the environment, animals or humans by the bacterium (Centers for Disease Control and Prevention, 2022). The mechanisms through which pathogen blocking is occurring are yet unknown. Understanding these mechanisms is critical to preclude any resistance development in the mosquitoes against the bacterium. Interestingly, there is evidence to show that Wolbachia alters metabolic homeostasis of the mosquito vector during infection (Figure 2D) (Caragata et al., 2014; Molloy et al., 2016; Koh et al., 2020; Manokaran et al., 2020; Nascimento da Silva et al., 2022). Given the reliance on metabolism for viral replication in the mosquito (discussed above), several studies have hypothesized that metabolic competition versus commensalism may be a mechanism for Wolbachia-mediated pathogen blocking.
Manokaran et al., showed that Aag2 cells infected with the Wolbachia (wMel strain) had reduced abundances of acyl-carnitines which seemed detrimental for both DENV and ZIKV replication. Supplementation with acyl-carnitines restored DENV and ZIKV replication in Wolbachia infected cells (Manokaran et al., 2020). In the metabolomic study by Chotiwan, et al., acyl-carnitines were increased significantly in DENV2-infected mosquito midguts reinforcing the hypothesis that acyl-carnitines may play a proviral role in DENV replication and their regulation could be a possible mechanism of pathogen blocking by Wolbachia. Two reports exist for the intersection of SPs in insects and Wolbachia. Initially Rong et al. showed that Wolbachia infection induced the expression of miRNAs known to regulate genes with functions in sphingolipid metabolism (Rong et al., 2014). This was confirmed by Molloy et al, that recently showed a complete depletion of sphingolipids during Wolbachia infection of Ae. albopictus cells (Molloy et al., 2016).
Infection with wMel has also been shown to influence oviposition, expression of egg yolk precursor genes as well as altered excretion of the blood meal in Ae. aegypti mosquitoes (Pimenta de Oliveira et al., 2017). The results of this study indicated delayed expression of genes required for development of eggs which in turn might have delayed yolk deposition creating a lag in oviposition. To further support their results, they call attention to studies by Caragata et al., that demonstrates how cholesterol levels were reduced by 15-25% in Wolbachia (wMelPop strain) infected Ae. aegypti mosquitoes (Caragata et al., 2014). Cholesterol is considered a primary precursor of the 20-hydroxyecdysone hormone that is an important regulator of oogenesis (Raikhel et al., 2002).
Competition for host resources such as cholesterol is also a proposed mechanism of pathogen blocking by Wolbachia (Caragata et al., 2013). The study conducted in Wolbachia (wMelPop and wMelICS) infected Drosophila flies fed with a cholesterol enriched diet has shown weakened pathogen blocking capabilities in comparison to flies fed with a standard diet. Both insect and virus are unable to synthesize their own cholesterol and depend on cholesterol taken up in the diet. Further, many of the arboviruses require host cholesterol to enter host cells and replicate (Mackenzie et al., 2007; Lee et al., 2008; Puerta-Guardo et al., 2010; Soto-Acosta et al., 2013; García Cordero et al., 2014). Thus, it is not surprising that manipulation of host cholesterol by Wolbachia could lead to perturbed viral entry and replication (Caragata et al., 2013). These observations are further supported by quantitative proteomics studies on Aag2 cells and Ae. aegypti midguts that revealed differential expression of proteins related to vesicular trafficking, lipid metabolism and unfolded protein response in response to Wolbachia infection (Geoghegan et al., 2017). Elevated levels of esterified cholesterol were detected in Wolbachia infected cells possibly resulting from perturbed intracellular cholesterol trafficking (Geoghegan et al., 2017). Reversal of this accumulation by treating with cyclodextrins restored dengue replication in these cells, suggesting free cholesterol was required for viral replication and sequestration of cholesterol through esterification seemed to enhance pathogen blocking. However, in this same study, Neiman Pick Type C1 and C2 (NPC1 and 2) proteins, responsible for retro recycling of cholesterol were elevated in expression compared to controls. Several studies have shown that elevated levels of NPC1 and 2 are beneficial to DENV replication. Therefore, the exact nature of the block in cholesterol trafficking and its relationship to NPC1 activity and viral replication remains to be explored further.
7 Conclusions
Living organisms display substantial diversity in morphology, anatomy, behavior, and ecology. In concert with this diversity, they are also analogous to each other based on fundamental molecular traits mirrored in metabolism and biochemical mechanisms of inheritance. Attributing to the fact that metabolites are universal molecules that are the intermediates and products of genetic expression, studying metabolism to answer fundamental biological questions related to changes in physiological status in an organism in response to external stimuli or infection is becoming a promising avenue to explore. Among numerous pathogens imposing health burdens on global populations, arboviruses occupy a prominent position due to their causation of severe disease with a lack of successful intervention approaches. Many arboviruses such as dengue, Zika and chikungunya are vectored primarily by mosquitoes. Even though mosquito biology has been studied for many years, severe gaps remain in our understanding of the complex virus-vector interactions that continue to support disease transmission. Given the intimate connections between vector metabolism and its biology as well as the metabolic impact of pathogens and the microbiome on mosquito biology, the literature reviewed here suggest that exploiting metabolism-targeted intervention strategies will be transformative.
Author contributions
OR, NC, KS and RP wrote the manuscript. OR and RP assembled the figures and tables. All authors contributed to the article and approved the submitted version.
Funding
OR was supported by a R01AI151166 NIH/NIAID to RP. NC was funded by the Boettcher Foundation, WWBRA Early Career Investigator Award to RP. KS was funded by RO1AI121211 NIH/NIAID.
Acknowledgments
The authors acknowledge Paul S. Soma, Ph.D. and Hannah M. Laurence, DVM for critical reading of the manuscript.
Conflict of interest
The authors declare that the research was conducted in the absence of any commercial or financial relationships that could be construed as a potential conflict of interest.
Publisher’s note
All claims expressed in this article are solely those of the authors and do not necessarily represent those of their affiliated organizations, or those of the publisher, the editors and the reviewers. Any product that may be evaluated in this article, or claim that may be made by its manufacturer, is not guaranteed or endorsed by the publisher.
References
Abdul Rahim, N. A., Othman, M., Sabri, M., Stanley, D. W. (2018). “A midgut digestive phospholipase A2 in larval mosquitoes, aedes albopictus and culex quinquefasciatus.” enzyme research. Hindawi. Available at: https://www.hindawi.com/journals/er/2018/9703413/.
Acharya, U., Acharya, J. K. (2005). Enzymes of sphingolipid metabolism in drosophila melanogaster. Cell Mol. Life Sci. 62 (2), 128–142. doi: 10.1007/s00018-004-4254-1
Acharya, J. K., Dasgupta, U., Rawat, S. S., Yuan, C., Sanxaridis, P. D., Yonamine, I., et al. (2008). Cell-nonautonomous function of ceramidase in photoreceptor homeostasis. Neuron 57 (1), 69–79. doi: 10.1016/j.neuron.2007.10.041
Adolfi, A., Poulton, B., Anthousi, A., Macilwee, S., Ranson, H., Lycett, G. J. (2019). Functional genetic validation of key genes conferring insecticide resistance in the major African malaria vector, anopheles gambiae. Proc. Natl. Acad. Sci. 116 (51), 25764–25772. doi: 10.1073/pnas.1914633116
Alabaster, A., Isoe, J., Zhou, G., Lee, A., Murphy, A., Day, W. A., et al. (2011). Deficiencies in acetyl-CoA carboxylase and fatty acid synthase 1 differentially affect eggshell formation and blood meal digestion in aedes aegypti. Insect Biochem. Mol. Biol. 41 (12), 946–955. doi: 10.1016/j.ibmb.2011.09.004
Aliota, M. T., Peinado, S. A., Velez, I. D., Osorio, J. E. (2016). The wMel strain of wolbachia reduces transmission of zika virus by aedes aegypti. Sci. Rep. 6 (1), 28792. doi: 10.1038/srep28792
Apte-Deshpande, A., Paingankar, M., Gokhale, M. D., Deobagkar, D. N. (2012). Serratia odorifera a midgut inhabitant of aedes aegypti mosquito enhances its susceptibility to dengue-2 virus. PloS One 7 (7), e40401. doi: 10.1371/journal.pone.0040401
Apte-Deshpande, A. D., Paingankar, M. S., Gokhale, M. D., Deobagkar, D. N. (2014). Serratia odorifera mediated enhancement in susceptibility of aedes aegypti for chikungunya virus. Indian J. Med. Res. 139 (5), 762–768. doi: 10.1371/journal.pone.004040
Armstrong, P. M., Ehrlich, H. Y., Magalhaes, T., Miller, M. R., Conway, P. J., Bransfield, A., et al. (2020). Successive blood meals enhance virus dissemination within mosquitoes and increase transmission potential. Nat. Microbiol. 5 (2), 239–247. doi: 10.1038/s41564-019-0619-y
Arrese, E. L., Soulages, J. L. (2010). Insect fat body: Energy, metabolism, and regulation. Annu. Rev. Entomol. 55 (1), 207–225. doi: 10.1146/annurev-ento-112408-085356
Atella, G. C., Shahabuddin, M. (2002). Differential partitioning of maternal fatty acid and phospholipid in neonate mosquito larvae. J. Exp. Biol. 205 (Pt 23), 3623–3630. doi: 10.1242/jeb.205.23.3623
Attardo, G. M., Hansen, I. A., Raikhel, A. S. (2005). Nutritional regulation of vitellogenesis in mosquitoes: Implications for anautogeny. Insect Biochem. Mol. Biol. 35 (7), 661–675. doi: 10.1016/j.ibmb.2005.02.013
Bakermans, A. J., van Weeghel, M., Denis, S., Nicolay, K., Prompers, J. J., Houten, S. M. (2013). Carnitine supplementation attenuates myocardial lipid accumulation in long-chain acyl-CoA dehydrogenase knockout mice. J. Inherit Metab. Dis. 36 (6), 973–981. doi: 10.1007/s10545-013-9604-4
Barletta, A. B. F., Alves, L. R., Nascimento Silva, M. C. L., Sim, S., Dimopoulos, G., Liechocki, S., et al. (2016). Emerging role of lipid droplets in aedes aegypti immune response against bacteria and dengue virus. Sci. Rep. 6 (1), 19928. doi: 10.1038/srep19928
Bartz, R., Li, W.-H., Venables, B., Zehmer, J. K., Roth, M. R., Welti, R., et al. (2007). Lipidomics reveals that adiposomes store ether lipids and mediate phospholipid traffic. J. Lipid Res. 48 (4), 837–847. doi: 10.1194/jlr.M600413-JLR200
Bass, C., Jones, C. M. (2016). Mosquitoes boost body armor to resist insecticide attack. Proc. Natl. Acad. Sci. 113 (33), 9145–9147. doi: 10.1073/pnas.1610992113
Batz, Z. A., Armbruster, P. A. (2018). Diapause-associated changes in the lipid and metabolite profile of the Asian tiger mosquito, aedes albopictus. J. Exp. Biol 221 (24). doi: 10.1242/jeb.189480
Bauer, R. (2010). Towards understanding regulation of energy homeostasis by ceramide synthases. Results Probl Cell Differ. 52, 175–181. doi: 10.1007/978-3-642-14426-4_14
Beenakkers, A. M., van der Horst, D. J., Van Marrewijk, W. J. (1985). Insect lipids and lipoproteins, and their role in physiological processes. Prog. Lipid Res. 24 (1), 19–67. doi: 10.1016/0163-7827(85)90007-4
Bentley, M. D., Day, J. F. (1989). Chemical ecology and behavioral aspects of mosquito oviposition. Annu. Rev. Entomol. 34 (1), 401–421. doi: 10.1146/annurev.en.34.010189.002153
Bhatt, S., Gething, P. W., Brady, O. J., Messina, J. P., Farlow, A. W., Moyes, C. L., et al. (2013). The global distribution and burden of dengue. Nature 496 (7446), 504–507. doi: 10.1038/nature12060
Blomquist, G. J., Borgeson, C. E., Vundla, M. (1991). Polyunsaturated fatty acids and eicosanoids in insects. Insect Biochem. 21 (1), 99–106. doi: 10.1016/0020-1790(91)90069-Q
Boëte, C., Koella, J. C. (2003). Evolutionary ideas about genetically manipulated mosquitoes and malaria control. Trends Parasitol. 19 (1), 32–38. doi: 10.1016/S1471-4922(02)00003-X
Bosio, C. F., Fulton, R. E., Salasek, M. L., Beaty, B. J., Black, W. C. 4. (2000). Quantitative trait loci that control vector competence for dengue-2 virus in the mosquito aedes aegypti. Genetics 156 (2), 687–698. doi: 10.1093/genetics/156.2.687
Bottino-Rojas, V., Ferreira-Almeida, I., Nunes, R. D., Feng, X., Pham, T. B., Kelsey, A., et al. (2022). Beyond the eye: Kynurenine pathway impairment causes midgut homeostasis dysfunction and survival and reproductive costs in blood-feeding mosquitoes. Insect Biochem. Mol. Biol. 142, 103720. doi: 10.1016/j.ibmb.2022.103720
Brady, O. J., Gething, P. W., Bhatt, S., Messina, J. P., Brownstein, J. S., Hoen, A. G., et al. (2012). Refining the global spatial limits of dengue virus transmission by evidence-based consensus. PloS Negl. Trop. Dis. 6 (8), e1760. doi: 10.1371/journal.pntd.0001760
Brady, O. J., Golding, N., Pigott, D. M., Kraemer, M. U. G., Messina, J. P., Reiner, R. C., Jr., et al. (2014). Global temperature constraints on aedes aegypti and ae. albopictus persistence and competence for dengue virus transmission. Parasit Vectors 7 (1), 338. doi: 10.1186/1756-3305-7-338
Brasaemle, D. L. (2007). Thematic review series: Adipocyte biology. the perilipin family of structural lipid droplet proteins: Stabilization of lipid droplets and control of lipolysis. J. Lipid Res. 48 (12), 2547–2559. doi: 10.1194/jlr.R700014-JLR200
Briegel, H. (1990). Fecundity, metabolism, and body size in anopheles (Diptera: Culicidae), vectors of malaria. J. Med. Entomol. 27 (5), 839–850. doi: 10.1093/jmedent/27.5.839
Briegel, H., Hefti, M., DiMarco, E. (2002). Lipid metabolism during sequential gonotrophic cycles in large and small female aedes aegypti. J. Insect Physiol. 48 (5), 547–554. doi: 10.1016/S0022-1910(02)00072-0
Briegel, H., Timmermann, S. E. (2001). Aedes albopictus (Diptera: Culicidae): Physiological aspects of development and reproduction. J. Med. Entomol. 38 (4), 566–571. doi: 10.1603/0022-2585-38.4.566
Brito, L. P., Linss, J. G. B., Lima-Camara, T. N., Belinato, T. A., Peixoto, A. A., Lima, J. B. P., et al. (2013). Assessing the effects of aedes aegypti kdr mutations on pyrethroid resistance and its fitness cost. PloS One 8 (4), e60878. doi: 10.1371/journal.pone.0060878
Brown, D. A. (2001). Lipid droplets: Proteins floating on a pool of fat. Curr. Biol. 11 (11), R446–R449. doi: 10.1016/S0960-9822(01)00257-3
Burlandy, F. M., Ferreira, D. F., Rebello, M. A. (2004). Inhibition of vesicular stomatitis virus replication by prostaglandin A1 in aedes albopictus cells. Z Naturforsch. C 59 (1–2), 127–131. doi: 10.1515/znc-2004-1-224
Butters, T. D., Hughes, R. C. (1981). “Phospholipids and glycolipids in subcellular fractions of mosquito aedes aegypti cells.”. In Vitro 17 (9), 831–838.
Canavoso, L. E., Jouni, Z. E., Karnas, K. J., Pennington, J. E., Wells, M. A. (2001). FAT METABOLISM IN INSECTS. Annu. Rev. Nutr. 21 (1), 23–46. doi: 10.1146/annurev.nutr.21.1.23
Caragata, E. P., Rancès, E., Hedges, L. M., Gofton, A. W., Johnson, K. N., O’Neill, S. L., et al. (2013). Dietary cholesterol modulates pathogen blocking by wolbachia. PloS Pathog. 9 (6), e1003459. doi: 10.1371/journal.ppat.1003459
Caragata, E. P., Rancès, E., O’Neill, S. L., McGraw, E. A. (2014). Competition for amino acids between wolbachia and the mosquito host, aedes aegypti. Microb. Ecol. 67 (1), 205–218. doi: 10.1007/s00248-013-0339-4
Carnec, X., Meertens, L., Dejarnac, O., Perera-Lecoin, M., Hafirassou, M. L., Kitaura, J., et al. (2015). The phosphatidylserine and phosphatidylethanolamine receptor CD300a binds dengue virus and enhances infection. J. Virol. 90 (1), 92–102. doi: 10.1128/JVI.01849-15
Carro, A. C., Damonte, E. B. (2013). Requirement of cholesterol in the viral envelope for dengue virus infection. Virus Res. 174 (1–2), 78–87. doi: 10.1016/j.virusres.2013.03.005
Centers for Disease Control and Prevention (2022) Mosquitoes with wolbachia for reducing numbers of aedes aegypti mosquitoes. Available at: https://www.cdc.gov/mosquitoes/mosquito-control/community/emerging-methods/wolbachia.html.
Cermelli, S., Guo, Y., Gross, S. P., Welte, M. A. (2006). The lipid-droplet proteome reveals that droplets are a protein-storage depot. Curr. Biol. 16 (18), 1783–1795. doi: 10.1016/j.cub.2006.07.062
Chancey, C., Grinev, A., Volkova, E., Rios, M. (2015). The global ecology and epidemiology of west nile virus. BioMed. Res. Int. 2015. doi: 10.1155/2015/376230
Chapman, R. F. (2013). The insects structure and function, 5th edition. Eds. Simpson, S. J., Douglas, A. E. (Cambridge, England: Cambridge University Press), 962.
Chen, M., Du, Y., Nomura, Y., Zhorov, B. S., Dong, K. (2020). Chronology of sodium channel mutations associated with pyrethroid resistance in aedes aegypti. Arch. Insect Biochem. Physiol. 104 (2). doi: 10.1002/arch.21686
Chen, K., Lin, G., Haelterman, N. A., Ho, T. S.-Y., Li, T., Li, Z., et al. (2016). Loss of frataxin induces iron toxicity, sphingolipid synthesis, and Pdk1/Mef2 activation, leading to neurodegeneration. Elife 5. doi: 10.7554/eLife.16043.018
Chen, T.-Y., Smartt, C. T., Shin, D. (2021). Permethrin resistance in aedes aegypti affects aspects of vectorial capacity. Insects 12 (1), 71. doi: 10.3390/insects12010071
Chotiwan, N., Andre, B. G., Sanchez-Vargas, I., Islam, M. N., Grabowski, J. M., Hopf-Jannasch, A., et al. (2018). Dynamic remodeling of lipids coincides with dengue virus replication in the midgut of aedes aegypti mosquitoes. PloS Pathog. 14 (2), e1006853. doi: 10.1371/journal.ppat.1006853
Chotiwan, N., Brito-Sierra, C. A., Ramirez, G., Lian, E., Grabowski, J. M., Graham, B., et al. (2022). Expression of fatty acid synthase genes and their role in development and arboviral infection of aedes aegypti. Parasit Vectors 15 (1), 233. doi: 10.1186/s13071-022-05336-1
Christensen, B. M., LaFond, M. M., Christensen, L. A. (1986). Defense reactions of mosquitoes to filarial worms: Effect of host age on the immune response to dirofilaria immitis microfilariae. J. Parasitol. 72 (2), 212. doi: 10.2307/3281593
Chu, J., Xing, C., Du, Y., Duan, T., Liu, S., Zhang, P., et al. (2021). Pharmacological inhibition of fatty acid synthesis blocks SARS-CoV-2 replication. Nat. Metab. 3 (11), 1466–1475. doi: 10.1038/s42255-021-00479-4
Clayton, R. B. (1964). The utilization of sterols by insects. J. Lipid Res. 5, 3–19. doi: 10.1016/S0022-2275(20)40254-8
Clayton, R. B., Hinkle, P. C., Smith, D. A., Edwards, A. M. (1964). The intestinal absorption of cholesterol, its esters and some related sterols and analogues in the roac, eurycotis floridana. Comp. Biochem. Physiol. 11, 333–350. doi: 10.1016/0010-406X(64)90001-5
Clements, A. N. (1992). “Development, nutrition and reproduction,” in The biology of mosquitoes, vol. 1. (London: Chapman & Hall), 509.
Cook, P. E., McMeniman, C. J., O’Neill, S. L. (2008). Modifying insect population age structure to control vector-borne disease. Adv. Exp. Med. Biol. 627, 126–140. doi: 10.1007/978-0-387-78225-6_11
Coon, K. L., Brown, M. R., Strand, M. R. (2016). Gut bacteria differentially affect egg production in the anautogenous mosquito aedes aegypti and facultatively autogenous mosquito aedes atropalpus (Diptera: Culicidae). Parasit Vectors 9 (1), 375. doi: 10.1186/s13071-016-1660-9
Coon, K. L., Valzania, L., McKinney, D. A., Vogel, K. J., Brown, M. R., Strand, M. R. (2017). Bacteria-mediated hypoxia functions as a signal for mosquito development. Proc. Natl. Acad. Sci. 114 (27). doi: 10.1073/pnas.1702983114
Correa, M. A., Matusovsky, B., Brackney, D. E., Steven, B. (2018). Generation of axenic aedes aegypti demonstrate live bacteria are not required for mosquito development. Nat. Commun. 9 (1), 4464. doi: 10.1038/s41467-018-07014-2
Cui, L., Lee, Y. H., Thein, T. L., Fang, J., Pang, J., Ooi, E. E., et al. (2016). Serum metabolomics reveals serotonin as a predictor of severe dengue in the early phase of dengue fever. PloS Negl. Trop. Dis. 10 (4), e0004607. doi: 10.1371/journal.pntd.0004607
Dadd, R. H., Kleinjan, J. E. (1979). Essential fatty acid for the mosquito culex pipiens: Arachidonic acid. J. Insect Physiol. 25 (6), 495–502. doi: 10.1016/S0022-1910(79)80008-6
Dadd, R. H., Kleinjan, J. E., Stanley-Samuelson, D. W. (1987). Polyunsaturated fatty acids of mosquitos reared with single dietary polyunsaturates. Insect Biochem. 17, 7-16. doi: 10.1016/0020-1790(87)90137-5
da Encarnação Sá-Guimarães, T., Salles, T. S., Rocha dos Santos, C., Moreira, M. F., de Souza, W., Caldas, L. A. (2021). Route of zika virus infection in aedes aegypti by transmission electron microscopy. BMC Microbiol. 21 (1), 300. doi: 10.1186/s12866-021-02366-0
Das De, T., Sharma, P., Tevatiya, S., Chauhan, C., Kumari, S., Yadav, P., et al. (2022). Bidirectional microbiome-Gut-Brain-Axis communication influences metabolic switch-associated responses in the mosquito anopheles culicifacies. Cells 11 (11), 1798. doi: 10.3390/cells11111798
Dasgupta, U., Bamba, T., Chiantia, S., Karim, P., Tayoun, A. N. A., Yonamine, I., et al. (2009). Ceramide kinase regulates phospholipase c and phosphatidylinositol 4, 5, bisphosphate in phototransduction. Proc. Natl. Acad. Sci. U.S.A. 106 (47), 20063–20068. doi: 10.1073/pnas.0911028106
Davis, E. E. (1976). A receptor sensitive to oviposition site attractants on the antennae of the mosquito, aedes aegypti. J. Insect Physiol. 22 (10), 1371–1376. doi: 10.1016/0022-1910(76)90160-8
Davoodi, S., Galenza, A., Panteluk, A., Deshpande, R., Ferguson, M., Grewal, S., et al. (2019). The immune deficiency pathway regulates metabolic homeostasis in drosophila. J. Immunol. 202 (9), 2747–2759. doi: 10.4049/jimmunol.1801632
Deng, J., Guo, Y., Su, X., Liu, S., Yang, W., Wu, Y., et al. (2021). Impact of deltamethrin-resistance in aedes albopictus on its fitness cost and vector competence. PloS Negl. Trop. Dis. 15 (4), e0009391. doi: 10.1371/journal.pntd.0009391
Deretic, V., Levine, B. (2009). Autophagy, immunity, and microbial adaptations. Cell Host Microbe 5 (6), 527–549. doi: 10.1016/j.chom.2009.05.016
Dettloff, M., Wittwer, D., Weise, C., Wiesner, A. (2001). Lipophorin of lower density is formed during immune responses in the lepidopteran insect galleria mellonella. Cell Tissue Res. 306 (3), 449–458. doi: 10.1007/s00441-001-0468-9
Dong, D., Zhang, Y., Smykal, V., Ling, L., Raikhel, A. S. (2018). HR38, an ortholog of NR4A family nuclear receptors, mediates 20-hydroxyecdysone regulation of carbohydrate metabolism during mosquito reproduction. Insect Biochem. Mol. Biol. 96, 19–26. doi: 10.1016/j.ibmb.2018.02.003
Downer, R. G. H., Matthews, J. R. (1976). Patterns of lipid distribution and utilisation in insects. Am. Zool. 16 (4), 733–745. doi: 10.1093/icb/16.4.733
Edi, C. V., Djogbénou, L., Jenkins, A. M., Regna, K., Muskavitch, M. A. T., Poupardin, R., et al. (2014). CYP6 P450 enzymes and ACE-1 duplication produce extreme and multiple insecticide resistance in the malaria mosquito anopheles gambiae. PloS Genet. 10 (3), e1004236. doi: 10.1371/journal.pgen.1004236
Edman, J. D. (1970). Rate of digestion of vertebrate blood in aedes aegypti (L.). Am. J. Trop. Med. Hyg. 19 (6), 1031–1033. doi: 10.4269/ajtmh.1970.19.1031
Ehrlich, J. C. (2022) Mosquitoes. Available at: https://www.jcehrlich.com/mosquitoes/how-long-do-mosquitoes-live/.
Ekoka, E., Maharaj, S., Nardini, L., Dahan-Moss, Y., Koekemoer, L. L. (2021). 20-hydroxyecdysone (20E) signaling as a promising target for the chemical control of malaria vectors. Parasit Vectors 14 (1), 86. doi: 10.1186/s13071-020-04558-5
El-Bacha, T., Midlej, V., Pereira da Silva, A. P., Silva da Costa, L., Benchimol, M., Galina, A., et al. (2007). Mitochondrial and bioenergetic dysfunction in human hepatic cells infected with dengue 2 virus. Biochim. Biophys. Acta 1772 (10), 1158–1166. doi: 10.1016/j.bbadis.2007.08.003
Enayati, A. A., Ranson, H., Hemingway, J. (2005). Insect glutathione transferases and insecticide resistance. Insect Mol. Biol. 14 (1), 3–8. doi: 10.1111/j.1365-2583.2004.00529.x
European Center for Disease Prevention and Control (2015) Microcephaly in Brazil potentially linked to the zika virus epidemic, ECDC assesses the risk. Available at: https://www.ecdc.europa.eu/en/publications-data/rapid-risk-assessment-microcephaly-brazil-potentially-linked-zika-virus-epidemic.
European Center for Disease Prevention and Control (2022) Chikungunya worldwide overview. Available at: https://www.ecdc.europa.eu/en/chikungunya-monthly.
Farese, R. V., Walther, T. C. (2009). Lipid droplets finally get a little r-E-S-P-E-C-T. Cell 139 (5), 855–860. doi: 10.1016/j.cell.2009.11.005
Feng, Y., Peng, Y., Wen, H., Song, X., An, Y., Tang, H., et al. (2021). Microbial tryptophan catabolism affects the vector competence of Anopheles. bioRxiv. doi: 10.1101/2021.02.15.431262
Feyereisen, R. (2012). “Insect CYP genes and P450 enzymes,” in Insect molecular biology and biochemistry (Elsevier: Academic Press), 236–316.
Fontaine, K. A., Sanchez, E. L., Camarda, R., Lagunoff, M. (2015). Dengue virus induces and requires glycolysis for optimal replication. J. Virol. 89 (4), 2358–2366. doi: 10.1128/JVI.02309-14
Ford, P. S., Van Heusden, M. C. (1994). Triglyceride-rich lipophorin in aedes aegypti (Diptera: Culicidae). J. Med. Entomol. 31 (3), 435–441. doi: 10.1093/jmedent/31.3.435
Forman, H. J., Zhang, H., Rinna, A. (2009). Glutathione: Overview of its protective roles, measurement, and biosynthesis. Mol. Aspects Med. 30 (1–2), 1–12. doi: 10.1016/j.mam.2008.08.006
Franklinos, L. H. V., Jones, K. E., Redding, D. W., Abubakar, I. (2019). The effect of global change on mosquito-borne disease. Lancet Infect. Dis. 19 (9), e302–e312. doi: 10.1016/S1473-3099(19)30161-6
Fu, Q., Inankur, B., Yin, J., Striker, R., Lan, Q. (2015). Sterol carrier protein 2, a critical host factor for dengue virus infection, alters the cholesterol distribution in mosquito Aag2 cells. J. Med. Entomol. 52 (5), 1124–1134. doi: 10.1093/jme/tjv101
Gabrieli, P., Kakani, E. G., Mitchell, S. N., Mameli, E., Want, E. J., Mariezcurrena Anton, A., et al. (2014). Sexual transfer of the steroid hormone 20E induces the postmating switch in anopheles gambiae. Proc. Natl. Acad. Sci. 111 (46), 16353–16358. doi: 10.1073/pnas.1410488111
García Cordero, J., León Juárez, M., González-Y-Merchand, J. A., Cedillo Barrón, L., Gutiérrez Castañeda, B. (2014). Caveolin-1 in lipid rafts interacts with dengue virus NS3 during polyprotein processing and replication in HMEC-1 cells. PloS One 9 (3), e90704. doi: 10.1371/journal.pone.0090704
Geoghegan, V., Stainton, K., Rainey, S. M., Ant, T. H., Dowle, A. A., Larson, T., et al. (2017). Perturbed cholesterol and vesicular trafficking associated with dengue blocking in wolbachia-infected aedes aegypti cells. Nat. Commun. 8 (1), 526. doi: 10.1038/s41467-017-00610-8
Gillespie, L. K., Hoenen, A., Morgan, G., Mackenzie, J. M. (2010). The endoplasmic reticulum provides the membrane platform for biogenesis of the flavivirus replication complex. J. Virol. 84 (20), 10438–10447. doi: 10.1128/JVI.00986-10
Girard, Y. A., Popov, V., Wen, J., Han, V., Higgs, S. (2005). Ultrastructural study of West Nile virus pathogenesis in culex pipiens quinquefasciatus (Diptera: Culicidae). J. Med. Entomol. 42 (3), 429–444. doi: 10.1093/jmedent/42.3.429
Gray, L., Asay, B. C., Hephaestus, B., McCabe, R., Pugh, G., Markle, E. D., et al. (2022). Back to the future: Quantifying wing Wear as a method to measure mosquito age. Am. J. Trop. Med. Hyg. 107 (3), 689–700. doi: 10.4269/ajtmh.21-1173
Gray, E. M., Bradley, T. J. (2003). Metabolic rate in female culex tarsalis (Diptera: Culicidae) : Age, size, activity, and feeding effects. J. Med. Entomol. 40 (6), 903–911. doi: 10.1603/0022-2585-40.6.903
Guan, X. L., Cestra, G., Shui, G., Kuhrs, A., Schittenhelm, R. B., Hafen, E., et al. (2013). Biochemical membrane lipidomics during drosophila development. Dev. Cell. 24 (1), 98–111. doi: 10.1016/j.devcel.2012.11.012
Guo, Y., Cordes, K. R., Farese, R. V., Walther, T. C. (2009). Lipid droplets at a glance. J. Cell Sci. 122 (6), 749–752. doi: 10.1242/jcs.037630
Gwadz, R. W., Spielman, A. (1973). Corpus allatum control of ovarian development in aedes aegypti. J. Insect Physiol. 19 (7), 1441–1448. doi: 10.1016/0022-1910(73)90174-1
Hagedorn, H. H., Shapiro, J. P., Hanaoka, K. (1979). Ovarian ecdysone secretion is controlled by a brain hormone in an adult mosquito. Nature 282 (5734), 92–94. doi: 10.1038/282092a0
Hancock, P. A., Wiebe, A., Gleave, K. A., Bhatt, S., Cameron, E., Trett, A., et al. (2018). Associated patterns of insecticide resistance in field populations of malaria vectors across Africa. Proc. Natl. Acad. Sci. 115 (23), 5938–5943. doi: 10.1073/pnas.1801826115
Hannun, Y. A., Obeid, L. M. (2008). Principles of bioactive lipid signalling: lessons from sphingolipids. Nat. Rev. Mol. Cell Biol. 9 (2), 139–150. doi: 10.1038/nrm2329
Hazelton, G. A., Lang, C. A. (1983). Glutathione biosynthesis in the aging adult yellow-fever mosquito [ aedes aegypti (Louisville)]. Biochem. J. 210 (2), 289–295. doi: 10.1042/bj2100289
Heaton, N. S., Perera, R., Berger, K. L., Khadka, S., LaCount, D. J., Kuhn, R. J., et al. (2010). Dengue virus nonstructural protein 3 redistributes fatty acid synthase to sites of viral replication and increases cellular fatty acid synthesis. Proc. Natl. Acad. Sci. U.S.A. 107 (40), 17345–17350. doi: 10.1073/pnas.1010811107
Heaton, N. S., Randall, G. (2010). Dengue virus-induced autophagy regulates lipid metabolism. Cell Host Microbe 8 (5), 422–432. doi: 10.1016/j.chom.2010.10.006
Hilgenboecker, K., Hammerstein, P., Schlattmann, P., Telschow, A., Werren, J. H. (2008). How many species are infected with wolbachia? – a statistical analysis of current data. FEMS Microbiol. Lett. 281 (2), 215–220. doi: 10.1111/j.1574-6968.2008.01110.x
Hillyer, J. F., Schmidt, S. L., Fuchs, J. F., Boyle, J. P., Christensen, B. M. (2004). Age-associated mortality in immune challenged mosquitoes (Aedes aegypti) correlates with a decrease in haemocyte numbers. Cell Microbiol. 7 (1), 39–51. doi: 10.1111/j.1462-5822.2004.00430.x
Holthuis, J. C., Menon, A. K. (2014). Lipid landscapes and pipelines in membrane homeostasis. Nature 510, 48–57. doi: 10.1038/nature13474
Horvath, T. D., Dagan, S., Scaraffia, P. Y. (2021). Unraveling mosquito metabolism with mass spectrometry-based metabolomics. Trends Parasitol. 37 (8), 747–761. doi: 10.1016/j.pt.2021.03.010
Hou, Y., Wang, X.-L., Saha, T. T., Roy, S., Zhao, B., Raikhel, A. S., et al. (2015). Temporal coordination of carbohydrate metabolism during mosquito reproduction. PloS Genet. 11 (7), e1005309. doi: 10.1371/journal.pgen.1005309
Ibrahim, S. S., Riveron, J. M., Bibby, J., Irving, H., Yunta, C., Paine, M. J. I., et al. (2015). Allelic variation of cytochrome P450s drives resistance to bednet insecticides in a major malaria vector. PloS Genet. 11 (10), e1005618. doi: 10.1371/journal.pgen.1005618
Ikeshoji, T., Ichimoto, I., Konishi, J., Naoshima, Y., Ueda, H. (1979). 7, 11-dimethyloctadecane: An ovipositional attractant for aedes aegypti produced by pseudomonas aeruginosa on capric acid substrate. J. Pestic Sci. 4 (2), 187–194. doi: 10.1584/jpestics.4.187
Ikeshoji, T., Saito, K., Yano, A. (1975). Bacterial production of the ovipositional attactants for mosquitoes on fatty acid substrates. Appl. Entomol Zool. 10 (3), 239–242. doi: 10.1303/aez.10.239
Iovinella, I., Caputo, B., Michelucci, E., Dani, F. R., della Torre, A. (2015). Candidate biomarkers for mosquito age-grading identified by label-free quantitative analysis of protein expression in aedes albopictus females. J. Proteomics 128, 272–279. doi: 10.1016/j.jprot.2015.08.002
Ishak, R., Tovey, D. G., Howard, C. R. (1988). Morphogenesis of yellow fever virus 17D in infected cell cultures. J. Gen. Virol. 69 (Pt 2), 325–335. doi: 10.1099/0022-1317-69-2-325
Jenkin, H. M., McMeans, E., Anderson, L. E., Yang, T. K. (1975). Comparison of phospholipid composition of aedes aegypti and aedes albopictus cells obtained from logarithmic and stationary phases of growth. Lipids 10 (11), 686–694. doi: 10.1007/BF02532762
Jenkins, S. P., Brown, M. R., Lea, A. O. (1992). Inactive prothoracic glands in larvae and pupae of aedes aegypti: Ecdysteroid release by tissues in the thorax and abdomen. Insect Biochem. Mol. Biol. 22 (6), 553–559. doi: 10.1016/0965-1748(92)90032-A
Johnson, B. J., Hugo, L. E., Churcher, T. S., Ong, O. T. W., Devine, G. J. (2020). Mosquito age grading and vector-control programmes. Trends Parasitol. 36 (1), 39–51. doi: 10.1016/j.pt.2019.10.011
Junjhon, J., Pennington, J. G., Edwards, T. J., Perera, R., Lanman, J., Kuhn, R. J. (2014). Ultrastructural characterization and three-dimensional architecture of replication sites in dengue virus-infected mosquito cells. J. Virol. 88 (9), 4687–4697. doi: 10.1128/JVI.00118-14
Jupatanakul, N., Sim, S., Dimopoulos, G. (2014). Aedes aegypti ML and niemann-pick type c family members are agonists of dengue virus infection. Dev. Comp. Immunol. 43 (1), 1–9. doi: 10.1016/j.dci.2013.10.002
Kasai, S., Komagata, O., Itokawa, K., Shono, T., Ng, L. C., Kobayashi, M., et al. (2014). Mechanisms of pyrethroid resistance in the dengue mosquito vector, aedes aegypti: Target site insensitivity, penetration, and metabolism. PloS Negl. Trop. Dis. 8 (6), e2948. doi: 10.1371/journal.pntd.0002948
Kaufmann, C., Brown, M. R. (2008). Regulation of carbohydrate metabolism and flight performance by a hypertrehalosaemic hormone in the mosquito anopheles gambiae. J. Insect Physiol. 54 (2), 367–377. doi: 10.1016/j.jinsphys.2007.10.007
Khormi, H. M., Kumar, L. (2014). Climate change and the potential global distribution of aedes aegypti: Spatial modelling using GIS and CLIMEX. Geospat Health 8 (2), 405–415. doi: 10.4081/gh.2014.29
King, J. G., Hillyer, J. F. (2013). Spatial and temporal in vivo analysis of circulating and sessile immune cells in mosquitoes: Hemocyte mitosis following infection. BMC Biol. 11 (1), 55. doi: 10.1186/1741-7007-11-55
Kler, R. S., Jackson, S., Bartlett, K., Bindoff, L. A., Eaton, S., Pourfarzam, M., et al. (1991). Quantitation of acyl-CoA and acylcarnitine esters accumulated during abnormal mitochondrial fatty acid oxidation. J. Biol. Chem. 266 (34), 22932–22938. doi: 10.1016/S0021-9258(18)54444-6
Knabb, M. T., Saffitz, J. E., Corr, P. B., Sobel, B. E. (1986). The dependence of electrophysiological derangements on accumulation of endogenous long-chain acyl carnitine in hypoxic neonatal rat myocytes. Circ. Res. 58 (2), 230–240. doi: 10.1161/01.RES.58.2.230
Koh, C., Islam, M. N., Ye, Y. H., Chotiwan, N., Graham, B., Belisle, J. T., et al. (2020). Dengue virus dominates lipid metabolism modulations in wolbachia-coinfected aedes aegypti. Commun. Biol. 3 (1), 1–14. doi: 10.1038/s42003-020-01254-z
Kohyama-Koganeya, A., Nabetani, T., Miura, M., Hirabayashi, Y. (2011). Glucosylceramide synthase in the fat body controls energy metabolism in drosophila. J. Lipid Res. 52 (7), 1392–1399. doi: 10.1194/jlr.M014266
Koves, T. R., Ussher, J. R., Noland, R. C., Slentz, D., Mosedale, M., Ilkayeva, O., et al. (2008). Mitochondrial overload and incomplete fatty acid oxidation contribute to skeletal muscle insulin resistance. Cell Metab. 7 (1), 45–56. doi: 10.1016/j.cmet.2007.10.013
Koyama, T., Texada, M. J., Halberg, K. A., Rewitz, K. (2020). Metabolism and growth adaptation to environmental conditions in drosophila. Cell Mol. Life Sci. 77 (22), 4523–4551. doi: 10.1007/s00018-020-03547-2
Kraemer, M. U. G., Sinka, M. E., Duda, K. A., Mylne, A. Q. N., Shearer, F. M., Barker, C. M., et al. (2015). The global distribution of the arbovirus vectors aedes aegypti and ae. albopictus Elife 4, e08347. doi: 10.7554/eLife.08347.014
Kraut, R. (2011). Roles of sphingolipids in drosophila development and disease. J. Neurochem. 116 (5), 764–778. doi: 10.1111/j.1471-4159.2010.07022.x
Krebs, K. C., Lan, Q. (2003). Isolation and expression of a sterol carrier protein-2 gene from the yellow fever mosquito, aedes aegypti. Insect Mol. Biol. 12 (1), 51–60. doi: 10.1046/j.1365-2583.2003.00386.x
Kuerschner, L., Moessinger, C., Thiele, C. (2008). Imaging of lipid biosynthesis: How a neutral lipid enters lipid droplets. Traffic 9 (3), 338–352. doi: 10.1111/j.1600-0854.2007.00689.x
Lee, C.-J., Lin, H.-R., Liao, C.-L., Lin, Y.-L. (2008). Cholesterol effectively blocks entry of flavivirus. J. Virol. 82 (13), 6470–6480. doi: 10.1128/JVI.00117-08
Li, Y. Y., Li, Y. Y., Wang, G., Li, J., Zhang, M., Wu, J., et al. (2022). Differential metabolome responses to deltamethrin between resistant and susceptible anopheles sinensis. Ecotoxicol Environ. Saf. 237, 113553. doi: 10.1016/j.ecoenv.2022.113553
Lima, V. L. A., Dias, F., Nunes, R. D., Pereira, L. O., Santos, T. S. R., Chiarini, L. B., et al. (2012). The antioxidant role of xanthurenic acid in the aedes aegypti midgut during digestion of a blood meal. PloS One 7 (6), e38349. doi: 10.1371/journal.pone.0038349
Lin, Y-CY-H, Chen, Y.-C., Kao, T.-Y., Lin, Y-CY-H, Hsu, T.-E., Wu, Y.-C., et al. (2014). Diacylglycerol lipase regulates lifespan and oxidative stress response by inversely modulating TOR signaling in drosophila and c. elegans. Aging Cell. 13 (4), 755–764. doi: 10.1111/acel.12232
Lin, C-YC-K, Tseng, C.-K., Wu, Y.-H., Liaw, C.-C., Lin, C-YC-K, Huang, C.-H., et al. (2017). Cyclooxygenase-2 facilitates dengue virus replication and serves as a potential target for developing antiviral agents. Sci. Rep. 7 (1), 44701. doi: 10.1038/srep44701
Ling, L., Raikhel, A. S. (2021). Cross-talk of insulin-like peptides, juvenile hormone, and 20-hydroxyecdysone in regulation of metabolism in the mosquito aedes aegypti. Proc. Natl. Acad. Sci. U.S.A. 118 (6). doi: 10.1073/pnas.2023470118
Liu, B. (2012). Therapeutic potential of cyclodextrins in the treatment of niemann–pick type c disease. Clin. Lipidol. 7 (3), 289–301. doi: 10.2217/clp.12.31
Liu, Y.-Y., Liang, X.-D., Liu, C.-C., Cheng, Y., Chen, H., Baloch, A. S., et al. (2021). Fatty acid synthase is involved in classical swine fever virus replication by interaction with NS4B. J. Virol. 95 (17). doi: 10.1128/JVI.00781-21
Mackenzie, J. M., Khromykh, A. A., Parton, R. G. (2007). Cholesterol manipulation by West Nile virus perturbs the cellular immune response. Cell Host Microbe 2 (4), 229–239. doi: 10.1016/j.chom.2007.09.003
Maier, T., Leibundgut, M., Ban, N. (2008). The crystal structure of a mammalian fatty acid synthase. Sci. (80- ). 321 (5894), 1315–1322. doi: 10.1126/science.1161269
Manokaran, G., Flores, H. A., Dickson, C. T., Narayana, V. K., Kanojia, K., Dayalan, S., et al. (2020). Modulation of acyl-carnitines, the broad mechanism behind wolbachia-mediated inhibition of medically important flaviviruses in aedes aegypti. Proc. Natl. Acad. Sci. U.S.A. 117 (39), 24475–24483. doi: 10.1073/pnas.1914814117
Martín-Acebes, M. A., Blázquez, A.-B., Jiménez de Oya, N., Escribano-Romero, E., Saiz, J.-C. (2011). West Nile Virus replication requires fatty acid synthesis but is independent on phosphatidylinositol-4-Phosphate lipids. PloS One 6 (9), e24970. doi: 10.1371/journal.pone.0024970
Martínez, B. A., Yeudall, S., Hoyle, R. G., Castle, J. D., Leitinger, N., Bland, M. L. (2020). Innate immune signaling in drosophila shifts anabolic lipid metabolism from triglyceride storage to phospholipid synthesis in an ER stress-dependent manner. bioRxiv 16 (11), e1009192. doi: 10.1371/journal.pgen.1009192
Martin-Park, A., Gomez-Govea, M. A., Lopez-Monroy, B., Treviño-Alvarado, V. M., Torres-Sepúlveda M del, R., López-Uriarte, G. A., et al. (2017). Profiles of amino acids and acylcarnitines related with insecticide exposure in culex quinquefasciatus (Say). PloS One 12 (1), e0169514. doi: 10.1371/journal.pone.0169514
Mazeaud, C., Anton, A., Pahmeier, F., Sow, A. A., Cerikan, B., Freppel, W., et al. (2021). The biogenesis of dengue virus replication organelles requires the ATPase activity of valosin-containing protein. Viruses 13 (10), 2092. doi: 10.3390/v13102092
McMeans, E., Yang, T. K., Anderson, L. E., Jenkin, H. M. (1975). Comparison of lipid composition of aedes aegypti and aedes albopictus cells obtained from logarithmic and stationary phases of growth. Lipids 10, 99–104. doi: 10.1007/BF02532163
Melo, C. F. O. R., De Oliveira, D. N., De Oliveira Lima, E., Guerreiro, T. M., Esteves, C. Z., Beck, R. M., et al. (2016). A lipidomics approach in the characterization of zika-infected mosquito cells: Potential targets for breaking the transmission cycle. PloS One 11 (10). doi: 10.1371/journal.pone.0164377
Mérida, I., Ávila-Flores, A., Merino, E. (2008). Diacylglycerol kinases: At the hub of cell signalling. Biochem. J. 409 (1), 1–18. doi: 10.1042/BJ20071040
Miller, S., Kastner, S., Krijnse-Locker, J., Bühler, S., Bartenschlager, R. (2007). The non-structural protein 4A of dengue virus is an integral membrane protein inducing membrane alterations in a 2K-regulated manner. J. Biol. Chem. 282 (12), 8873–8882. doi: 10.1074/jbc.M609919200
Mitchell, S. N., Stevenson, B. J., Müller, P., Wilding, C. S., Egyir-Yawson, A., Field, S. G., et al. (2012). Identification and validation of a gene causing cross-resistance between insecticide classes in anopheles gambiae from Ghana. Proc. Natl. Acad. Sci. 109 (16), 6147–6152. doi: 10.1073/pnas.1203452109
Moller-Tank, S., et al. (2014). “Characterizing functional domains for TIM-mediated enveloped virus entry.” J. Virol. 88 (12), 6702–6713. doi: 10.1128/JVI.00300-14
Molloy, J. C., Sommer, U., Viant, M. R., Sinkins, S. P. (2016). Wolbachia modulates lipid metabolism in aedes albopictus mosquito cells. Appl. Environ. Microbiol. 82 (10), 3109–3120. doi: 10.1128/AEM.00275-16
Moreira, L. A., Iturbe-Ormaetxe, I., Jeffery, J. A., Lu, G., Pyke, A. T., Hedges, L. M., et al. (2009). A wolbachia symbiont in aedes aegypti limits infection with dengue, chikungunya, and plasmodium. Cell 139 (7), 1268–1278. doi: 10.1016/j.cell.2009.11.042
Moyes, C. L., Vontas, J., Martins, A. J., Ng, L. C., Koou, S. Y., Dusfour, I., et al. (2017). Contemporary status of insecticide resistance in the major aedes vectors of arboviruses infecting humans. PloS Negl. Trop. Dis. 11 (7), e0005625. doi: 10.1371/journal.pntd.0005625
Müller, P., Warr, E., Stevenson, B. J., Pignatelli, P. M., Morgan, J. C., Steven, A., et al. (2008). Field-caught permethrin-resistant anopheles gambiae overexpress CYP6P3, a P450 that metabolises pyrethroids. PloS Genet. 4 (11), e1000286. doi: 10.1371/journal.pgen.1000286
Muturi, E. J., Dunlap, C., Smartt, C. T., Shin, D. (2021). Resistance to permethrin alters the gut microbiota of aedes aegypti. Sci. Rep. 11 (1), 14406. doi: 10.1038/s41598-021-93725-4
Muturi, E. J., Kim, C.-H., Alto, B. W., Berenbaum, M. R., Schuler, M. A. (2011). Larval environmental stress alters aedes aegypti competence for sindbis virus. Trop. Med. Int. Heal. 16 (8), 955–964. doi: 10.1111/j.1365-3156.2011.02796.x
Mweya, C. N., Kimera, S. I., Stanley, G., Misinzo, G., Mboera, L. E. G. G. (2016). Climate change influences potential distribution of infected aedes aegypti Co-occurrence with dengue epidemics risk areas in Tanzania. PloS One 11 (9), e0162649. doi: 10.1371/journal.pone.0162649
Nasci, R. S., Mitchell, C. J. (1994). Larval diet, adult size, and susceptibility of aedes aegypti (Diptera: Culicidae) to infection with Ross river virus. J. Med. Entomol. 31 (1), 123–126. doi: 10.1093/jmedent/31.1.123
Nascimento da Silva, J., Calixto Conceição, C., Cristina Ramos de Brito, G., Costa Santos, D., Martins da Silva, R., Arcanjo, A., et al. (2022). Wolbachia pipientis modulates metabolism and immunity during aedes fluviatilis oogenesis. Insect Biochem. Mol. Biol. 146, 103776. doi: 10.1016/j.ibmb.2022.103776
Nasheri, N., Joyce, M., Rouleau, Y., Yang, P., Yao, S., Tyrrell, D. L., et al. (2013). Modulation of fatty acid synthase enzyme activity and expression during hepatitis c virus replication. Chem. Biol. 20 (4), 570–582. doi: 10.1016/j.chembiol.2013.03.014
Nayar, J. K., Van Handel, E. (1971). The fuel for sustained mosquito flight. J. Insect Physiol. 17 (3), 471–481. doi: 10.1016/0022-1910(71)90026-6
Nene, V., Wortman, J. R., Lawson, D., Haas, B., Kodira, C., Tu, Z. J., et al. (2007). Genome sequence of aedes aegypti, a major arbovirus vector. Science 316 (5832), 1718–1723. doi: 10.1126/science.1138878
Offerdahl, D. K., Dorward, D. W., Hansen, B. T., Bloom, M. E. (2012). A three-dimensional comparison of tick-borne flavivirus infection in mammalian and tick cell lines. PloS One 7 (10), e47912. doi: 10.1371/journal.pone.0047912
Okech, B., Arai, M., Matsuoka, H. (2006). The effects of blood feeding and exogenous supply of tryptophan on the quantities of xanthurenic acid in the salivary glands of anopheles stephensi (Diptera: Culicidae). Biochem. Biophys. Res. Commun. 341 (4), 1113–1118. doi: 10.1016/j.bbrc.2006.01.079
Olofsson, S.-O., Boström, P., Andersson, L., Rutberg, M., Perman, J., Borén, J. (2009). Lipid droplets as dynamic organelles connecting storage and efflux of lipids. Biochim. Biophys. Acta - Mol. Cell Biol. Lipids. 1791 (6), 448–458. doi: 10.1016/j.bbalip.2008.08.001
Olzmann, J. A., Carvalho, P. (2019). Dynamics and functions of lipid droplets. Nat. Rev. Mol. Cell Biol. 20 (3), 137–155. doi: 10.1038/s41580-018-0085-z
Oppenoorth, F. J. (1984). Biochemistry of insecticide resistance. Pestic Biochem. Physiol. 22 (2), 187–193. doi: 10.1016/0048-3575(84)90088-9
Parker-Crockett, C., Connelly, C. R., Siegfried, B., Alto, B. (2021). Influence of pyrethroid resistance on vector competency for zika virus by aedes aegypti (Diptera: Culicidae). J. Med. Entomol. 58 (4), 1908–1916. doi: 10.1093/jme/tjab035
Pavlidi, N., Monastirioti, M., Daborn, P., Livadaras, I., Van Leeuwen, T., Vontas, J. (2012). Transgenic expression of the aedes aegypti CYP9J28 confers pyrethroid resistance in drosophila melanogaster. Pestic Biochem. Physiol. 104 (2), 132–135. doi: 10.1016/j.pestbp.2012.07.003
Pennington, J. E., Nussenzveig, R. H., Van Heusden, M. C. (1996). Lipid transfer from insect fat body to lipophorin: comparison between a mosquito triacylglycerol-rich lipophorin and a sphinx moth diacylglycerol-rich lipophorin. J. Lipid Res. 37 (5), 1144–1152. doi: 10.1016/S0022-2275(20)42023-1
Pennington, J. E., Wells, M. A. (2002). Triacylglycerol-rich lipophorins are found in the dipteran infraorder culicomorpha, not just in mosquitoes. J. Insect Sci. 2 (15), 1–5. doi: 10.1093/jis/2.1.15
Perera, R., Kuhn, R. J. (2008). Structural proteomics of dengue virus. Curr. Opin. Microbiol. 11 (4), 369–377. doi: 10.1016/j.mib.2008.06.004
Perera, R., Riley, C., Isaac, G., Hopf-Jannasch, A. S., Moore, R. J., Weitz, K. W., et al. (2012). Dengue virus infection perturbs lipid homeostasis in infected mosquito cells. PloS Pathog. 8 (3), e1002584. doi: 10.1371/journal.ppat.1002584
Perry, A. S., Fay, R. W. (1967). Correlation of chemical constitution and physical properties of fatty acid esters with oviposition response of aedes aegypti. Mosquito News journal 27, 2, 175–183.
Petzel, D. H., Stanley-Samuelson, D. W. (1992). Inhibition of eicosanoid biosynthesis modulates basal fluid secretion in the malpighian tubules of the yellow fever mosquito (Aedes aegypti). J. Insect Physiol. 38 (1), 1–8. doi: 10.1016/0022-1910(92)90016-7
Pimenta de Oliveira, S., Dantas de Oliveira, C., Viana Sant’Anna, M. R., Carneiro Dutra, H. L., Caragata, E. P., Moreira, L. A. (2017). Wolbachia infection in aedes aegypti mosquitoes alters blood meal excretion and delays oviposition without affecting trypsin activity. Insect Biochem. Mol. Biol. 87, 65–74. doi: 10.1016/j.ibmb.2017.06.010
Pinch, M., Mitra, S., Rodriguez, S. D., Li, Y., Kandel, Y., Dungan, B., et al. (2021). Fat and happy: Profiling mosquito fat body lipid storage and composition post-blood meal. Front. Insect Sci. 1. doi: 10.3389/finsc.2021.693168
Prud’homme, S. M., Renault, D., David, J.-P., Reynaud, S. (2018). Multiscale approach to deciphering the molecular mechanisms involved in the direct and intergenerational effect of ibuprofen on mosquito aedes aegypti. Environ. Sci. Technol. 52 (14), 7937–7950. doi: 10.1021/acs.est.8b00988
Puerta-Guardo, H., Mosso, C., Medina, F., Liprandi, F., Ludert, J. E., del Angel, R. M. (2010). Antibody-dependent enhancement of dengue virus infection in U937 cells requires cholesterol-rich membrane microdomains. J. Gen. Virol. 91 (2), 394–403. doi: 10.1099/vir.0.015420-0
Raikhel, A. S., Kokoza, V. A., Zhu, J., Martin, D., Wang, S.-F., Li, C., et al. (2002). Molecular biology of mosquito vitellogenesis: from basic studies to genetic engineering of antipathogen immunity. Insect Biochem. Mol. Biol. 32 (10), 1275–1286. doi: 10.1016/S0965-1748(02)00090-5
Ramirez, J. L., de Almeida Oliveira, G., Calvo, E., Dalli, J., Colas, R. A., Serhan, C. N., et al. (2015). A mosquito lipoxin/lipocalin complex mediates innate immune priming in anopheles gambiae. Nat. Commun. 6 (1), 7403. doi: 10.1038/ncomms8403
Ramirez, J. L., Short, S. M., Bahia, A. C., Saraiva, R. G., Dong, Y., Kang, S., et al. (2014). Chromobacterium Csp_P reduces malaria and dengue infection in vector mosquitoes and has entomopathogenic and In vitro anti-pathogen activities. PloS Pathog. 10 (10), e1004398. doi: 10.1371/journal.ppat.1004398
Ramirez, J. L., Souza-Neto, J., Torres Cosme, R., Rovira, J., Ortiz, A., Pascale, J. M., et al. (2012). Reciprocal tripartite interactions between the aedes aegypti midgut microbiota, innate immune system and dengue virus influences vector competence. PloS Negl. Trop. Dis. 6 (3), e1561. doi: 10.1371/journal.pntd.0001561
Reid, W. R., Thornton, A., Pridgeon, J. W., Becnel, J. J., Tang, F., Estep, A., et al. (2014). Transcriptional analysis of four family 4 P450s in a Puerto Rico strain of aedes aegypti (Diptera: Culicidae) compared with an Orlando strain and their possible functional roles in permethrin resistance. J. Med. Entomol. 51 (3), 605–615. doi: 10.1603/ME13228
Renkonen, O., Luukkonen, A., Brummer-Korvenkontio, M. (2002). Lipids of cultured mosquito cells (Aedes albopictus): Comparison with cultured mammalian fibroblasts (BHK 21 cells). Biochim. Biophys. Acta (BBA) - Lipids Lipid Metab.
Reyes-Ruiz, J. M., Osuna-Ramos, J. F., De Jesús-González, L. A., Hurtado-Monzón, A. M., Farfan-Morales, C. N., Cervantes-Salazar, M., et al. (2019). Isolation and characterization of exosomes released from mosquito cells infected with dengue virus. Virus Res. 266, 1–14. doi: 10.1016/j.virusres.2019.03.015
Richard, A. S., et al. (2015). “Virion-associated phosphatidylethanolamine promotes TIM1-mediated infection by Ebola, dengue, and West Nile viruses. “Proc. Natl. Acad. Sci. U. S. A. 112, 14682–14687. doi: 10.1073/pnas.1508095112
Richards, S. L., White, A. V., Balanay, J. A. G. (2017). Potential for sublethal insecticide exposure to impact vector competence of aedes albopictus (Diptera: Culicidae) for dengue and zika viruses. Res. Rep. Trop. Med. 8, 53–57. doi: 10.2147/RRTM.S133411
Rivera-Pérez, C., Clifton, M. E., Noriega, F. G. (2017). How micronutrients influence the physiology of mosquitoes. Curr. Opin. Insect Sci. 23, 112–117. doi: 10.1016/j.cois.2017.07.002
Riveron, J. M., Ibrahim, S. S., Chanda, E., Mzilahowa, T., Cuamba, N., Irving, H., et al. (2014). The highly polymorphic CYP6M7 cytochrome P450 gene partners with the directionally selected CYP6P9a and CYP6P9b genes to expand the pyrethroid resistance front in the malaria vector anopheles funestus in Africa. BMC Genomics 15 (1), 817. doi: 10.1186/1471-2164-15-817
Robert Burger, J., Hou C, A. S., Hall, C., Brown, J. H. (2021). Universal rules of life: Metabolic rates, biological times and the equal fitness paradigm. Ecol. Lett. 24 (6), 1262–1281. doi: 10.1111/ele.13715
Romanelli, D., Casartelli, M., Cappellozza, S., de Eguileor, M., Tettamanti, G. (2016). Roles and regulation of autophagy and apoptosis in the remodelling of the lepidopteran midgut epithelium during metamorphosis. Sci. Rep. 6 (1), 32939. doi: 10.1038/srep32939
Rong, X., Zhang, Y.-K., Zhang, K.-J., Hong, X.-Y. (2014). Identification of wolbachia-responsive microRNAs in the two-spotted spider mite, tetranychus urticae. BMC Genomics 15 (1), 1122. doi: 10.1186/1471-2164-15-1122
Roosendaal, J., Westaway, E. G., Khromykh, A., Mackenzie, J. M. (2006). Regulated cleavages at the West Nile virus NS4A-2K-NS4B junctions play a major role in rearranging cytoplasmic membranes and golgi trafficking of the NS4A protein. J. Virol. 80 (9), 4623–4632. doi: 10.1128/JVI.80.9.4623-4632.2006
Rothwell, C., Lebreton, A., Young Ng, C., Lim, J. Y. H., Liu, W., Vasudevan, S., et al. (2009). Cholesterol biosynthesis modulation regulates dengue viral replication. Virology 389 (1–2), 8–19. doi: 10.1016/j.virol.2009.03.025
Roy, S., Saha, T. T., Johnson, L., Zhao, B., Ha, J., White, K. P., et al. (2015). Regulation of gene expression patterns in mosquito reproduction. PloS Genet. 11 (8), e1005450. doi: 10.1371/journal.pgen.1005450
Rückert, C., Ebel, G. D. (2018). How do virus–mosquito interactions lead to viral emergence? Trends Parasitol. 34 (4). doi: 10.1016/j.pt.2017.12.004
Russo, R., Haange, S.-B., Rolle-Kampczyk, U., von Bergen, M., Becker, J. M., Liess, M. (2018). Identification of pesticide exposure-induced metabolic changes in mosquito larvae. Sci. Total Environ. 643, 1533–1541. doi: 10.1016/j.scitotenv.2018.06.282
Salazar, M. I., Richardson, J. H., Sánchez-Vargas, I., Olson, K. E., Beaty, B. J. (2007). Dengue virus type 2: replication and tropisms in orally infected aedes aegypti mosquitoes. BMC Microbiol. 7 (1), 9. doi: 10.1186/1471-2180-7-9
Samsa, M. M., Mondotte, J. A., Iglesias, N. G., Assunção-Miranda, I., Barbosa-Lima, G., Da Poian, A. T., et al. (2009). Dengue virus capsid protein usurps lipid droplets for viral particle formation. PloS Pathog. 5 (10). doi: 10.1371/journal.ppat.1000632
Sanders, H. R., Evans, A. M., Ross, L. S., Gill, S. S. (2003). Blood meal induces global changes in midgut gene expression in the disease vector, aedes aegypti. Insect Biochem. Mol. Biol. 33 (11), 1105–1122. doi: 10.1016/S0965-1748(03)00124-3
Sarri, E., Sicart, A., Lázaro-Diéguez, F., Egea, G. (2011). Phospholipid synthesis participates in the regulation of diacylglycerol required for membrane trafficking at the golgi complex. J. Biol. Chem. 286 (32), 28632–28643. doi: 10.1074/jbc.M111.267534
Sawadogo, S. P., Diabaté, A., Toé, H. K., Sanon, A., Lefevre, T., Baldet, T., et al. (2013). Effects of age and size on anopheles gambiae s.s. Male mosquito mating success. J. Med. Entomol. 50 (2), 285–293. doi: 10.1603/me12041
Scott, J. G. (1990). Investigating mechanisms of insecticide resistance: Methods, strategies, and pitfalls BT - pesticide resistance in arthropods. Eds. Roush, R. T., Tabashnik, B. E. (Boston, MA: Springer US), 39–57.
Scott, T. W., Takken, W. (2012). Feeding strategies of anthropophilic mosquitoes result in increased risk of pathogen transmission. Trends Parasitol. 28 (3), 114–121. doi: 10.1016/j.pt.2012.01.001
Short, S. M., Mongodin, E. F., MacLeod, H. J., Talyuli, O. A. C., Dimopoulos, G. (2017). Amino acid metabolic signaling influences aedes aegypti midgut microbiome variability. PloS Negl. Trop. Dis. 11 (7), e0005677. doi: 10.1371/journal.pntd.0005677
Silva, E. R. M. N., Santos, L. V., Caiado, M. S., Hastenreiter, L. S. N., Fonseca, S. R. R., Carbajal-de-la-Fuente, A. L., et al. (2021). The influence of larval density on triacylglycerol content in aedes aegypti (Linnaeus) (Diptera: Culicidae). Arch. Insect Biochem. Physiol. 106 (2). doi: 10.1002/arch.21757
Singh, P., Kumar, P., Pande, V., Kumar, V., Dhiman, R. C. (2022). Untargeted metabolomics-based response analysis of temperature and insecticide exposure in aedes aegypti. Sci. Rep. 12 (1), 2066. doi: 10.1038/s41598-022-05630-z
Smith, L. B., Kasai, S., Scott, J. G. (2016). Pyrethroid resistance in aedes aegypti and aedes albopictus: Important mosquito vectors of human diseases. Pestic Biochem. Physiol. 133, 1–12. doi: 10.1016/j.pestbp.2016.03.005
Soto-Acosta, R., Mosso, C., Cervantes-Salazar, M., Puerta-Guardo, H., Medina, F., Favari, L., et al. (2013). The increase in cholesterol levels at early stages after dengue virus infection correlates with an augment in LDL particle uptake and HMG-CoA reductase activity. Virology 442 (2), 132–147. doi: 10.1016/j.virol.2013.04.003
Stanley, D. W., Miller, J. S. (2006). Eicosanoid actions in insect cellular immune functions. Entomol Exp. Appl. 119 (1), 1–13. doi: 10.1111/j.1570-7458.2006.00406.x
Stanley, D., Shapiro, M. (2007). Eicosanoid biosynthesis inhibitors increase the susceptibility of lymantria dispar to nucleopolyhedrovirus LdMNPV. J. Invertebr Pathol. 95 (2), 119–124. doi: 10.1016/j.jip.2007.02.002
Stephenson, C. J., Coatsworth, H., Waits, C. M., Nazario-Maldonado, N. M., Mathias, D. K., Dinglasan, R. R., et al. (2021). Geographic partitioning of dengue virus transmission risk in Florida. Viruses 13 (11), 2232. doi: 10.3390/v13112232
Stevenson, B. J., Bibby, J., Pignatelli, P., Muangnoicharoen, S., O’Neill, P. M., Lian, L.-Y., et al. (2011). Cytochrome P450 6M2 from the malaria vector anopheles gambiae metabolizes pyrethroids: Sequential metabolism of deltamethrin revealed. Insect Biochem. Mol. Biol. 41 (7), 492–502. doi: 10.1016/j.ibmb.2011.02.003
Stillwell, W. (2016). Bioactive lipids. in: An introduction to biological membranes (Elsevier: Elsevier Science), 453–478.
Stone, S. J., Levin, M. C., Zhou, P., Han, J., Walther, T. C., Farese, R. V. (2009). The endoplasmic reticulum enzyme DGAT2 is found in mitochondria-associated membranes and has a mitochondrial targeting signal that promotes its association with mitochondria. J. Biol. Chem. 284 (8), 5352–5361. doi: 10.1074/jbc.M805768200
Strode, C., Wondji, C. S., David, J.-P., Hawkes, N. J., Lumjuan, N., Nelson, D. R., et al. (2008). Genomic analysis of detoxification genes in the mosquito aedes aegypti. Insect Biochem. Mol. Biol. 38 (1), 113–123. doi: 10.1016/j.ibmb.2007.09.007
Sushchik, N. N., Yurchenko, Y. A., Gladyshev, M. I., Belevich, O. E., Kalachova, G. S., Kolmakova, A. A. (2013). Comparison of fatty acid contents and composition in major lipid classes of larvae and adults of mosquitoes (Diptera: Culicidae) from a steppe region. Insect Sci. 20 (5), 585–600. doi: 10.1111/j.1744-7917.2012.01582.x
Tauchi-Sato, K., Ozeki, S., Houjou, T., Taguchi, R., Fujimoto, T. (2002). The surface of lipid droplets is a phospholipid monolayer with a unique fatty acid composition. J. Biol. Chem. 277 (46), 44507–44512. doi: 10.1074/jbc.M207712200
Tettamanti, G., Casartelli, M. (2019). Cell death during complete metamorphosis. Philos. Trans. R Soc. B Biol. Sci. 374 (1783), 20190065. doi: 10.1098/rstb.2019.0065
Tian, L., Ma, L., Guo, E., Deng, X., Ma, S., Xia, Q., et al. (2013). 20-hydroxyecdysone upregulates atg genes to induce autophagy in the bombyx fat body. Autophagy 9 (8), 1172–1187. doi: 10.4161/auto.24731
Tongluan, N., Ramphan, S., Wintachai, P., Jaresitthikunchai, J., Khongwichit, S., Wikan, N., et al. (2017). Involvement of fatty acid synthase in dengue virus infection. Virol. J. 14 (1), 28. doi: 10.1186/s12985-017-0685-9
Tortoriello, G., Rhodes, B. P., Takacs, S. M., Stuart, J. M., Basnet, A., Raboune, S., et al. (2013). Targeted lipidomics in drosophila melanogaster identifies novel 2-monoacylglycerols and n-acyl amides. PloS One 8 (7), e67865. doi: 10.1371/journal.pone.0067865
Townsend, D., Jenkin, H. M., Yang, T. K. (1972). Lipid analysis of aedes aegypti cells cultivated in vitro. biochim biophys acta. Biochimica et Biophysica Acta journal 260, 1, 20–25. doi: 10.1016/0005-2760(72)90069-0
Tree, M. O., Londono-Renteria, B., Troupin, A., Clark, K. M., Colpitts, T. M., Conway, M. J. (2019). Dengue virus reduces expression of low-density lipoprotein receptor-related protein 1 to facilitate replication in aedes aegypti. Sci. Rep. 9 (1), 6352. doi: 10.1038/s41598-019-42803-9
Troy, S., Anderson, W. A., Spielman, A. (1975). Lipid content of maturing ovaries of aedes aegypti mosquitoes. Comp. Biochem. Physiol. B. 50 (3), 457–461. doi: 10.1016/0305-0491(75)90258-8
Tsetsarkin, K. A., Vanlandingham, D. L., McGee, C. E., Higgs, S. (2007). A single mutation in chikungunya virus affects vector specificity and epidemic potential. PloS Pathog. 3 (12), e201. doi: 10.1371/journal.ppat.0030201
Valzania, L., Coon, K. L., Vogel, K. J., Brown, M. R., Strand, M. R. (2018). Hypoxia-induced transcription factor signaling is essential for larval growth of the mosquito aedes aegypti. Proc. Natl. Acad. Sci. 115 (3), 457–465. doi: 10.1073/pnas.1719063115
van den Berg, H., da Silva Bezerra, H. S., Al-Eryani, S., Chanda, E., Nagpal, B. N., Knox, T. B., et al. (2021). Recent trends in global insecticide use for disease vector control and potential implications for resistance management. Sci. Rep. 11 (1), 23867. doi: 10.1038/s41598-021-03367-9
Van der Horst, D. J., Ryan, R. O. (2017). BT-RM in LS. Lipid Transport 4, 225-246. doi: 10.1016/B978-0-12-809633-8.04045-0
Van Heusden, M. C., Erickson, B. A., Pennington, J. E. (1997). Lipophorin levels in the yellow fever mosquito, aedes aegypti, and the effect of feeding. Arch. Insect Biochem. Physiol. 34 (3), 301–312. doi: 10.1002/(SICI)1520-6327(1997)34:3<301::AID-ARCH5>3.0.CO;2-X
van Meer, G., Voelker, D. R., Feigenson, G. W. (2008). Membrane lipids: Where they are and how they behave. Nat. Rev. Mol. Cell Biol. 9 (2), 112–124. doi: 10.1038/nrm2330
Vera-Maloof, F. Z., Saavedra-Rodriguez, K., Penilla-Navarro, R. P., D. Rodriguez-Ramirez, A., Dzul, F., Manrique-Saide, P., et al. (2020). Loss of pyrethroid resistance in newly established laboratory colonies of aedes aegypti. PloS Negl. Trop. Dis. 14 (3), e0007753. doi: 10.1371/journal.pntd.0007753
Vial, T., Tan, W. L., Deharo, E., Missé, D., Marti, G., Pompon, J. (2020). Mosquito metabolomics reveal that dengue virus replication requires phospholipid reconfiguration via the remodeling cycle. Proc. Natl. Acad. Sci. U.S.A. 117 (44), 27627–27636. doi: 10.1073/pnas.2015095117
Vial, T., Tan, W. L., Xiang, B. W. W., Missé, D., Deharo, E., Marti, G., et al. (2019). Dengue virus reduces AGPAT1 expression to alter phospholipids and enhance infection in aedes aegypti. PloS Pathog. 15 (12), e1008199. doi: 10.1371/journal.ppat.1008199
Vogel, K. J., Valzania, L., Coon, K. L., Brown, M. R., Strand, M. R. (2017). Transcriptome sequencing reveals Large-scale changes in axenic aedes aegypti larvae. Dimopoulos G editor PloS Negl. Trop. Dis. 11 (1), e0005273. doi: 10.1371/journal.pntd.0005273
Vontas, J., Katsavou, E., Mavridis, K. (2020). Cytochrome P450-based metabolic insecticide resistance in anopheles and aedes mosquito vectors: Muddying the waters. Pestic Biochem. Physiol. 170, 104666. doi: 10.1016/j.pestbp.2020.104666
Vyazunova, I., Lan, Q. (2010). Yellow fever mosquito sterol carrier protein-2 gene structure and transcriptional regulation. Insect Mol. Biol. 19 (2), 205–215. doi: 10.1111/j.1365-2583.2009.00959.x
Walker, T., Johnson, P. H., Moreira, L. A., Iturbe-Ormaetxe, I., Frentiu, F. D., McMeniman, C. J., et al. (2011). The wMel wolbachia strain blocks dengue and invades caged aedes aegypti populations. Nature 476 (7361), 450–453. doi: 10.1038/nature10355
Wang, L., Fontaine, A., Gaborit, P., Guidez, A., Issaly, J., Girod, R., et al. (2022). Interactions between vector competence to chikungunya virus and resistance to deltamethrin in aedes aegypti laboratory lines? Med. Vet. Entomol. 36 (4), 486–495. doi: 10.1111/mve.12593
Wang, X., Hou, Y., Saha, T. T., Pei, G., Raikhel, A. S., Zou, Z. (2017). Hormone and receptor interplay in the regulation of mosquito lipid metabolism. Proc. Natl. Acad. Sci. 114 (13), E2709–E2718. doi: 10.1073/pnas.1619326114
Wang, D., Yang, J., Pandya, J., Clark, J. M., Harrington, L. C., Murdock, C. C., et al. (2022). Quantitative age grading of mosquitoes using surface-enhanced raman spectroscopy. Anal. Sci. Adv. 3 (1–2), 47–53. doi: 10.1002/ansa.202100052
Welsch, S., Miller, S., Romero-Brey, I., Merz, A., Bleck, C. K. E. E., Walther, P., et al. (2009). Composition and three-dimensional architecture of the dengue virus replication and assembly sites. Cell Host Microbe 5 (4), 365–375. doi: 10.1016/j.chom.2009.03.007
Whitfield, S. G., Murphy, F. A., Sudia, W. D. (1973). St. Louis encephalitis virus: An ultrastructural study of infection in a mosquito vector. Virology 56 (1), 70–87. doi: 10.1016/0042-6822(73)90288-2
World Health Organization (2020) Chikungunya. Available at: https://www.who.int/news-room/fact-sheets/detail/chikungunya.
World Health Organization (2022) Zika virus disease. Available at: https://www.who.int/health-topics/zika-virus-disease#tab=tab_1.
Wu, W.-L., Ho, L.-J., Chang, D.-M., Chen, C.-H., Lai, J.-H. (2009). Triggering of DC migration by dengue virus stimulation of COX-2-dependent signaling cascades in vitro highlights the significance of these cascades beyond inflammation. Eur. J. Immunol. 39 (12), 3413–3422. doi: 10.1002/eji.200939306
Xi, Z., Ramirez, J. L., Dimopoulos, G. (2008). The aedes aegypti toll pathway controls dengue virus infection. PloS Pathog. 4 (7), e1000098. doi: 10.1371/journal.ppat.1000098
Xu, J., Morisseau, C., Yang, J., Mamatha, D. M., Hammock, B. D. (2015). Epoxide hydrolase activities and epoxy fatty acids in the mosquito culex quinquefasciatus. Insect Biochem. Mol. Biol. 59, 41–49. doi: 10.1016/j.ibmb.2015.02.004
Xu, J., Su, X., Bonizzoni, M., Zhong, D., Li, Y., Zhou, G., et al. (2018). Comparative transcriptome analysis and RNA interference reveal CYP6A8 and SNPs related to pyrethroid resistance in aedes albopictus. PloS Negl. Trop. Dis. 12 (11), e0006828. doi: 10.1371/journal.pntd.0006828
Yunta, C., Hemmings, K., Stevenson, B., Koekemoer, L. L., Matambo, T., Pignatelli, P., et al. (2019). Cross-resistance profiles of malaria mosquito P450s associated with pyrethroid resistance against WHO insecticides. Pestic Biochem. Physiol. 161, 61–67. doi: 10.1016/j.pestbp.2019.06.007
Zhao, L., Alto, B., Shin, D., Yu, F. (2018). The effect of permethrin resistance on aedes aegypti transcriptome following ingestion of zika virus infected blood. Viruses 10 (9), 470. doi: 10.3390/v10090470
Zhou, G., Flowers, M., Friedrich, K., Horton, J., Pennington, J., Wells, M. A. (2004a). Metabolic fate of [14C]-labeled meal protein amino acids in aedes aegypti mosquitoes. J. Insect Physiol. 50 (4), 337–349. doi: 10.1016/j.jinsphys.2004.02.003
Zhou, G., Pennington, J. E., Wells, M. A. (2004b). Utilization of pre-existing energy stores of female aedes aegypti mosquitoes during the first gonotrophic cycle. Insect Biochem. Mol. Biol. 34 (9), 919–925. doi: 10.1016/j.ibmb.2004.05.009
Zhou, G., Miesfeld, R. L. (2009). Energy metabolism during diapause in culex pipiens mosquitoes. J. Insect Physiol. 55 (1), 40–46. doi: 10.1016/j.jinsphys.2008.10.002
Ziegler, R. (1991). Changes in lipid and carbohydrate metabolism during starvation in adult manduca sexta. J. Comp. Physiol. B Biochem. Syst. Environ. Physiol. 161 (2), 125–131. doi: 10.1007/BF00262874
Ziegler, R. (1997). Lipid synthesis by ovaries and fat body of aedes aegypti (Diptera: Culicidae). Eur. J. Entomol. 94 (3), 385–391.
Ziegler, R., Ibrahim, M. M. (2001). Formation of lipid reserves in fat body and eggs of the yellow fever mosquito, aedes aegypti. J. Insect Physiol. 47 (6), 623–627. doi: 10.1016/S0022-1910(00)00158-X
Ziegler, R., Vanantwerpen, R. (2006). Lipid uptake by insect oocytes. Insect Biochem. Mol. Biol. 36 (4), 264–272. doi: 10.1016/j.ibmb.2006.01.014
Keywords: Aedes aegypti, mosquito, Wolbachia, metabolism, lipids, dengue, Zika, virus
Citation: Ratnayake OC, Chotiwan N, Saavedra-Rodriguez K and Perera R (2023) The buzz in the field: the interaction between viruses, mosquitoes, and metabolism. Front. Cell. Infect. Microbiol. 13:1128577. doi: 10.3389/fcimb.2023.1128577
Received: 20 December 2022; Accepted: 24 March 2023;
Published: 26 April 2023.
Edited by:
Daniel Ruzek, Veterinary Research Institute (VRI), CzechiaReviewed by:
W. Robert Shaw, Harvard University, United StatesFabrizio Lombardo, Sapienza University of Rome, Italy
Copyright © 2023 Ratnayake, Chotiwan, Saavedra-Rodriguez and Perera. This is an open-access article distributed under the terms of the Creative Commons Attribution License (CC BY). The use, distribution or reproduction in other forums is permitted, provided the original author(s) and the copyright owner(s) are credited and that the original publication in this journal is cited, in accordance with accepted academic practice. No use, distribution or reproduction is permitted which does not comply with these terms.
*Correspondence: Rushika Perera, UnVzaGlrYS5wZXJlcmFAY29sb3N0YXRlLmVkdQ==