- 1State Key Laboratory of Silkworm Genome Biology, Southwest University, Chongqing, China
- 2The Ninth People's Hospital of Chongqing, Affiliated Hospital of Southwest University, Chongqing, China
- 3Cancer Center, Medical Research Institute, Southwest University, Chongqing, China
- 4Jinfeng Laboratory, Chongqing, China
Mycobacterium tuberculosis (Mtb), the causative agent of Tuberculosis (TB), remains a pathogen of great interest on a global scale. This airborne pathogen affects the lungs, where it interacts with macrophages. Acidic pH, oxidative and nitrosative stressors, and food restrictions make the macrophage’s internal milieu unfriendly to foreign bodies. Mtb subverts the host immune system and causes infection due to its genetic arsenal and secreted effector proteins. In vivo and in vitro research have examined Mtb-host macrophage interaction. This interaction is a crucial stage in Mtb infection because lung macrophages are the first immune cells Mtb encounters in the host. This review summarizes Mtb effectors that interact with macrophages. It also examines how macrophages control and eliminate Mtb and how Mtb manipulates macrophage defense mechanisms for its own survival. Understanding these mechanisms is crucial for TB prevention, diagnosis, and treatment.
1 Introduction
The host mucosal barrier is formed by macrophages, epithelial lining, inflammation responses, and released soluble substances (Graves and Milovanova, 2019). Macrophages are programmed to detect invading pathogens, activate microbicidal mechanisms, and coordinate the subsequent immune responses (Ravesloot-Chávez et al., 2021). Macrophages are located in every part of the body, and in the lungs, two main populations of macrophages have been identified, including alveolar macrophages (tissue-resident alveolar macrophages and monocyte-derived alveolar macrophages), and interstitial macrophages (Hou et al., 2021). The first group, on the inner surface of the lungs, accounts for 55% of lung immune cells (Hume et al., 2020) and suppresses intracellular pathogens, including Mtb (Hou et al., 2021).
Mtb is an obligate intracellular pathogen affecting about 10 million people each year among which 1.5 million lost their lives, and these statistics have been worsen with the new coronavirus disease (COVID-19) pandemic that has reversed gains and set back the fight against TB by several years (Global Tuberculosis Report, 2021). This airborne pathogen enters the lungs through the respiratory tract and firstly attacks aveolar macrophages. This Mtb-host macrophage interaction is a critical step for Mtb to successfully establish the infection. Mtb infection process can be randomly resumed as follows: 1) Mtb attachment by pathogen-associated molecular patterns (PAMPs); 2) Mtb identification by host macrophage pattern recognition receptors (PRRs) (Table 1); and 3) macrophage stimulation and activation of intracellular cascades and signaling pathways (BoseDasgupta and Pieters, 2018; Yassine et al., 2021). Mtb's intracellular interaction with macrophages is complex and challenging because of the hostile intracellular macrophage milieu (ROS and RNS production, low pH, food depletion, etc.) (Weiss and Schaible, 2015). Despite macrophages' impassable barrier, Mtb can evade host macrophage defenses via evolved escaping strategies to successfully create infection (Flentie et al., 2016; Chandra et al., 2022). This escape ability affects TB control, including low vaccine efficacy, multi- and extensively-drug resistance (MDR-XDR), extended therapy, and high TB incidence.
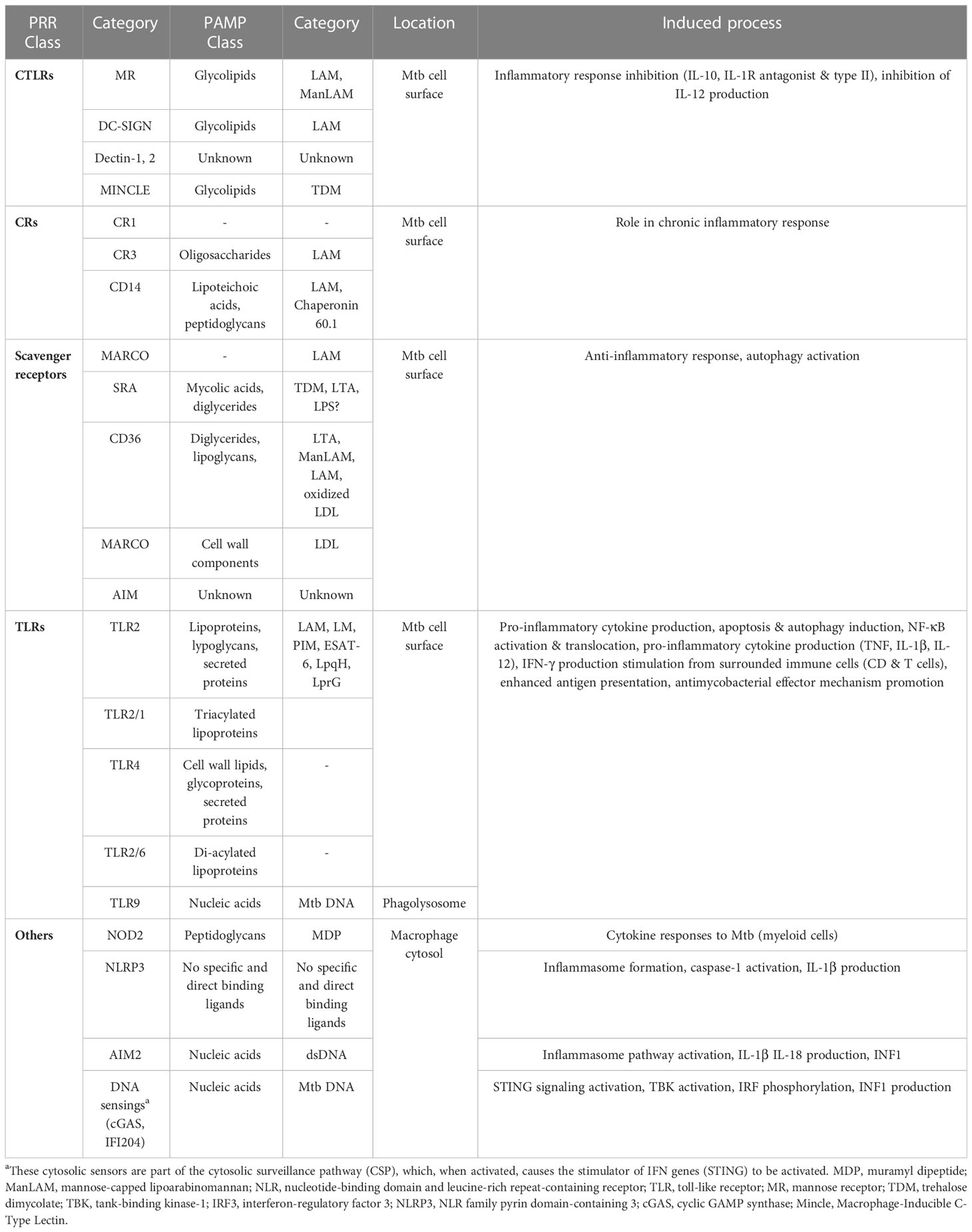
Table 1 Interaction between Mtb PAMPs and macrophage PRRs during recognition (Stamm et al., 2015; Ravesloot-Chávez et al., 2021; Tsolaki et al., 2021).
Understanding Mtb and host macrophage coevolution will help treat and control TB. Despite significant research on diverse aspects of the Mtb-macrophage crosstalk, this subject is not entirely understood. Is it because Mtb's genetic arsenal enables it to circonstancially adapt to and survive within macrophage hostile environment? (Flentie et al., 2016). We discuss Mtb's major determinants and emerging molecular processes characterizing several stages of this interaction to elucidate essential mechanisms and reveal potential molecular targets.
2 Mtb effectors interacting with macrophages
Protein and lipid effectors produced by Mtb regulate the functions of macrophages and the inflammatory process (Chandra et al., 2022). The coding information of those effectors are embeded in the Mtb genome (4000 genes) (TB Database, http://tbdb.bu.edu/tbdb_sysbio/GenomesIndex.html). The mycobacterial cell envelope is a complex architectural structure consisting of a typical plasma membrane, a layer of peptidoglycans covalently attached to polysaccharides (arabinogalactans), which have their penta-arabinosyl ends esterified by mycolic acids (Brennan and Nikaido, 1995). Lipoproteins, peptidoglycans, trehalose mono- and di-mycolates, phosphatidylinositol mannosides, lipomannan, and lipoarabinomannan are all components of the Mtb cell wall that are exposed to extracellular environments (Stamm et al., 2015; Madacki et al., 2019). Other factors, such as regulatory genes (DosR, WhiB, PhoP), adenylyl cyclase protein kinases (pknG), and enzymes (Mce family proteins, for example), do not fit into the surface-exposed group (miscellaneous factors) (Madacki et al., 2019). The virulence factors mentioned above play a critical role in host-pathogen interactions, with reports stating their involvement in host-cell recognition (Vergne et al., 2014) and phagosome maturation arrest (Chatterjee et al., 1992; Fratti et al., 2003). Researchers have recently uncovered new information regarding the cell membrane's secretion mechanisms and complex mycobacterial lipids (Madacki et al., 2019). SapM and PknG are exported by the SecA2 secretion system and interfere with the acidification and maturation of the phagosome-containing Mtb (Zulauf et al., 2018). Other export routes involve the sec secretory pathway, the twin-arginine translocation (TAT) pathway, and the ESX/type VII. TAT secretion system-carried mycolyl transferases catalyze the formation of TDM by attaching mycolate residues to arabinogalactan (Belisle et al., 1997). Proteins in the PE/PPE family, so-called for the N-terminal Pro-Glu and Pro-Pro-Glu motifs they share, are released via the ESX-5 secretion system and may contribute to Mtb pathogenicity. Reduced PPE protein secretion, decreased cell-wall integrity, and severe attenuation, were seen in Mtb strains lacking ESX-5 (Bottai et al., 2012). However, further research is needed to reveal the underlying virulence components of the Mtb cell wall.
3 Mtb-macrophage interaction
3.1 Early Mtb-macrophage interaction
Blocking Mtb-macrophage contact and entrance into human cells can prevent TB. Mtb is an airborne pathogen that spreads from sick to healthy people. When the latter inhale Mtb-containing droplet nuclei, they reach the lungs' alveoli. Indirect (opsonization) and direct Mtb recognitions by macrophages have been reported. Indirect Mtb detection uses soluble factors (collectins, complement systems, etc.) that chemically modifiy Mtb and facilitate its internalization inside the macrophage. Recruitment of host cell molecules to Mtb cell surface has been demonstrated as well. In contrast, direct Mtb detection uses non-soluble factors that identify Mtb ligands named PAMPs.
Specific macrophage PRRs identify Mtb PAMPs on the cell surface or in the intracellular macrophage environment (phagolysosome and cytosol). Mincle and Macro receptors interact with TDM on the Mtb surface, whereas PRRs such as MR, DC-SIGN, and Dectin-2 recognize Mtb glycolipids (ManLAM). Besides, NOD2 in the cytosol detects MDP released by Mtb peptidoglycans. The TLR9 detects phagolysosomal Mtb DNA, while the ESX-1 secretion system breaks the phagosomal membrane, thus allowing the cytosolic recognition of Mtb DNA and subsequent cGAS/STING induction. Other cascade reactions activated downstream, such as phagosome biogenesis, endosomal trafficking, autophagy, or secretion of soluble factors can be benefic or detrimental for the pathogen or the macrophage (Stamm et al., 2015). For instance, indentification of the pathogenic Mtb DNA by TLR9 increased M1 macrophage-derived human monocyte antimicrobial mechanisms through phenotypic alterations, excessive TNF-α production, and autophagy activation (Ruiz et al., 2019). PRRs-activated immune signals such as PI3K, IRGM, and mTOR inhibition can also induce autophagy after Mtb infection (Deretic, 2012; Pareja and Colombo, 2013; Ruiz et al., 2019). However, more research is needed into how macrophages recognize Mtb, especially its nucleic acids.
3.2 Activation and polarization of macrophages
Classically activated macrophages (M1) or th1-dependent responses and alternatively activated macrophages (M2) or th2-dependent responses are two basic polarized macrophage subgroups (Gordon, 2003). M1 macrophages have metabolic reprogramming associated to Warburg effect, high NO output, high antigen presentation, and pro-inflammatory cytokine production (IL-1, IL-6, IL-12, IL-23, and CXCL9), allowing them to establish a powerful immune response against intracellular pathogens. M2 macrophages, in contrast, fight germs less effectively and repair wounds. Different stimulatory factors polarize the M1 and M2 phenotypes. TLR agonists, cytokines (IFN-γ, TNF-α, GMCSF), or chemokines can all stimulate M1 macrophages. Anti-inflammatory cytokines (IL-4, IL-10, and IL-13), glucocorticoids, immune complexes (IC), and LPS induce M2 macrophages (Wang et al., 2019).
Macrophage polarization is critical for Mtb innate immunity, and Mtb effectors are engaged in that process. For instance, the virulence-associated antigen, ESAT-6, induces the M1 phenotype in early Mtb infection and later causes the transition to M2 (Refai et al., 2018). Mtb-secreted antigens (PPE36, EspC, or Rv1987), heat-shock proteins (Hsp16.3), or genes like Rv2882c have been demonstrated to polarize macrophages in vitro or in mouse models, via the activation of crucial intracellular processes and the generation of soluble factors (Blumenthal et al., 2009; Choi et al., 2016; Guo et al., 2019; Gong et al., 2020; Zhang et al., 2020; Sha et al., 2021). However, how Mtb interferes with macrophage polarization is not holistically understood. Table 2 summarizes recent updates of macrophage activation/polarization during Mtb infection.
4 Activation of macrophage innate defenses
Critical molecular mechanisms allow host macrophages to take advantage of the crosstalk, as indicated in the sections below and in Figure 1.
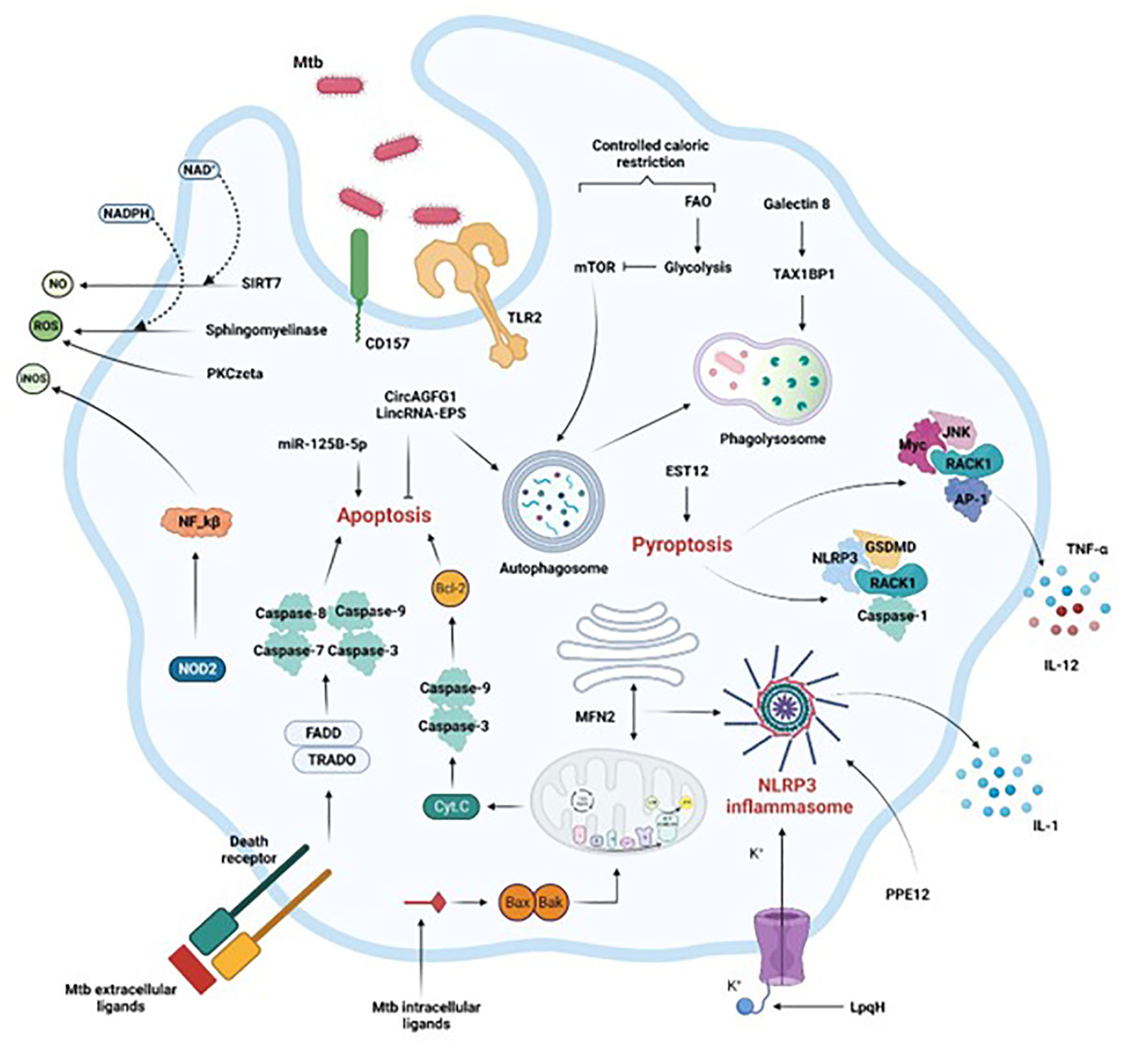
Figure 1 Activation of macrophage immune mechanisms to control Mtb infection. Crucial intracellular processes, such as autophagy, apoptosis, pyroptosis, and inflammation associated with the secretion of antimicrobial compounds (NO, iNOS, ROS) are induced following Mtb infection. Extrinsic or intrinsic activation of apoptosis by Mtb intracellular or extracellular effectors respectively leads to Mtb control. Mtb control is also achieved by efficient phagosome maturation and fusion to the lysosome (phagolysosome), coupled with reduced availability of calories characterized by the shift from FAO to glycolysis. Finally, the secretion of soluble factors through inflammasome and pyroptosis activation, or the production of ROS or RNS via the NF-kB pathway or through sphingomyelinases restrict the pathogen progression in the macrophage as well. FAO, fatty acid oxidation, Casp, caspase, ER, endoplasmic reticulum. Section 4 provides more details.
4.1 Cell death activation
4.1.1 Autophagy
Autophagy is an essential homeostatic process that is triggered by cellular stimuli (such as nutrient starvation) and involves nutrient regeneration, protein and organelle degradation, as well as the clearance of intracellular pathogens. Autophagic induction in macrophages is an effective mechanism to enhance intracellular killing of Mtb (Songane et al., 2012), and polymorphisms in autophagy-related genes (ATGs), especially the autophagy gene IRGM, are associated with susceptibility to TB (Intemann et al., 2009; Lu et al., 2016).
Antibacterial autophagy recruits proteins that deliver intracellular bacteria to the lysosome for degradation by ubiquitination. Sirtuin 3 (SIRT3) maintains respiratory functions. SIRT3 promotes anti-mycobacterial defenses by coordinating mitochondrial and autophagic functions (Kim et al., 2019).
Autophagy and immunometabolism drive anti-TB immunity through balanced AMPK-mTOR activation (Qu et al., 2020). This axis regulates the transcription factor TFEB, which is involved in autophagy and lysosomal biogenesis (Paik and Jo, 2020). We know little about TFEB's antimicrobial effects on autophagy and immunometabolism.
Galectins recognize Mtb-containing phagosomes and promote anti-Mtb autophagy. Galectin-8 recently interacted with the selective autophagy adapter TAX1BP1 (Deretic, 2012). BCL2-associated athanogene 2 (BAG2) reduced ER stress-induced cell apoptosis in Mtb-infected macrophages via autophagic flux and selective autophagy, revealing a potential host defense mechanism linking BAG2 to ER stress and autophagy during Mtb infection (Liang et al., 2020). The autophagy triggered by BAG2 required the dissociation of BECN1 and Bcl2 via MAPK/ERK (Liang et al., 2020).
4.1.2 Apoptosis
Apoptosis is a physiological type of cell death induced by mitochondrial-mediated (intrinsically) or death receptor (extrinsically) pathways (Behar et al., 2011). Macrophage apoptosis can eliminate Mtb growth by direct antimicrobial effects or encapsulation in apoptotic bodies, which recruits new macrophages and dendritic cells (Lee et al., 2009). Activation of the Bcl-2 protein family, Bak- or Bax-mediated mitochondrial membrane damage and cytochrome C release, death receptors (Fas and TNFR), caspase activation, apoptotic body formation, efferocytosis (recognition and engulfment of apoptotic bodies by professional phagocytes via several cell-surface receptors), and anti-inflammatory cytokines (TGF, IL-10), can subvert intrinsic and extrinsic apoptosis to control infection to a lesser extent (Section 2). As a result, the idea of using host-directed therapies (HDTs) like inhibitors of apoptosis (IAP) proteins to manage TB infection has gained attention (Arnett and Schlesinger, 2021; Stutz et al., 2021).
4.1.3 Pyroptosis
Pro-inflammatory cytokines are secreted during pyroptosis, another newly activated death process. This death process is important in inflammatory-related respiratory diseases like TB (Feng et al., 2022). Activating pyroptosis after Mtb infection may promote macrophage elimination of Mtb. Pyroptosis activation also involves the gasdermin (GSDM) family and several canonical and non-canonical inflammasome-induced pathways (caspases-1/3/6/7/GSDMB, caspase-8/GSDMC, caspase-8/GSDMD, and caspase-3/GSEME). Recently, Mtb effector EST12-induced pyroptosis activated the RACK1/NLRP3/caspase-1/GSDMD or RACK1-JNK-AP1-Myc signaling pathways to enhance the anti-mycobacterial inflammatory response (Qu et al., 2020; Wu et al., 2022). However, the precise molecular mechanisms that cause pyroptosis during TB remain enigmatic.
4.2 Cytotoxic molecule secretion
Macrophages produce bactericidal chemicals in response to Mtb invasion via a variety of ways. In macrophages, expression of the cofactor-dependent enzymatic activity mediates protective immunological responses via ROS and RNS. The production of ROS by acid sphingomyelinases via the NADPH oxidase and cathepsins enhanced infection control and BCG breakdown in macrophages, (Wu et al., 2020). Sirtuin (SIRT7) is a deacetylase that is activated by nicotinamide adenine dinucleotide (NAD+). Its expression in macrophages diminishes after Mtb infection. A recent study explained how the SIRT7-mediated protective mechanism leads to Mtb clearance in macrophages through NO generation and apoptosis modulation (Zhang et al., 2021). By producing ROS and NO, modified macrophage receptors produces a protective immune response. Recently, TLR2-PKCzeta-induced ROS generation via the CD157 receptor provided host macrophage tolerance to Mtb (Yang et al., 2019). Furthermore, following Mtb invasion, iNOS expression and NO generation in human macrophages were dependent on NOD2 expression and needed NF-κB activation (Landes et al., 2015). After Mtb infection of human macrophages, NOD2 activation increases iNOS synthesis and activity, indicating a novel molecular pathway.
4.3 Non-coding RNA expression
MicroRNAs (miRNAs) and long non-coding RNAs (lncRNAs) cannot encode proteins but are involved in gene regulation and Mtb infection. These entities regulate macrophage apoptosis and autophagy in active TB infection to target key host proteins for pathogen control and clearance. The circular RNA CircAGFG1 boosted autophagy and decreased apoptosis in active TB (Shi et al., 2020). Silencing miR-125b-5p could protect human macrophages from Mtb infection by inducing apoptosis and decreasing inflammation (Liu et al., 2020). This points to a specific area to focus on in the fight against Mtb.
Although the molecular link connecting lncRNAs and macrophages in TB is still obscure, lncRNA PCED1B-AS1 and lncRNA MIAT influence macrophage apoptosis and autophagy in active TB (Li et al., 2019; Jiang et al., 2021). In BCG-infected RAW264.7 macrophages, knockdown of lincRNA-EPS reduced apoptosis and increased autophagy (Ke et al., 2020). The mechanism of action of ncRNAs may offer fresh TB targets. However, these mechanisms are far to be exhaustive because novel host ncRNAs are continually discovered.
4.4 Inflammasome activation
The inflammasome is a multiprotein complex that includes the members of the nucleotide-binding domain and leucine-rich repeat (LRR)-containing (NLR) family and the pyrin and HIN domain (PYHIN) family. The inflammasome is important for immunity, human disease, and TB resistance. Host or pathogen components can activate the NLRP3 inflammasome (He et al., 2016). Mtb PPE13 increased IL-1β secretion via the NLRP3 inflammasome (Yang et al., 2020). Mtb lipoprotein LpqH has high immunogenicity and can activate the NLRP3 inflammasome through the potassium efflux route (Liu et al., 2021).
Mitochondria are essential for NLRP3 inflammasome activation as well. This importance depends on mitofusin 2 (MFN2), which participates in the creation of mitochondria-associated endoplasmic reticulum membranes (MAMs) and may be the platform for NLRP3 inflammasome production during Mtb infection. Mtb infection upregulated MFN2 expression to enhance NLRP3 inflammasome formation (Xu et al., 2020). IL-1 is a key cytokine in the immune response against TB (Silvério et al., 2021). However, there is still curiosity about how Mtb actually induces inflammation. Nevertheless, the capacity of Mtb clinical isolates to activate inflammasome and IL-1 varies. Beijing isolates, for example, showed varying effects on IL-1 and caspase-1 activation, but all clinical isolates caused lesser IL-1 release than H37Rv, indicating the involvement of NLRP3, AIM2, and an additional unknown sensor in IL-1 maturation (Subbarao et al., 2020).
4.5 Caloric restriction
Changes in immunometabolism are triggered by Mtb infection. Effector cell functions are susceptible to changes in the host’s nutritional status. Immune responses to infections are expensive in terms of energy expenditure, metabolic change, and food intake (Van den Bossche et al., 2017). In susceptible DBA/2 mice, pulmonary Mtb infection is mitigated by controlled caloric restriction (CR), which does not result in malnutrition. Mechanism-wise, CR caused immune cells to switch their metabolism from fatty acid oxidation (FAO) to glycolysis and reduced the mTOR activity associated with autophagy activation (Palma et al., 2021). CR is not only an unanticipated method of improving immunity to Mtb, but it may also provide a novel method of treating Mtb infection in places where TB is quickly expanding alongside overnutrition and obesity. This approach, however, has only been studied in mouse models. Nonetheless, more research is needed to determine whether or not it is applicable to human TB.
5 Manipulating macrophage immunity
Mtb can avoid, neutralize, or exploit macrophage contents for its intracellular survival. The following sections and Figure 2 describe recent and previously unknown escape strategies.
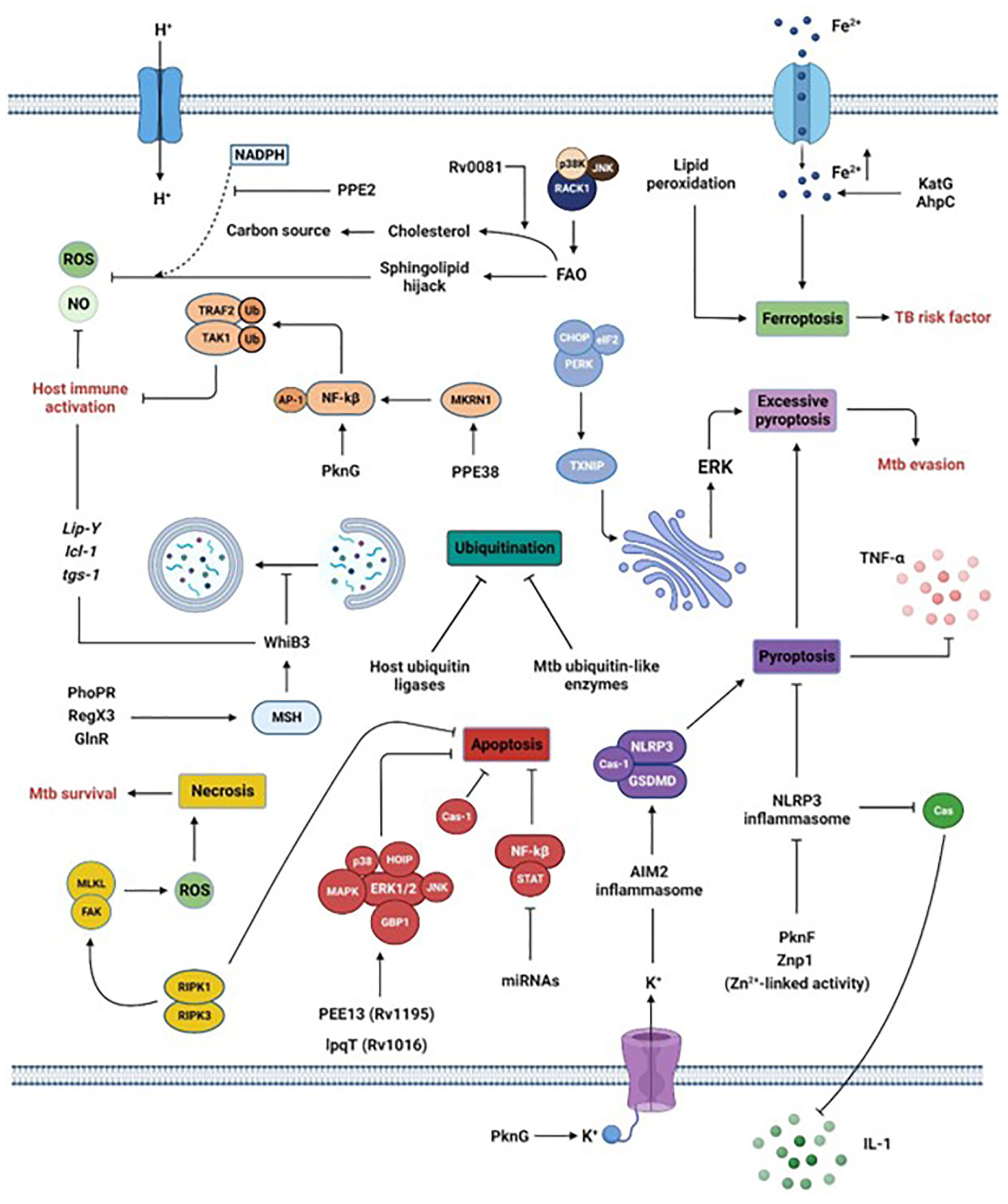
Figure 2 Manipulation of macrophage intracellular processes by Mtb. Via the secretion of its virulence factors, Mtb subverts crucial host processes. Mtb inhibits phagosome maturation via the MSH pathway, inhibits ubiquitination by acting as host ubiquitin-like enzymes or interfering with host ubiquitin ligases, reduces the secretion of pro-inflammatory cytokines by blocking inflammasome and pyroptosis, limits the generation of ROS or NO by interfering with sphingolipids or via the activation of the transcription factor WhiB3 or the lipid-associated gene cluster (Lip-Y, Icl-1, and tgs-1). Moreover, the promotion of ferroptosis via increased Fe2+ concentration or lipid peroxidation is a risk factor for individuals infected by Mtb. Finally, Mtb survival is also reached by inhibiting apoptosis while promoting necrosis. Section 5 provides more details.
5.1 Using cell deaths
Mtb hijacks host ubiquitination, autophagy, apoptosis, necrosis, pyroptosis, and ferroptosis.
5.1.1 Ubiquitination
Mtb uses its effectors as ubiquitin-like enzymes or interacts with host ubiquitin ligases to reduce host ubiquitination. The protein kinase G (PknG) is a unique ubiquitin-activating enzyme (E1) and ubiquitin ligase (E3) that initiates ubiquitination and degradation of TRAF2 and TAK1 to limit host immunological activation (Wang et al., 2021). The PknG-host interaction's mechanisms are unclear. PPE38, which is encoded by the region of difference 1 (RD1), interacts with the macrophage ubiquitin ligase (E3), Makorin Ring Finger Protein 1 (MKRN1), suppressing TRAF6-driven NF-kB and AP-1 signaling, cytokines (TNF-α, IL-6), and NO production (Dou et al., 2022). This inhibition of host immunity was also reported following lysine-11-linked ubiquitination of Rv0222 by the host E3 ubiquitin ligase ANAPC2 involving SHP1 and TRAF6, or deubiquitination of TRAF6 by HAUSP after PE_PGRS38 ectopic expression in M. smegmatis in murine bone marrow-derived macrophages (Wang et al., 2020; Kim et al., 2022). The processes described here are previously unknown strategies used by Mtb to decrease host immunity.
5.1.2 Autophagy
Mtb promotes intracellular survival by inhibiting macrophage autophagy. Many Mtb PE/PPE proteins suppress autophagy in Mtb-infected macrophages, either canonically or non-canonically (Strong et al., 2020). The enhanced intracellular survival (Eis) protein is the first Mtb effector shown to limit autophagy via IL-10 up-regulation and histone H3 acetylation (Shin et al., 2010; Duan et al., 2016). The strain-specific behavior of Mtb in interrupting the autophagy pathway is the blockade of autophagolysomal fusion (Ebrahimifard et al., 2022). Other key molecular mechanisms include classical Rab1A inhibition, suppression of TLR2- and MAPK-activated host functions, and decreased ERK1/2 activation (Strong et al., 2021; Strong et al., 2022). These findings demonstrate mycobacterial effectors directly interact with autophagy-initiating host proteins. PknG either dually-regulates autophagy, promotes autophagy induction by competitively binding to AKT's pleckstrin homology (PH) domain, or inhibits autophagosome maturation to restrict autophagy flux by targeting the host small GTPase RAB14 (Ge et al., 2022). Other unknown escaping strategies include induction of histone hypermethylation in ATGs (Sengupta et al., 2021), direct autophagy inhibition by RELL1 (Feng et al., 2020), miRNA inhibition of autophagy by targeting critical AGTs (ULK1, ATG7, ATG16L1, ATG4c, and NPC1) located on the lysosomal membrane during Mtb infection (Liu et al., 2020; Qu et al., 2021; Dong et al., 2022), and the implication of Mtb's sulfoglycolipids (SLs) and DIMs (Bah et al., 2020). While induction of host cell autophagy by starvation (starvation-induced autophagic elimination) is reported to kill the Mtb reference strain H37Rv via enhanced lysosomal delivery to mycobacterial phagosomes, its isolate Mtb Beijing strain, instead, easily resists and subdues this host blockade by exceptional upregulation of both Kxd1 and Plekhm2 genes’ expression (Laopanupong et al., 2021). KatG depletion using the CRISPR-dCas9 interference system in the Beijing isolate strain resulted in increased lysosomal delivery to its phagosome and decreased its survival upon autophagy induction by starvation (Siregar et al., 2022). This suggests the importance of KatG, Kxd1, and Plekhm2 in Mtb isolates to evade starvation-induced autophagic restriction and investigating their role in the Mtb H37Rv strain may provide interesting clues (Laopanupong et al., 2021; Siregar et al., 2022). Besides, Mtb determinants involved in this process are still widely unknown. To sum up, the mechanisms employed by Mtb to inhibit autophagy need to be understood to facilitate the design of new therapeutics or vaccines against TB.
5.1.3 Apoptosis
Several Mtb pathways exploit macrophage apoptosis. Mtb thwarts or causes apoptosis by its effector proteins, regulation of host ncRNAs, and anti-apoptotic cytokine production (IL-10, IL-17A). Effector proteins of cell wall-associated glycolipids, secretion systems, serine/threonine protein kinases, heat shock, stress responses, and virulence inhibit apoptosis via important signaling axes (serine protease cathepsin G, GBP1, ERK1/2 signaling, IL-12p40/IL-32, IL-1/IL-6/TNF-, ROS/c-JNK, LUBAC HOIP-NF-kB, JNK/p38 MAPK) (Jayakumar et al., 2008; Danelishvili et al., 2011; Dutta et al., 2012; Halder et al., 2015; Deng et al., 2016; Wang et al., 2016; Joseph et al., 2017; Wang et al., 2017; Yang et al., 2017; Zhang et al., 2018; Long et al., 2019; Abdalla et al., 2020; Ali et al., 2020; Paik and Jo, 2020; Asaad et al., 2021). Mtb effectors such as Rv1016c (LpqH) and PE13 (Rv1195) enhance the TLR-dependent macrophage apoptosis (early infection stage) and p38/ERK/NF-kB-dependent apoptosis (late infection stage) (Aguilo et al., 2013; Li et al., 2016). Mtb determinants reduce or suppress caspases, preventing macrophage apoptosis. They also reduce anti-apoptotic IL-10 and IL-17A (Ma et al., 2019; Zhang et al., 2019; Fu et al., 2020). Mtb uses host lncRNAs less frequently than miRNAs to control apoptosis. This exploitation may include NF-κB/STAT activattion (Li et al., 2020). Further study is needed to determine how Mtb suppresses apoptosis. Collectively, the aforesaid considerations support Mtb's manipulation of host apoptosis and create a possibility for host-directed therapy targeting apoptosis for TB control.
5.1.4 Necrosis
In order to promote infection development, Mtb infection inhibits apoptosis and induces necrosis by producing ROS (Zhang et al., 2005). The receptor-interacting protein kinase 3 (RIPK3) triggers numerous distinct pathways that prevent apoptosis and enhance necrosis instead, via ROS generation, and contribute to Mtb survival in macrophages infected with Mtb (Zhao et al., 2017). To trigger necrotic cell death, Mtb used focal adhesion kinase (FAK) in a time-dependent manner, first by exploiting RIPK1 and then, to a lesser extent, by using RIPK3-MLKL, to produce ROS (Afriyie-Asante et al., 2021).
5.1.5 Pyroptosis
Additionally, Mtb escape host clearance involves pyroptosis control. The processes rely on the NLRP3/caspase-1/GSDMD axis, potassium efflux linked with Mtb PnkF expression and ROS suppression, and Mtb phagosomal inhibition of AIM2 inflammasome activation (Feng et al., 2022). Still elusive, these mechanisms may need more study. Mtb-induced excessive pyroptosis also aids in the spread of Mtb (Beckwith et al., 2020; Fu et al., 2021; Li et al., 2022). This process relies on the NLRP3/caspase-1/ GSDMD axis connected with the potassium efflux, but also strongly on the ERS induction linked with TXNIP downstream upregulation and the PERK/eIF2/CHOP axis (Gong et al., 2019; Beckwith et al., 2020; Li et al., 2022). Inhibiting pyroptosis in Mtb-infected macrophages through the PERK/eIF2/TXNIP/NLRP3/caspase-1/GSDMD axis reduces lung tissue damage and Mtb dissemination (Fu et al., 2021; Li et al., 2022).
5.1.6 Ferroptosis
Ferroptosis is a new type of controlled cell death involving Fe2+ accumulation and lipid peroxidation. Glutathione peroxidase-4 (Gpx4) is an enzyme that plays a critical role in preventing iron-dependent lipid peroxidation-mediated cell death (ferroptosis), a process previously implicated in the necrotic pathology seen in Mtb-infected mice. Gpx4 is a crucial intracellular lipid peroxidation-detoxifying enzyme (Yant et al., 2003), and its inactivation not only participates in ferroptosis but also remains the main mechanism supporting this process (Krantz et al., 2005; Dixon et al., 2012; Yang et al., 2014). GPX4 and glutathione levels are reduced in active TB individuals, whereas those of free iron, mitochondrial superoxide, and lipid peroxidation are increased (Amaral et al., 2019; Amaral et al., 2022), suggesting a role of ferroptosis in Mtb infection. Besides, perturbed Fe2+ homeostasis is a TB risk factor and may serve as a TB diagnostic marker (Reddy et al., 2018; Dai et al., 2019; Meunier and Neyrolles, 2019). Ferritin deficiency-induced Fe2+ overload consequently promoted ROS-dependent lipid peroxidation, Mtb growth and dissemination, host death via accumulated lipid peroxidation, and ferroptosis of macrophages in Mtb-infected mice (Dow et al., 2021; Rastogi et al., 2021). Hence, ferroptosis seems to be closely related to pulmonary TB development and represents a potential target for pulmonary TB treatment. However, additional research is needed to elucidate the molecular mechanisms and signaling pathways underlying the connection between Mtb and ferroptosis.
5.2 Simultaneous manipulation of host cell deaths
Mtb successfully manipulates host immune responses by simultaneously activating more than one cell death mechanisms (aforementioned cell killing pathways). In vitro, human monocyte-derived macrophages (MDM)-infected virulent Mtb inhibits the apoptosis mediated by BCL-2 family molecules but, at the same time, increases the expression of molecules involved in apoptosis (BCL-2, BAX , and phosphorylated BCL-2), necroptosis (ASK1, p-38, RIPK1, RIPK3, and CASP8), and pyroptosis (NLRP3, CASP1, and IL-1β secretion) at the transcriptional and protein levels (Ramon-Luing et al., 2022). During the selective elimination of invading pathogens (xenophagy), autophagic receptor proteins (SQSTM1/p62, CALCOCO2/NDP52, and optineurin) are required, and Mtb co-opts ubiquitin effectors such as PtpA (Deretic et al., 2013; Wang et al., 2015; Chai et al., 2020). PE_PGRS41 reduces apoptosis and autophagy while boosting macrophage necrosis (Deng et al., 2017). Ferroptosis may contribute to Mtb-induced necrosis (Amaral et al., 2019). These data demonstrate Mtb's ability to control many macrophage pathways for survival.
5.3 Hypoxia, acidic pH, and harmful molecule neutralization
In macrophages, Mtb faces low pH, ROS, and RNS molecules. Mtb stress resistance is regulated by the cytosolic redox-sensing transcriptional regulator WhiB3. WhiB3 interacts with host gases and metabolic signals to maintain redox equilibrium (Saini et al., 2012). In addition, genes involved in lipid metabolism (lip-Y, Icl-1, and tgs-1) are also induced (Barrientos et al., 2022). Inhibition of the mycothiol (MSH) pathway inhibits WhiB3 activation (Mehta et al., 2016). Mtb's activation of WhiB3 in response to macrophage stressors is regulated by PhoPR, RegX3, and GlnR (Feng et al., 2018; You et al., 2019; Mahatha et al., 2020). WhiB3 activation causes redox homeostasis, down-regulation of innate immune genes, phagosomal maturation blockage, defective lysosomal trafficking, lipid anabolism regulation, virulence, and survival (Saini et al., 2012; Mehta et al., 2016; Feng et al., 2018; Mehta and Singh, 2019; You et al., 2019; Mahatha et al., 2020). However, how Mtb maintains redox balance and resistance in response to macrophage stressors is unknown. Mtb PPE2 inhibits NADPH-oxidase-mediated ROS generation (Srivastava et al., 2019). The nucleoid-associated protein M (NapM) binds to DnaA to increase Mtb's survival under stress and in macrophages (Liu et al., 2019). However, the latter is a previously undiscovered mycobacterial stress survival mechanism.
5.4 Granuloma nutrient utilization
Lipid metabolism affects host-pathogen interactions during Mtb infection (Davis and Ramakrishnan, 2009). Human TB develops a caseous granuloma, which implies pathogen-mediated disruption of host lipid metabolism (Kim et al., 2010). Immunohistological and biochemical investigations characterized the caseum protein and lipid species (Dawa et al., 2021). When FAO is inhibited in mouse macrophages, intracellular Mtb cannot develop (Chandra et al., 2020). Mtb can also hijack host sphingolipid balance or imitate sphingolipid enzymatic activity, which involves p38K- and JNK-dependent signaling cascades as well as surface 1-integrin and Rac1 activation (Speer et al., 2015; Li et al., 2016; Wu et al., 2018; Rolando and Buchrieser, 2019). Rv0081 promotes the use of cholesterol as the only carbon source in the granuloma (Dubey et al., 2021; Lata et al., 2022). In necroptotic granulomas, Fe2+ deficiency is a limiting Mtb growth factor. To avoid this difficult scenario, Mtb carefully controls the endogenous Fe2+ use by boosting the production of DNA repair and antioxidant activity-related proteins (KatG and AhpC) (Kurthkoti et al., 2017; Dow et al., 2021).
5.5 Limiting the activity of the inflammasome
Additionally, Mtb can prevent the activation of the NLRP3 inflammasome and subsequent pyroptosis in the host cell. Inhibition of the NLRP3 inflammasome linked with enhanced production of IL-1β in a caspase-1-dependent manner (Rastogi et al., 2021), is one way in which the serine/threonine kinase PknF assists Mtb in evading the host immune system. Upon inflammasome assembly, IL-1β is processed and activated, as mentioned above. A putative Zn2+ metalloprotease, encoded by the Mtb zmp1, inhibits inflammasome activation and IL-1β processing, thus reducing macrophage clearance of mycobacteria (Master et al., 2008). This is a previously unknown involvement for IL-1β in Mtb's modulation of the macrophage inflammasome.
5.6 Other Mtb evasion strategies
Modulation (upregulation) of transmembrane surface receptors (TREME2 or HRH1) can also evade macrophages (Dabla et al., 2022; Mo et al., 2022). Upregulation of these receptors activates STRING and p38MAPK-NOX2, inhibits pro-inflammatory cytokines (TNF-α, IL-1β, and ROS), and promotes anti-inflammatory cytokines (IFN-β and IL-10) (Dabla et al., 2022). Mtb effectors such as the early secreted protein target 12 (EST12), the methyltransferase (Rv1515c) encoded by the RD 6, or the dormancy regulator DosS, repress macrophage immune defenses (ROS, RNS, phagolysosomal maturation, proinflammatory response, inflammasome, antigen presentation) by activating the JAK2-STAT5a signaling pathway (Gautam et al., 2019; Yang et al., 2021; Rani et al., 2022; Yao et al., 2022). Mtb escape strategies are becoming increasingly non-negligible, and the aforementioned are recent and far to be exhaustive.
6 Concluding remarks and perspectives
The dialogue between Mtb and the host macrophage involves a permanent and stage-dependent interaction of Mtb's PAMPs, on one side and the macrophage's PRRs, on the other side, followed by induction of a cascade of reactions and cellular processes that activate and polarize the host macrophage. Then, the latter can control and eliminate Mtb through several processes, such as autophagy, apoptosis, inflammasome activation, ncRNA expression, phagosomal acidification, and the production of antimicrobial molecules. Unfortunately, these harsh conditions show limitations in that Mtb can subdue and manipulate them for survival. This imbalance in Mtb-macrophage crosstalks, where macrophages fail to holistically control Mtb infection, supported by the increasing discovery of previously unknown Mtb escape strategies, should be the critical point to tackle in TB control. Knowing that Mtb continually evolves and adapts, we are tempted to say that the aims assigned by the WHO to end TB may not be met by 2030. However, the fact that Mtb can simultaneously manipulate several host cell death processes may open new windows that facilitate the engineering of therapeutics with multitarget activity. Additionally, urgent attention is needed to further screen and functionally characterize Mtb's possible potential virulence factors and unveil novel mechanisms that may serve to identify new drug targets and elaborate appropriate therapeutics. Finally, it would be wise to accentuate the research by boosting host defense mechanisms and targeting critical axes of Mtb's escape mechanisms.
Author contributions
HB and UAEM reviewed the literature, designed the figures, and wrote the manuscript. YY, JP, LL, and MW designed the tables and critically revised the manuscript. XK and HC drafted the study and revised the manuscript. All authors contributed to the article and approved the submitted version.
Funding
This work was supported by the National Natural Science Foundation of China (31802142), the Doctoral Start-up Fund of Southwest University (SWU120019, SWU020023), the Fundamental Research Funds for the Central Universities (XDJK2019C089), and the China Postdoctoral Science Foundation (2019T120801 and 2017M620408).
Acknowledgments
In addition, we want to thank Liadrine Moukendza Koundi for the critical and fruitful discussion regarding this study.
Conflict of interest
The authors declare that the research was conducted in the absence of any commercial or financial relationships that could be construed as a potential conflict of interest.
Publisher’s note
All claims expressed in this article are solely those of the authors and do not necessarily represent those of their affiliated organizations, or those of the publisher, the editors and the reviewers. Any product that may be evaluated in this article, or claim that may be made by its manufacturer, is not guaranteed or endorsed by the publisher.
References
Abdalla, A. E., Ejaz, H., Mahjoob, M. O., Alameen, A. A.M., Abosalif, K. O.A., Elamir, M. Y.M., et al. (2020). Intelligent mechanisms of macrophage apoptosis subversion by mycobacterium. Pathogens 9 (3), 218. doi: 10.3390/pathogens9030218
Afriyie-Asante, A., Dabla, A., Dagenais, A., Berton, S., Smyth, R., Sun, J.. (2021). Mycobacterium tuberculosis exploits focal adhesion kinase to induce necrotic cell death and inhibit reactive oxygen species production. Front. Immunol. 12, 742370. doi: 10.3389/fimmu.2021.742370
Aguilo, J. I., Alonso, H., Uranga, S., Marinova, D., Arbués, A., de Martino, A, et al. (2013). ESX-1-induced apoptosis is involved in cell-to-cell spread of mycobacterium tuberculosis. Cell Microbiol. 15 (12), 1994–2005. doi: 10.1111/cmi.12169
Ali, M. K., Zhen, G., Nzungize, L., Stojkoska, A., Duan, X., Li, C., et al. (2020). Mycobacterium tuberculosis PE31 (Rv3477) attenuates host cell apoptosis and promotes recombinant m. smegmatis intracellular survival via up-regulating GTPase guanylate binding protein-1. Front. Cell Infect. Microbiol. 10, 40. doi: 10.3389/fcimb.2020.00040
Amaral, E. P., Costa, D. L., Namasivayam, S., Riteau, N., Kamenyeva, O., Mittereder, L., et al. (2019). A major role for ferroptosis in mycobacterium tuberculosis-induced cell death and tissue necrosis. J. Exp. Med. 216 (3), 556–570. doi: 10.1084/jem.20181776
Amaral, E. P., Foreman, T. W., Namasivayam, S., Hilligan, K. L., Kauffman, K. D., Barbosa Bomfim, C. C., et al. (2022). GPX4 regulates cellular necrosis and host resistance in mycobacterium tuberculosis infection. J. Exp. Med. 219 (11), e20220504. doi: 10.1084/jem.20220504
Arnett, E., Schlesinger, L. S. (2021). Live and let die: TB control by enhancing apoptosis. Immunity 54 (8), 1625–1627. doi: 10.1016/j.immuni.2021.07.010
Arora, S. K., Alam, A., Naqvi, N., Ahmad, J., Sheikh, J. A., Rahman, S. A., et al. (2020). Immunodominant mycobacterium tuberculosis protein Rv1507A elicits Th1 response and modulates host macrophage effector functions. Front. Immunol. 11, 1199. doi: 10.3389/fimmu.2020.01199
Asaad, M., Kaisar Ali, M., Abo-Kadoum, M. A., Lambert, N., Gong, Z., Wang, H., et al. (2021). Mycobacterium tuberculosis PPE10 (Rv0442c) alters host cell apoptosis and cytokine profile via linear ubiquitin chain assembly complex HOIP-NF-κB signaling axis. Int. Immunopharmacol 94, 107363.
Bah, A., Sanicas, M., Nigou, J., Guilhot, C., Astarie-Dequeker, C., Vergne, I.. (2020). The lipid virulence factors of mycobacterium tuberculosis exert multilayered control over autophagy-related pathways in infected human macrophages. Cells 9 (3), 666. doi: 10.3390/cells9030666
Barrientos, O. M., Langley, E., González, Y., Cabello, C., Torres, M., Guzmán-Beltrán, S.. (2022). Mycobacterium tuberculosis whiB3 and lipid metabolism genes are regulated by host induced oxidative stress. Microorganisms 10 (9), 1821. doi: 10.3390/microorganisms10091821
Beckwith, K. S., Beckwith, M. S., Ullmann, S., Sætra, R. S., Kim, H., Marstad, A., et al. (2020). Plasma membrane damage causes NLRP3 activation and pyroptosis during mycobacterium tuberculosis infection. Nat. Commun. 11 (1), 2270. doi: 10.1038/s41467-020-16143-6
Behar, S. M., Martin, C. J., Booty, M. G., Nishimura, T., Zhao, X., Gan, H. X., et al. (2011). Apoptosis is an innate defense function of macrophages against mycobacterium tuberculosis. Mucosal Immunol. 4 (3), 279–287. doi: 10.1038/mi.2011.3
Belisle, J. T., Vissa, V. D., Sievert, T., Takayama, K., Brennan, P. J., Besra, G. S. (1997). Role of the major antigen of mycobacterium tuberculosis in cell wall biogenesis. Science 276 (5317), 1420–1422. doi: 10.1126/science.276.5317.1420
Blumenthal, A., Kobayashi, T., Pierini, L. M., Banaei, N., Ernst, J. D., Miyake, K., et al. (2009). RP105 facilitates macrophage activation by mycobacterium tuberculosis lipoproteins. Cell Host Microbe 5 (1), 35–46. doi: 10.1016/j.chom.2008.12.002
BoseDasgupta, S., Pieters, J. (2018). Macrophage-microbe interaction: lessons learned from the pathogen mycobacterium tuberculosis. Semin. Immunopathol. 40 (6), 577–591. doi: 10.1007/s00281-018-0710-0
Bottai, D., Di Luca, M., Majlessi, L., Frigui, W., Simeone, R., Sayes, F., et al. (2012). Disruption of the ESX-5 system of mycobacterium tuberculosis causes loss of PPE protein secretion, reduction of cell wall integrity and strong attenuation. Mol. Microbiol. 83 (6), 1195–1209. doi: 10.1111/j.1365-2958.2012.08001.x
Brennan, P. J., Nikaido, H. (1995). The envelope of mycobacteria. Annu. Rev. Biochem. 64, 29–63. doi: 10.1146/annurev.bi.64.070195.000333
Chai, Q., Wang, L., Liu, C. H., Ge, B.. (2020). New insights into the evasion of host innate immunity by mycobacterium tuberculosis. Cell Mol. Immunol. 17 (9), 901–913. doi: 10.1038/s41423-020-0502-z
Chandra, P., He, L., Zimmerman, M., Yang, G., Köster, S., Ouimet, M., et al. (2020). Inhibition of fatty acid oxidation promotes macrophage control of mycobacterium tuberculosis. mBio 11 (4), e01139–20. doi: 10.1128/mBio.01139-20
Chandra, P., Grigsby, S. J., Philips, J. A. (2022). Immune evasion and provocation by mycobacterium tuberculosis. Nat. Rev. Microbiol. p, 1–17. doi: 10.1038/s41579-022-00763-4
Chatterjee, D., Lowell, K., Rivoire, B., McNeil, M. R., Brennan, P. J.. (1992). Lipoarabinomannan of mycobacterium tuberculosis. Capping with mannosyl residues in some strains. J. Biol. Chem. 267 (9), 6234–6239.
Choi, H. G., Choi, S., Back, Y. W., Park, H. S., Bae, H. S., Choi, C. H., et al. (2016). Mycobacterium tuberculosis Rv2882c protein induces activation of macrophages through TLR4 and exhibits vaccine potential. PloS One 11 (10), e0164458. doi: 10.1371/journal.pone.0164458
Dabla, A., Liang, Y. C., Rajabalee, N., Irwin, C., Moonen, C. G. J., Willis, J. V., et al. (2022). TREM2 promotes immune evasion by mycobacterium tuberculosis in human macrophages. mBio 13 (4), e0145622. doi: 10.1128/mbio.01456-22
Dai, Y., Shan, W., Yang, Q., Guo, J., Zhai, R., Tang, X., et al. (2019). Biomarkers of iron metabolism facilitate clinical diagnosis in mycobacterium tuberculosis infection. Thorax 74 (12), 1161–1167. doi: 10.1136/thoraxjnl-2018-212557
Danelishvili, L., Everman, J. L., McNamara, M. J., Bermudez, L. E. (2011). Inhibition of the plasma-Membrane-Associated serine protease cathepsin G by mycobacterium tuberculosis Rv3364c suppresses caspase-1 and pyroptosis in macrophages. Front. Microbiol. 2, 281. doi: 10.3389/fmicb.2011.00281
Davis, J. M., Ramakrishnan, L. (2009). The role of the granuloma in expansion and dissemination of early tuberculous infection. Cell 136 (1), 37–49. doi: 10.1016/j.cell.2008.11.014
Dawa, S., Menon, D., Arumugam, P., Bhaskar, A. K., Mondal, M., Rao, V., et al. (2021). Inhibition of granuloma triglyceride synthesis imparts control of mycobacterium tuberculosis through curtailed inflammatory responses. Front. Immunol. 12, 722735. doi: 10.3389/fimmu.2021.722735
Deng, W., Yang, W., Zeng, J., Abdalla, A. E., Xie, J. (2016). Mycobacterium tuberculosis PPE32 promotes cytokines production and host cell apoptosis through caspase cascade accompanying with enhanced ER stress response. Oncotarget 7 (41), 67347–67359. doi: 10.18632/oncotarget.12030
Deng, W., Long, Q., Zeng, J., Li, P., Yang, W., Chen, X, et al. (2017). Mycobacterium tuberculosis PE_PGRS41 enhances the intracellular survival of m. smegmatis within macrophages via blocking innate immunity and inhibition of host defense. Sci. Rep. 7, 46716. doi: 10.1038/srep46716
Deretic, V. (2012). Autophagy: An emerging immunological paradigm. J. Immunol. 189 (1), 15–20. doi: 10.4049/jimmunol.1102108
Deretic, V., Saitoh, T., Akira, S. (2013). Autophagy in infection, inflammation and immunity. Nat. Rev. Immunol. 13 (10), 722–737. doi: 10.1038/nri3532
Dixon, S. J., Lemberg, K. M., Lamprecht, M. R., Skouta, R., Zaitsev, E. M., Gleason, C. E, et al. (2012). Ferroptosis: An iron-dependent form of nonapoptotic cell death. Cell 149 (5), 1060–1072. doi: 10.1016/j.cell.2012.03.042
Dong, W., Wang, G., Feng, J., Li, P., Wang, R., Lu, H., et al. (2022). MiR-25 blunts autophagy and promotes the survival of mycobacterium tuberculosis by regulating NPC1. iScience 25 (5), 104279. doi: 10.1016/j.isci.2022.104279
Dou, Y., Xie, Y., Zhang, L., Liu, S., Xu, D., Wei, Y., et al. (2022). Host MKRN1-mediated mycobacterial PPE protein ubiquitination suppresses innate immune response. Front. Immunol. 13, 880315. doi: 10.3389/fimmu.2022.880315
Dow, A., Sule, P., O'Donnell, T. J., Burger, A., Mattila, J. T., Antonio, B., et al. (2021). Zinc limitation triggers anticipatory adaptations in mycobacterium tuberculosis. PloS Pathog. 17 (5), e1009570. doi: 10.1371/journal.ppat.1009570
Duan, L., Yi, M., Chen, J., Li, S., Chen, W. (2016). Mycobacterium tuberculosis EIS gene inhibits macrophage autophagy through up-regulation of IL-10 by increasing the acetylation of histone H3. Biochem. Biophys. Res. Commun. 473 (4), 1229–1234. doi: 10.1016/j.bbrc.2016.04.045
Dubey, N., Khan, M. Z., Kumar, S., Sharma, A., Das, L., Bhaduri, A, et al. (2021). Mycobacterium tuberculosis peptidyl prolyl isomerase a interacts with host integrin receptor to exacerbate disease progression. J. Infect. Dis. 224 (8), 1383–1393. doi: 10.1093/infdis/jiab081
Dutta, N. K., Mehra, S., Martinez, A. N., Alvarez, X., Renner, N. A., Morici, L. A., et al. (2012). The stress-response factor SigH modulates the interaction between mycobacterium tuberculosis and host phagocytes. PloS One 7 (1), e28958. doi: 10.1371/journal.pone.0028958
Ebrahimifard, N., Hadifar, S., Kargarpour Kamakoli, M., Behrouzi, A., Khanipour, S., Fateh, A., et al. (2022). Strain-specific behavior of mycobacterium tuberculosis in interruption of autophagy pathway in human alveolar type II epithelial A549 cells. Iran BioMed. J. 26 (4), 313–323. doi: 10.52547/ibj.3586
Feng, L., Chen, S., Hu, Y. (2018). PhoPR positively regulates whiB3 expression in response to low pH in pathogenic mycobacteria. J. Bacteriol 200 (8), e00766–17. doi: 10.1128/JB.00766-17
Feng, L., Hu, J., Zhang, W., Dong, Y., Xiong, S., Dong, C. (2020). RELL1 inhibits autophagy pathway and regulates mycobacterium tuberculosis survival in macrophages. Tuberculosis (Edinb) 120, 101900. doi: 10.1016/j.tube.2020.101900
Feng, Y., Li, M., Yangzhong, X., Zhang, X., Zu, A., Hou, Y., et al. (2022). Pyroptosis in inflammation-related respiratory disease. J. Physiol. Biochem 78 (4), 721–737. doi: 10.1007/s13105-022-00909-1
Flentie, K., Garner, A. L., Stallings, C. L. (2016). Mycobacterium tuberculosis transcription machinery: Ready to respond to host attacks. J. Bacteriol 198 (9), 1360–1373. doi: 10.1128/JB.00935-15
Fratti, R. A., Chua, J., Vergne, I., Deretic, V.. (2003). Mycobacterium tuberculosis glycosylated phosphatidylinositol causes phagosome maturation arrest. Proc. Natl. Acad. Sci. U.S.A. 100 (9), 5437–5442. doi: 10.1073/pnas.0737613100
Fu, B., Xue, W., Zhang, H., Zhang, R., Feldman, K., Zhao, Q., et al. (2020). MicroRNA-325-3p facilitates immune escape of mycobacterium tuberculosis through targeting LNX1 via NEK6 accumulation to promote anti-apoptotic STAT3 signaling. mBio 11 (3). doi: 10.1128/mBio.00557-20
Fu, Y., Shen, J., Li, Y., Liu, F., Ning, B., Zheng, Y., et al. (2021). Inhibition of the PERK/TXNIP/NLRP3 axis by baicalin reduces NLRP3 inflammasome-mediated pyroptosis in macrophages infected with mycobacterium tuberculosis. Mediators Inflammation 2021, 1805147. doi: 10.1155/2021/1805147
Gautam, U. S., Mehra, S., Kumari, P., Alvarez, X., Niu, T., Tyagi, J. S., et al. (2019). Mycobacterium tuberculosis sensor kinase DosS modulates the autophagosome in a DosR-independent manner. Commun. Biol. 2, 349. doi: 10.1038/s42003-019-0594-0
Ge, P., Lei, Z., Yu, Y., Lu, Z., Qiang, L., Chai, Q., et al. (2022). M. tuberculosis PknG manipulates host autophagy flux to promote pathogen intracellular survival. Autophagy 18 (3), 576–594. doi: 10.1080/15548627.2021.1938912
Gong, Z., Kuang, Z., Li, H., Li, C., Ali, M. K., Huang, et al. (2019). Regulation of host cell pyroptosis and cytokines production by mycobacterium tuberculosis effector PPE60 requires LUBAC mediated NF-κB signaling. Cell Immunol. 335, 41–50. doi: 10.1016/j.cellimm.2018.10.009
Gong, Z., Yang, W., Zhang, H., Xiang, X., Zeng, J., Han, S., et al. (2020). Mycobacterium tuberculosis Rv3717 enhances the survival of mycolicibacterium smegmatis by inhibiting host innate immune and caspase-dependent apoptosis. Infect. Genet. Evol. 84, 104412. doi: 10.1016/j.meegid.2020.104412
Gordon, S. (2003). Alternative activation of macrophages. Nat. Rev. Immunol. 3 (1), 23–35. doi: 10.1038/nri978
Graves, D. T., Milovanova, T. N. (2019). Mucosal immunity and the FOXO1 transcription factors. Front. Immunol. 10, 2530. doi: 10.3389/fimmu.2019.02530
Guo, Q., Bi, J., Li, M., Ge, W., Xu, Y., Fan, W., et al. (2019). ESX secretion-associated protein c from mycobacterium tuberculosis induces macrophage activation through the toll-like receptor-4/Mitogen-Activated protein kinase signaling pathway. Front. Cell Infect. Microbiol. 9, 158. doi: 10.3389/fcimb.2019.00158
Halder, P., Kumar, R., Jana, K., Chakraborty, S., Ghosh, Z., Kundu, M., et al. (2015). Gene expression profiling of mycobacterium tuberculosis lipoarabinomannan-treated macrophages: A role of the bcl-2 family member A1 in inhibition of apoptosis in mycobacteria-infected macrophages. IUBMB Life 67 (9), 726–736. doi: 10.1002/iub.1430
Hawerkamp, H. C., van Geelen, L., Korte, J., Di Domizio, J., Swidergall, M., Momin, A. A., et al. (2020). Interleukin-26 activates macrophages and facilitates killing of mycobacterium tuberculosis. Sci. Rep. 10 (1), 17178. doi: 10.1038/s41598-020-73989-y
He, Y., Hara, H., Núñez, G. (2016). Mechanism and regulation of NLRP3 inflammasome activation. Trends Biochem. Sci. 41 (12), 1012–1021. doi: 10.1016/j.tibs.2016.09.002
Hou, F., Xiao, K., Tang, L., Xie, L.. (2021). Diversity of macrophages in lung homeostasis and diseases. Front. Immunol. 12, 753940. doi: 10.3389/fimmu.2021.753940
Hume, P. S., Gibbings, S. L., Jakubzick, C. V., Tuder, R. M., Curran-Everett, D., Henson, P. M., et al. (2020). Localization of macrophages in the human lung via design-based stereology. Am. J. Respir. Crit. Care Med. 201 (10), 1209–1217. doi: 10.1164/rccm.201911-2105OC
Intemann, C. D., Thye, T., Niemann, S., Browne, E. N., Amanua Chinbuah, M., Enimil, A, et al. (2009). Autophagy gene variant IRGM -261T contributes to protection from tuberculosis caused by mycobacterium tuberculosis but not by m. africanum strains. PloS Pathog. 5 (9), e1000577. doi: 10.1371/journal.ppat.1000577
Jayakumar, D., Jacobs, Jr. W. R., Narayanan, S. (2008). Protein kinase e of mycobacterium tuberculosis has a role in the nitric oxide stress response and apoptosis in a human macrophage model of infection. Cell Microbiol. 10 (2), 365–374. doi: 10.1111/j.1462-5822.2007.01049.x
Jiang, F., Lou, J., Zheng, X. M., Yang, X. Y.. (2021). LncRNA MIAT regulates autophagy and apoptosis of macrophage infected by mycobacterium tuberculosis through the miR-665/ULK1 signaling axis. Mol. Immunol. 139, 42–49. doi: 10.1016/j.molimm.2021.07.023
Joseph, S., Yuen, A., Singh, V., Hmama, Z. (2017). Mycobacterium tuberculosis Cpn60.2 (GroEL2) blocks macrophage apoptosis via interaction with mitochondrial mortalin. Biol. Open 6 (4), 481–488. doi: 10.1242/bio.023119
Ke, Z., Lu, J., Zhu, J., Yang, Z., Jin, Z., Yuan, L. (2020). Down-regulation of lincRNA-EPS regulates apoptosis and autophagy in BCG-infected RAW264.7 macrophages via JNK/MAPK signaling pathway. Infect. Genet. Evol. 77, 104077. doi: 10.1016/j.meegid.2019.104077
Kim, M. J., Wainwright, H. C., Locketz, M., Bekker, L. G., Walther, G. B., Dittrich, C., et al. (2010). Caseation of human tuberculosis granulomas correlates with elevated host lipid metabolism. EMBO Mol. Med. 2 (7), 258–274. doi: 10.1002/emmm.201000079
Kim, T. S., Jin, Y. B., Kim, Y. S., Kim, S., Kim, J. K., Lee, H. M., et al. (2019). SIRT3 promotes antimycobacterial defenses by coordinating mitochondrial and autophagic functions. Autophagy 15 (8), 1356–1375. doi: 10.1080/15548627.2019.1582743
Kim, J. S., Kim, H. K., Cho, E., Mun, S. J., Jang, S., Jang, J., et al. (2022). PE_PGRS38 interaction with HAUSP downregulates antimycobacterial host defense via TRAF6. Front. Immunol. 13, 862628. doi: 10.3389/fimmu.2022.862628
Krantz, B. A., Melnyk, R. A., Zhang, S., Juris, S. J., Lacy, D. B., Wu, Z., et al. (2005). A phenylalanine clamp catalyzes protein translocation through the anthrax toxin pore. Science 309 (5735), 777–781. doi: 10.1126/science.1113380
Kurthkoti, K., Amin, H., Marakalala, M. J., Ghanny, S., Subbian, S., Sakatos, A., et al. (2017). The capacity of mycobacterium tuberculosis to survive iron starvation might enable it to persist in iron-deprived microenvironments of human granulomas. mBio 8 (4), e01092–17. doi: 10.1128/mBio.01092-17
Landes, M. B., Rajaram, M. V., Nguyen, H., Schlesinger, L. S. (2015). Role for NOD2 in mycobacterium tuberculosis-induced iNOS expression and NO production in human macrophages. J. Leukoc. Biol. 97 (6), 1111–1119. doi: 10.1189/jlb.3A1114-557R
Laopanupong, T., Prombutara, P., Kanjanasirirat, P., Benjaskulluecha, S., Boonmee, A., Palaga, T., et al. (2021). Lysosome repositioning as an autophagy escape mechanism by mycobacterium tuberculosis Beijing strain. Sci. Rep. 11 (1), 4342. doi: 10.1038/s41598-021-83835-4
Lata, S., Mahatha, A. C., Mal, S., Gupta, U. D., Kundu, M., Basu, J. (2022). Unraveling novel roles of the mycobacterium tuberculosis transcription factor Rv0081 in regulation of the nucleoid-associated proteins Lsr2 and EspR, cholesterol utilization, and subversion of lysosomal trafficking in macrophages. Mol. Microbiol. 117 (5), 1104–1120. doi: 10.1111/mmi.14895
Lee, J., Hartman, M., Kornfeld, H. (2009). Macrophage apoptosis in tuberculosis. Yonsei Med. J. 50 (1), 1–11. doi: 10.3349/ymj.2009.50.1.1
Li, H., Li, Q., Yu, Z., Zhou, M., Xie, J. (2016). Mycobacterium tuberculosis PE13 (Rv1195) manipulates the host cell fate via p38-ERK-NF-κB axis and apoptosis. Apoptosis 21 (7), 795–808. doi: 10.1007/s10495-016-1249-y
Li, C., Peng, H., Japtok, L., Seitz, A., Riehle, A., Wilker, B., et al. (2016). Inhibition of neutral sphingomyelinase protects mice against systemic tuberculosis. Front. Biosci. (Elite Ed) 8 (2), 311–325. doi: 10.2741/E769
Li, M., Cui, J., Niu, W., Huang, J., Feng, T., Sun, B., et al. (2019). Long non-coding PCED1B-AS1 regulates macrophage apoptosis and autophagy by sponging miR-155 in active tuberculosis. Biochem. Biophys. Res. Commun. 509 (3), 803–809. doi: 10.1016/j.bbrc.2019.01.005
Li, D., Gao, C., Zhao, L., Zhang, Y. (2020). Inflammatory response is modulated by lincRNACox2 via the NF−κB pathway in macrophages infected by mycobacterium tuberculosis. Mol. Med. Rep. 21 (6), 2513–2521. doi: 10.3892/mmr.2020.11053
Li, Y., Fu, Y., Sun, J., Shen, J., Liu, F., Ning, B., et al. (2022). Tanshinone IIA alleviates NLRP3 inflammasome-mediated pyroptosis in mycobacterium tuberculosis-(H37Ra-) infected macrophages by inhibiting endoplasmic reticulum stress. J. Ethnopharmacol 282, 114595. doi: 10.1016/j.jep.2021.114595
Liang, S., Wang, F., Bao, C., Han, J., Guo, Y., Liu, F., et al. (2020). BAG2 ameliorates endoplasmic reticulum stress-induced cell apoptosis in mycobacterium tuberculosis-infected macrophages through selective autophagy. Autophagy 16 (8), 1453–1467. doi: 10.1080/15548627.2019.1687214
Liu, Y., Xie, Z., Zhou, X., Li, W., Zhang, H., He, Z. G.. (2019). NapM enhances the survival of mycobacterium tuberculosis under stress and in macrophages. Commun. Biol. 2, 65. doi: 10.1038/s42003-019-0314-9
Liu, G., Wan, Q., Li, J., Hu, X., Gu, X., Xu, S. (2020). Silencing miR-125b-5p attenuates inflammatory response and apoptosis inhibition in mycobacterium tuberculosis-infected human macrophages by targeting DNA damage-regulated autophagy modulator 2 (DRAM2). Cell Cycle 19 (22), 3182–3194. doi: 10.1080/15384101.2020.1838792
Liu, K., Hong, D., Zhang, F., Li, X., He, M., Han, X., et al. (2020). MicroRNA-106a inhibits autophagy process and antimicrobial responses by targeting ULK1, ATG7, and ATG16L1 during mycobacterial infection. Front. Immunol. 11, 610021. doi: 10.3389/fimmu.2020.610021
Liu, L., Zhai, K., Chen, Y., Chen, X., Wang, G., Wu, L. (2021). Effect and mechanism of mycobacterium tuberculosis lipoprotein LpqH in NLRP3 inflammasome activation in mouse ana-1 macrophage. BioMed. Res. Int 2021, 8239135. doi: 10.1155/2021/8239135
Long, Q., Xiang, X., Yin, Q., Li, S., Yang, W., Sun, H., et al. (2019). PE_PGRS62 promotes the survival of mycobacterium smegmatis within macrophages via disrupting ER stress-mediated apoptosis. J. Cell Physiol. 234 (11), 19774–19784. doi: 10.1002/jcp.28577
Lu, Y., Li, Q., Peng, J., Zhu, Y., Wang, F., Wang, C., et al. (2016). Association of autophagy-related IRGM polymorphisms with latent versus active tuberculosis infection in a Chinese population. Tuberculosis (Edinb) 97, 47–51. doi: 10.1016/j.tube.2016.01.001
Ma, J., Chen, X. L., Sun, Q. (2019). microRNA-579 upregulation mediates death of human macrophages with mycobacterium tuberculosis infection. Biochem. Biophys. Res. Commun. 518 (2), 219–226. doi: 10.1016/j.bbrc.2019.08.035
Madacki, J., Mas Fiol, G., Brosch, R. (2019). Update on the virulence factors of the obligate pathogen mycobacterium tuberculosis and related tuberculosis-causing mycobacteria. Infect. Genet. Evol. 72, 67–77. doi: 10.1016/j.meegid.2018.12.013
Mahatha, A. C., Mal, S., Majumder, D., Saha, S., Ghosh, A., Basu, J., et al. (2020). RegX3 activates whiB3 under acid stress and subverts lysosomal trafficking of mycobacterium tuberculosis in a WhiB3-dependent manner. Front. Microbiol. 11, 572433. doi: 10.3389/fmicb.2020.572433
Master, S. S., Rampini, S. K., Davis, A. S., Keller, C., Ehlers, S., Springer, B., et al. (2008). Mycobacterium tuberculosis prevents inflammasome activation. Cell Host Microbe 3 (4), 224–232. doi: 10.1016/j.chom.2008.03.003
Mehta, M., Rajmani, R. S., Singh, A. (2016). Mycobacterium tuberculosis WhiB3 responds to vacuolar pH-induced changes in mycothiol redox potential to modulate phagosomal maturation and virulence. J. Biol. Chem. 291 (6), 2888–2903. doi: 10.1074/jbc.M115.684597
Mehta, M., Singh, A. (2019). Mycobacterium tuberculosis WhiB3 maintains redox homeostasis and survival in response to reactive oxygen and nitrogen species. Free Radic. Biol. Med. 131, 50–58. doi: 10.1016/j.freeradbiomed.2018.11.032
Meunier, E., Neyrolles, O. (2019). Die another way: Ferroptosis drives tuberculosis pathology. J. Exp. Med. 216 (3), 471–473. doi: 10.1084/jem.20190038
Mo, S., Guo, J., Ye, T., Zhang, X., Zeng, J., Xu, Y., et al. (2022). Mycobacterium tuberculosis utilizes host histamine receptor H1 to modulate reactive oxygen species production and phagosome maturation via the p38MAPK-NOX2 axis. mBio 13 (5), e0200422. doi: 10.1128/mbio.02004-22
Paik, S., Jo, E. K. (2020). An interplay between autophagy and immunometabolism for host defense against mycobacterial infection. Front. Immunol. 11, 603951. doi: 10.3389/fimmu.2020.603951
Palma, C., La Rocca, C., Gigantino, V., Aquino, G., Piccaro, G., Di Silvestre, D., et al. (2021). Caloric restriction promotes immunometabolic reprogramming leading to protection from tuberculosis. Cell Metab. 33 (2), 300–318.e12. doi: 10.1016/j.cmet.2020.12.016
Pareja, M. E., Colombo, M. I. (2013). Autophagic clearance of bacterial pathogens: molecular recognition of intracellular microorganisms. Front. Cell Infect. Microbiol. 3, 54. doi: 10.3389/fcimb.2013.00054
Qu, Z., Zhou, J., Zhou, Y., Xie, Y., Jiang, Y., Wu, J., et al. (2020). Mycobacterial EST12 activates a RACK1-NLRP3-gasdermin d pyroptosis-IL-1β immune pathway. Sci. Adv. 6 (43), eaba4733. doi: 10.1126/sciadv.aba4733
Qu, Y., Gao, Q., Wu, S., Xu, T., Jiang, D., Xu, G. (2021). MicroRNA-142-3p inhibits autophagy and promotes intracellular survival of mycobacterium tuberculosis by targeting ATG16L1 and ATG4c. Int. Immunopharmacol 101 (Pt A), 108202. doi: 10.1016/j.intimp.2021.108202
Ramon-Luing, L. A., Olvera, Y., Flores-Gonzalez, J., Palacios, Y., Carranza, C., Aguilar-Duran, Y., et al. (2022). Diverse cell death mechanisms are simultaneously activated in macrophages infected by virulent mycobacterium tuberculosis. Pathogens 11 (5), 492. doi: 10.3390/pathogens11050492
Rani, A., Alam, A., Ahmad, F. P. M., Saurabh, A., Zarin, S., Mitra, D. K., et al. (2022). Mycobacterium tuberculosis methyltransferase Rv1515c can suppress host defense mechanisms by modulating immune functions utilizing a multipronged mechanism. Front. Mol. Biosci. 9, 906387. doi: 10.3389/fmolb.2022.906387
Rastogi, S., Ellinwood, S., Augenstreich, J., Mayer-Barber, K. D., Briken, V. (2021). Mycobacterium tuberculosis inhibits the NLRP3 inflammasome activation via its phosphokinase PknF. PloS Pathog. 17 (7), e1009712. doi: 10.1371/journal.ppat.1009712
Ravesloot-Chávez, M. M., Van Dis, E., Stanley, S. A. (2021). The innate immune response to mycobacterium tuberculosis infection. Annu. Rev. Immunol. 39, 611–637. doi: 10.1146/annurev-immunol-093019-010426
Reddy, V. P., Chinta, K. C., Saini, V., Glasgow, J. N., Hull, T. D., Traylor, A., et al. (2018). Ferritin h deficiency in myeloid compartments dysregulates host energy metabolism and increases susceptibility to mycobacterium tuberculosis infection. Front. Immunol. 9, 860. doi: 10.3389/fimmu.2018.00860
Refai, A., Gritli, S., Barbouche, M. R., Essafi, M. (2018). Mycobacterium tuberculosis virulent factor ESAT-6 drives macrophage differentiation toward the pro-inflammatory M1 phenotype and subsequently switches it to the anti-inflammatory M2 phenotype. Front. Cell Infect. Microbiol. 8, 327. doi: 10.3389/fcimb.2018.00327
Rolando, M., Buchrieser, C. (2019). A comprehensive review on the manipulation of the sphingolipid pathway by pathogenic bacteria. Front. Cell Dev. Biol. 7, 168. doi: 10.3389/fcell.2019.00168
Ruiz, A., Guzmán-Beltrán, S., Carreto-Binaghi, L. E., Gonzalez, Y., Juárez, E., et al. (2019). DNA From virulent m. tuberculosis induces TNF-α production and autophagy in M1 polarized macrophages. Microb. Pathog. 132, 166–177. doi: 10.1016/j.micpath.2019.04.041
Saini, V., Farhana, A., Steyn, A. J. (2012). Mycobacterium tuberculosis WhiB3: a novel iron-sulfur cluster protein that regulates redox homeostasis and virulence. Antioxid Redox Signal 16 (7), 687–697. doi: 10.1089/ars.2011.4341
Sengupta, S., Nayak, B., Meuli, M., Sander, P., Mishra, S., Sonawane, A. (2021). Mycobacterium tuberculosis phosphoribosyltransferase promotes bacterial survival in macrophages by inducing histone hypermethylation in autophagy-related genes. Front. Cell Infect. Microbiol. 11, 676456. doi: 10.3389/fcimb.2021.676456
Sha, S., Shi, Y., Tang, Y., Jia, L., Han, X., Liu, Y., et al. (2021). Mycobacterium tuberculosis Rv1987 protein induces M2 polarization of macrophages through activating the PI3K/Akt1/mTOR signaling pathway. Immunol. Cell Biol. 99 (6), 570–585. doi: 10.1111/imcb.12436
Shi, Q., Wang, J., Yang, Z., Liu, Y. (2020). CircAGFG1modulates autophagy and apoptosis of macrophages infected by mycobacterium tuberculosis via the notch signaling pathway. Ann. Transl. Med. 8 (10), 645. doi: 10.21037/atm.2020-20-3048
Shin, D. M., Jeon, B. Y., Lee, H. M., Jin, H. S., Yuk, J. M., Song, C. H., et al. (2010). Mycobacterium tuberculosis eis regulates autophagy, inflammation, and cell death through redox-dependent signaling. PloS Pathog. 6 (12), e1001230. doi: 10.1371/journal.ppat.1001230
Silvério, D., Gonçalves, R., Appelberg, R., Saraiva, M. (2021). Advances on the role and applications of interleukin-1 in tuberculosis. mBio 12 (6), e0313421. doi: 10.1128/mBio.03134-21
Siregar, T. A. P., Prombutara, P., Kanjanasirirat, P., Kunkaew, N., Tubsuwan, A., Boonmee, A., et al. (2022). The autophagy-resistant mycobacterium tuberculosis Beijing strain upregulates KatG to evade starvation-induced autophagic restriction. Pathog. Dis. 80 (1), ftac004. doi: 10.1093/femspd/ftac004
Songane, M., Kleinnijenhuis, J., Netea, M. G., van Crevel, R. (2012). The role of autophagy in host defence against mycobacterium tuberculosis infection. Tuberculosis (Edinb) 92 (5), 388–396. doi: 10.1016/j.tube.2012.05.004
Speer, A., Sun, J., Danilchanka, O., Meikle, V., Rowland, J. L., Walter, K., et al. (2015). Surface hydrolysis of sphingomyelin by the outer membrane protein Rv0888 supports replication of mycobacterium tuberculosis in macrophages. Mol. Microbiol. 97 (5), 881–897. doi: 10.1111/mmi.13073
Srivastava, S., Battu, M. B., Khan, M. Z., Nandicoori, V. K., Mukhopadhyay, S. (2019). Mycobacterium tuberculosis PPE2 protein interacts with p67(phox) and inhibits reactive oxygen species production. J. Immunol. 203 (5), 1218–1229. doi: 10.4049/jimmunol.1801143
Stamm, C. E., Collins, A. C., Shiloh, M. U. (2015). Sensing of mycobacterium tuberculosis and consequences to both host and bacillus. Immunol. Rev. 264 (1), 204–219. doi: 10.1111/imr.12263
Strong, E. J., Jurcic Smith, K. L., Saini, N. K., Ng, T. W., Porcelli, S. A., Lee, S., et al. (2020). Identification of autophagy-inhibiting factors of mycobacterium tuberculosis by high-throughput loss-of-Function screening. Infect. Immun. 88 (12), e00269–20. doi: 10.1128/IAI.00269-20
Strong, E. J. (2021). Mycobacterium tuberculosis PE_PGRS20 and PE_PGRS47 proteins inhibit autophagy by interaction with Rab1A. mSphere 6 (4), e0054921. doi: 10.1128/mSphere.00549-21
Strong, E. J., Ng, T. W., Porcelli, S. A., Lee, S. (2022). Mycobacterium tuberculosis PPE51 inhibits autophagy by suppressing toll-like receptor 2-dependent signaling. mBio 13 (3), e0297421. doi: 10.1128/mbio.02974-21
Stutz, M. D., Allison, C. C., Ojaimi, S., Preston, S. P., Doerflinger, M., Arandjelovic, P., et al. (2021). Macrophage and neutrophil death programs differentially confer resistance to tuberculosis. Immunity 54 (8), 1758–1771.e7. doi: 10.1016/j.immuni.2021.06.009
Subbarao, S., Sanchez-Garrido, J., Krishnan, N., Shenoy, A. R., Robertson, B. D. (2020). Genetic and pharmacological inhibition of inflammasomes reduces the survival of mycobacterium tuberculosis strains in macrophages. Sci. Rep. 10 (1), 3709. doi: 10.1038/s41598-020-60560-y
Tsolaki, A. G., Varghese, P. M., Kishore, U. (2021). Innate immune pattern recognition receptors of mycobacterium tuberculosis: Nature and consequences for pathogenesis of tuberculosis. Adv. Exp. Med. Biol. 1313, 179–215. doi: 10.1007/978-3-030-67452-6_9
Van den Bossche, J., O’Neill, L. A., Menon, D. (2017). Macrophage immunometabolism: Where are we (Going)? Trends Immunol. 38 (6), 395–406. doi: 10.1016/j.it.2017.03.001
Vergne, I., Gilleron, M., Nigou, J. (2014). Manipulation of the endocytic pathway and phagocyte functions by mycobacterium tuberculosis lipoarabinomannan. Front. Cell Infect. Microbiol. 4, 187. doi: 10.3389/fcimb.2014.00187
Wang, J., Li, B. X., Ge, P. P., Li, J., Wang, Q., Gao, G. F., et al. (2015). Mycobacterium tuberculosis suppresses innate immunity by coopting the host ubiquitin system. Nat. Immunol. 16 (3), 237–245. doi: 10.1038/ni.3096
Wang, J., Teng, J. L., Zhao, D., Ge, P., Li, B., Woo, P. C., et al. (2016). The ubiquitin ligase TRIM27 functions as a host restriction factor antagonized by mycobacterium tuberculosis PtpA during mycobacterial infection. Sci. Rep. 6, 34827. doi: 10.1038/srep34827
Wang, J., Ge, P., Qiang, L., Tian, F., Zhao, D., Chai, Q., et al. (2017). The mycobacterial phosphatase PtpA regulates the expression of host genes and promotes cell proliferation. Nat. Commun. 8 (1), 244. doi: 10.1038/s41467-017-00279-z
Wang, L. X., Zhang, S. X., Wu, H. J., Rong, X. L., Guo, J. (2019). M2b macrophage polarization and its roles in diseases. J. Leukoc. Biol. 106 (2), 345–358. doi: 10.1002/JLB.3RU1018-378RR
Wang, L., Wu, J., Li, J., Yang, H., Tang, T., Liang, H., et al. (2020). Host-mediated ubiquitination of a mycobacterial protein suppresses immunity. Nature 577 (7792), 682–688. doi: 10.1038/s41586-019-1915-7
Wang, J., Ge, P., Lei, Z., Lu, Z., Qiang, L., Chai, Q., et al. (2021). Mycobacterium tuberculosis protein kinase G acts as an unusual ubiquitinating enzyme to impair host immunity. EMBO Rep. 22 (6), e52175. doi: 10.15252/embr.202052175
Weiss, G., Schaible, U. E. (2015). Macrophage defense mechanisms against intracellular bacteria. Immunol. Rev. 264 (1), 182–203. doi: 10.1111/imr.12266
Wu, Y., Li, C., Riehle, A., Pollmeier, B., Gulbins, E., Grassmé, H. (2018). Mycobacterial infection is promoted by neutral sphingomyelinase 2 regulating a signaling cascade leading to activation of β1-integrin. Cell Physiol. Biochem. 51 (4), 1815–1829. doi: 10.1159/000495683
Wu, Y., Li, C., Peng, H., Swaidan, A., Riehle, A., Pollmeier, B., et al. (2020). Acid sphingomyelinase contributes to the control of mycobacterial infection via a signaling cascade leading from reactive oxygen species to cathepsin d. Cells 9 (11), 2406. doi: 10.3390/cells9112406
Wu, J., Luo, F. L., Xie, Y., Xiong, H., Gao, Y., Liu, G., et al. (2022). EST12 regulates myc expression and enhances anti-mycobacterial inflammatory response via RACK1-JNK-AP1-Myc immune pathway. Front. Immunol. 13, 943174. doi: 10.3389/fimmu.2022.943174
Xu, F., Qi, H., Li, J., Sun, L., Gong, J., Chen, Y., et al. (2020). Mycobacterium tuberculosis infection up-regulates MFN2 expression to promote NLRP3 inflammasome formation. J. Biol. Chem. 295 (51), 17684–17697. doi: 10.1074/jbc.RA120.014077
Yang, W. S., SriRamaratnam, R., Welsch, M. E., Shimada, K., Skouta, R., Viswanathan, V. S., et al. (2014). Regulation of ferroptotic cancer cell death by GPX4. Cell 156 (1-2), 317–331. doi: 10.1016/j.cell.2013.12.010
Yang, W., Deng, W., Zeng, J., Ren, S., Ali, M. K., Gu, Y., et al. (2017). Mycobacterium tuberculosis PE_PGRS18 enhances the intracellular survival of m. smegmatis via altering host macrophage cytokine profiling and attenuating the cell apoptosis. Apoptosis 22 (4), 502–509. doi: 10.1007/s10495-016-1336-0
Yang, Q., Liao, M., Wang, W., Zhang, M., Chen, Q., Guo, J., et al. (2019). CD157 confers host resistance to mycobacterium tuberculosis via TLR2-CD157-PKCzeta-Induced reactive oxygen species production. mBio 10 (4), 345–358. doi: 10.1128/mBio.01949-19
Yang, Y., Xu, P., He, P., Shi, F., Tang, Y., Guan, C., et al. (2020). Mycobacterial PPE13 activates inflammasome by interacting with the NATCH and LRR domains of NLRP3. FASEB J. 34 (9), 12820–12833. doi: 10.1096/fj.202000200RR
Yang, W., Liu, M., Yu, X., Huang, Y., Zeng, J., Dai, Y., et al. (2021). Mycobacterium tuberculosis Rv1515c antigen enhances survival of m. smegmatis within macrophages by disrupting the host defence. Microb. Pathog. 153, 104778. doi: 10.1016/j.micpath.2021.104778
Yant, L. J., Ran, Q., Rao, L., Van Remmen, H., Shibatani, T., Belter, J. G., et al. (2003). The selenoprotein GPX4 is essential for mouse development and protects from radiation and oxidative damage insults. Free Radic. Biol. Med. 34 (4), 496–502. doi: 10.1016/S0891-5849(02)01360-6
Yao, Q., Xie, Y., Xu, D., Qu, Z., Wu, J., Zhou, Y., et al. (2022). Lnc-EST12, which is negatively regulated by mycobacterial EST12, suppresses antimycobacterial innate immunity through its interaction with FUBP3. Cell Mol. Immunol. 19 (8), 883–897. doi: 10.1038/s41423-022-00878-x
Yassine, E., Galiwango, R., Ssengooba, W., Ashaba, F., Joloba, M. L., Zalwango, S., et al. (2021). Assessing a transmission network of mycobacterium tuberculosis in an African city using single nucleotide polymorphism threshold analysis. Microbiologyopen 10 (3), e1211. doi: 10.1002/mbo3.1211
You, D., Xu, Y., Yin, B. C., Ye, B. C. (2019). Nitrogen regulator GlnR controls redox sensing and lipids anabolism by directly activating the whiB3 in mycobacterium smegmatis. Front. Microbiol. 10, 74. doi: 10.3389/fmicb.2019.00074
Zhang, J., Jiang, R., Takayama, H., Tanaka, Y. (2005). Survival of virulent mycobacterium tuberculosis involves preventing apoptosis induced by bcl-2 upregulation and release resulting from necrosis in J774 macrophages. Microbiol. Immunol. 49 (9), 845–852. doi: 10.1111/j.1348-0421.2005.tb03673.x
Zhang, W., Lu, Q., Dong, Y., Yue, Y., Xiong, S. (2018). Rv3033, as an emerging anti-apoptosis factor, facilitates mycobacteria survival via inhibiting macrophage intrinsic apoptosis. Front. Immunol. 9, 2136. doi: 10.3389/fimmu.2018.02136
Zhang, Y., Li, S., Liu, Q., Long, R., Feng, J., Qin, H., et al. (2020). Mycobacterium tuberculosis heat-shock protein 16.3 induces macrophage M2 polarization through CCRL2/CX3CR1. Inflammation 43 (2), 487–506. doi: 10.1007/s10753-019-01132-9
Zhang, S., Liu, Y., Zhou, X., Ou, M., Xiao, G., Li, F., et al. (2021). Sirtuin 7 regulates nitric oxide production and apoptosis to promote mycobacterial clearance in macrophages. Front. Immunol. 12, 779235. doi: 10.3389/fimmu.2021.779235
Zhang, D., Yi, Z., Fu, Y. (2019). Downregulation of miR-20b-5p facilitates mycobacterium tuberculosis survival in RAW 264.7 macrophages via attenuating the cell apoptosis by mcl-1 upregulation. J. Cell Biochem. 120 (4), 5889–5896. doi: 10.1002/jcb.27874
Zhao, X., Khan, N., Gan, H., Tzelepis, F., Nishimura, T., Park, S. Y., et al. (2017). Bcl-x(L) mediates RIPK3-dependent necrosis in m. tuberculosis-infected macrophages. Mucosal Immunol. 10 (6), 1553–1568. doi: 10.1038/mi.2017.12
Keywords: intracellular pathogen, Mycobacterium tuberculosis, host macrophage, molecular interaction, immune control, immune evasion, tuberculosis control
Citation: Bo H, Moure UAE, Yang Y, Pan J, Li L, Wang M, Ke X and Cui H (2023) Mycobacterium tuberculosis-macrophage interaction: Molecular updates. Front. Cell. Infect. Microbiol. 13:1062963. doi: 10.3389/fcimb.2023.1062963
Received: 06 October 2022; Accepted: 13 February 2023;
Published: 03 March 2023.
Edited by:
Timothy Keiffer, Louisiana State University Health Shreveport, United StatesReviewed by:
Abhishek Mishra, Houston Methodist Research Institute, United StatesDiego Luis Costa, Department of Biochemistry and Immunology, University of São Paulo, Brazil
Copyright © 2023 Bo, Moure, Yang, Pan, Li, Wang, Ke and Cui. This is an open-access article distributed under the terms of the Creative Commons Attribution License (CC BY). The use, distribution or reproduction in other forums is permitted, provided the original author(s) and the copyright owner(s) are credited and that the original publication in this journal is cited, in accordance with accepted academic practice. No use, distribution or reproduction is permitted which does not comply with these terms.
*Correspondence: Hongjuan Cui, aGN1aUBzd3UuZWR1LmNu; Xiaoxue Ke, a2V4aWFveHVlQDEyNi5jb20=
†These authors have contributed equally to this work and share first authorship