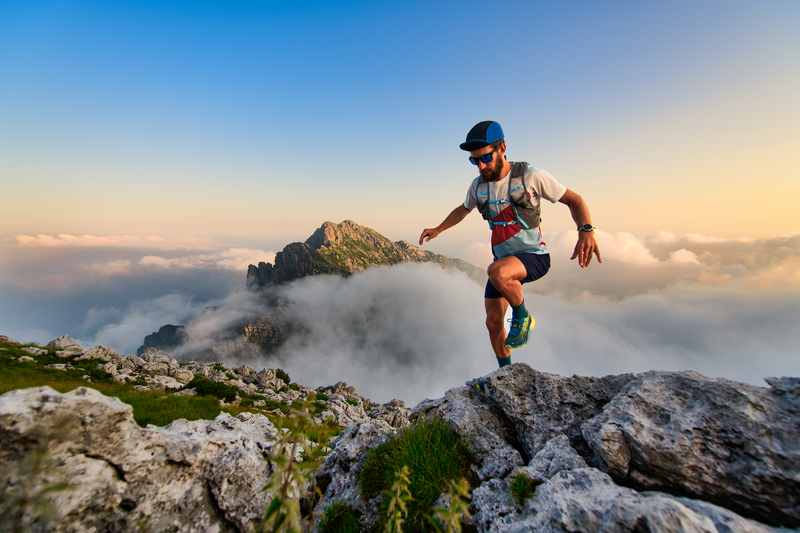
95% of researchers rate our articles as excellent or good
Learn more about the work of our research integrity team to safeguard the quality of each article we publish.
Find out more
ORIGINAL RESEARCH article
Front. Cell. Infect. Microbiol. , 03 February 2023
Sec. Molecular Bacterial Pathogenesis
Volume 13 - 2023 | https://doi.org/10.3389/fcimb.2023.1093393
This article is part of the Research Topic The pathogenic Flavobacteriaceae: recent advances in the understanding of physiology, genetics, and virulence View all 7 articles
Flavobacterium columnare causes columnaris disease in freshwater fish in both natural and aquaculture settings. This disease is often lethal, especially when fish population density is high, and control options such as vaccines are limited. The type IX secretion system (T9SS) is required for F. columnare virulence, but secreted virulence factors have not been fully identified. Many T9SS-secreted proteins are predicted peptidases, and peptidases are common virulence factors of other pathogens. T9SS-deficient mutants, such as ΔgldN and ΔporV, exhibit strong defects in secreted proteolytic activity. The F. columnare genome has many peptidase-encoding genes that may be involved in nutrient acquisition and/or virulence. Mutants lacking individual peptidase-encoding genes, or lacking up to ten peptidase-encoding genes, were constructed and examined for extracellular proteolytic activity, for growth defects, and for virulence in zebrafish and rainbow trout. Most of the mutants retained virulence, but a mutant lacking 10 peptidases, and a mutant lacking the single peptidase TspA exhibited decreased virulence in rainbow trout fry, suggesting that peptidases contribute to F. columnare virulence.
Flavobacterium columnare is a Gram-negative bacterium belonging to the phylum Bacteroidota that causes columnaris disease in freshwater fish (Declercq et al., 2013). This is of particular concern in aquaculture, where high population density of fish leads to greater chances of infection and mortality (Suomalainen et al., 2005; Wagner et al., 2006; Pulkkinen et al., 2010). F. columnare colonizes the gills, skin, and fins, resulting in skin lesions, pigment loss, fin erosion, and respiratory distress (Decostere et al., 1999; Wagner et al., 2006; Pulkkinen et al., 2010; Declercq et al., 2013). Virulence mechanisms of F. columnare are poorly understood, and adequate control and prevention measures are lacking.
The type IX secretion system (T9SS) is required for F. columnare virulence (Li et al., 2017; Thunes et al., 2022). The T9SS secretes soluble and cell-surface associated proteins (Sato et al., 2010; Shrivastava et al., 2013). Proteins secreted by the F. columnare T9SS are potential virulence factors, and most have not been studied. These proteins typically have a signal peptide (SP) at the N-terminus and a conserved C-terminal domain (CTD) at the opposite end that is required for secretion (McBride, 2019). The function of the CTD was first identified during studies of gingipain protease secretion by another member of the Bacteroidota, Porphyromonas gingivalis (Seers et al., 2006). Secreted proteins use the Sec system to cross the cytoplasmic membrane, and then use the T9SS to transit the outer membrane. Three types of CTDs are found on different T9SS-secreted proteins: type A (TIGR04183), type B (TIGR04131), and type C (cl41395) (Kulkarni et al., 2017; Lu et al., 2020; Thunes et al., 2022).
Some proteins that lack recognizable CTDs may also require the T9SS to leave the cell. This was suggested by liquid chromatography-tandem mass spectrometry (LC-MS/MS) experiments that found such proteins in the cell-free spent media of wild-type cultures but not in those from a T9SS-deficient mutant (Li et al., 2017; Thunes et al., 2022). These nonCTD proteins may be recognized by the T9SS by a different mechanism, or their secretion may be only indirectly affected by loss of the T9SS. For example, the T9SS may secrete a cell surface component of another secretion system that handles the nonCTD proteins. Alternatively, the unnatural accumulation of proteins with T9SS-CTDs in the periplasm of a T9SS-defective mutant could interfere with another secretion system or could otherwise impact the cell surface and alter secretion. Regardless of the cause, these extracellular ‘nonCTD’ proteins that require the T9SS for their apparent secretion are also possible virulence factors.
Of the previously identified secreted F. columnare proteins in cell-free spent media, thirty-five were identified as predicted peptidases, and eleven of these had obvious T9SS CTDs (Thunes et al., 2022). Such secreted peptidases with T9SS-CTDs are referred to in this paper as ‘CTD-peptidases’. Peptidases (also referred to as proteases) are secreted by bacteria, fungi, and other organisms (Clarke et al., 2016). They are often used for nutrient acquisition and may also contribute to virulence (Mckerrow et al., 1993; Klemba and Goldberg, 2002; Kolar et al., 2013; Ruiz-Perez and Nataro, 2014; Yang et al., 2015; Zhang et al., 2015). P. gingivalis secretes its major virulence factors, gingipain proteases, via its T9SS (Vincent et al., 2017; Benedyk et al., 2019). F. columnare secreted peptidases may contribute to the skin lesions and necrosis that are characteristic signs of columnaris disease (Newton et al., 1997; Dumpala et al., 2010). Targeting peptidases as treatment or preventative strategies has been effective for some pathogens (Klemba and Goldberg, 2002; Clarke et al., 2016), providing a rationale to study F. columnare secreted peptidases.
The fish pathogen Flavobacterium psychrophilum also secretes many peptidases that were proposed as potential virulence factors (Duchaud et al., 2007). Mutation of several peptidase-encoding genes reduced proteolytic activities but had no effect on virulence in rainbow trout (Pérez-Pascual et al., 2011; Gómez et al., 2015). For both F. psychrophilum and F. columnare the large number of predicted secreted peptidases makes it difficult to determine which, if any, contribute to virulence. It also suggests the possibility that partial redundancy may occur between some of the peptidases.
Recently, F. columnare strain MS-FC-4 was shown to be more amenable to genetic manipulation than other strains examined (Thunes et al., 2022). This allowed efficient construction of strains with multiple genes deleted (Thunes et al., 2022; Conrad et al., 2022a; Conrad et al., 2022b). Here we took advantage of the relative ease of genetic manipulation and constructed a series of gene deletion mutants to explore the roles of 20 peptidases in F. columnare virulence in fish.
Cell-free spent culture fluid of wild-type F. columnare strain MS-FC-4 was previously analyzed for proteins that were secreted by wild-type cells, but were secreted poorly, if at all, by the T9SS-deficient mutant ΔgldN (Thunes et al., 2022). Thirty-five secreted proteins predicted to be peptidases were identified in that study. We reexamined the data from that study and identified three additional predicted peptidases (C6N29_07780, C6N29_08680, and C6N29_08590) (Table S1). Twelve of the 38 secreted peptidases had CTDs predicted to target them for secretion by the T9SS. We refer to these as ‘CTD-peptidases’, and the corresponding peptidase-encoding genes as ‘CTD-peptidase genes’. Ten CTD-peptidase genes were deleted, generating mutants lacking these genes (Tables 1, S1, S2). Deletions were constructed sequentially, resulting in ‘chain mutants’ lacking up to ten CTD-peptidase genes. Two of the CTD-peptidase genes (porU and C6N29_09900) were not deleted in this study. PorU functions in secretion, where it cleaves T9SS CTDs (Glew et al., 2012). Because T9SS mutants have already been characterized for virulence defects (Li et al., 2017; Thunes et al., 2022), we did not focus on this peptidase in this study, and thus did not delete porU. We attempted, but failed, to delete C6N29_09900 from chain mutant FCB167, which lacks 10 other CTD-peptidase genes. We did not delete five other CTD-peptidase genes (C6N29_02760, C6N29_05620, C6N29_08010, C6N29_12610, C6N29_12705), for which the encoded proteins were not detected in cell-free culture fluid (Thunes et al., 2022).
Table 1 Peptidase mutants used in this studya.
A second chain deletion mutant was constructed lacking genes encoding peptidases that appeared to require the T9SS for secretion but that lacked obvious T9SS CTDs. We refer to these secreted peptidases as ‘nonCTD-peptidases’ (Table 1). Genes were prioritized primarily based on number of spectral counts of gene products in cell-free culture fluid from wild-type cells compared to those of the T9SS-deficient ΔgldN mutant (Table S1). Both chain mutants started with deletion of the CTD-peptidase gene, ΔC6N29_05800. A mutant (FCB54) lacking this gene and also lacking two nonCTD-peptidase genes (ΔC6N29_11545 and ΔC6N29_11550) was previously shown to exhibit decreased proteolytic activity (Thunes et al., 2022). FCB54 was chosen as the background to delete additional nonCTD-peptidase genes, resulting in the ‘nonCTD-peptidase chain’ mutants. Sequential deletions resulted in chain mutants lacking up to nine nonCTD-peptidase genes, each of which also lacked the CTD-peptidase gene ΔC6N29_05800 (Table 1).
Conserved domains associated with peptidases were identified in the proteins encoded by the CTD-peptidase genes and nonCTD-peptidase genes that were deleted in this study (Table 2). The proteins were diverse, belonging to different peptidase families. Fourteen were predicted to be metallopeptidases. Sixteen of the peptidases are similar in sequence to peptidases of the related fish pathogen, F. psychrophilum (Table 2).
Wild-type and CTD-peptidase gene deletion mutants were examined for extracellular proteolytic activity (Figure 1A). The T9SS-deficient ΔgldN mutant, which is deficient in secretion of peptidases targeted to the T9SS (Thunes et al., 2022), was also included in this analysis. In most cases, the sequential deletion of CTD-peptidase genes resulted in a partial decline in extracellular proteolytic activity. The largest changes occurred upon deletion of the fourth (C6N29_10335) and ninth (C6N29_08145) of the CTD-peptidase genes that were targeted. The mutants lacking 9 or 10 peptidase-encoding genes showed severe defects in extracellular proteolytic activity compared to wild type. These defects were similar to those of the T9SS-defective ΔgldN mutant.
Figure 1 Effect of deletion of genes encoding peptidases with T9SS CTDs on extracellular proteolytic activities. (A) Strains examined were wild-type F. columnare (WT); FCB20 (Δ1-CTD-P); FCB98 (Δ2-CTD-P); FCB105 (Δ3-CTD-P); FCB109 (Δ4-CTD-P); FCB134 (Δ5-CTD-P); FCB139 (Δ6-CTD-P); FCB142 (Δ7-CTD-P); FCB145 (Δ8-CTD-P); FCB155 (Δ9-CTD-P); FCB167 (Δ10-CTD-P); ΔgldN. (B) Strains examined were wild-type F. columnare (WT); FCB155 (Δ9-CTD-P); FCB222 (ΔC6N29_08145); FCB167 (Δ10-CTD-P); FCB230 (ΔC6N29_13645); ΔC6N29_13645 mutant with wild-type C6N29_13645 restored to the chromosome (ΔC6N29_13645C); Δ10-CTD-P mutant with wild-type C6N29_13645 restored to the chromosome (Δ10-CTD-PC); ΔgldN. Statistics correspond to one-way ANOVA with Tukey post-test comparing all conditions to wild type and each other. Same letter: nonsignificant.
We deleted the 9th (C6N29_08145) and 10th (C6N29_13645) CTD-peptidase genes from wild-type cells to determine if either of these contributed a large amount of extracellular proteolytic activity by itself. Deletion of C6N29_08145 from wild-type cells did not cause a significant reduction in proteolysis, whereas deletion of C6N29_13645 caused a partial reduction of proteolytic activity (Figure 1B). A wild-type copy of C6N29_13645 (the 10th CTD-peptidase) was recombined into its native site on the chromosome of the single mutant (ΔC6N29_13645) and of the Δ10-CTD-P chain mutant to determine if secreted proteolytic activity was restored. This restored proteolysis to the single mutant (ΔC6N29_13645) but not to the Δ10-CTD-P mutant. The defects in the Δ9-CTD-P and Δ10-CTD-P mutants appear to be caused by the cumulative effects of the deletion of multiple peptidase-encoding genes rather than primarily by the loss of either C6N29_08145 or C6N29_13645.
NonCTD-peptidase gene deletion chain mutants were also examined for extracellular proteolytic activity (Figure 2). These mutants had deletions spanning genes that lacked identifiable T9SS-associated CTDs, but each also lacked one CTD-peptidase gene, ΔC6N29_05800. The parent strain FCB54 (which we refer to here as Δ2-nonCTD-P), lacking a CTD-peptidase gene and two nonCTD-peptidase genes, exhibited less extracellular proteolytic activity than did wild type, as was previously reported (Thunes et al., 2022). Mutants lacking up to 6-nonCTD-peptidase genes displayed levels of extracellular proteolytic activity similar to the parent strain, FCB54. Deletion of the 7th nonCTD-peptidase gene C6N29_14605 restored proteolytic activity to near wild type levels. In contrast, deletion of the eighth nonCTD-peptidase gene C6N29_12115, resulted in decreased proteolytic activity.
Figure 2 Effect of deletion of genes encoding peptidases that lack T9SS CTDs on extracellular proteolytic activities. (A) Strains examined were wild-type F. columnare (WT); FCB54 (Δ2-nonCTD-P); FCB100 (Δ3-nonCTD-P); FCB101 (Δ4-nonCTD-P); FCB102 (Δ5-nonCTD-P); FCB103 (Δ6-nonCTD-P); FCB107 (Δ7-nonCTD-P); FCB117 (Δ8-nonCTD-P); FCB137 (Δ9-nonCTD-P); ΔgldN. (B) Strains examined were WT; FCB107 (Δ7-nonCTD-P); FCB117 (Δ8-nonCTD-P); FCB147 (ΔC6N29_12115); FCB137 (Δ9-nonCTD-P); FCB159 (ΔC6N29_06855); ΔC6N29_12115 complemented with plasmid pHM23 (ΔC6N29_12115C); Δ8-nonCTD-P complemented with plasmid pHM23 (Δ8-nonCTD-PC); ΔgldN. Statistics correspond to one-way ANOVA with Tukey post-test comparing all conditions to wild type and each other. Same letter and color: nonsignificant.
Single deletion mutants lacking the 8th (C6N29_12115) and 9th (C6N29_06855) nonCTD-peptidase genes were constructed, as were complemented strains. Deletion of the 8th (C6N29_12115) or 9th (C6N29_06855) nonCTD-peptidase genes from wild-type cells each caused a reduction in proteolysis compared to wild type. Complementation by introduction of multicopy plasmid pHM23 carrying C6N29_12115 into the single deletion mutant (ΔC6N29_12115) and into the Δ8-nonCTD-P mutant resulted in extracellular proteolytic activity in excess of that exhibited by wild-type cells (Figure 2B).
C6N29_08680 is listed as a potential peptidase-encoding gene in the MEROPS peptidase database (https://www.ebi.ac.uk/merops/) (Rawlings et al., 2018). Unlike the other peptidase-encoding genes studied here it encodes a predicted tail-specific protease, and we thus refer to the gene as tspA. The encoded protein, TspA, lacks a T9SS CTD. In Gram-negative bacteria, tail-specific proteases, also referred to as carboxy-terminal processing proteases, are typically periplasmic (Hoge et al., 2011). As the names imply, they typically cleave specific C-terminal regions from target proteins. They are thought to impact the bacterium primarily by their effects on these bacterial target proteins. A tspA deletion mutant was constructed and screened for proteolytic activity. The deletion mutant exhibited extracellular proteolytic activity per mg bacterial cell protein that was similar to that of the wild type (Figure S1).
Wild-type F. columnare and the peptidase mutants were examined for virulence against germ-free zebrafish larvae in a challenge experiment (Figure 3). No significant differences in virulence were seen for any of the mutants when compared to the wild type. In contrast, the T9SS-deficient ΔgldN mutant was avirulent, as previously reported (Thunes et al., 2022). A challenge experiment was also conducted for mutants lacking nine and ten CTD-peptidase genes (Δ9-CTD-P and Δ10-CTD-P, respectively) and a mutant lacking one CTD-peptidase gene and nine nonCTD-peptidase genes (Δ9-nonCTD-P) to examine virulence in adult zebrafish. No defects in virulence (as determined by monitoring percent survival after challenge) were observed in either case (Figure S2).
Figure 3 Virulence of F. columnare wild type and peptidase mutants toward germ-free (GF) zebrafish larvae. Fish were infected at 6 days post-fertilization by immersion at 104 colony-forming-units (CFU)/mL. Zero days post-infection (dpi) corresponds to the day of infection. Mean survival is represented by a thick horizontal bar with error bars for standard deviation. “GF” (germ-free) indicates noninfected larvae. Length of survival is shown for larvae exposed to the strains indicated. The number of days of survival for fish challenged with any of the peptidase gene deletion mutant strains were not significantly different from those of the wild type. The survival for fish challenged with the ΔgldN mutant was not significantly different from the noninfected GF control.
Each CTD-peptidase chain mutant (Table 1) was tested for virulence in juvenile rainbow trout (also referred to as fry) (Figure 4). Only the Δ10-CTD-P chain mutant showed decreased virulence compared to wild type (Figure 4A and data not shown). Fish that survived exposure to Δ10-CTD-P were maintained for 28 days and then examined for sensitivity to challenge with the wild type. These fish were not protected against the later challenge (Figure 4B). Chain mutants in which nonCTD-peptidase-encoding genes were deleted were also examined for virulence (Figure 4C). A decrease in virulence was observed in the Δ9-nonCTD-P chain mutant, but not in the Δ8-nonCTD-P mutant. The mutants described above were also examined for virulence against rainbow trout alevin (sac-fry). The mutant lacking 10-CTD-peptidase-encoding genes (Δ10-CTD-P) showed a decrease in virulence in rainbow trout alevin (Figure 5). Other CTD and nonCTD chain mutants tested caused similar mortality to wild type in alevin (data not shown).
Figure 4 Effect of deletion of peptidase-encoding genes on virulence in juvenile rainbow trout. (A) Rainbow trout were exposed by immersion to wild-type and mutant strains and survival was monitored for 21 days. The final challenge concentrations were 3.4 × 107 CFU/mL (WT), 6.6 × 105 CFU/mL (ΔgldN), 2.6 × 107 CFU/mL (Δ9-CTD-P), 1.3 × 107 CFU/mL (Δ10-CTD-P). Significant differences in percent survival for fish challenged were observed between the WT and Δ10-CTD-P (P<0.0001). (B) Fish that survived exposure to Δ10-CTD-P (Δ10-CTD-P survivors) were examined for resistance to wild-type cells. Naïve fish and Δ10-CTD-P survivors were maintained for 28 d and then exposed to wild-type cells at 3.8 × 107 CFU/ml. Survival was monitored for 12 d. Fish were exposed to TYES without bacteria as a control. (C) Rainbow trout were exposed by immersion to wild-type and mutant strains and survival was monitored. The final challenge concentrations were 3.4 × 107 CFU/mL (WT), 6.6 × 105 CFU/mL (ΔgldN), 3.6 × 107 (Δ8-nonCTD-P), 2.7 × 107 CFU/mL (Δ9-nonCTD-P). Significant differences in percent survival for fish challenged were observed between the WT and Δ9-nonCTD-P (P<0.0001).
Figure 5 Effect of deletion of peptidase-encoding genes on virulence in rainbow trout alevin (sac-fry). Alevin were exposed by immersion to F. columnare strains and monitored for 8 d. The final challenge concentrations were 6.9 × 106 CFU/mL (WT), 1.1 × 107 CFU/mL (ΔgldN), and 8.0 × 106 CFU/mL (Δ10-CTD-P). Significant differences in percent survival for fish challenged were observed between the WT and Δ10-CTD-P (P<0.0001).
A single deletion mutant lacking the 10th CTD-peptidase gene (C6N29_13645) was examined for virulence in juvenile rainbow trout (Figure 6). ΔC6N29_13645 displayed similar virulence to wild type, indicating that deletion of the single gene, C6N29_13645, was not responsible for the defect in virulence observed in Δ10 CTD-P. Introduction of C6N29_13645 by genomic integration into the Δ10-CTD-P background restored virulence to wild-type levels. Thus, C6N29_13645 appears to be important for F. columnare virulence, but deletion of other peptidase-encoding genes and C6N29_13645 was necessary for defects in virulence.
Figure 6 Effect of deletion of peptidase-encoding genes on virulence of F. columnare in juvenile rainbow trout. Rainbow trout were exposed by immersion to wild-type and mutant strains and survival was monitored for 21 days. Strains examined were wild-type F. columnare (WT); ΔgldN; Δ10-CTD-P; Δ9-CTD-P; ΔC6N29_13645; Δ10-CTD-P complemented by chromosomal insertion of C6N29_13645 (Δ10-CTD-PC). The final challenge concentrations were 7.6 × 106 CFU/mL (WT), 9.3 × 106 CFU/mL (ΔgldN), 7.1 × 106 CFU/mL (Δ10-CTD-P), 7.3 × 106 CFU/mL (Δ9-CTD-P), 7.6 × 106 CFU/mL (ΔC6N29_13645), and 5.4 × 106 CFU/mL (Δ10-CTD-PC). Significant differences in percent survival for fish challenged were observed between the WT and Δ10-CTD-P (P<0.0001).
Deletion of C6N29_08680 (ΔtspA) caused decreased virulence in rainbow trout alevin, and rainbow trout juveniles, and adult zebrafish (Figure 7 and Figure S3). Juvenile rainbow trout that survived exposure to ΔtspA were maintained for 28 days and then challenged with wild-type F. columnare. Previous exposure to ΔtspA did not protect most fish from later exposure to wild type F. columnare (Figure 7C).
Figure 7 Effect of deletion of tspA, which encodes a tail-specific protease, on virulence. Strains examined were wild-type F. columnare (WT), ΔtspA, and ΔgldN. (A) Rainbow trout alevin were exposed by immersion to F. columnare and survival was monitored for 8 d. The final challenge concentrations were 6.9 × 106 CFU/mL (WT), 1.1 × 107 CFU/mL (ΔgldN), and 9.0× 105 CFU/mL (ΔtspA). Significant differences in percent survival for fish challenged were observed between the WT and ΔtspA (P<0.0001). (B) Rainbow trout juveniles were exposed by immersion to F. columnare strains and survival was monitored for 21 d. The final challenge concentrations were 3.4 × 107 CFU/mL (WT), 6.6 × 105 CFU/mL (ΔgldN), and 9.6 × 106 CFU/mL (ΔtspA). Significant differences in percent survival for fish challenged were observed between the WT and ΔtspA (P<0.0001). (C) Juvenile rainbow trout that survived exposure to ΔtspA (ΔtspA survivors) were examined for resistance to wild-type cells. Naïve fish and ΔtspA survivors were maintained for 28 d and then exposed to wild-type cells at 3.8 × 107 CFU/mL. Survival was monitored for 12 d. Fish were exposed to TYES without bacteria as a control. Significant differences in percent survival for fish challenged were observed between naïve fish and ΔtspA survivors (P<0.05).
Strains with severe defects in proteolytic activity or virulence were tested for differences in growth rate and survival compared to wild type (Figure 8). Mutants lacking peptidase genes with T9SS CTDs (Figure 8A) did not exhibit obvious growth defects compared to wild type. The T9SS-deficient mutant ΔgldN also grew as well as the wild type, as was also previously shown (Thunes et al., 2022). We also grew the strains in 50% TYES, which has half of the nutrients of the full-strength medium. In this medium the ΔgldN mutant unexpectedly outperformed the wild type at later time points, perhaps indicating greater stability of the mutant after cells reached stationary phase of growth.
Figure 8 Growth curves for wild-type (WT) and mutant F. columnare strains. Cells were grown in TYES medium or 50% TYES medium at 28°C in a 48-well plate for 36 hours. Growth curves were performed in triplicate and error bars indicate standard error of the mean. (A) WT; ΔgldN; Δ9-CTD-P; Δ10-CTD-P. (B) WT; ΔgldN; Δ8-nonCTD-P; Δ9-nonCTD-P. Significant differences in bacterial cell density were observed in 50% TYES for Δ8-nonCTD-P and Δ9-nonCTD-P compared to the WT (P<0.0001). (C) WT; ΔgldN; ΔtspA, and ΔtspA complemented with pHM22 (ΔtspAc). Significant differences in bacterial cell density were observed in TYES for ΔtspA compared to the WT (P < 0.05).
The Δ8-nonCTD-P mutant grew similar to wild type in TYES (Figure 8B). In contrast, the Δ9-nonCTD-P mutant exhibited a growth defect, reaching a lower maximum optical density. In 50% TYES, both mutants exhibited growth defects compared to the wild type.
Deletion of the tail-specific protease gene, tspA, caused a growth defect in TYES (Figure 8C), resulting in decreased final optical density compared to the wild type. This defect was less noticeable in 50%TYES medium. The growth defect exhibited by this mutant may have contributed to the decreased virulence that was observed for cells grown in TYES and used to challenge rainbow trout (Figure 7).
F. columnare causes high mortality and large economic losses in freshwater aquaculture. Although the T9SS has been implicated in columnaris disease (Li et al., 2017; Thunes et al., 2022), little is known regarding which of the many secreted proteins impact virulence. Secreted proteases have previously been suggested as virulence factors of F. columnare (Bertolini and Rohovec, 1992; Newton et al., 1997; Declercq et al., 2013). Disruption of the T9SS by deletion of gldN or porV greatly reduces extracellular proteolytic activity and eliminates virulence (Li et al., 2017; Thunes et al., 2022). Here we selected 20 of 38 identified genes encoding secreted peptidases for deletion to determine if any are critical for virulence. We focused only on those peptidases that were present in cell-free spent TYES medium collected from wild-type cultures at mid-log phase, and that were secreted at much lower level if at all by the avirulent and T9SS-defective ΔgldN mutant (Thunes et al., 2022). The wild-type cells used in our fish challenge experiments were grown to the same growth phase in TYES, so it is likely that they also produced and secreted these peptidases.
The expression of so many peptidases makes functional redundancy likely. Because of this we constructed CTD-peptidase and nonCTD-peptidase chain mutants lacking multiple peptidases. Mutants from both deletion chains exhibited defects in extracellular proteolysis. Defects in extracellular proteolytic activity similar to those of the ΔgldN mutant were observed for mutants in both chains that lacked nine or ten protease-encoding genes. Investigation of single gene deletions and complemented strains suggested that the source of the proteolysis defects in the CTD chain mutant was a cumulative effect of deletion of multiple genes. However, deletion of the single nonCTD peptidase gene C6N29_03570 also resulted in a significant reduction in proteolysis. Surprisingly, deletion of one peptidase-encoding gene (C6N29_14605) from a strain lacking seven other peptidase-encoding genes resulted in increased levels of proteolysis (Figure 2, Δ7-nonCTD-P). We do not know the reason for this, but one possibility is that deletion of the peptidase-encoding gene caused a regulatory effect that increased expression of other peptidases.
In challenges of larval and adult zebrafish, chain mutants lacking up to 10 CTD-peptidase genes, and up to nine nonCTD-peptidase genes were as virulent as the wild type. Similarly in rainbow trout fry, chain mutants lacking up to nine CTD-peptidase genes, and up to eight nonCTD-peptidase genes were fully virulent. However, a mutant lacking 10 CTD-peptidase genes and a mutant lacking nine nonCTD-peptidase genes exhibited partial defects in virulence for rainbow trout fry, and the Δ10-CTD-P mutant also exhibited decreased virulence in rainbow trout alevin. Virulence was restored to the Δ10-CTD-P mutant by introduction of C6N29_13645, the 10th CTD-peptidase gene that had been deleted in the Δ10-CTD-P chain mutant. However, a mutant lacking just C6N29_13645 was fully virulent. Together, these results suggest that C6N29_13645 is important for virulence, but that the decreased virulence observed for the Δ10-CTD-P mutant resulted from the cumulative effect of deletion of C6N29_13645 and other peptidase-encoding genes. This suggests partial redundancy of function of some peptidases involved in virulence.
Previously, a mutant lacking three genes, the CTD-peptidase gene C6N29_05800 and two nonCTD-peptidase genes, C6N29_11545 and C6N29_11550, displayed a decrease in virulence in rainbow trout fry compared to wild type (Thunes et al., 2022). In contrast, in the current study mutants lacking these genes appeared to be fully virulent. This discrepancy may be the result of differences in the challenge doses used in the different studies. Regardless, in both studies the absence of these three genes did not result in avirulence.
Unlike other mutants lacking single peptidase-encoding genes, deletion of tspA, which encodes a predicted tail-specific protease, caused decreased mortality in adult zebrafish, juvenile rainbow trout, and rainbow trout alevin. In contrast, deletion of tspA did not cause a noticeable decrease in extracellular proteolytic activity. This is not surprising because as a bacterial tail-specific protease, TspA is expected to process specific regions of select bacterial proteins rather than to function as a general protease. We do not know the function of TspA, but it likely impacts the functions of the specific proteins that it processes. The ΔtspA mutant exhibited a growth defect in the rich growth medium, TYES. We do not know the reason for this growth defect, but it may explain the decreased virulence of this mutant. In other bacteria, mutations in genes encoding tail-specific proteases have resulted in defects in growth, virulence, and sensitivity to various stresses (Saoud et al., 2021; Sommerfield and Darwin, 2022).
Juvenile rainbow trout that survived exposure to Δ10-CTD-P were not protected against later exposure to the wild type. These mutants may not have mounted enough of an infection to result in a strong immune response. Alternatively, the peptidases that were absent in the mutant may have been protective antigens, and in their absence, a protective immune response may not occur. Further study of mutants lacking secreted proteins may identify some that are attenuated for virulence but that generate protective immune responses.
The Δ10-CTD-P gene deletion mutant exhibited decreased virulence in rainbow trout fry, but not in adult or larval zebrafish. There are many differences between zebrafish and rainbow trout, and between the challenge protocols used, that may account for these results. For example, zebrafish are warm-water fish, whereas rainbow trout are cold-water fish. Unlike some other temperature-dependent aquatic pathogens (Tang et al., 2020), F. columnare can infect different hosts at different temperatures. The temperatures used in the challenges (26°C to 28°C for zebrafish and 16°C for rainbow trout) may have altered F. columnare gene expression, enzyme activity, or enzyme stability, affecting virulence. Adult or larval zebrafish and juvenile rainbow trout may also have differences in their innate resistance to F. columnare strains.
Peptidases have been suggested as virulence factors for F. columnare and related Flavobacterium fish pathogens (Declercq et al., 2013). Deletion of a pair of F. psychrophilum peptidase-encoding genes resulted in decreased proteolytic activity but resulted in no significant defect in virulence (Pérez-Pascual et al., 2011). This mimics many of the results presented here for F. columnare. Only the ΔtspA, Δ10-CTD-P, and Δ9-nonCTD-P mutants displayed reduced virulence in rainbow trout. Peptidases appear to be important for virulence, but understanding their exact roles requires further study. We deleted many genes encoding predicted peptidases, but our study was not exhaustive. Other F. columnare peptidase-encoding genes that were not examined here could also contribute to virulence.
The results presented here indicate that secreted CTD-peptidases and nonCTD-peptidases contribute to virulence. Some peptidases have significant effects individually on virulence, some appear to be redundant, and others may have little if any effect on virulence. Further studies are needed to fully elucidate which of the many peptidases are most critical, and under what conditions they are expressed. Such proteins could be targets for therapeutics or vaccine development.
F. columnare wild-type strain MS-FC-4 (Evenhuis and LaFrentz, 2016; Bartelme et al., 2018) and mutants derived from this strain were grown at 28°C for liquid cultures, and at 30°C for agar cultures, in tryptone yeast extract salts (TYES) medium as previously described (Cain and LaFrentz, 2007; Thunes et al., 2022). F. columnare cultures used for rainbow trout challenges were grown in TYES-2xMg, which is identical to TYES except that it contains twice as much MgSO4. E. coli strains were grown at 37°C in lysogeny broth (LB) (Bertani, 1951). The strains and plasmids used in this study are listed in Table S2, and primers are listed in Table S3. Antibiotics were used in the following concentrations unless otherwise specified: 100 µg/mL ampicillin, 1 µg/mL tetracycline, and 1 µg/mL tobramycin.
Plasmids were transferred from E. coli S17-1 λ pir into F. columnare strain MS-FC-4 by conjugation as previously described (Thunes et al., 2022).
In-frame gene deletion mutants were constructed as previously described (Li et al., 2015; Li et al., 2017; Thunes et al., 2022). These deletions leave upstream and downstream regions unaltered to limit the possibility of polar effects on downstream genes. To delete C6N29_05315, a 2.2 kbp region upstream of C6N29_05315 was amplified by PCR using Phusion DNA polymerase (New England Biolabs, Ipswich, MA) and primers 2270 (adding a KpnI site) and 2271 (adding a XbaI site). The product was digested with KpnI and XbaI and ligated into pMS75 that had been digested with the same enzymes, to produce pNT23. A 2.5 kbp region downstream of C6N29_05315 was amplified using primers 2272 (adding a XbaI site) and 2273 (adding a PstI site). The product was digested with XbaI and PstI and ligated into pNT23 that had been digested with the same enzymes, to generate pNT32. Plasmid pNT32 was transferred to F. columnare MS-FC-4 by conjugation, and colonies with the plasmid recombined into the chromosome were obtained by selecting for tetracycline resistance. Colonies were streaked for isolation on TYES containing 5 µg/mL tetracycline and isolated colonies were grown in liquid without tetracycline to allow for plasmid loss. The cells were plated on TYES media containing 5% sucrose and the mutant was obtained by selecting for sucrose resistance. PCR was performed to confirm the deletion. The other gene deletion mutants were constructed in the same way, using the primers listed in Table S3 to obtain the appropriate upstream and downstream regions to construct the plasmids listed in Table S2.
A 1.3 kbp fragment spanning C6N29_12115 was amplified using Phusion DNA polymerase and primers 2535 (adding a KpnI site) and 2536 (adding a SphI site). The product was digested with KpnI and SphI and ligated into the shuttle vector pCP23, which was digested with the same enzymes, to produce pHM23. pHM23 was transferred into the F. columnare C6N29_12115 deletion mutant by conjugation. Plasmid complementation of other mutants was conducted in the same way, using the plasmids listed in Table S2, and the primers listed in Table S3.
A 7.4 kbp fragment spanning C6N29_13645 and 2 kbp regions upstream and downstream was amplified using Q5 DNA Polymerase (New England Biolabs, Ipswich, MA) and primers 2298 (adding a KpnI site) and 2301 (adding a PstI site). The product was digested with KpnI and PstI and ligated into the suicide vector pMS75, which was digested with the same enzymes, to produce pNT76. pNT76 was transferred into the F. columnare C6N29_13645 deletion mutant by conjugation. Colonies containing pNT76 recombined into the chromosome were selected using tetracycline resistance (5 µg/mL tetracycline). These were then grown without antibiotic to allow plasmid loss by a second recombination event, and plated on media containing sucrose to eliminate cells that retained the integrated plasmid. Sucrose resistant colonies were screened using PCR to confirm the reinsertion of C6N29_13645 into its native site.
Growth curves were performed as previously described with slight modifications (Thunes et al., 2022). F. columnare cultures were streaked from freezer stocks onto TYES agar and incubated for 24 h at 30°C. These cultures were used to inoculate 50 mL of TYES broth and were incubated 14 h at 28°C shaking at 200 rpm. Cultures were standardized to an OD600 of 0.5 and used to inoculate wells in a 48-well polystyrene plate. 50 µL of culture and 950 µL of TYES were added to each well. All cultures were analyzed in triplicate using TYES as a negative control and blank. Plates were incubated at 28°C with shaking at 200 rpm and readings were taken at two-hour intervals for 36 h using a CLARIOstar Microplate Reader (BMG Labtech, Ortenberg, Germany).
Proteolytic activity was quantified using azocasein as a substrate as previously described (Thunes et al., 2022), except that the F. columnare cultures were grown for two additional hours before analysis. In particular, strains were incubated in 50 mL TYES broth overnight at 28°C with shaking at 200 rpm. These overnight cultures were standardized to an OD600 of 0.5 and then 120 µL was used to inoculate 6 mL TYES broth. Triplicate cultures were incubated at 28°C with shaking at 200 rpm for 22 h (Thunes et al., 2022). Cells were removed by centrifugation and the supernatant was filtered to result in cell-free spent culture fluid. Protein concentration was determined for bacterial cell pellets, and protease activity was determined on the cell-free spent culture fluid as described (Thunes et al., 2022).
Zebrafish (Danio rerio) challenges were performed as previously described (Thunes et al., 2022), with minor modifications as indicated below. F. columnare wild-type, mutant, and complemented mutant strains were grown in TYES overnight at 28°C. 5 ml of overnight culture was diluted into 25 mL of fresh TYES and grown until OD600 reached 0.5. To determine the number of live cells per mL, cultures were serially diluted and plated on TYES agar. To test the virulence of each strain, naïve adult Eckwill zebrafish were immersed in a solution of 0.5 ml F. columnare cells and 99.5 mL dechlorinated Milwaukee municipal tap water for 30 min at 26°C. Control fish were exposed to 0.5 mL growth medium without F. columnare in 99.5 mL of water. After exposure fish were moved to tanks with 2 L fresh water at 28°C and observed for up to ten days for signs of infection. Each treatment was performed in triplicate tanks, with each tank containing five zebrafish in 2 L water. Mortalities were recorded daily. A minimum of 20% of the fish that died were examined for the presence of bacteria phenotypic of F. columnare (yellow, rhizoid, tobramycin resistant colonies) by swabbing gills, fins, and skin, streaking on TYES agar containing tobramycin, and incubating for 2 d at 30°C. F. columnare was detected in all mortalities tested. No signs of disease were observed prior to challenge and no indications of F. columnare or columnaris disease were observed in the uninfected control tanks or in the maintenance tanks at any time.
In some cases, survivors of challenges with F. columnare mutants were examined for resistance to later infection by wild-type F. columnare. For these experiments, fish were maintained in 2 L of water for 28 d after the initial exposure to the mutant strain. Some of the fish were then challenged with the wild type, as described above, and others were exposed to the same volume of TYES medium without bacteria. Naïve fish were also challenged with the wild type at the same time.
Germ-free zebrafish larvae were challenged with wild-type, mutant, and complemented strains of F. columnare strain MS-FC-4 at 28°C as previously described (Stressmann et al., 2021). Briefly, 10 to 12 germ-free larvae (6 days postfertilization) were exposed to 104 colony-forming-units (CFU)/mL of washed F. columnare cells for 3 hours in 25-cm3 culture flasks with vented caps containing 20 mL of sterile mineral water. They were then transferred individually to 24-well plates containing 2 mL sterile water per well. Larvae were fed every 48 h with 50 µL of germ-free Tetrahymena thermophila per well. Mortalities were counted daily and measured in days-post-infection (dpi) with 0 dpi corresponding to the infection day. All zebrafish larval experiments were stopped at 9 dpi, and zebrafish were euthanized with tricaine (MS-222) (Sigma-Aldrich; catalog no. E10521). Each experiment was repeated at least twice.
Rainbow trout (Oncorhynchus mykiss) challenges for juveniles and alevin were performed using rainbow trout reared from certified disease-free eggs from Troutlodge Inc., Sumner, WA. Trout were maintained as described previously (Thunes et al., 2022) at the USDA-ARS National Center for Cool and Cold Water Aquaculture research facility in Kearneysville, WV in flow through water at a rate of 1 L/min, at 12.5°C, until the challenge weight of ~1.3 g was met. The fish in this facility are checked yearly for multiple diseases including columnaris disease, and except for fish in the challenge room, they are certified disease-free. No signs of disease were observed prior to challenge and no indications of F. columnare or columnaris disease were observed in the uninfected control tanks or in the maintenance tanks at any time. Fish were moved to challenge aquaria 1 week prior to immersion challenge to acclimate to the elevated water temperature of 16°C.
Wild-type, mutant and complemented F. columnare strains were each used for immersion challenges. Frozen bacterial stocks were stored at -80°C in 75% TYES-2xMg broth and 25% glycerol. Bacterial cultures, for challenges, were grown as previously described (Evenhuis and LaFrentz, 2016; Conrad et al., 2022a). Briefly, cultures were incubated at 30°C with shaking at 150 rpm until OD540 of 0.5 to 0.6 was reached, at which point the cells were used for the challenge.
Challenges of fry were performed using triplicate 3 L tanks with restricted water flows (~200 mL/min) at 16°C. Each tank contained 40 fish of approximately 1.35 g each. Water flows were stopped for the immersion challenge and tanks were inoculated with bacterial cultures and incubated for 0.5 h after which water flows were resumed. Control tanks were inoculated with sterile TYES-2xMg broth. Serial dilutions of water samples from each tank after inoculation were plated on TYES-2xMg agar to determine CFU/mL. Mortalities were removed and counted daily. The data for triplicate tanks of each strain were pooled and survivor fractions for each strain were calculated. Challenges continued for 21 days or until 3 days without recorded mortalities post-exposure. Approximately 16% of mortalities were randomly tested by homogenizing gill tissue and streaking on TYES-2xMg agar plates to determine if F. columnare was present. Confirmation of F. columnare was determined by morphological observation of yellow, rhizoid, adherent colonies and by amplifying 16S rRNA genes and confirming the genomovar by enzymatic digestion (HaeIII) and gel electrophoresis as previously described (Triyanto and Wakabayashi, 1999; Olivares-Fuster et al., 2007; LaFrentz et al., 2014). F. columnare was detected in all mortalities tested and all were genomovar I and genetic group 1, as expected for strain MS-FC-4.
Challenges of rainbow trout alevin were performed as previously described (Evenhuis et al., 2021). In brief, 100 alevin were challenged 3 days post-hatch in 3-liter tanks. Total CFUs are given in the figures. Mortalities were removed daily and whole alevin were homogenized and streaked on TYES agar plates to determine the presence of F. columnare.
Genome sequences were analyzed for T9SS genes encoding proteins that belong to appropriate TIGRFAM multiple-sequence alignment families (Haft et al., 2013). The genomes were also examined for genes encoding predicted secreted proteins that have type A CTDs (TIGR04183) and type B CTDs (TIGR04131 and pfam13585) (Veith et al., 2017). In each case, the trusted cutoffs assigned by The J. Craig Venter Institute (JCVI) that allow identification of the vast majority of family members with vanishingly few false positives (Haft et al., 2013) were used. Some bioinformatic data were taken from (Thunes et al., 2022). Predicted F. columnare T9SS peptidases listed in Table 2 and Table S1 were identified by BLASTP analyses, and by using resources on the MEROPS website (https://www.ebi.ac.uk/merops/) (Rawlings et al., 2018) and the National Center for Biotechnology Information (NCBI) conserved domain searches (Marchler-Bauer et al., 2015; Marchler-Bauer et al., 2017).
A value of p < 0.05 was considered significant. For characterization assays a one-way ANOVA with Tukey’s post-test was used to analyze differences between treatment groups, unless otherwise noted. Error bars represent SEM (standard error of the mean), except for Figure 3, where they represent standard deviation. Kaplan-Meier survival analyses (Kaplan and Meier, 1958) were performed on fish challenge data. GraphPad Prism version 9.4.1 (GraphPad Software, LLC) was used to compute statistical tests.
The original contributions presented in the study are included in the article/Supplementary Material. Further inquiries can be directed to the corresponding author.
The animal study was reviewed and approved by the University of Wisconsin-Milwaukee Institutional Animal Care and Use Committee (adult zebrafish studies), the Institut Pasteur institutional Animal Health and Care Committees under permit # dap200043 (zebrafish larvae studies), and the US Department of Agriculture NCCCWA Institutional Animal Care and Use Committee (rainbow trout challenges, as described in Protocol #176).
NT and MM conceived and designed the study and performed the bioinformatic analyses. MM, JE, and J-MG obtained funding to support the research. NT and HM constructed all bacterial mutants and performed challenge experiments on adult zebrafish. RC constructed two plasmids used in mutant construction. D-PP, RS and J-MG performed and analyzed all experiments on zebrafish larvae. JE, RL, and CB performed all experiments involving rainbow trout. NT performed and analyzed growth experiments and protease assays, and analyzed adult zebrafish and rainbow trout challenge data. NT wrote the initial draft of the manuscript. NT, MM, HM, D-PP, RS, JE, J-MG and RL contributed to manuscript revision. All authors approved the submitted version.
This work was funded in part by United States Department of Agriculture-ARS CRIS projects 8082-32000-006-00-D and 5090-31320-004-00D and by cooperative agreements 5090-31320-004-03S and 58-5090-1-022, and by grant NA18OAR4170097, project R/SFA-20 from the University of Wisconsin Sea Grant Institute under grants from the National Sea Grant College Program, National Oceanic and Atmospheric Administration, U.S. Department of Commerce, and the State of Wisconsin. JMG, DPP and RS were funded by the French government’s Investissement d’Avenir Program, Laboratoire d’Excellence “Integrative Biology of Emerging Infectious Diseases” (grant ANR-10-LABX-62-IBEID) and by an Institut Carnot Pasteur MS fellowship. The views contained in this document are those of the authors and should not be interpreted as necessarily representing the official policies, either expressed or implied, of the U.S. Government. Mention of trade name, proprietary product, or specific equipment does not constitute a guarantee or warranty by the USDA and does not imply its approval to the exclusion of other products that may be suitable.
The authors declare that the research was conducted in the absence of any commercial or financial relationships that could be construed as a potential conflict of interest.
All claims expressed in this article are solely those of the authors and do not necessarily represent those of their affiliated organizations, or those of the publisher, the editors and the reviewers. Any product that may be evaluated in this article, or claim that may be made by its manufacturer, is not guaranteed or endorsed by the publisher.
The Supplementary Material for this article can be found online at: https://www.frontiersin.org/articles/10.3389/fcimb.2023.1093393/full#supplementary-material
Bartelme, R. P., Barbier, P., Lipscomb, R. S., LaPatra, S. E., Newton, R. J., Evenhuis, J. P., et al. (2018). Draft genome sequence of the fish pathogen Flavobacterium columnare strain MS-FC-4. Genome Announc 6 (20), e00429–e00418. doi: 10.1128/genomeA.00429-18
Benedyk, M., Marczyk, A., Chruscicka, B. (2019). Type IX secretion system is pivotal for expression of gingipain-associated virulence of Porphyromonas gingivalis. Mol. Oral. Microbiol. 34 (6), 237–244. doi: 10.1111/omi.12268
Bertani, G. (1951). Studies on lysogenesis i. the mode of phage liberation by lysogenic Escherichia coli. J. Bacteriol. 62, 293–300. doi: 10.1128/jb.62.3.293-300.1951
Bertolini, J. M., Rohovec, J. S. (1992). Electrophoretic detection of proteases from different Flexibacter columnaris strains and assessment of their variability. Dis. Aquat. Organisms 12, 121–128. doi: 10.3354/dao012121
Cain, K. D., LaFrentz, B. R. (2007). Laboratory maintenance of Flavobacterium psychrophilum and Flavobacterium columnare. Curr. Protoc. Microbiol. 6, 13B.11.11–13B.11.12. doi: 10.1002/9780471729259.mc13b01s6
Clarke, S. C., Dumesic, P. A., Homer, C. M., O'Donoghue, A. J., La Greca, F., Pallova, L., et al. (2016). Integrated activity and genetic profiling of secreted peptidases in Cryptococcus neoformans reveals an aspartyl peptidase required for low pH survival and virulence. PloS Pathog. 12 (12), e1006051. doi: 10.1371/journal.ppat.1006051
Chen, I. M. A., Chu, K., Palaniappan, K., Ratner, A., Huang, J. H., Huntemann, M., et al. (2021). The IMG/M data management and analysis system v.6.0: New tools and advanced capabilities. Nucleic Acids Res. 49 (D1), D751–D763. doi: 10.1093/nar/gkaa939
Conrad, R. A., Evenhuis, J. P., Lipscomb, R., Birkett, C., McBride, M. J. (2022a). Siderophores produced by the fish pathogen Flavobacterium columnare strain MS-FC-4 are not essential for its virulence. Appl. Environ. Microbiol. 88, e0094822. doi: 10.1128/aem.00948-22
Conrad, R. A., Evenhuis, J. P., Lipscomb, R. S., Pérez-Pascual, D., Stevick, R. J., Birkett, C., et al. (2022b). Flavobacterium columnare ferric iron uptake systems are required for virulence. Front. Cell. Infect. Microbiol. 12. doi: 10.3389/fcimb.2022.1029833
Declercq, A. M., Haesebrouck, F., Van den Broeck, W., Bossier, P., Decostere, A. (2013). Columnaris disease in fish: a review with emphasis on bacterium-host interactions. Vet. Res. 44, 27. doi: 10.1186/1297-9716-44-27
Decostere, A., Haesebrouck, F., Van Driessche, E., Charlier, G., Ducatelle, R. (1999). Characterization of the adhesion of Flavobacterium columnare (Flexibacter columnaris) to gill tissue. J. Fish Dis. 22 (6), 465–474. doi: 10.1046/J.1365-2761.1999.00198.X
Duchaud, E., Boussaha, M., Loux, V., Bernardet, J. F., Michel, C., Kerouault, B., et al. (2007). Complete genome sequence of the fish pathogen Flavobacterium psychrophilum. Nat. Biotechnol. 25, 763–769. doi: 10.1038/nbt1313
Dumpala, P. R., Gulsoy, N., Lawrence, M. L., Karsi, A. (2010). Proteomic analysis of the fish pathogen Flavobacterium columnare. Proteome Sci. 8, 26. doi: 10.1186/1477-5956-8-26
Evenhuis, J. P., LaFrentz, B. R. (2016). Virulence of Flavobacterium columnare genomovars in rainbow trout Oncorhynchus mykiss. Dis. Aquat Organ 120 (3), 217–224. doi: 10.3354/dao03027
Evenhuis, J. P., Lipscomb, R., Birkett, C. (2021). Virulence variations of Flavobacterium columnare in rainbow trout (Oncorhynchus mykiss) eyed eggs and alevin. J. Fish Dis. 44 (5), 533–539. doi: 10.1111/jfd.13343
Glew, M. D., Veith, P. D., Peng, B., Chen, Y. Y., Gorasia, D. G., Yang, Q., et al. (2012). PG0026 is the c-terminal signal peptidase of a novel secretion system of Porphyromonas gingivalis. J. Biol. Chem. 287, 24605–24617. doi: 10.1074/jbc.M112.369223
Gómez, E., Alvarez, B., Duchaud, E., Guijarro, J. A. (2015). Development of a markerless deletion system for the fish-pathogenic bacterium Flavobacterium psychrophilum. PloS One 10, e0117969. doi: 10.1371/journal.pone.0117969
Haft, D. H., Selengut, J. D., Richter, R. A., Harkins, D., Basu, M. K., Beck, E. (2013). TIGRFAMs and genome properties in 2013. Nucleic Acids Res. 41, D387–D395. doi: 10.1093/nar/gks1234
Hoge, R., Laschinski, M., Jaeger, K. E., Wilhelm, S., Rosenau, F. (2011). The subcellular localization of a c-terminal processing protease in Pseudomonas aeruginosa. FEMS Microbiol. Lett. 316 (1), 23–30. doi: 10.1111/j.1574-6968.2010.02181.x
Kaplan, E. L., Meier, P. (1958). Nonparametric estimation from incomplete observations. J. Amer. Statist. Assoc. 53, 457–481. doi: 10.2307/2281868
Klemba, M., Goldberg, D. E. (2002). Biological roles of proteases in parasitic protozoa. Annu. Rev. Biochem. 71, 275–305. doi: 10.1146/annurev.biochem.71.090501.145453
Kolar, S. L., Ibarra, J. A., Rivera, F. E., Mootz, J. M., Davenport, J. E., Stevens, S. M., et al. (2013). Extracellular proteases are key mediators of Staphylococcus aureus virulence via the global modulation of virulence-determinant stability. Microbiologyopen 2 (1), 18–34. doi: 10.1002/mbo3.55
Kulkarni, S. S., Zhu, Y., Brendel, C. J., McBride, M. J. (2017). Diverse c-terminal sequences involved in Flavobacterium johnsoniae protein secretion. J. Bacteriol. 199 (12), e00884–e00816. doi: 10.1128/JB.00884-16
LaFrentz, B. R., Waldbieser, G. C., Welch, T. J., Shoemaker, C. A. (2014). Intragenomic heterogeneity in the 16S rRNA genes of Flavobacterium columnare and standard protocol for genomovar assignment. J. Fish Dis. 37 (7), 657–669. doi: 10.1111/jfd.12166
Li, N., Qin, T., Zhang, X. L., Huang, B., Liu, Z. X., Xie, H. X., et al. (2015). Gene deletion strategy to examine the involvement of the two chondroitin lyases in Flavobacterium columnare virulence. Appl. Environ. Microbiol. 81 (21), 7394–7402. doi: 10.1128/AEM.01586-15
Li, N., Zhu, Y., LaFrentz, B. R., Evenhuis, J. P., Hunnicutt, D. W., Conrad, R. A., et al. (2017). The type IX secretion system is required for virulence of the fish pathogen Flavobacterium columnare. Appl. Environ. Microbiol. 83 (23), e01769–e01717. doi: 10.1128/AEM.01769-17
Lu, S. N., Wang, J. Y., Chitsaz, F., Derbyshire, M. K., Geer, R. C., Gonzales, N. R., et al. (2020). CDD/SPARCLE: the conserved domain database in 2020. Nucleic Acids Res. 48, D265–D268. doi: 10.1093/nar/gkz991
Marchler-Bauer, A., Bo, Y., Han, L. Y., He, J. E., Lanczycki, C. J., Lu, S. N., et al. (2017). CDD/SPARCLE: functional classification of proteins via subfamily domain architectures. Nucleic Acids Res. 45 (D1), D200–D203. doi: 10.1093/nar/gkw1129
Marchler-Bauer, A., Derbyshire, M. K., Gonzales, N. R., Lu, S. N., Chitsaz, F., Geer, L. Y., et al. (2015). CDD: NCBI's conserved domain database. Nucleic Acids Res. 43 (D1), D222–D226. doi: 10.1093/nar/gku1221
McBride, M. J. (2019). Bacteroidetes gliding motility and the type IX secretion system. Microbiol. Spectr. 7 (1), PSIB–0002-2018. doi: 10.1128/microbiolspec.PSIB-0002-2018
Mckerrow, J. H., Sun, E., Rosenthal, P. J., Bouvier, J. (1993). The proteases and pathogenicity of parasitic protozoa. Annu. Rev. Microbiol. 47, 821–853. doi: 10.1146/annurev.micro.47.1.821
Newton, J. C., Wood, T. M., Hartley, M. M. (1997). Isolation and partial characterization of extracellular proteases produced by isolates of Flavobacterium columnare derived from catfish. J. Aquat Anim. Health 9, 75–85. doi: 10.1577/1548-8667(1997)009<0075:IAPCOE>2.3.CO;2
Olivares-Fuster, O., Baker, J. L., Terhune, J. S., Shoemaker, C. A., Klesius, P. H., Arias, C. R. (2007). Host-specific association between Flavobacterium columnare genomovars and fish species. Systematic Appl. Microbiol. 30 (8), 624–633. doi: 10.1016/j.syapm.2007.07.003
Pérez-Pascual, D., Gómez, E., Alvarez, B., Méndez, J., Reimundo, P., Navais, R., et al. (2011). Comparative analysis and mutation effects of fpp2-fpp1 tandem genes encoding proteolytic extracellular enzymes of Flavobacterium psychrophilum. Microbiol 157, 1196–1204. doi: 10.1099/mic.0.046938-0
Pulkkinen, K., Suomalainen, L. R., Read, A. F., Ebert, D., Rintamäki, P., Valtonen, E. T. (2010). Intensive fish farming and the evolution of pathogen virulence: the case of columnaris disease in Finland. Proc. R. Soc B 277, 593–600. doi: 10.1098/rspb.2009.1659
Rawlings, N. D., Alan, J., Thomas, P. D., Huang, X. D., Bateman, A., Finn, R. D. (2018). The MEROPS database of proteolytic enzymes, their substrates and inhibitors in 2017 and a comparison with peptidases in the PANTHER database. Nucleic Acids Res. 46 (D1), D624–D632. doi: 10.1093/nar/gkx1134
Rochat, T., Perez-Pascual, D., Nilsen, H., Carpentier, M., Bridel, S., Bernardet, J. F., et al. (2019). Identification of a novel elastin-degrading enzyme from the fish pathogen Flavobacterium psychrophilum. Appl. Environ. Microbiol. 85, e02535–18. doi: 10.1128/AEM.02535-18
Ruiz-Perez, F., Nataro, J. P. (2014). Bacterial serine proteases secreted by the autotransporter pathway: classification, specificity, and role in virulence. Cell. Mol. Life Sci. 71 (5), 745–770. doi: 10.1007/s00018-013-1355-8
Saoud, J., Mani, T., Faucher, S. P. (2021). The tail-specific protease is important for Legionella pneumophila to survive thermal stress in water and inside amoebae. Appl. Environ. Microbiol. 87 (9), e02975–20. doi: 10.1128/AEM.02975-20
Sato, K., Naito, M., Yukitake, H., Hirakawa, H., Shoji, M., McBride, M. J., et al. (2010). A protein secretion system linked to bacteroidete gliding motility and pathogenesis. Proc. Natl. Acad. Sci. U.S.A. 107, 276–281. doi: 10.1073/pnas.0912010107
Secades, P., Alvarez, B., Guijarro, J. A. (2001). Purification and characterization of a psychrophilic, calcium-induced, growth-phase-dependent metalloprotease from the fish pathogen Flavobacterium psychrophilum. Appl. Environ. Microbiol. 67, 2436–2444. doi: 10.1128/AEM.67.6.2436-2444.2001
Secades, P., Alvarez, B., Guijarro, J. A. (2003). Purification and properties of a new psychrophilic metalloprotease (Fpp2) in the fish pathogen Flavobacterium psychrophilum. FEMS Microbiol. Lett. 226, 273–279. doi: 10.1016/S0378-1097(03)00599-8
Seers, C. A., Slakeski, N., Veith, P. D., Nikolof, T., Chen, Y. Y., Dashper, S. G., et al. (2006). The RgpB c-terminal domain has a role in attachment of RgpB to the outer membrane and belongs to a novel c-terminal-domain family found in Porphyromonas gingivalis. J. Bacteriol. 188 (17), 6376–6386. doi: 10.1128/Jb.00731-06
Shrivastava, A., Johnston, J. J., van Baaren, J. M., McBride, M. J. (2013). Flavobacterium johnsoniae GldK, GldL, GldM, and SprA are required for secretion of the cell surface gliding motility adhesins SprB and RemA. J. Bacteriol. 195 (14), 3201–3212. doi: 10.1128/JB.00333-13
Sommerfield, A. G., Darwin, A. J. (2022). Bacterial carboxyl-terminal processing proteases play critical roles in the cell envelope and beyond. J. Bacteriol. 204 (4). doi: 10.1128/jb.00628-21
Stressmann, F. A., Bernal-Bayard, J., Perez-Pascual, D., Audrain, B., Rendueles, O., Briolat, V., et al. (2021). Mining zebrafish microbiota reveals key community-level resistance against fish pathogen infection. ISME J. 15 (3), 702–719. doi: 10.1038/s41396-020-00807-8
Suomalainen, L. R., Tiirola, M. A., Valtonen, E. T. (2005). Influence of rearing conditions on Flavobacterium columnare infection of rainbow trout, Oncorhynchus mykiss (Walbaum). J. Fish Dis. 28, 271–277. doi: 10.1111/j.1365-2761.2005.00631.x
Tang, Y., Xin, G., Zhao, L. M., Huang, L. X., Qin, Y. X., Su, Y. Q., et al. (2020). Novel insights into host-pathogen interactions of large yellow croakers (Larimichthys crocea) and pathogenic bacterium Pseudomonas plecoglossicida using time-resolved dual RNA-seq of infected spleens. Zoological Res. 41 (3), 314–327. doi: 10.24272/j.issn.2095-8137.2020.035
Thunes, N. C., Conrad, R. A., Mohammed, H. H., Zhu, Y., Barbier, P., Evenhuis, J. P., et al. (2022). Type IX secretion system effectors and virulence of the model Flavobacterium columnare strain MS-FC-4. Appl. Environ. Microbiol. 88 (3), e0170521. doi: 10.1128/AEM.01705-21
Triyanto, H., Wakabayashi, H. (1999). Genotypic diversity of strains of Flavobacterium columnare from diseased fishes. Fish Pathol. 34, 65–71. doi: 10.3147/jsfp.34.65
Veith, P. D., Glew, M. D., Gorasia, D. G., Reynolds, E. C. (2017). Type IX secretion: the generation of bacterial cell surface coatings involved in virulence, gliding motility and the degradation of complex biopolymers. Mol. Microbiol. 106 (1), 35–53. doi: 10.1111/mmi.13752
Vincent, M. S., Canestrari, M. J., Leone, P., Stathopulos, J., Ize, B., Zoued, A., et al. (2017). Characterization of the Porphyromonas gingivalis type IX secretion trans-envelope PorKLMNP core complex. J. Biol. Chem. 292 (8), 3252–3261. doi: 10.1074/jbc.M116.765081
Wagner, B. A., Wise, D. J., Khoo, L. H., Terhune, J. S. (2006). The epidemiology of bacterial diseases in food-size channel catfish. J. Aquat. Anim. Health 18, 263–272. doi: 10.1577/1548-8667(2002)014<0263:TEOBDI>2.0.CO;2
Yang, Y., Wen, Y. J., Cai, Y. N., Vallee, I., Boireau, P., Liu, M. Y., et al. (2015). Serine proteases of parasitic helminths. Korean J. Parasitol. 53 (1), 1–11. doi: 10.3347/kjp.2015.53.1.1
Keywords: flavobacterium, fish pathogen, gene deletion, type IX secretion system (T9SS), protease
Citation: Thunes NC, Mohammed HH, Evenhuis JP, Lipscomb RS, Pérez-Pascual D, Stevick RJ, Birkett C, Conrad RA, Ghigo J-M and McBride MJ (2023) Secreted peptidases contribute to virulence of fish pathogen Flavobacterium columnare. Front. Cell. Infect. Microbiol. 13:1093393. doi: 10.3389/fcimb.2023.1093393
Received: 08 November 2022; Accepted: 18 January 2023;
Published: 03 February 2023.
Edited by:
Manuel L. Lemos, University of Santiago de Compostela, SpainReviewed by:
Mark L. Lawrence, Mississippi State University, United StatesCopyright © 2023 Thunes, Mohammed, Evenhuis, Lipscomb, Pérez-Pascual, Stevick, Birkett, Conrad, Ghigo and McBride. This is an open-access article distributed under the terms of the Creative Commons Attribution License (CC BY). The use, distribution or reproduction in other forums is permitted, provided the original author(s) and the copyright owner(s) are credited and that the original publication in this journal is cited, in accordance with accepted academic practice. No use, distribution or reproduction is permitted which does not comply with these terms.
*Correspondence: Mark J. McBride, bWNicmlkZUB1d20uZWR1
Disclaimer: All claims expressed in this article are solely those of the authors and do not necessarily represent those of their affiliated organizations, or those of the publisher, the editors and the reviewers. Any product that may be evaluated in this article or claim that may be made by its manufacturer is not guaranteed or endorsed by the publisher.
Research integrity at Frontiers
Learn more about the work of our research integrity team to safeguard the quality of each article we publish.