- 1Department of Microbiology and Immunology, University of Otago, Dunedin, New Zealand
- 2Maurice Wilkins, Centre for Molecular Biodiscovery, The University of Auckland, Auckland, New Zealand
- 3School of Pharmacy, Jinan University, Guangzhou, China
- 4State Key Laboratory of Respiratory Disease, Guangzhou Institutes of Biomedicine and Health (GIBH), Chinese Academy of Sciences (CAS), Guangzhou, China
- 5China-New Zealand Joint Laboratory of Biomedicine and Health, Guangzhou Institutes of Biomedicine and Health (GIBH), Chinese Academy of Sciences (CAS), Guangzhou, China
- 6Guangdong-Hong Kong-Macau Joint Laboratory of Respiratory Infectious Diseases, Guangzhou, China
- 7University of Chinese Academy of Sciences (UCAS), Beijing, China
Mycobacterium tuberculosis remains a leading cause of infectious disease morbidity and mortality for which new drug combination therapies are needed. Mycobacterial bioenergetics has emerged as a promising space for the development of novel therapeutics. Further to this, unique combinations of respiratory inhibitors have been shown to have synergistic or synthetic lethal interactions, suggesting that combinations of bioenergetic inhibitors could drastically shorten treatment times. Realizing the full potential of this unique target space requires an understanding of which combinations of respiratory complexes, when inhibited, have the strongest interactions and potential in a clinical setting. In this review, we discuss (i) chemical-interaction, (ii) genetic-interaction and (iii) chemical-genetic interaction studies to explore the consequences of inhibiting multiple mycobacterial respiratory components. We provide potential mechanisms to describe the basis for the strongest interactions. Finally, whilst we place an emphasis on interactions that occur with existing bioenergetic inhibitors, by highlighting interactions that occur with alternative respiratory components we envision that this information will provide a rational to further explore alternative proteins as potential drug targets and as part of unique drug combinations.
Introduction
M. tuberculosis, the causative agent of tuberculosis (TB), is an obligate human pathogen and significant cause of infectious disease morbidity and mortality, being responsible for an estimated 5.8 million new infections and 1.3 million deaths among HIV-negative people and an additional 214 000 among HIV-positive people (WHO, 2021). Combination drug regimens are favored for the treatment of TB due to their ability to increase efficacy, delay the development of resistance and reduce toxic side effects. Favorable drug interactions are typically the result of either (I) therapeutic synergy, when the effect (i.e. growth inhibition or bacterial killing) of drug combinations is greater than the sum of the individual drugs, or (II) synthetic lethal interactions, when the individual drugs alone are non-lethal, whilst the combination results in killing. Alternatively, some drug combinations serve to prevent the emergence of drug resistance, with additive drugs preventing the isolation of resistant mutants despite not increasing the killing of the partner compound. The need for combination therapies for the treatment of M. tuberculosis is also necessitated by additional factors including (I) the unique cellular structure of M. tuberculosis that makes it inherently tolerant of many antibiotics, (II) the ability of M. tuberculosis to switch into a metabolically inactive state that is phenotypically tolerant to many antibiotics and host-immune responses, and (III) unequal drug penetration at the site of infection (Kerantzas and Jacobs, 2017). As a result, drug-susceptible strains of M. tuberculosis are treated with a frontline combination regimen of four antibiotics for two months followed by four months of isoniazid and rifampicin. Typically the frontline regimen achieves cure rates of 85%. Unfortunately, the prolonged treatment time of available regimens can lead to patient non-compliance, which ultimately drives the acquisition of drug resistance. Consequently, despite the extensive use of combination therapies, there is clinical resistance to all available antibiotics. Treatment options for drug resistant strains are limited, often highly toxic and require even longer treatment times, typically >9 months (Lange et al., 2018), with much lower cure rates. Whilst new regimens, including the BPaL regimen, can reduce treatment length of drug-resistant strains to 6-months, there remains an urgent need for new drug classes and new approaches to the design of combination therapies to prevent drug resistance and further reduce treatment times.
The discovery and subsequent FDA approval of the F1Fo-ATP synthase inhibitor bedaquiline (BDQ) has shifted the focus of many drug-discovery programs towards the development of bioenergetic inhibitors i.e., compounds that target the mycobacterial electron transport chain (ETC) (Andries et al., 2005; Diacon et al., 2014; Cook et al., 2017). The mycobacterial ETC is a series of membrane-bound or membrane-associated enzymes that are responsible for coupling the oxidation of electron donors that are generated from central carbon metabolism to the reduction of oxygen as a terminal electron acceptor. Several of these enzymes are proton pumping, allowing for the establishment of a proton motive force (PMF) that is used to generate ATP via oxidative phosphorylation (Cook et al., 2017; Hards et al., 2020). Several recent studies have highlighted the benefit of combining bioenergetic inhibitors to accelerate the rate of bacterial clearance from various infection models. More importantly, the clinical efficacy of multiple bioenergetic inhibitors is highlighted by the FDA approval of the BPaL combination therapy that consists of two bioenergetic inhibitors and one translational inhibitor (Conradie et al., 2020).
The focus of this review is to highlight recent advances in our understanding of interactions between bioenergetic components and their associated inhibitors. We discuss interactions identified (either synergistic or antagonistic) from chemical, genetic and chemical-genetic and interaction studies. Whilst each strategy has drawbacks, each approach has contributed novel insights.
Respiratory components as targets for bioenergetic inhibitors in M. tuberculosis
Here for context, we provide a brief description of each component that forms the mycobacterial ETC. Whilst the majority of this context is based on results from M. tuberculosis, we do highlight results obtained from the fast-growing model organism Mycobacterium smegmatis. We acknowledge that there may be differences between these species and have highlighted this when it may be a confounding factor. Prior reviews should be consulted for a more detailed discussion of each component and their functions (Cook et al., 2017; Hards and Cook, 2018; Hards et al., 2020; Hasenoehrl et al., 2020).
Mycobacterial electron donating dehydrogenases
The mycobacterial ETC has several primary dehydrogenases that couple the oxidation of respiratory substrates or recycling of redox cofactors from the citric acid cycle to the reduction of the electron carrier menaquinone. Dehydrogenases that donate electrons to the ETC, including glycerol-3-phosphate dehydrogenase, carbon-monoxide dehydrogenase, proline dehydrogenase or L-lactate dehydrogenase are not directly linked to the citric acid cycle and are not discussed in this review, although they are expertly reviewed elsewhere (Rhee et al., 2011; Cook et al., 2014; Cook et al., 2017; Hasenoehrl et al., 2020).
M. tuberculosis has two classes of NADH: menaquinone oxidoreductases that couple the oxidation of NADH to the reduction of menaquinone (Weinstein et al., 2005; Yano et al., 2006). This includes a proton-pumping type I NADH dehydrogenase (NDH-1) and a non-proton pumping type II NADH dehydrogenase (NDH-2) (Figure 1). Prior work has demonstrated that NDH-1, is analogous to mitochondrial Complex-1 and is not required for the growth of M. tuberculosis (Rao et al., 2008; Heikal et al., 2014; Heikal et al., 2018; Petri et al., 2018; Vilcheze et al., 2018; Beites et al., 2019). M. tuberculosis has two copies of NDH-2, encoded by ndh and ndhA (Vilcheze et al., 2018). Genetic studies have demonstrated that ndh and ndhA can be individually deleted, but cannot be simultaneously deleted when grown in the presence of fatty acids (Vilcheze et al., 2018; Beites et al., 2019). Growth in the absence of fatty acids, restores this conditional essentiality (Beites et al., 2019). Somewhat unexpectedly, and in contrast to prior chromosomal deletion mutants, the use of CRISPR interference demonstrated that the transcriptional repression of ndh, but not ndhA, is sufficient to impair the growth of M. tuberculosis (Bosch et al., 2021; McNeil et al., 2021). Furthermore, the transcriptional repression of ndh resulted in bacterial killing in both M. tuberculosis and Mycobacterium smegmatis, a fast-growing non-pathogenic mycobacterial species (McNeil et al., 2022). The discrepancies in phenotypes associated with the genetic inhibition of ndh are likely to be a result of differences in experimental approaches with chromosomal deletion mutants selecting for metabolically adapted strains that are able to grow in the absence of ndh, whilst transcriptional inhibition may observe the immediate effects of inhibiting ndh prior to metabolic adaptation (Weinstein et al., 2005; Yano et al., 2006; Heikal et al., 2016).
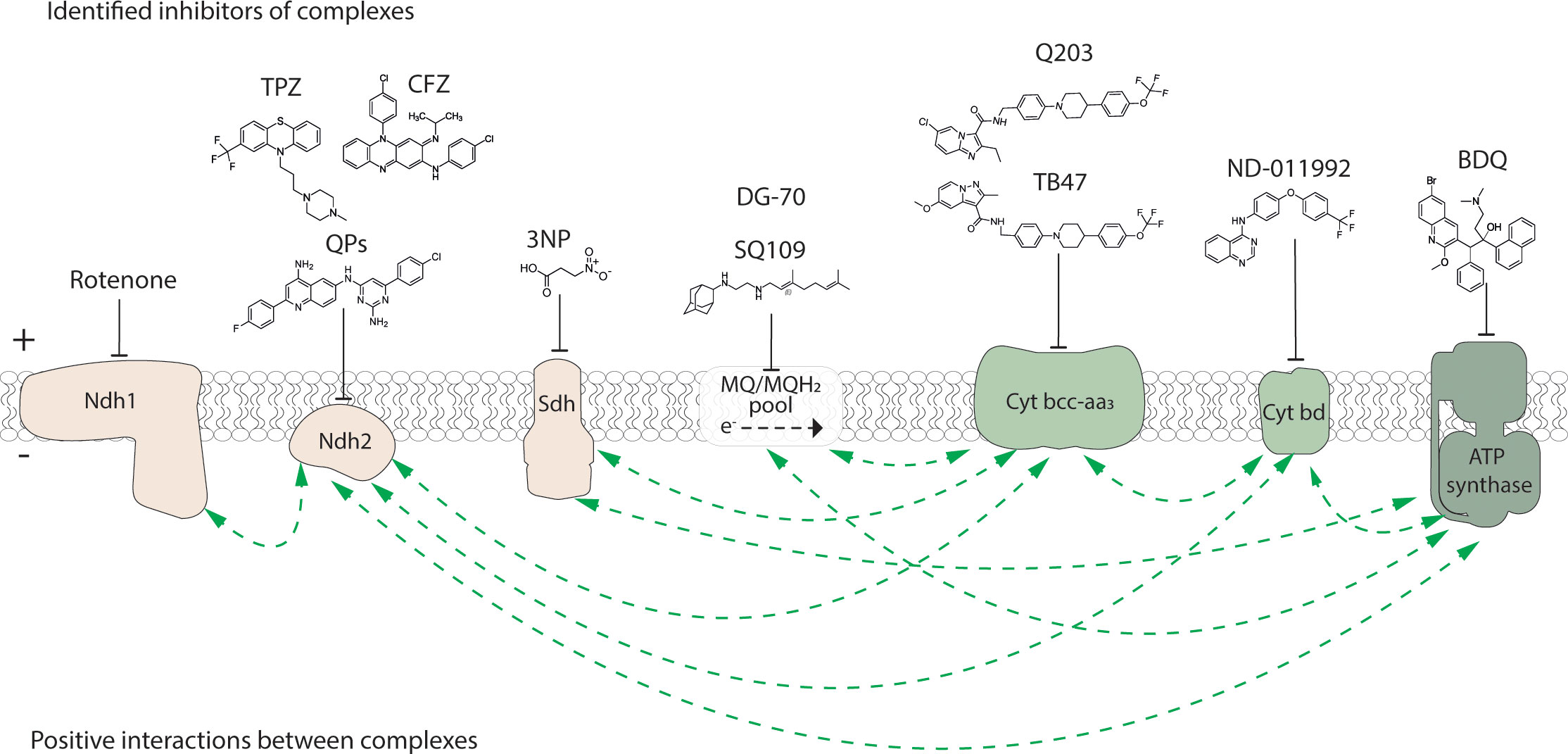
Figure 1 Inhibitors of the electron transport chain and ATP synthase of M. tuberculosis. Known inhibitors of respiratory components, example structures and their targets are illustrated with flathead arrows. Flathead arrow placement does not reflect the specific site of inhibition. The dashed arrow in the MQ/MQH2 pool represents the direction of electron flow. Electron transport chain complexes and the ATP synthase are coloured as: election donors, beige; electron acceptors, green. Plus (+) and minus (-) symbols represent high and low proton concentration gradient across the cytoplasmic membrane, respectively. QPs, quinolinyl pyrimidines; TPZ, trifluoperazine; CFZ, clofazimine; 3NP, 3-nitropropionate; BDQ, bedaquiline. Positive interactions (i.e. synergy or synthetic lethality) between bioenergetic components as reported from either a chemical, genetic or chemical-genetic interaction study and as described in the body of the manuscript are shown with dashed green arrows.
Two mycobacterial succinate dehydrogenase enzymes (i.e., SDH-1 and SDH-2) directly link the citric acid cycle to the ETC, by coupling the oxidation of succinate to the reduction of menaquinone in mycobacterial species (Berney and Cook, 2010; Pecsi et al., 2014; Heikal et al., 2016; Hards et al., 2019; Hards et al., 2020). Prior genetic strategies in M. tuberculosis have demonstrated that each complex can be individually deleted and are functionally redundant as a SDH-1 and SDH-2 double mutant was non-viable (Hartman et al., 2014). Interestingly, deletion of SDH-1 in M. tuberculosis disrupted cellular respiration, accelerated cell death during stationary phase and had a survival defect during chronic infection (Hartman et al., 2014). SDH-2 was essential in M. smegmatis (Pecsi et al., 2014), further supporting the hypothesis that these enzymes perform unique, yet overlapping functions. Definitive evidence that SDH enzymes are indeed important for survival and persistence come from the study of the succinate dehydrogenase suicide inhibitor 3-nitropropionate (3NP) (Eoh and Rhee, 2013). 3NP is a valuable tool for in vitro studies of SDH, but cannot be used to assess the chemical inhibition of SDH in an infection model because of its associated toxicities. Inhibiting M. tuberculosis SDH (SDH-1 or 2 or both) using 3NP resulted in a time-dependent killing of M. tuberculosis during adaptation to hypoxia/persistence (Eoh and Rhee, 2013). Re-aeration of these cultures in the presence of 3NP also inhibited growth. Treatment of non-replicating/hypoxia cultures of M. smegmatis with 3NP leads to cell death via a mechanism that dissipates the membrane potential (Pecsi et al., 2014). Taken together these studies establish that sustained metabolism of succinate through SDH is an essential component of M. tuberculosis metabolic adaptation to hypoxia/persistence and that SDH inhibitors will target these cells. Resolving the mechanisms of functional redundancy and specific functions of each SDH enzyme in combination with recently published structures will assist in the design of chemical inhibitors (Gong et al., 2020; Zhou X. et al., 2021).
Like SDH enzymes, mycobacterial malate:quinone oxidoreductase (MQO) couples the oxidation of malate as part of the citric acid cycle to the reduction of menaquinone (Harold et al., 2022). M. tuberculosis also encodes a malate-dehydrogenases (MDH) that couples the oxidation of malate to the recycling of NAD+ to NADH. CRISPR interference of both mdh and mqo expression in M. tuberculosis impairs growth more severely than either gene knockdown alone (Harold et al., 2022). It has been proposed that exergonic MQO activity powers mycobacterial growth under non-energy limiting conditions, and that endergonic MDH activity complements MQO activity, but at an energetic cost for mycobacterial growth (Harold et al., 2022). M. tuberculosis utilises MDH to facilitate a reductive TCA cycle to produce fumarate (oxaloacetate reduction) for use as a terminal electron acceptor (Watanabe et al., 2011). This provides an alternative electron acceptor for M. tuberculosis in conditions of hypoxia thereby providing a mechanism to maintain the membrane potential and recycle reducing agents. M. tuberculosis can also use Mqo and Mdh in combination to form a futile cycle to provide a pseudo NDH-2-type enzyme that is able to complement the growth of ndh2 mutants (Miesel et al., 1998; Vilcheze et al., 2005). Rittershaus et al. (2018) demonstrated that MDH is required for the metabolism and survival of M. tuberculosis in vitro and in vivo. Moreover, chemical inhibitors of M. tuberculosis MDH had the ability to inhibit growth and rapidly kill hypoxic quiescent cells (non-replicating) in vitro and during infection of the murine lung (Rittershaus et al., 2018). We propose that the addition of an inhibitor of MQO, in combination with MDH inhibitors, may enhance the growth inhibition of M. tuberculosis further while still retaining potent activity against non-replicating populations. In this regard, MQO is not found in mammalian cells and potent inhibitors have been reported that target the mitochondrial malate:quinone oxidoreductase of the malarial parasite Plasmodium falciparum (Hartuti et al., 2018). The significant structural differences reported between the human and mycobacterial MDH enzymes (Rittershaus et al., 2018) suggest a combination therapy of MQO and MDH inhibitors may be attractive for TB drug development.
Mycobacterial electron carriers
Menaquinone is the primary electron carrying lipoquinone in mycobacteria that is responsible for shuttling electrons between respiratory dehydrogenases and terminal oxidases (Cook et al., 2017). Menaquione biosynthesis occurs via a series of enzymes that are similar to the E. coli synthesizing genes menA-J (Cook et al., 2017). Despite the importance of menaquinone biosynthesis in ETC function, not all menaquinone synthesizing genes are designated as essential genes (Dhiman et al., 2009; Griffin et al., 2011; Cook et al., 2017; Bosch et al., 2021; McNeil et al., 2021). Interestingly, targeted transcriptional repression of menA and menD with CRISPR interference had no effect on mycobacterial growth (McNeil et al., 2021). This is in contrast to transposon mutagenesis and whole genome CRISPR-interference studies, in which both genes were designated as being necessary for mycobacterial growth (Griffin et al., 2011; Bosch et al., 2021). Prior studies have demonstrated that the consequences of CRISPRi mediated inhibition of individual metabolic genes can be mitigated by metabolic buffering, in which multiple cell divisions are needed to deplete enzymes or enzyme products below levels needed to produce a bacterial phenotype (Donati et al., 2021). Whether this is the case for menaquione biosynthesis genes in M. tuberculosis requires further investigation. Nevertheless, the absence of menaquinone from humans, makes it an attractive drug target. Multiple small molecules have been identified as inhibitors of various menaqinone biosynthetic enzymes including MenA and MenD (Dhiman et al., 2009; Fang et al., 2010; Debnath et al., 2012; Berube et al., 2019; Bashiri et al., 2020). Further to this, recent metagenomic guided drug discovery efforts recently identified novel antibiotic classes that directly bind menaquinone, several of which had in vitro and ex vivo activity against M. tuberculosis (Li et al., 2022).
Electron accepting terminal oxidases
Under aerobic conditions, a supercomplex of cytochrome bc1 (Complex III; qcrBCD) and an aa3-type cytochrome c oxidase (Complex IV; ctaBCDE), herein referred to as Cyt-bc1aa3, couples the transfer of (i) electrons from reduced menaquinol to cytochrome c to (ii) proton translocation and the generation of a PMF (Gong et al., 2018; Wiseman et al., 2018). Both complexes are essential for mycobacterial growth, yet the deletion or transcriptional inhibition of either complex in M. tuberculosis results in a bacteriostatic phenotype (Beites et al., 2019; Bosch et al., 2021; McNeil et al., 2021; McNeil et al., 2022). Numerous small molecule inhibitors of cytochrome bc1 have been identified (Kang et al., 2014; Lu et al., 2019; Yu et al., 2021; Zeng et al., 2021), of which Q203 (Telacebec) is the most advanced through clinical trials (Pethe et al., 2013). Structural studies have demonstrated that Q203 binds to the menaquinone binding site, effectively blocking menaquinone oxidation (Zhou S. et al., 2021). This mechanism of action is supported by resistance against Q203 and the majority of other Cyt-bc1aa3 inhibitors being at residues near the menaquinone-binding site (Liu et al., 2019; Lu et al., 2019; Yanofsky et al., 2021; Zhou S. et al., 2021).
Cytochrome bd-type menaquinol oxidase (Cyt-bd) is an alternative non-proton pumping high-affinity terminal oxidase that is only found in Prokaryotes (Borisov et al., 2021). From an energetic point of view, Cyt-bd is considered less efficient as it does not translocate protons. Despite this, Cyt-bd is able to generate a PMF by transmembrane charge separation with an H+/e ratio of 0.94 ± 0.18 (Borisov et al., 2011). Cyt-bd is encoded by cydAB as part of the cydABDC operon, with both genes being non-essential for mycobacterial growth (McNeil et al., 2021). Because of the reduced efficiency but higher affinity, Cyt-bd functions predominantly under low oxygen conditions in vitro (Kana et al., 2001; Berney and Cook, 2010; Aung et al., 2014) and in vivo (Shi et al., 2005; Cai et al., 2021). Cyt-bd becomes essential for mycobacterial growth when the Cyt-bc1aa3 supercomplex is deleted or expression inhibited (Matsoso et al., 2005; Arora et al., 2014; Lu et al., 2015; Kalia et al., 2017; Lu et al., 2018; Beites et al., 2019; Lee et al., 2019; Bosch et al., 2021; Hards et al., 2022).
Mycobacterial F1Fo ATP synthase
The PMF generated by the mycobacterial ETC is used by the F1Fo-ATP synthase to generate ATP from ADP and inorganic phosphate. The mycobacterial F1Fo-ATP synthase is encoded by the of atpBEFHAGDC operon, with all genes being essential for mycobacterial growth (Griffin et al., 2011; Zhang et al., 2012; Bosch et al., 2021; McNeil et al., 2021) even on fermentable carbon sources like glucose (Tran and Cook, 2005). Furthermore, the transcriptional repression of atpE and atpB not only inhibited mycobacterial growth, but resulted in rapid bacterial killing (McNeil et al., 2020). BDQ (Sirturo®) was approved by the US FDA in 2012 for the treatment of adults with pulmonary MDR-TB (Diacon et al., 2012; Cohen, 2013; Diacon et al., 2014). BDQ is generally bactericidal and can kill drug-resistant mycobacterial species and dormant bacilli (Diacon et al., 2012; Diacon et al., 2014). It acts quickly compared to most TB drugs, but still requires many weeks of therapy and BDQ-resistance has been reported, including in treatment-naïve populations (Andries et al., 2014; Villellas et al., 2017). BDQ targets the F1Fo-ATP synthase of M. tuberculosis and binds to the c-subunit rotor in the membrane-embedded part of the F1Fo-ATP synthase (Andries et al., 2005a; Preiss et al., 2015) decreasing intracellular ATP levels (Koul et al., 2007; Koul et al., 2008; Koul et al., 2014). Structural analysis demonstrated that BDQ binds to ATP synthase ‘leading’ and ‘lagging’ sites of subunit c and to a lesser degree subunit a, resulting in major conformational changes in mycobacterial ATP synthase (Guo et al., 2021; Montgomery et al., 2021). BDQ mediated inhibition of ATP synthesis also has downstream effects on a number of metabolic processes including the inhibition of glutamine synthetase (Wang et al., 2019) and the rerouting of mycobacterial metabolism that places an increased reliance on glycolysis and substrate level phosphorylation to generate cellular ATP (Mackenzie et al., 2020). BDQ also dissipates the ΔpH component of the PMF in mycobacteria (Hards et al., 2015; Hards et al., 2018). This depends on target-based accumulation of BDQ and leads to an uncoupled microenvironment around the F1Fo-ATP synthase (Hards et al., 2018). Several new inhibitors of the mycobacterial F1Fo-ATP synthase have been identified (Singh et al., 2015; Tantry et al., 2017; Narang et al., 2019; Kamariah et al., 2020; Hards et al., 2022). However, further studies are needed to determine their clinical safety and efficacy.
Interactions with mycobacterial respiratory components
Mycobacterial respiratory components have emerged as a promising target space, not only for the development of novel therapeutics, but also as part of unique combination therapies. This is due to the observation that the inhibition of many mycobacterial respiratory components have positive interactions, not only with other respiratory components, but other cellular processes in general. For the remainder of this review, we describe each of these interactions and provide possible mechanistic explanations. We present interactions identified from either chemical, genetic and chemical-genetic interaction studies. We consider both (i) synergistic interactions (i.e. when the sum of the outcome is greater than the individual components) and (ii) synthetic lethal interactions, (i.e. when the loss/inhibition of neither component is lethal but combined results in cell death) as being “positive interactions”. We also discuss examples of antagonism, as identifying the basis for these unfavorable interactions will aid in the development of improved combination therapeutics. Whilst the following sections place an emphasis on interactions with clinically advanced inhibitors and their targets, i.e. BDQ, Q203 and pretomanid, we also discuss possible interactions between other respiratory components in the hope that this will highlight priority targets for future drug development.
Interactions with the F1Fo-ATP synthase and the inhibitor BDQ
Numerous inhibitors of the mycobacterial F1Fo-ATP synthase have been identified (Singh et al., 2015; Tantry et al., 2017; Narang et al., 2019; Hotra et al., 2020; Kamariah et al., 2020; Denny, 2021; Choi et al., 2022; Hards et al., 2022), of which BDQ is the most clinically advanced and was FDA approved for the treatment of multi-drug resistant M. tuberculosis in 2012 (Diacon et al., 2012; Cohen, 2013; Diacon et al., 2014). The bioenergetic consequences of inhibiting the F1FO-ATP synthase have resulted in ATP synthase inhibitors, including BDQ, having favorable interactions with other respiratory inhibitors. For example, several studies have demonstrated interactions between simultaneous inhibition of menaquinone biosynthesis and ATP synthase function (Sukheja et al., 2017; Berube et al., 2019). Inhibitors of MenA, which catalyzes the penultimate step in menaquinone biosynthesis had a synergistic killing interaction with BDQ, resulting in complete sterilization of M. tuberculosis cultures within 14 – 21 days of treatment (Berube et al., 2019). The synergy between inhibition of menaquinone biosynthesis and the ATP synthase was not observed in genetic studies, although this may be attributable to variations in model organism (i.e. M. smegmatis vs M. tuberculosis) or experimental approach (i.e. genetic-genetic interactions vs chemical-chemical interactions) (Sukheja et al., 2017; Berube et al., 2019; McNeil et al., 2022).
The non-proton pumping type II NADH dehydrogenase (NDH-2) is essential for mycobacterial growth. Phenothiazine-like compounds including CFZ have long been thought to kill through a redox cycle involving reduction by NDH-2 and spontaneous re-oxidation by O2, producing toxic levels of ROS (Yano et al., 2006; Yano et al., 2011; Heikal et al., 2016). As BDQ stimulates the respiration rate of M. tuberculosis (Hards et al., 2015; Lamprecht et al., 2016; Hards and Cook, 2018; Hards et al., 2018), this results in an accumulation of reducing equivalents that potentiates CFZ reduction, increasing ROS production. This drives the rapid sterilization of M. tuberculosis in vitro and in macrophages when BDQ and CFZ are used in combination (Lamprecht et al., 2016; Berube and Parish, 2018). Genetic studies have also reported synergistic interactions between the mycobacterial NDH-2 and the ATP synthase (McNeil et al., 2022). CRISPRi knockdown of ndh and atpE in M. smegmatis is bactericidal by itself and thus prevents the identification of synergistic interactions (McNeil et al., 2022). McNeil et al. (2022) overcame this by engineering sub-optimal sgRNAs that produced a bacteriostatic phenotype. This allowed for the identification of interactions between genes that when knocked down in combination, resulted in cell death (McNeil et al., 2022). This multiplexed approach identified a synthetic lethal interaction between NDH-2 and the ATP synthase that killed M. smegmatis (McNeil et al., 2022). Further to this, an NDH-2 conditional mutant in M tuberculosis was also more sensitive to growth inhibition by BDQ (Beites et al., 2019).
Chemical and genetic interaction studies have failed to show an interaction between inhibition of the ATP synthase and primary terminal oxidase Cyt-bcc-aa3 (Lamprecht et al., 2016; Beites et al., 2019; McNeil et al., 2022). Despite this, deletion of the alternative terminal oxidase Cyt-bd has been shown to increase sensitivity of M. tuberculosis to killing by BDQ (Berney et al., 2014; Kalia et al., 2017). This interaction wasn’t reproduced in genetic studies, again possibly due to differences in experimental approach or model system (McNeil et al., 2022).
Given the cellular importance of ATP, it is surprising that following BDQ exposure metabolic remodeling allows mycobacterial to initially tolerate BDQ, with minimal killing observed in the first five days of exposure (Koul et al., 2014; Hards et al., 2015). However, disrupting the metabolic response to BDQ can remove this initial tolerance and sensitize M. tuberculosis to rapid killing. For example, substrate level phosphorylation is prioritized for the generation of ATP production following BDQ exposure (Mackenzie et al., 2020). As such genetic inhibition of glycolysis by deleting pyruvate kinase pykA increased BDQ lethality, sterilizing cultures within 5-6 days. Interestingly, this synergy between glycolysis and ATP synthase inhibition only occurred at specific glycolytic nodes, with deletion of the first rate-limiting site in glycolysis, i.e. phosphofructokinase not increasing BDQ lethality. BDQ exposure also has downstream effects on cellular glutamine levels, with the depletion of ATP limiting the ability of glutamine synthetase to convert glutamate into glutamine (Wang et al., 2019). Reduced glutamine levels suggested that BDQ treated cells would have increased susceptibility to the targeted inhibition of glutamine synthetase. Consequently, BDQ synergized with methionine sulfoximine, an inhibitor of glutamine synthetase resulting in increased BDQ lethality (Wang et al., 2019). BDQ has also been shown to synergize with inhibitors of mycobacterial stress response and cell wall synthesis (Lechartier et al., 2012; Wang et al., 2019; Yang et al., 2019).
The most promising combination therapy involving BDQ, is the BPaL regime, consisting of BDQ, pretomanid and linezolid. BPaL is an all oral regime, that when utilized over a 6 month period achieve 90% favorable outcomes in patients with extensively drug-resistant or non-responsive multi-drug resistant M. tuberculosis (Conradie et al., 2020). Whilst the BPaL regimen has since been FDA approved, BDQ has significant toxicity issues including hepatotoxicity, irregular cardiac rhythm, and increases in blood bilirubin (Moodliar et al., 2021; Yao et al., 2022). There are also concerns associated with BDQ resistance, which is the result of (i) mutations in the transcriptional regulator Rv0678 that up-regulate the expression of the efflux pump MmpL5 or (ii) in residues of ATP synthase that prevent BDQ binding. Clinical resistance to BDQ via mutations in the transcriptional regulator Rv0678 have already been reported, even in treatment naïve populations prior to the inclusion of BDQ (Huitric et al., 2010; Andries et al., 2014; Hartkoorn et al., 2014; Villellas et al., 2017).
Inhibitors of the mycobacterial F1Fo-ATP synthase are promising anti-tubercular agents, particularly given their ability to synergize with a range of other inhibitors suggesting that they could form the backbone of novel therapeutic regimens. Whilst BDQ highlights the potential for this class of compound, issues with toxicity and resistance highlight the need for improved derivatives or completely new chemical classes of ATP synthase inhibitors.
Interactions with cytochrome oxidase bcc-aa3 oxidase and the inhibitor Q203
M. tuberculosis respires oxygen via two terminal respiratory oxidases, Cyt-bcc-aa3 and Cyt-bd (Cook et al., 2017). Numerous small molecule inhibitors of the cytochrome bc1 complex have been identified (Abrahams et al., 2012; Pethe et al., 2013; Kang et al., 2014; Lu et al., 2019; Yanofsky et al., 2021; Zhou S. et al., 2021), of which Q203 (aka Telacebec) is the most advanced through clinical trials whilst TB47 is in preclinical development (Pethe et al., 2013; Kang et al., 2014; Lu et al., 2019). M. tuberculosis is able to control the flow of electrons to either oxidase providing metabolic flexibility when either oxidase is deleted or inhibited (Lamprecht et al., 2016). This has been demonstrated by the ability to obtain gene deletions of Cyt-bcc-aa3 or Cyt-bd independently, whilst the chemical or genetic inhibition of the Cyt-bcc-aa3 stops growth but the cells remain viable (bacteriostatic) (Beites et al., 2019; Bosch et al., 2021; McNeil et al., 2021; McNeil et al., 2022). Despite this, various studies have demonstrated that blocking this functional redundancy results in improved inhibitory outcomes. For example, chemical-genetic studies demonstrated that the deletion of one terminal oxidase, increases susceptibility to inhibition of the alternative, with mutants lacking Cyt-bcc-aa3 having increased susceptibility to inhibitors of Cyt-bd (Lu et al., 2018; Lee et al., 2021), and mutants lacking Cyt-bd having increased susceptibility to inhibitors of Cyt-bcc-aa3 (Arora et al., 2014; Lu et al., 2015; Kalia et al., 2017; Moosa et al., 2017; Liu et al., 2019; Lu et al., 2019; Chong et al., 2020). These positive interactions are supported by the observation that Q203 completely inhibits respiration in the Cyt-bd mutant (Lamprecht et al., 2016). Further to this, the simultaneous inhibition of both terminal oxidases has been shown to have synthetic lethal phenotypes, resulting in cell death (Kalia et al., 2017; Lu et al., 2018; Liu et al., 2019; Lee et al., 2021). Importantly, this bactericidal outcome of dual terminal oxidase inhibition is conserved when using diverse carbon sources under replicating conditions, under non-replicating conditions and in murine models of infection (Kalia et al., 2017; Lee et al., 2021; McNeil et al., 2022). Subsequently, this has spurred interest in identifying and developing improved inhibitors of Cyt-bd. Recent examples include ND-011992 (Lee et al., 2021), MQL-H2 (Harikishore et al., 2020) and HM2-16F (Hards et al., 2022), all of which have synthetic lethal interactions with Q203. The less-than-optimal pharmacokinetic properties of ND-011992 make it unsuitable for development and alternative chemical scaffolds targeting cytochrome bd are required (Lee et al., 2019). The recent high-resolution structures of cytochrome bd from M. tuberculosis and M. smegmatis now provide a detailed molecular framework for the discovery of new bd inhibitors (Safarian et al., 2021; Wang et al., 2021). Interestingly, in Mycobacterium ulcerans, which has a non-functional Cyt-bd and relies exclusively on Cyt-bcc-aa3 as a terminal oxidase, inhibitors of Cyt-bcc-aa3 are bactericidal (Scherr et al., 2018; Liu et al., 2019). Alternatively, naturally occurring polymorphisms in the Cyt-bcc-aa3 of Mycobacterium abscessus provide a high level of resistance to Q203 (Sorayah et al., 2019). Combined, this data demonstrates that starving M. tuberculosis, and potentially other mycobacterial species, of their ability to use oxygen as a terminal electron acceptor can result in bactericidal outcomes and is a vulnerable target for drug discovery (Bajeli et al., 2020; Lee B. S. et al., 2020; Sviriaeva et al., 2020).
Menaquinone is the primary electron carrier in M. tuberculosis (Dhiman et al., 2009; Debnath et al., 2012; Cook et al., 2017). Interestingly, the majority of Cyt-bc1aa3 inhibitors bind to the menaquinone binding site to block menaquinone oxidation (Lu et al., 2019; Yanofsky et al., 2021). Studies have demonstrated interactions between simultaneous inhibition of menaquinone biosynthesis and inhibition of Cyt-bcc-aa3 (Berube et al., 2019). Inhibitors of MenA, which catalyzes the penultimate step in menaquinone biosynthesis, synergized with a cytochrome bc1-aa3 inhibitor (Berube et al., 2019). The synergy between inhibition of menaquinone biosynthesis and the cytochrome bc1-aa3 terminal oxidase was further demonstrated in genetic studies, where the simultaneous transcriptional repression of menD and qcrB resulted in stronger growth inhibition than the single knockdown of either gene alone (McNeil et al., 2022). The inhibition of menaquinone biosynthesis not only reduces available menaquinone, but it is also likely to reduce the levels of intracellular ATP needed to sustain growth and survival (Dhiman et al., 2009; Sukheja et al., 2017). This coupled with the bioenergetic effects of Cyt-bcc-aa3 inhibitors that ultimately lead to a reduction in the intracellular levels of ATP would result in target synergy and bacterial cell death.
Several studies have also reported synergistic interactions between the mycobacterial NADH dehydrogenase type II enzymes (NDH-2) and the Cyt-bcc-aa3 terminal oxidase in mycobacteria (Beites et al., 2019; McNeil et al., 2022). CRISPRi knockdowns have uncovered a synthetic lethal interaction between NDH-2 and the Cyt-bcc-aa3 that killed M. smegmatis faster than the dual knockdown of qcrB and cydB on both fermentable and non-fermentable carbon sources (McNeil et al., 2022). Studies have also reported synergistic killing between CFZ, a proposed inhibitor of NDH-2 and inhibitors of Cyt-bcc-aa3 including Q203 and phenoxy alkyl benzimidazoles (PABs) under both replicating and non-replicating conditions (Lamprecht et al., 2016) (Berube and Parish, 2018). However, there are conflicting reports regarding this interaction as the deletion of ctaE-qcrCAB did not alter sensitivity to growth inhibition by NDH-2 inhibitors, including CFZ (Beites et al., 2019). It should be noted, that the same authors conclude that CFZ does not require NDH-2 to inhibit the growth of M. tuberculosis as conditional inactivation of NDH-2 (i.e. ndhA + ndh double mutant) in M. tuberculosis had no effect on the growth inhibitory properties of CFZ, (Beites et al., 2019). Between these various studies, differences in (i) biological readouts, i.e. bacterial viability vs growth inhibition, (ii) experimental approaches of gene deletion compared to gene knockdown and (iii) experimental model i.e. M. tuberculosis or M. smegmatis may go some way to explaining these reported differences. Despite this, we conclude that there is sufficient evidence to suggest that whilst targeting NDH-2 may not synergize with the growth inhibitory properties of Cyt-bcc-aa3 inhibition, they may offer another potential avenue to enhance the bactericidal activity of the normally bacteriostatic Cyt-bcc-aa3 inhibitors.
Cyt-bcc-aa3 inhibition also synergizes with the first line M. tuberculosis drugs PZA (PZA) and rifampicin (RIF) in a mouse model (Lu et al., 2019). Specifically, the Cyt-bcc-aa3 TB47 inhibitor exhibited a potent synergy with subtherapeutic doses of PZA and RIF, causing a 4- and 5-fold reduction in lung CFU compared to the respective monotherapy (Lu et al., 2019). Here, it was proposed that a disruption to the NADH/NAD+ ratio by TB47 may explain the synergy of TB47 with RIF and PZA. TB47 also exhibited a highly unique synergistic activity with clarithromycin in vitro and in mouse models against different mycobacterial species (Liu et al., 2020; Yu et al., 2020). TB47-containing 3-drug regimens cured Buruli ulcer in vivo in ≤ 2 weeks when dosed daily or in ≤ 3 weeks when dose twice per week (6 doses in total) compared to the current first-line treatment of RIF and streptomycin which required 8 weeks and had 26.67% mice relapsing post treatment (Gao et al., 2021).
The bioenergetic consequences of Cyt-bcc-aa3 inhibition, that lead to reduced ATP levels and severely impaired bacterial growth have also been shown to antagonise the bactericidal activities of the anti-tubercular drugs isoniazid (INH) and moxifloxacin (Lee et al., 2019). Specifically, the metabolic downregulation following Q203 exposure dissipated the transient increase in intracellular respiration that is associated with cell death and was observed following INH or moxifloxacin exposure alone (Lee et al., 2019). This is consistent with the antagonism frequently seen between bactericidal and bacteriostatic antibiotics (Ocampo et al., 2014). Interestingly, a known efflux pump inhibitor verapamil increased the potency of Q203 against M. tuberculosis, indicating that the upregulation of efflux pumps may be associated with low level resistance to Q203 (Jang et al., 2017). This further highlights how an improved understanding of the mechanisms of antibiotic mediated mycobacterial death are essential to the improved design of optimized combination therapies.
Interactions between other mycobacterial respiratory components
There are many bioenergetic components in M. tuberculosis for which there are no bona-fide small molecule inhibitor with favorable pharmacological properties. Given the resource investment required to advance small molecule through the drug development pipeline, it is essential that high priority targets with the greatest chance of clinical success when used in combination therapies are identified and validated. Here we discuss interactions between other mycobacterial respiratory components, specifically NADH dehydrogenase, succinate dehydrogenase, malate dehydrogenase and menaquinone biosynthesis. We acknowledge that there may be is overlap with prior sections.
NADH dehydrogenase inhibitor interactions
A synthetically lethal interaction exists between the two different NADH dehydrogenase enzymes (NDH-1 and NDH-2) in M. tuberculosis, with the deletion of NDH-2 (ΔndhΔndhA) rendering M. tuberculosis susceptible to killing by NDH-1 inhibitors such as rotenone, which normally has no effect on the growth of M. tuberculosis (Beites et al., 2019). Of the two enzymes, NDH-2 is being actively pursued as a drug target as it is the primary mycobacterial NADH dehydrogenase (Weinstein et al., 2005; Shirude et al., 2012; Dunn et al., 2014; Harbut et al., 2018; Vilcheze et al., 2018; Beites et al., 2019) and is absent from mammalian mitochondria. To date, two main classes of compound interact with the mycobacterial NDH-2 enzymes: the phenothiazines [e.g., thioridazine (THZ), chlorpromazine (CPZ)] and CFZ, and both compounds have a range of positive interactions reported both in vitro and in vivo. For example, THZ and CPZ have been shown to enhance the activity of TB antibiotics including RIF and streptomycin (Crowle et al., 1992; Viveiros and Amaral, 2001).
While not technically an inhibitor of NDH-2, the mechanism of action of CFZ is linked to NDH-2 activity (Yano et al., 2011). CFZ is thought to kill through via a NDH-2 recycling mechanism that produces toxic levels of ROS (Yano et al., 2011). Accordingly, inhibitors that potentiate CFZ reduction and thus ROS production are synergistic with CFZ. Has highlighted from prior sections, this includes both BDQ and Q203 (Lamprecht et al., 2016). Inhibition of the Cytbc1-aa3 oxidase with phenoxy alkyl benzimidazoles (PABs) synergized with CFZ under replicating and non-replicating conditions (Berube and Parish, 2018). These interactions are consistent with genetic studies, showing that the inactivation of ndh with either atpE or qcrB having a bactericidal phenotype (McNeil et al., 2022). Moreover, CFZ has been previously shown to directly compete with menaquinone for reduction by NDH-2 and, as such, menaquinone biosynthesis inhibitors synergize strongly with CFZ to kill M. tuberculosis (Berube et al., 2019). Interestingly, deletion of cytochrome bd synergized with NDH-2 inhibition in M. tuberculosis (Beites et al., 2019) and the deletion of cytochrome bd sensitized M. smegmatis to killing by CFZ at concentrations that were bacteriostatic against wild-type (Lu et al., 2015). This is despite no interaction being observed between the dual knockdown of cydB and ndh or nuoD in M. smegmatis (McNeil et al., 2022). These differences may be partly explained by choice of model system, experimental approach, or recent evidence suggesting that CFZ doesn’t require NDH-2 for inhibitory activity against M. tuberculosis (Beites et al., 2019). Further, studies in murine infection models have shown that CFZ synergizes with RIF, INH and PZA to halve the treatment period for drug-sensitive M. tuberculosis (Li et al., 2017) as well as potentiating the activity of second-line drug regimens against drug-resistant M. tuberculosis (Grosset et al., 2013). This suggests that targeting NDH-2 offers another potential avenue to enhance the activity of the normally bacteriostatic Cytbc1-aa3 inhibitors although further work is required to reconcile some experimental differences.
While several promising synergies have been identified between inhibiting the mycobacterial NADH dehydrogenases and other respiratory complexes, it is also worth noting that there is also the potential for antagonistic interactions to occur, particularly between INH and CFZ. Mutations in ndh have previously been shown to confer INH resistance in M. tuberculosis, M. smegmatis, and M. bovis BCG (Miesel et al., 1998; Lee et al., 2001; Vilcheze et al., 2005), and both targeted protein depletion and chemical inhibition of NDH-2 attenuate INH activity in M. tuberculosis (Harbut et al., 2018; Koh et al., 2022). This antagonism is postulated to be due to alterations in the NADH/NAD+ ratio preventing the formation of the active INH-NAD adduct or competing with its binding to the NADH-dependent enoyl-ACP reductase, InhA (Miesel et al., 1998; Lee et al., 2001; Vilcheze et al., 2005; Koh et al., 2022). Despite this, genetic deletion of ndh and ndhA alone or in combination did not alter INH susceptibility in M. tuberculosis, which was attributed to insufficient NADH accumulation in these mutants to affect INH activity (Vilcheze et al., 2018; Beites et al., 2019). Nevertheless, future NDH-2 inhibitors should be investigated for their potential interactions with INH. Likewise, as CFZ is activated by NDH-2, interactions between NDH-2 inhibitors and CFZ should be evaluated. Importantly, two recent studies demonstrated that CFZ retains bactericidal activity against M. tuberculosis in the absence of NDH enzymes (Vilcheze et al., 2018; Beites et al., 2019), suggesting that antagonism with NDH-2 inhibitors is unlikely as other mechanisms of CFZ activation or activity exist independently of NDH-2.
SDH and MQO/MDH inhibitor interactions
Whereas the NADH dehydrogenases inhibitor interactions have been well studied, the remaining electron-donating complexes are comparatively under studied and under explored as drug targets. A recent study showed that inhibition of SDH activity using CRISPRi sensitizes M. tuberculosis to growth inhibition and killing by BDQ and the cytochrome bcc-aa3 inhibitors, Q203 and TB47 (Adolph et al., 2022). Likewise, knockdown of qcrB or ctaC with sdhA2 synergized in M. smegmatis (McNeil et al., 2022). Moreover, when gene knockdown was induced simultaneously with drug treatment, impaired succinate oxidation prevented the emergence of INH and PA-824(pretomanid) resistance, suggesting that SDH inhibitors may also be effective anti-resistance agents when used in combination with INH or pretomanid (Adolph et al., 2022). However, when SDH enzymes were pre-depleted prior to antibiotic challenge an antagonistic interaction was observed with both INH and pretomanid and the other cell wall inhibitors tested; ethionamide (ETH), EMB and SQ109 (Adolph et al., 2022). This is similar to the observations that BDQ and Q203 can attenuate the bactericidal activity of INH, EMB and ETH by preventing a drug-induced lethal ATP burst (Shetty and Dick, 2018; Lee et al., 2019; Zeng et al., 2021). Given the absence of specific SDH inhibitors, it remains to be determined which of these interactions are observed at the chemical level.
Inhibition of the two malate oxidizing enzymes, MDH and MQO, using CRISPRi synergizes to significantly impair the growth of M. tuberculosis compared to the knockdown of either gene alone (Harold et al., 2022). Interactions beyond this have yet to be investigated. However, it is possible that MDH or MQO inhibitors would synergize with NDH inhibitors as the net reaction of MQO and MDH acting in concert is the same as the NADH dehydrogenase reaction (Blaza et al., 2017), suggesting these three enzymes may be functionally redundant (Molenaar et al., 2000). Consistent with this, MDH was shown to be able to compensate for ndh mutants in M. smegmatis (Miesel et al., 1998; Vilcheze et al., 2005) and CRISPRi studies showed a weakly additive interaction between the knockdown of ndh and mqo in M. smegmatis (McNeil et al., 2022). However, these interactions require further investigation in M. tuberculosis.
Menaquinone biosynthesis inhibitor interactions
Several studies have demonstrated that chemical or genetic inhibition of menaquinone biosynthesis sensitizes mycobacteria to further inhibition of various other respiratory complexes (Sukheja et al., 2017; Berube et al., 2019; McNeil et al., 2022). Specifically, inhibition of MenA, which catalyzes the penultimate step in menaquinone biosynthesis, synergized with sub-bactericidal concentrations of BDQ, CFZ and the cytochrome bcc-aa3 inhibitor, ND-10885, resulting in complete sterilization of M. tuberculosis cultures within 14 - 21 days of treatment (Berube et al., 2019). Likewise, inhibition of the terminal biosynthetic enzyme, MenG, with the biphenyl amide DG70 also synergized with BDQ, again sterilizing cultures within 21 days (Sukheja et al., 2017). DG70 displayed further synergy with pretomanid, sterilizing cultures of M. bovis BCG within 10 days, as well as having an additive interaction with the NDH-2 inhibitor, THZ (Sukheja et al., 2017).
As previously highlighted, the synergy between inhibition of menaquinone biosynthesis and the cytochrome bc1-aa3 terminal oxidase was genetically validated (McNeil et al., 2022). Interestingly, CRISPRi did not recreate the synergy between BDQ (atpE knockdown) and menaquinone biosynthesis inhibition (menD knockdown), and the synthetic lethality between ndh knockdown (representing CFZ and THZ interactions) and menD was only observed when glycerol was used as the primary carbon source (McNeil et al., 2022). This is likely due to insufficient gene knockdown using CRISPRi or metabolic buffering of residual menaquinone preventing an observable interaction (Donati et al., 2021; McNeil et al., 2022). Nevertheless, disruption of menaquinone biosynthesis appears to be broadly synergistic with other bioenergetic inhibitors in M. tuberculosis. Mechanistically, this may be explained by the fact that MenA and MenG inhibitors completely block mycobacterial oxygen consumption (Dhiman et al., 2009; Sukheja et al., 2017) and reduce intracellular ATP levels by > 50%, which may sensitize M. tuberculosis to further perturbations of ETC activity, resulting in lethal disruptions to ATP synthesis and PMF generation.
Beyond the respiratory chain, inhibition of MenG with DG70 has also been shown to synergize with RIF, as well as significantly enhancing the activity INH (Sukheja et al., 2017). The dual treatment of log phase cultures of M. bovis BCG with INH and DG70 rapidly increased the rate of killing compared to either drug alone and sterilized cultures within 10 days (Sukheja et al., 2017). As previously highlighted, inhibiting respiration by targeting the ATP synthase or cytochrome bcc-aa3 terminal oxidase prevents INH killing of M. tuberculosis and M. bovis BCG (Shetty and Dick, 2018; Lee et al., 2019; Zeng et al., 2021), raising concerns that bioenergetic inhibitors may have unintended antagonistic interactions with conventional TB drugs. However, the potent synergy between DG70 and INH demonstrates that this is not always the case and provides an alternative approach to retaining the benefits of bioenergetic inhibitors (i.e., bactericidal activity under replicating and non-replicating conditions) while avoiding antagonistic interactions with current TB drugs. Defining the mechanisms for these different interactions will allow for further improvements in the design of combination therapies involving bioenergetic inhibitors.
In addition to specific inhibitors of the menaquinone biosynthesis pathway, SQ109, which is undergoing clinical development for the treatment of drug-resistant M. tuberculosis, has also reported to target both MenA and MenG (Li et al., 2014), in addition to functioning as an uncoupler and MmpL3 inhibitor (Feng et al., 2015). SQ109 synergizes with INH and RIF in vitro and in chronic mice models of infection (Chen et al., 2006; Nikonenko et al., 2007) providing another example of potential synergies that can be achieved by targeting menaquinone biosynthesis. However, given the multiple mechanisms of action of SQ109, it is unclear to what degree inhibition of menaquinone biosynthesis contributes to this synergy.
Overall, menaquinone biosynthesis inhibitors offer a broad range of synergies and avoids antagonistic interactions seen by some bioenergetic inhibitors suggesting they could be efficacious in a broad range of regimens. Particularly promising is the ability to enhance the activity of currently available bioenergetic inhibitors, namely BDQ, pretomanid and terminal oxidase inhibitors Q203.
Interactions with the F420-dependent respiratory inhibitor pretomanid
Pretomanid is a bicyclic nitroimidazole derivative that was discovered when testing 3-substituted bicyclic nitroimidazole-containing compounds for antitubercular activity (Stover et al., 2000; Manjunatha et al., 2006a; Manjunatha et al., 2009). Treatment with pretomanid in vitro is bactericidal at sub-micromolar concentrations in M. tuberculosis and it shows a narrow spectrum of activity that ranges from low to no activity in non-tubercular Mycobacteria (Stover et al., 2000; Manjunatha et al., 2006a; Manjunatha et al., 2006b; Manjunatha et al., 2009; Zheng et al., 2022). Whilst not a strict bioenergetic inhibitor, pretomanid kills both replicating as well as hypoxic non-replicating bacilli by targeting both energy production and cell wall synthesis of M. tuberculosis (Singh et al., 2008; Manjunatha et al., 2009). Catalysis of pretomanid from a prodrug to an active form is carried out by the F420-dependent nitroreductase Ddn (Manjunatha et al., 2006a; Manjunatha et al., 2009; Lee B. M. et al., 2020). Ddn is suggested to protect against oxidative stress by reduction of quinones to dihydroquinones, which avoids the cytotoxic effects of semiquinones (Gurumurthy et al., 2012; Gurumurthy et al., 2013). Resistance to pretomanid is through mutations in genes essential for F420 synthesis or recycling pathways including ddn, fgd1, or the fbi gene products (Lee B. M. et al., 2020; Gomez-Gonzalez et al., 2021), all of which are non-essential under replicating conditions (Griffin et al., 2011). No cross-resistance to bicyclic nitroimidazole has been observed with any other class of anti-tubercular drug (Stover et al., 2000; Matsumoto et al., 2006).
The bactericidal activity of pretomanid is driven by the respiratory poisoning and inhibition of mycolic acid synthesis (Stover et al., 2000; Singh et al., 2008). Transcriptional profiling of pretomanid-treated M. tuberculosis under aerobic conditions showed dysregulation of genes controlling cell wall synthesis such as the iniBAC operon, as well as genes that respond to respiratory poisoning such as the cydABDC operon (Boshoff et al., 2004). This respiratory poisoning has differing effects on replicating and non-replicating cells: non-replicating cells under hypoxic conditions are unable to exit dormancy and replicating cells are unable to synthesize the cell wall. Similar mechanisms of killing of pretomanid have been observed in studies of the FDA approved drug delamanid that is also a bicyclic nitroimidazole (Matsumoto et al., 2006; Xavier and Lakshmanan, 2014). Metabolomics of pretomanid-treated M. smegmatis showed the additional accumulation of phosphate sugars, consistent with reduced FGD1 activity in the pentose-phosphate pathway, leading to cell arrest through accumulation of the toxic metabolite methylglyoxal that modifies peptides and DNA (Murata-Kamiya and Kamiya, 2001; Thornalley et al., 2010; Baptista et al., 2018). This multi-target phenotype of pretomanid suggests that it has the potential to synergize with bioenergetic inhibitors in addition to inhibitors that target cell wall synthesis.
Pretomanid was FDA approved in August 2019 as part of the BPaL regime, an all oral treatment for extensively drug-resistant or treatment non-responsive multi-drug resistant tuberculosis (Keam, 2019). BPaL consists of BDQ, PMD, and linezolid, and in clinical trials showed a favorable results, with 90% of patients achieving a culture negative status (Conradie et al., 2020). The positive results of this combination suggests that pretomanid has positive interactions with either BDQ or linezolid. Potential synergy between BDQ and pretomanid has been identified in part through pretomanid-led repression of the transcription factor Rv0880 that activates a tolerance response and leads to survival against BDQ (Peterson et al., 2016). Multiple links between pretomanid-induced disruption of the stress response appear to underpin further synergistic interactions. This includes pretomanid-mediated reduction in the expression of dormancy genes, contributing to a reduced oxygen consumption and ATP levels of the bacilli leading to a synergistic interaction between the Cyt-bc1aa3 inhibitor TB47 (Zeng et al., 2021). The biphenyl amide DG70 that targets MenG required for menaquinone biosynthesis also showed enhanced bactericidal activity with pretomanid against in vitro cultures of M. tuberculosis (Sukheja et al., 2017).
Synergistic drug interactions with pretomanid have also been observed in mice with addition of PZA or moxifloxacin to combination of BDQ plus pretomanid showing reduced time to relapse compared to BDQ + pretomanid alone, and the combination of all four drugs showed a further decrease in relapse (Tasneen et al., 2011; Li et al., 2017). The addition of linezolid to BDQ plus pretomanid treatment of M. tuberculosis HN878 in mice showed lower efficacy compared to BDQ plus pretomanid alone but greater efficacy for the treatment of M. tuberculosis H37Rv. This highlights that differences between geographic lineages of M. tuberculosis may confound the efficacy of not only individual drugs but unique drug combinations (Bigelow et al., 2020).
Review Conclusion
Inhibitors of mycobacterial bioenergetics have been shown to have significant clinical potential, in combating both the spread of drug resistance and in reducing treatment times for drug susceptible strains of M. tuberculosis. Much of this potential relies on the ability of metabolic inhibitors to disrupt mycobacterial metabolism and potentiate the activity of other anti-tuberculosis agents, in particular other inhibitors of mycobacterial bioenergetics or metabolism. Achieving the full clinical potential of bioenergetic combinations requires a thorough understanding of the consequences of inhibiting specific bioenergetic nodes, both in isolation and in combination with other metabolic dysregulation. Whilst significant insights have been gained from studying the effects of on-target chemical inhibitors, many potential drug targets lack bona-fide chemical inhibitors. Genetic tools to deplete essential genes at a transcriptional and/or a translation level have filled this gap and provided significant insights into the consequences of inhibiting mycobacterial bioenergetics as well as the potential for highly efficacious combination therapies. The continued advancement and application of the mycobacterial genetics should allow for the continued investigation and prioritization of drug targets and unique combination therapies centered around bioenergetic inhibitors. There is also a need for an improved understanding of the mechanisms that provide resistance to bioenergetic inhibitors, including those in pre-existing clinical isolates, as they will allow for the development of companion diagnostic tools to further the clinical efficacy and lifespan of these novel agents and combinations.
Author contributions
All authors contributed to the writing and editing of this review.
Funding
This work was supported by the Joint Research Health Research Council of New Zealand (HRC)-NSFC Collaboration grant numbers 20/1211 (to GC), 82061128001 (to TZ), and partially by the Chinese Academy of Sciences (154144KYSB20190005, YJKYYQ20210026). This work was also supported by Joint funding from the Maurice Wilkins Centre and the Chinese Academy of Sciences (awarded to MM, GC, and ZL). The funders had no role in study design, data collection, and analysis, decision to publish, or preparation of the manuscript.
Conflict of interest
The authors declare that the research was conducted in the absence of any commercial or financial relationships that could be construed as a potential conflict of interest.
Publisher’s note
All claims expressed in this article are solely those of the authors and do not necessarily represent those of their affiliated organizations, or those of the publisher, the editors and the reviewers. Any product that may be evaluated in this article, or claim that may be made by its manufacturer, is not guaranteed or endorsed by the publisher.
References
Abrahams, K. A., Cox, J. A. G., Spivey, V. L., Loman, N. J., Pallen, M. J., Constantinidou, C., et al. (2012). Identification of novel imidazo[1,2-a]pyridine inhibitors targeting m. tuberculosis QcrB. PloS One 7 (12), e52951. doi: 10.1371/journal.pone.0052951.
Adolph, C., McNeil, M. B., Cook, G. M. (2022). Impaired succinate oxidation prevents growth and influences drug susceptibility in Mycobacterium tuberculosis. mBio, e0167222. doi: 10.1128/mbio.01672-22
Andries, K., Verhasselt, P., Guillemont, J., Gohlmann, H. W., Neefs, J. M., Winkler, H., et al. (2005). A diarylquinoline drug active on the ATP synthase of mycobacterium tuberculosis. Science 307, 223–227. doi: 10.1126/science.1106753
Andries, K., Villellas, C., Coeck, N., Thys, K., Gevers, T., Vranckx, L., et al. (2014). Acquired resistance of mycobacterium tuberculosis to bedaquiline. PloS One 9 (7), e102135. doi: 10.1371/journal.pone.0102135
Arora, K., Ochoa-Montano, B., Tsang, P. S., Blundell, T. L., Dawes, S. S., Mizrahi, V., et al. (2014). Respiratory flexibility in response to inhibition of cytochrome c oxidase in mycobacterium tuberculosis. Antimicrob. Agents Chemother. 58 (11), 6962–6965. doi: 10.1128/AAC.03486-14
Aung, H. L., Berney, M., Cook, G. M. (2014). Hypoxia-activated cytochrome bd expression in mycobacterium smegmatis is cyclic AMP receptor protein dependent. J. Bacteriol. 196 (17), 3091–3097. doi: 10.1128/JB.01771-14
Bajeli, S., Baid, N., Kaur, M., Pawar, G. P., Chaudhari, V. D., Kumar, A. (2020). Terminal respiratory oxidases: A targetables vulnerability of mycobacterial bioenergetics? Front. Cell Infect. Microbiol. 10. doi: 10.3389/fcimb.2020.589318
Baptista, R., Fazakerley, D. M., Beckmann, M., Baillie, L., Mur, L. A. J. (2018). Untargeted metabolomics reveals a new mode of action of pretomanid (PA-824). Sci. Rep. 8 (1), 5084. doi: 10.1038/s41598-018-23110-1
Bashiri, G., Nigon, L. V., Jirgis, E. N. M., Ho, N. A. T., Stanborough, T., Dawes, S. S., et al. (2020). Allosteric regulation of menaquinone (vitamin K2) biosynthesis in the human pathogen mycobacterium tuberculosis. J. Biol. Chem. 295 (12), 3759–3770. doi: 10.1074/jbc.RA119.012158
Beites, T., O'Brien, K., Tiwari, D., Engelhart, C. A., Walters, S., Andrews, J., et al. (2019). Plasticity of the mycobacterium tuberculosis respiratory chain and its impact on tuberculosis drug development. Nat. Commun. 10 (1), 4970. doi: 10.1038/s41467-019-12956-2
Berney, M., Cook, G. M. (2010). Unique flexibility in energy metabolism allows mycobacteria to combat starvation and hypoxia. PloS One 5 (1), e8614. doi: 10.1371/journal.pone.0008614
Berney, M., Hartman, T. E., Jacobs, W. R., Jr. (2014). A mycobacterium tuberculosis cytochrome bd oxidase mutant is hypersensitive to bedaquiline. MBio 5 (4), e01275–e01214. doi: 10.1128/mBio.01275-14
Berube, B. J., Parish, T. (2018). Combinations of respiratory chain inhibitors have enhanced bactericidal activity against mycobacterium tuberculosis. Antimicrob. Agents Chemother. 62 (1), e01677-17. doi: 10.1128/AAC.01677-17
Berube, B. J., Russell, D., Castro, L., Choi, S. R., Narayanasamy, P., Parish, T. (2019). Novel MenA inhibitors are bactericidal against mycobacterium tuberculosis and synergize with electron transport chain inhibitors. Antimicrob. Agents Chemother. 63 (6), e02661–18. doi: 10.1128/AAC.02661-18
Bigelow, K. M., Tasneen, R., Chang, Y. S., Dooley, K. E., Nuermberger, E. L. (2020). Preserved efficacy and reduced toxicity with intermittent linezolid dosing in combination with bedaquiline and pretomanid in a murine tuberculosis model. Antimicrob. Agents Chemother. 64 (10), e01178-20. doi: 10.1128/AAC.01178-20
Blaza, J. N., Bridges, H. R., Aragao, D., Dunn, E. A., Heikal, A., Cook, G. M., et al. (2017). The mechanism of catalysis by type-II NADH: Quinone oxidoreductases. Sci. Rep. 7, 40165. doi: 10.1038/srep40165
Borisov, V. B., Murali, R., Verkhovskaya, M. L., Bloch, D. A., Han, H., Gennis, R. B., et al. (2011). Aerobic respiratory chain of escherichia coli is not allowed to work in fully uncoupled mode. Proc. Natl. Acad. Sci. U.S.A. 108 (42), 17320–17324. doi: 10.1073/pnas.1108217108
Borisov, V. B., Siletsky, S. A., Paiardini, A., Hoogewijs, D., Forte, E., Giuffre, A., et al. (2021). Bacterial oxidases of the cytochrome bd family: Redox enzymes of unique structure, function, and utility as drug targets. Antioxid. Redox Signal 34 (16), 1280–1318. doi: 10.1089/ars.2020.8039
Bosch, B., DeJesus, M. A., Poulton, N. C., Zhang, W., Engelhart, C. A., Zaveri, A., et al. (2021). Genome-wide gene expression tuning reveals diverse vulnerabilities of m. Tuberc. Cell 184 (17), 4579–4592 e4524. doi: 10.1016/j.cell.2021.06.033
Boshoff, H. I., Myers, T. G., Copp, B. R., McNeil, M. R., Wilson, M. A., Barry, C. (2004). The transcriptional responses of mycobacterium tuberculosis to inhibitors of metabolism: Novel insights into drug mechanisms of action. J. Biol. Chem 279 (38), 40174–84. doi: 10.1074/jbc.M406796200
Cai, Y., Jaecklein, E., Mackenzie, J. S., Papavinasasundaram, K., Olive, A. J., Chen, X., et al. (2021). Host immunity increases mycobacterium tuberculosis reliance on cytochrome bd oxidase. PloS Pathog. 17 (7), e1008911. doi: 10.1371/journal.ppat.1008911
Chen, P., Gearhart, J., Protopopova, M., Einck, L., Nacy, C. A. (2006). Synergistic interactions of SQ109, a new ethylene diamine, with front-line antitubercular drugs in vitro. J. Antimicrob. Chemother. 58 (2), 332–337. doi: 10.1093/jac/dkl227
Choi, P. J., Lu, G. L., Sutherland, H. S., Giddens, A. C., Franzblau, S. G., Cooper, C. B., et al. (2022). Synthetic studies towards isomeric pyrazolopyrimidines as potential ATP synthesis inhibitors of mycobacterium tuberculosis. structural correction of reported n-(6-(2-(dimethylamino)ethoxy)-5-fluoropyridin-3-yl)-2-(4-fluorophenyl)-5-(triflu oromethyl)pyrazolo[1,5-alpha]pyrimidin-7-amine. Tetrahedron Lett. 90. doi: 10.1016/j.tetlet.2021.153611
Chong, S. M. S., Manimekalai, M. S. S., Sarathy, J. P., Williams, Z. C., Harold, L. K., Cook, G. M., et al. (2020). Antituberculosis activity of the antimalaria cytochrome bcc oxidase inhibitor SCR0911. ACS Infect. Dis. 6 (4), 725–737. doi: 10.1021/acsinfecdis.9b00408
Cohen, J. (2013). Infectious disease. approval of novel TB drug celebrated–with restraint. Science 339 (6116), 130. doi: 10.1126/science.339.6116.130
Conradie, F., Diacon, A. H., Ngubane, N., Howell, P., Everitt, D., Crook, A. M., et al. (2020). Treatment of highly drug-resistant pulmonary tuberculosis. N. Engl. J. Med. 382 (10), 893–902. doi: 10.1056/NEJMoa1901814
Cook, G. M., Greening, C., Hards, K., Berney, M. (2014). Energetics of pathogenic bacteria and opportunities for drug development. Adv. Microb. Physiol. 65, 1–62. doi: 10.1016/bs.ampbs.2014.08.001
Cook, G. M., Hards, K., Dunn, E., Heikal, A., Nakatani, Y., Greening, C., et al. (2017). Oxidative phosphorylation as a target space for tuberculosis: Success, caution, and future directions. Microbiol. Spectr. 5 (3), TBTB2-0014-2016. doi: 10.1128/microbiolspec.TBTB2-0014-2016
Crowle, A. J., Douvas, G. S., May, M. H. (1992). Chlorpromazine: A drug potentially useful for treating mycobacterial infections. Chemotherapy 38 (6), 410–9. doi: 10.1159/000239036
Debnath, J., Siricilla, S., Wan, B., Crick, D. C., Lenaerts, A. J., Franzblau, S. G., et al. (2012). Discovery of selective menaquinone biosynthesis inhibitors against mycobacterium tuberculosis. J. Med. Chem. 55 (8), 3739–3755. doi: 10.1021/jm201608g
Denny, W. A. (2021). Inhibitors of F1F0-ATP synthase enzymes for the treatment of tuberculosis and cancer. Future Med. Chem. 13 (10), 911–926. doi: 10.4155/fmc-2021-0010
Dhiman, R. K., Mahapatra, S., Slayden, R. A., Boyne, M. E., Lenaerts, A., Hinshaw, J. C., et al. (2009). Menaquinone synthesis is critical for maintaining mycobacterial viability during exponential growth and recovery from non-replicating persistence. Mol. Microbiol. 72 (1), 85–97. doi: 10.1111/j.1365-2958.2009.06625.x
Diacon, A. H., Donald, P. R., Pym, A., Grobusch, M., Patientia, R. F., Mahanyele, R., et al. (2012). Randomized pilot trial of eight weeks of bedaquiline (TMC207) treatment for multidrug-resistant tuberculosis: Long-term outcome, tolerability, and effect on emergence of drug resistance. Antimicrob. Agents Chemother. 56 (6), 3271–3276. doi: 10.1128/AAC.06126-11
Diacon, A. H., Pym, A., Grobusch, M. P., de los Rios, J. M., Gotuzzo, E., Vasilyeva, I., et al. (2014). Multidrug-resistant tuberculosis and culture conversion with bedaquiline. N. Engl. J. Med. 371 (8), 723–732. doi: 10.1056/NEJMoa1313865
Donati, S., Kuntz, M., Pahl, V., Farke, N., Beuter, D., Glatter, T., et al. (2021). Multi-omics analysis of CRISPRi-knockdowns identifies mechanisms that buffer decreases of enzymes in e. coli metabolism. Cell Syst. 12 (1), 56–67 e56. doi: 10.1016/j.cels.2020.10.011
Dunn, E. A., Roxburgh, M., Larsen, L., Smith, R. A., McLellan, A. D., Heikal, A., et al. (2014). Incorporation of triphenylphosphonium functionality improves the inhibitory properties of phenothiazine derivatives in mycobacterium tuberculosis. Bioorganic Medicinal Chem. 22 (19), 5320–5328. doi: 10.1016/j.bmc.2014.07.050
Eoh, H., Rhee, K. Y. (2013). Multifunctional essentiality of succinate metabolism in adaptation to hypoxia in mycobacterium tuberculosis. Proc. Natl. Acad. Sci. U.S.A. 110 (16), 6554–6559. doi: 10.1073/pnas.1219375110
Fang, M., Toogood, R. D., Macova, A., Ho, K., Franzblau, S. G., McNeil, M. R., et al. (2010). Succinylphosphonate esters are competitive inhibitors of MenD that show active-site discrimination between homologous alpha-ketoglutarate-decarboxylating enzymes. Biochemistry 49 (12), 2672–2679. doi: 10.1021/bi901432d
Feng, X., Zhu, W., Schurig-Briccio, L. A., Lindert, S., Shoen, C., Hitchings, R., et al. (2015). Antiinfectives targeting enzymes and the proton motive force. Proc. Natl. Acad. Sci. U.S.A. 112 (51), E7073–E7082. doi: 10.1073/pnas.1521988112
Gao, Y., Hameed, H. M. A., Liu, Y., Guo, L., Fang, C., Tian, X., et al. (2021). Ultra-short-course and intermittent TB47-containing oral regimens produce stable cure against buruli ulcer in a murine model and prevent the emergence of resistance for mycobacterium ulcerans. Acta Pharm. Sin. B 11 (3), 738–749. doi: 10.1016/j.apsb.2020.11.007
Gomez-Gonzalez, P. J., Perdigao, J., Gomes, P., Puyen, Z. M., Santos-Lazaro, D., Napier, G., et al. (2021). Genetic diversity of candidate loci linked to mycobacterium tuberculosis resistance to bedaquiline, delamanid and pretomanid. Sci. Rep. 11 (1), 19431. doi: 10.1038/s41598-021-98862-4
Gong, H., Gao, Y., Zhou, X., Xiao, Y., Wang, W., Tang, Y., et al. (2020). Cryo-EM structure of trimeric mycobacterium smegmatis succinate dehydrogenase with a membrane-anchor SdhF. Nat. Commun. 11 (1), 4245. doi: 10.1038/s41467-020-18011-9
Gong, H., Li, J., Xu, A., Tang, Y., Ji, W., Gao, R., et al. (2018). An electron transfer path connects subunits of a mycobacterial respiratory supercomplex. Science 362 (6418). doi: 10.1126/science.aat8923
Griffin, J. E., Gawronski, J. D., Dejesus, M. A., Ioerger, T. R., Akerley, B. J., Sassetti, C. M. (2011). High-resolution phenotypic profiling defines genes essential for mycobacterial growth and cholesterol catabolism. PloS Pathog. 7 (9), e1002251. doi: 10.1371/journal.ppat.1002251
Grosset, J. H., Tyagi, S., Almeida, D. V., Converse, P. J., Li, S. Y., Ammerman, N. C., et al. (2013). Assessment of clofazimine activity in a second-line regimen for tuberculosis in mice. Am. J. Respir. Crit. Care Med. 188 (5), 608–612. doi: 10.1164/rccm.201304-0753OC
Guo, H., Courbon, G. M., Bueler, S. A., Mai, J., Liu, J., Rubinstein, J. L. (2021). Structure of mycobacterial ATP synthase bound to the tuberculosis drug bedaquiline. Nature 589 (7840), 143–147. doi: 10.1038/s41586-020-3004-3
Gurumurthy, M., Mukherjee, T., Dowd, C. S., Singh, R., Niyomrattanakit, P., Tay, J. A., et al. (2012). Substrate specificity of the deazaflavin-dependent nitroreductase from mycobacterium tuberculosis responsible for the bioreductive activation of bicyclic nitroimidazoles. FEBS J. 279 (1), 113–125. doi: 10.1111/j.1742-4658.2011.08404.x
Gurumurthy, M., Rao, M., Mukherjee, T., Rao, S. P., Boshoff, H. I., Dick, T., et al. (2013). A novel F(420) -dependent anti-oxidant mechanism protects mycobacterium tuberculosis against oxidative stress and bactericidal agents. Mol. Microbiol. 87 (4), 744–755. doi: 10.1111/mmi.12127
Harbut, M. B., Liu, R., Yang, B., Yano, T., Vilcheze, C., Lockner, J., et al. (2018). Small molecules targeting mycobacterium tuberculosis type II NADH dehydrogenase with antimycobacterial activity. Angew Chem. Int. Ed Engl 57 (13), 3478–3482 doi: 10.1002/anie.201800260
Hards, K., Adolph, C., Harold, L. K., McNeil, M. B., Cheung, C. Y., Jinich, A., et al. (2020). Two for the price of one: Attacking the energetic-metabolic hub of mycobacteria to produce new chemotherapeutic agents. Prog. Biophys. Mol. Biol. 152, 35–44. doi: 10.1016/j.pbiomolbio.2019.11.003
Hards, K., Cheung, C. Y., Waller, N., Adolph, C., Keighley, L., Tee, Z. S., et al. (2022). An amiloride derivative is active against the F1Fo-ATP synthase and cytochrome bd oxidase of mycobacterium tuberculosis. Commun. Biol. 5 (1), 166. doi: 10.1038/s42003-022-03110-8
Hards, K., Cook, G. M. (2018). Targeting bacterial energetics to produce new antimicrobials. Drug Resist. Update 36, 1–12. doi: 10.1016/j.drup.2017.11.001
Hards, K., McMillan, D. G. G., Schurig-Briccio, L. A., Gennis, R. B., Lill, H., Bald, D., et al. (2018). Ionophoric effects of the antitubercular drug bedaquiline. Proc. Natl. Acad. Sci. U.S.A. 115 (28), 7326–7331. doi: 10.1073/pnas.1803723115
Hards, K., Robson, J. R., Berney, M., Shaw, L., Bald, D., Koul, A., et al. (2015). Bactericidal mode of action of bedaquiline. J. Antimicrob. Chemother. 70 (7), 2028–2037. doi: 10.1093/jac/dkv054
Hards, K., Rodriguez, S. M., Cairns, C., Cook, G. M. (2019). Alternate quinone coupling in a new class of succinate dehydrogenase may potentiate mycobacterial respiratory control. FEBS Lett. 593 (5), 475–486. doi: 10.1002/1873-3468.13330
Harikishore, A., Chong, S. S. M., Ragunathan, P., Bates, R. W., Gruber, G. (2020). Targeting the menaquinol binding loop of mycobacterial cytochrome bd oxidase. Mol. Divers 25 (1), 517–524. doi: 10.1007/s11030-020-10034-0.
Harold, L. K., Jinich, A., Hards, K., Cordeiro, A., Keighley, L. M., Cross, A., et al. (2022). Deciphering functional redundancy and energetics of malate oxidation in mycobacteria. J. Biol. Chem. 298 (5), 101859. doi: 10.1016/j.jbc.2022.101859
Hartkoorn, R. C., Uplekar, S., Cole, S. T. (2014). Cross-resistance between clofazimine and bedaquiline through upregulation of MmpL5 in mycobacterium tuberculosis. Antimicrob. Agents Chemother. 58 (5), 2979–2981. doi: 10.1128/AAC.00037-14
Hartman, T., Weinrick, B., Vilcheze, C., Berney, M., Tufariello, J., Cook, G. M., et al. (2014). Succinate dehydrogenase is the regulator of respiration in mycobacterium tuberculosis. PloS Pathog. 10 (11), e1004510. doi: 10.1371/journal.ppat.1004510
Hartuti, E. D., Inaoka, D. K., Komatsuya, K., Miyazaki, Y., Miller, R. J., Xinying, W., et al. (2018). Biochemical studies of membrane bound plasmodium falciparum mitochondrial l-malate:quinone oxidoreductase, a potential drug target. Biochim. Biophys. Acta Bioenerg. 1859 (3), 191–200. doi: 10.1016/j.bbabio.2017.12.004
Hasenoehrl, E. J., Wiggins, T. J., Berney, M. (2020). Bioenergetic inhibitors: Antibiotic efficacy and mechanisms of action in mycobacterium tuberculosis. Front. Cell Infect. Microbiol. 10. doi: 10.3389/fcimb.2020.611683
Heikal, A., Hards, K., Cheung, C. Y., Menorca, A., Timmer, M. S., Stocker, B. L., et al. (2016). Activation of type II NADH dehydrogenase by quinolinequinones mediates antitubercular cell death. J. Antimicrob. Chemother. 71 (10), 2840–2847. doi: 10.1093/jac/dkw244
Heikal, A., Nakatani, Y., Dunn, E., Weimar, M. R., Day, C. L., Baker, E. N., et al. (2014). Structure of the bacterial type II NADH dehydrogenase: A monotopic membrane protein with an essential role in energy generation. Mol. Microbiol. 91 (5), 950–964. doi: 10.1111/mmi.12507
Heikal, A., Nakatani, Y., Jiao, W., Wilson, C., Rennison, D., Weimar, M. R., et al. (2018). 'Tethering' fragment-based drug discovery to identify inhibitors of the essential respiratory membrane protein type II NADH dehydrogenase. Bioorg. Med. Chem. Lett. 28 (13), 2239–2243. doi: 10.1016/j.bmcl.2018.05.048
Hotra, A., Ragunathan, P., Ng, P. S., Seankongsuk, P., Harikishore, A., Sarathy, J. P., et al. (2020). Discovery of a novel mycobacterial f-ATP synthase inhibitor and its potency in combination with diarylquinolines. Angew Chem. Int. Ed Engl 59 (32), 13295–13304. doi: 10.1002/anie.202002546
Huitric, E., Verhasselt, P., Koul, A., Andries, K., Hoffner, S., Andersson, D. I. (2010). Rates and mechanisms of resistance development in mycobacterium tuberculosis to a novel diarylquinoline ATP synthase inhibitor. Antimicrob. Agents Chemother. 54 (3), 1022–1028. doi: 10.1128/AAC.01611-09
Jang, J., Kim, R., Woo, M., Jeong, J., Park, D. E., Kim, G., et al. (2017). Efflux attenuates the antibacterial activity of Q203 in mycobacterium tuberculosis. Antimicrob. Agents Chemother. 61 (7), e02637–16. doi: 10.1128/AAC.02637-16
Kalia, N. P., Hasenoehrl, E. J., Ab Rahman, N. B., Koh, V. H., Ang, M. L. T., Sajorda, D. R., et al. (2017). Exploiting the synthetic lethality between terminal respiratory oxidases to kill mycobacterium tuberculosis and clear host infection. Proc. Natl. Acad. Sci. U.S.A 114 (28), 7426–7431 doi: 10.1073/pnas.1706139114
Kamariah, N., Ragunathan, P., Shin, J., Saw, W. G., Wong, C. F., Dick, T., et al. (2020). Unique structural and mechanistic properties of mycobacterial f-ATP synthases: Implications for drug design. Prog. Biophys. Mol. Biol. 152, 64–73. doi: 10.1016/j.pbiomolbio.2019.11.006
Kana, B. D., Weinstein, E. A., Avarbock, D., Dawes, S. S., Rubin, H., Mizrahi, V. (2001). Characterization of the cydAB-encoded cytochrome bd oxidase from mycobacterium smegmatis. J. Bacteriol. 183 (24), 7076–7086. doi: 10.1128/JB.183.24.7076-7086.2001
Kang, S., Kim, R. Y., Seo, M. J., Lee, S., Kim, Y. M., Seo, M., et al. (2014). Lead optimization of a novel series of Imidazo[1,2-a]pyridine amides leading to a clinical candidate (Q203) as a multi- and extensively-drug resistant antituberculosis agent. J. Medicinal Chem 57 (12), 5293–305 doi: 10.1021/jm5003606
Keam, S. J. (2019). Pretomanid: First approval. Drugs 79 (16), 1797–1803. doi: 10.1007/s40265-019-01207-9
Kerantzas, C. A., Jacobs, W. R., Jr (2017). Origins of combination therapy for tuberculosis: Lessons for future antimicrobial development and application. mBio 8 (2), e01586–e01516. doi: 10.1128/mBio.01586-16
Koh, E. I., Oluoch, P. O., Ruecker, N., Proulx, M. K., Soni, V., Murphy, K. C., et al. (2022). Chemical-genetic interaction mapping links carbon metabolism and cell wall structure to tuberculosis drug efficacy. Proc. Natl. Acad. Sci. U.S.A. 119 (15), e2201632119. doi: 10.1073/pnas.2201632119
Koul, A., Dendouga, N., Vergauwen, K., Molenberghs, B., Vranckx, L., Willebrords, R., et al. (2007). Diarylquinolines target subunit c of mycobacterial ATP synthase. Nat. Chem. Biol. 3 (6), 323–324. doi: 10.1038/nchembio884
Koul, A., Vranckx, L., Dendouga, N., Balemans, W., Van den Wyngaert, I., Vergauwen, K., et al. (2008). Diarylquinolines are bactericidal for dormant mycobacteria as a result of disturbed ATP homeostasis. J. Biol. Chem. 283 (37), 25273–25280. doi: 10.1074/jbc.M803899200
Koul, A., Vranckx, L., Dhar, N., Gohlmann, H. W., Ozdemir, E., Neefs, J. M., et al. (2014). Delayed bactericidal response of mycobacterium tuberculosis to bedaquiline involves remodelling of bacterial metabolism. Nat. Commun. 5, 3369. doi: 10.1038/ncomms4369
Lamprecht, D. A., Finin, P. M., Rahman, M. A., Cumming, B. M., Russell, S. L., Jonnala, S. R., et al. (2016). Turning the respiratory flexibility of mycobacterium tuberculosis against itself. Nat. Commun. 7, 12393. doi: 10.1038/ncomms12393
Lange, C., Chesov, D., Heyckendorf, J., Leung, C. C., Udwadia, Z., Dheda, K. (2018). Drug-resistant tuberculosis: An update on disease burden, diagnosis and treatment. Respirology 23 (7), 656–673. doi: 10.1111/resp.13304
Lechartier, B., Hartkoorn, R. C., Cole, S. T. (2012). In vitro combination studies of benzothiazinone lead compound BTZ043 against mycobacterium tuberculosis. Antimicrob. Agents Chemother. 56 (11), 5790–5793. doi: 10.1128/AAC.01476-12
Lee, B. S., Hards, K., Engelhart, C. A., Hasenoehrl, E. J., Kalia, N. P., Mackenzie, J. S., et al. (2021). Dual inhibition of the terminal oxidases eradicates antibiotic-tolerant mycobacterium tuberculosis. EMBO Mol. Med. 13 (1), e13207. doi: 10.15252/emmm.202013207
Lee, B. M., Harold, L. K., Almeida, D. V., Afriat-Jurnou, L., Aung, H. L., Forde, B. M., et al. (2020). Predicting nitroimidazole antibiotic resistance mutations in mycobacterium tuberculosis with protein engineering. PloS Pathog. 16 (2), e1008287. doi: 10.1371/journal.ppat.1008287
Lee, B. S., Kalia, N. P., Jin, X. E. F., Hasenoehrl, E. J., Berney, M., Pethe, K. (2019). Inhibitors of energy metabolism interfere with antibiotic-induced death in mycobacteria. J. Biol. Chem. 294 (6), 1936–1943. doi: 10.1074/jbc.RA118.005732
Lee, B. S., Sviriaeva, E., Pethe, K. (2020). Targeting the cytochrome oxidases for drug development in mycobacteria. Prog. Biophys. Mol. Biol. 152, 45–54. doi: 10.1016/j.pbiomolbio.2020.02.001
Lee, A. S., Teo, A. S., Wong, S. Y. (2001). Novel mutations in ndh in isoniazid-resistant mycobacterium tuberculosis isolates. Antimicrob. Agents Chemother 45 (7), 2157–2159. doi: 10.1128/AAC.45.7.2157-2159.2001
Li, L., Koirala, B., Hernandez, Y., MacIntyre, L. W., Ternei, M. A., Russo, R., et al. (2022). Identification of structurally diverse menaquinone-binding antibiotics with in vivo activity against multidrug-resistant pathogens. Nat. Microbiol. 7 (1), 120–131. doi: 10.1038/s41564-021-01013-8
Li, K., Schurig-Briccio, L. A., Feng, X., Upadhyay, A., Pujari, V., Lechartier, B., et al. (2014). Multi-target drug discovery for tuberculosis and other infectious diseases. J. Med. Chem 57 (7), 3126–3139. doi: 10.1021/jm500131s
Li, S. Y., Tasneen, R., Tyagi, S., Soni, H., Converse, P. J., Mdluli, K., et al. (2017). Bactericidal and sterilizing activity of a novel regimen with bedaquiline, pretomanid, moxifloxacin, and pyrazinamide in a murine model of tuberculosis. Antimicrob. Agents Chemother. 61 (9), e00913-17. doi: 10.1128/AAC.00913-17
Liu, Y., Gao, Y., Liu, J., Tan, Y., Liu, Z., Chhotaray, C., et al. (2019). The compound TB47 is highly bactericidal against mycobacterium ulcerans in a buruli ulcer mouse model. Nat. Commun. 10 (1), 524. doi: 10.1038/s41467-019-08464-y
Liu, Y., Tan, Y., Islam, M. M., Cao, Y., Lu, X., Zeng, S., et al. (2020). Assessment of clofazimine and TB47 combination activity against mycobacterium abscessus using a bioluminescent approach. Antimicrob. Agents Chemother. 64 (3), e01881–19. doi: 10.1128/AAC.01881-19
Lu, P., Asseri, A. H., Kremer, M., Maaskant, J., Ummels, R., Lill, H., et al. (2018). The anti-mycobacterial activity of the cytochrome bcc inhibitor Q203 can be enhanced by small-molecule inhibition of cytochrome bd. Sci. Rep. 8 (1), 2625. doi: 10.1038/s41598-018-20989-8
Lu, P., Heineke, M. H., Koul, A., Andries, K., Cook, G. M., Lill, H., et al. (2015). The cytochrome bd-type quinol oxidase is important for survival of mycobacterium smegmatis under peroxide and antibiotic-induced stress. Sci. Rep. 5, 10333. doi: 10.1038/srep10333
Lu, X., Williams, Z., Hards, K., Tang, J., Cheung, C.-Y., Aung, H. L., et al. (2019). Pyrazolo [1, 5-a] pyridine inhibitor of the respiratory cytochrome bcc complex for the treatment of drug-resistant tuberculosis. ACS Infect. Dis. 5 (2), 239–249. doi: 10.1021/acsinfecdis.8b00225
Mackenzie, J. S., Lamprecht, D. A., Asmal, R., Adamson, J. H., Borah, K., Beste, D. J. V., et al. (2020). Bedaquiline reprograms central metabolism to reveal glycolytic vulnerability in mycobacterium tuberculosis. Nat. Commun. 11 (1), 6092. doi: 10.1038/s41467-020-19959-4
Manjunatha, U., Boshoff, H. I., Barry, C. E. (2009). The mechanism of action of PA-824: Novel insights from transcriptional profiling. Commun. Integr. Biol. 2 (3), 215–218. doi: 10.4161/cib.2.3.7926
Manjunatha, U. H., Boshoff, H., Dowd, C. S., Zhang, L., Albert, T. J., Norton, J. E., et al. (2006a). Identification of a nitroimidazo-oxazine-specific protein involved in PA-824 resistance in mycobacterium tuberculosis. Proc. Natl. Acad. Sci. U.S.A. 103 (2), 431–436. doi: 10.1073/pnas.0508392103
Manjunatha, U. H., Lahiri, R., Randhawa, B., Dowd, C. S., Krahenbuhl, J. L., Barry, C. E., 3rd (2006b). Mycobacterium leprae is naturally resistant to PA-824. Antimicrob. Agents Chemother. 50 (10), 3350–3354. doi: 10.1128/AAC.00488-06
Matsoso, L. G., Kana, B. D., Crellin, P. K., Lea-Smith, D. J., Pelosi, A., Powell, D., et al. (2005). Function of the cytochrome bc1-aa3 branch of the respiratory network in mycobacteria and network adaptation occurring in response to its disruption. J. Bacteriol. 187 (18), 6300–6308. doi: 10.1128/JB.187.18.6300-6308.2005
Matsumoto, M., Hashizume, H., Tomishige, T., Kawasaki, M., Tsubouchi, H., Sasaki, H., et al. (2006). OPC-67683, a nitro-dihydro-imidazooxazole derivative with promising action against tuberculosis in vitro and in mice. PloS Med. 3 (11), e466. doi: 10.1371/journal.pmed.0030466
McNeil, M. B., Keighley, L. M., Cook, J. R., Cheung, C. Y., Cook, G. M. (2021). CRISPR interference identifies vulnerable cellular pathways with bactericidal phenotypes in mycobacterium tuberculosis. Mol. Microbiol. 116 (4), 1033–1043. doi: 10.1111/mmi.14790
McNeil, M. B., Ryburn, H. W. K., Harold, L. K., Tirados, J. F., Cook, G. M. (2020). Transcriptional inhibition of the F1F0-type ATP synthase has bactericidal consequences on the viability of mycobacteria. Antimicrob. Agents Chemother. 64 (8), e00492-20. doi: 10.1128/AAC.00492-20
McNeil, M. B., Ryburn, H. W., Tirados, J., Cheung, C. Y., Cook, G. M. (2022). Multiplexed transcriptional repression identifies a network of bactericidal interactions between mycobacterial respiratory complexes. iScience 25 (1), 103573. doi: 10.1016/j.isci.2021.103573
Miesel, L., Weisbrod, T. R., Marcinkeviciene, J. A., Bittman, R., Jacobs, W. R. (1998). NADH dehydrogenase defects confer isoniazid resistance and conditional lethality in mycobacterium smegmatis. J. Bacteriol. 80 (9), 2459–2467. doi: 10.1128/JB.180.9.2459-2467.1998
Molenaar, D., van der Rest, M. E., Drysch, A., Yucel, R. (2000). Functions of the membrane-associated and cytoplasmic malate dehydrogenases in the citric acid cycle of corynebacterium glutamicum. J. Bacteriol. 182 (24), 6884–6891. doi: 10.1128/JB.182.24.6884-6891.2000
Montgomery, M. G., Petri, J., Spikes, T. E., Walker, J. E. (2021). Structure of the ATP synthase from mycobacterium smegmatis provides targets for treating tuberculosis. Proc. Natl. Acad. Sci. U.S.A. 118 (47), e2111899118. doi: 10.1073/pnas.2111899118
Moodliar, R., Aksenova, V., Frias, M. V. G., van de Logt, J., Rossenu, S., Birmingham, E., et al. (2021). Bedaquiline for multidrug-resistant TB in paediatric patients. Int. J. Tuberc. Lung Dis. 25 (9), 716–724. doi: 10.5588/ijtld.21.0022
Moosa, A., Lamprecht, D. A., Arora, K., Barry, C. E., 3rd, Boshoff, H. I. M., Ioerger, T. R., et al. (2017). Susceptibility of mycobacterium tuberculosis cytochrome bd oxidase mutants to compounds targeting the terminal respiratory oxidase, cytochrome c. Antimicrob. Agents Chemother. 61 (10), e01338-17. doi: 10.1128/AAC.01338-17
Murata-Kamiya, N., Kamiya, H. (2001). Methylglyoxal, an endogenous aldehyde, crosslinks DNA polymerase and the substrate DNA. Nucleic Acids Res. 29 (16), 3433–3438. doi: 10.1093/nar/29.16.3433
Narang, R., Kumar, R., Kalra, S., Nayak, S. K., Khatik, G. L., Kumar, G. N., et al. (2019). Recent advancements in mechanistic studies and structure activity relationship of FoF1 ATP synthase inhibitor as antimicrobial agent. Eur. J. Med. Chem. 182, 111644. doi: 10.1016/j.ejmech.2019.111644
Nikonenko, B. V., Protopopova, M., Samala, R., Einck, L., Nacy, C. A. (2007). Drug therapy of experimental tuberculosis (TB): Improved outcome by combining SQ109, a new diamine antibiotic, with existing TB drugs. Antimicrob. Agents Chemother. 51 (4), 1563–1565. doi: 10.1128/AAC.01326-06
Ocampo, P. S., Lazar, V., Papp, B., Arnoldini, M., Abel zur Wiesch, P., Busa-Fekete, R., et al. (2014). Antagonism between bacteriostatic and bactericidal antibiotics is prevalent. Antimicrob. Agents Chemother. 58 (8), 4573–4582. doi: 10.1128/AAC.02463-14
Pecsi, I., Hards, K., Ekanayaka, N., Berney, M., Hartman, T., Jacobs, W. R., Jr., et al. (2014). Essentiality of succinate dehydrogenase in mycobacterium smegmatis and its role in the generation of the membrane potential under hypoxia. MBio 5 (4), e01093-14. doi: 10.1128/mBio.01093-14
Peterson, E. J. R., Ma, S., Sherman, D. R., Baliga, N. S. (2016). Network analysis identifies Rv0324 and Rv0880 as regulators of bedaquiline tolerance in mycobacterium tuberculosis. Nat. Microbiol. 1 (8), 16078. doi: 10.1038/nmicrobiol.2016.78
Pethe, K., Bifani, P., Jang, J., Kang, S., Park, S., Ahn, S., et al. (2013). Discovery of Q203, a potent clinical candidate for the treatment of tuberculosis. Nat. Med. 19 (9), 1157–1160. doi: 10.1038/nm.3262
Petri, J., Shimaki, Y., Jiao, W., Bridges, H. R., Russell, E. R., Parker, E. J., et al. (2018). Structure of the NDH-2 - HQNO inhibited complex provides molecular insight into quinone-binding site inhibitors. Biochim. Biophys. Acta Bioenerg. 1859 (7), 482–490. doi: 10.1016/j.bbabio.2018.03.014
Preiss, L., Langer, J. D., Yildiz, O., Eckhardt-Strelau, L., Guillemont, J. E., Koul, A., et al. (2015). Structure of the mycobacterial ATP synthase fo rotor ring in complex with the anti-TB drug bedaquiline. Sci. Adv. 1 (4), e1500106. doi: 10.1126/sciadv.1500106
Rao, S. P., Alonso, S., Rand, L., Dick, T., Pethe, K. (2008). The protonmotive force is required for maintaining ATP homeostasis and viability of hypoxic, nonreplicating Mycobacterium tuberculosis. Proc. Natl. Acad. Sci. U.S.A. 105 (33), 11945–11950. doi: 10.1073/pnas.0711697105
Rhee, K. Y., Carvalho, L. P., Bryk, R., Ehrt, S., Marrero, J., Park, S. W., et al. (2011). Central carbon metabolism in mycobacterium tuberculosis: a unexpected frontier. Trends Microbiol 19 (7), 307–314 doi: 10.1016/j.tim.2011.03.008
Rittershaus, E. S. C., Baek, S. H., Krieger, I. V., Nelson, S. J., Cheng, Y. S., Nambi, S., et al. (2018). A lysine acetyltransferase contributes to the metabolic adaptation to hypoxia in Mycobacterium tuberculosis. Cell Chem. Biol. 25 (12), 1495–1505 e1493. doi: 10.1016/j.chembiol.2018.09.009
Safarian, S., Opel-Reading, H. K., Wu, D., Mehdipour, A. R., Hards, K., Harold, L. K., et al. (2021). The cryo-EM structure of the bd oxidase from m. tuberculosis reveals a unique structural framework and enables rational drug design to combat TB. Nat. Commun. 12 (1), 5236. doi: 10.1038/s41467-021-25537-z
Scherr, N., Bieri, R., Thomas, S. S., Chauffour, A., Kalia, N. P., Schneide, P., et al. (2018). Targeting the mycobacterium ulcerans cytochrome bc1:aa3 for the treatment of buruli ulcer. Nat. Commun. 9 (1), 5370. doi: 10.1038/s41467-018-07804-8
Shetty, A., Dick, T. (2018). Mycobacterial cell wall synthesis inhibitors cause lethal ATP burst. Front. Microbiol. 9. doi: 10.3389/fmicb.2018.01898
Shirude, P. S., Paul, P., Choudhury, N. R., Kedari, C., Bandodkar, B., Ugarkar, B. G. (2012). Quinolinyl pyrimidines: Potent inhibitors of NDH-2 as a novel class of anti-TB agents. ACS Medicinal Chem. Lett. 3 (9), 736–740. doi: 10.1021/ml300134b
Shi, L., Sohaskey, C. D., Kana, B. D., Dawes, S., North, R. J., Mizrahi, V., et al. (2005). Changes in energy metabolism of mycobacterium tuberculosis in mouse lung and under in vitro conditions affecting aerobic respiration. Proc. Natl. Acad. Sci. U.S.A. 102 (43), 15629–15634. doi: 10.1073/pnas.0507850102
Singh, R., Manjunatha, U., Boshoff, H. I., Ha, Y. H., Niyomrattanakit, P., Ledwidge, R., et al. (2008). PA-824 kills nonreplicating mycobacterium tuberculosis by intracellular NO release. Science 322 (5906), 1392–1395. doi: 10.1126/science.1164571
Singh, S., Roy, K. K., Khan, S. R., Kashyap, V. K., Sharma, A., Jaiswal, S., et al. (2015). Novel, potent, orally bioavailable and selective mycobacterial ATP synthase inhibitors that demonstrated activity against both replicating and non-replicating m. tuberculosis. Bioorg. Med. Chem. 23 (4), 742–752. doi: 10.1016/j.bmc.2014.12.060
Sorayah, R., Manimekalai, M. S. S., Shin, S. J., Koh, W. J., Gruber, G., Pethe, K. (2019). Naturally-occurring polymorphisms in QcrB are responsible for resistance to telacebec in mycobacterium abscessus. ACS Infect. Dis. 5 (12), 2055–2060. doi: 10.1021/acsinfecdis.9b00322
Stover, C. K., Warrener, P., VanDevanter, D. R., Sherman, D. R., Arain, T. M., Langhorne, M. H., et al. (2000). A small-molecule nitroimidazopyran drug candidate for the treatment of tuberculosis. Nature 405 (6789), 962–966. doi: 10.1038/35016103
Sukheja, P., Kumar, P., Mittal, N., Li, S. G., Singleton, E., Russo, R., et al. (2017). A novel small-molecule inhibitor of the mycobacterium tuberculosis demethylmenaquinone methyltransferase MenG is bactericidal to both growing and nutritionally deprived persister cells. MBio 8 (1), e02022-16. doi: 10.1128/mBio.02022-16
Sviriaeva, E., Subramanian Manimekalai, M. S., Gruber, G., Pethe, K. (2020). Features and functional importance of key residues of the mycobacterium tuberculosis cytochrome bd oxidase. ACS Infect. Dis 6 (7), 1697–1707 doi: 10.1021/acsinfecdis.9b00449
Tantry, S. J., Markad, S. D., Shinde, V., Bhat, J., Balakrishnan, G., Gupta, A. K., et al. (2017). Discovery of Imidazo[1,2-a]pyridine ethers and squaramides as selective and potent inhibitors of mycobacterial adenosine triphosphate (ATP) synthesis. J. Med. Chem. 60 (4), 1379–1399. doi: 10.1021/acs.jmedchem.6b01358
Tasneen, R., Li, S. Y., Peloquin, C. A., Taylor, D., Williams, K. N., Andries, K., et al. (2011). Sterilizing activity of novel TMC207- and PA-824-containing regimens in a murine model of tuberculosis. Antimicrob. Agents Chemother. 55 (12), 5485–5492. doi: 10.1128/AAC.05293-11
Thornalley, P. J., Waris, S., Fleming, T., Santarius, T., Larkin, S. J., Winklhofer-Roob, B. M., et al. (2010). Imidazopurinones are markers of physiological genomic damage linked to DNA instability and glyoxalase 1-associated tumour multidrug resistance. Nucleic Acids Res. 38 (16), 5432–5442. doi: 10.1093/nar/gkq306
Tran, S. L., Cook, G. M. (2005). The F1Fo-ATP synthase of Mycobacterium smegmatis is essential for growth. J. Bacteriol. 187 (14), 5023–5028. doi: 10.1128/JB.187.14.5023-5028.2005
Vilcheze, C., Weinrick, B., Leung, L. W., Jacobs, W. R., Jr. (2018). Plasticity of mycobacterium tuberculosis NADH dehydrogenases and their role in virulence. Proc. Natl. Acad. Sci. U. S. A. 115 (7), 1599–1604 doi: 10.1073/pnas.1721545115
Vilcheze, C., Weisbrod, T. R., Chen, B., Kremer, L., Hazbon, M. H., Wang, F., et al. (2005). Altered NADH/NAD+ ratio mediates coresistance to isoniazid and ethionamide in mycobacteria. Antimicrob. Agents Chemother. 49 (2), 708–720. doi: 10.1128/AAC.49.2.708-720.2005
Villellas, C., Coeck, N., Meehan, C. J., Lounis, N., de Jong, B., Rigouts, L., et al. (2017). Unexpected high prevalence of resistance-associated Rv0678 variants in MDR-TB patients without documented prior use of clofazimine or bedaquiline. J. Antimicrob. Chemother. 72 (3), 684–690. doi: 10.1093/jac/dkw502
Viveiros, M., Amaral, L. (2001). Enhancement of antibiotic activity against poly-drug resistant mycobacterium tuberculosis by phenothiazines. Int. J. Antimicrob. Agents 17 (3), 225–228. doi: 10.1016/s0924-8579(00)00343-5
Wang, W., Gao, Y., Tang, Y., Zhou, X., Lai, Y., Zhou, S., et al. (2021). Cryo-EM structure of mycobacterial cytochrome bd reveals two oxygen access channels. Nat. Commun. 12 (1), 4621. doi: 10.1038/s41467-021-24924-w
Wang, Z., Soni, V., Marriner, G., Kaneko, T., Boshoff, H. I. M., Barry, C. E., 3rd, et al. (2019). Mode-of-action profiling reveals glutamine synthetase as a collateral metabolic vulnerability of m. tuberculosis to bedaquiline. Proc. Natl. Acad. Sci. U.S.A. 116 (39), 19646–19651. doi: 10.1073/pnas.1907946116
Watanabe, S., Zimmermann, M., Goodwin, M. B., Sauer, U., Barry, C. E., 3rd, Boshoff, H. I. (2011). Fumarate reductase activity maintains an energized membrane in anaerobic mycobacterium tuberculosis. PloS Pathog. 7 (10), e1002287. doi: 10.1371/journal.ppat.1002287
Weinstein, E. A., Yano, T., Li, L. S., Avarbock, D., Avarbock, A., Helm, D., et al. (2005). Inhibitors of type II NADH:menaquinone oxidoreductase represent a class of antitubercular drugs. Proc. Natl. Acad. Sci. U.S.A. 102 (12), 4548–4553. doi: 10.1073/pnas.0500469102
Wiseman, B., Nitharwal, R. G., Fedotovskaya, O., Schafer, J., Guo, H., Kuang, Q., et al. (2018). Structure of a functional obligate complex III2IV2 respiratory supercomplex from mycobacterium smegmatis. Nat. Struct. Mol. Biol. 25 (12), 1128–1136. doi: 10.1038/s41594-018-0160-3
Xavier, A. S., Lakshmanan, M. (2014). Delamanid: A new armor in combating drug-resistant tuberculosis. J. Pharmacol. Pharmacother. 5 (3), 222–224. doi: 10.4103/0976-500X.136121
Yang, X., Yuan, T., Ma, R., Chacko, K. I., Smith, M., Deikus, G., et al. (2019). Mce3R stress-resistance pathway is vulnerable to small-molecule targeting that improves tuberculosis drug activities. ACS Infect. Dis. 5 (7), 1239–1251. doi: 10.1021/acsinfecdis.9b00099
Yanofsky, D. J., Di Trani, J. M., Krol, S., Abdelaziz, R., Bueler, S. A., Imming, P., et al. (2021). Structure of mycobacterial CIII2CIV2 respiratory supercomplex bound to the tuberculosis drug candidate telacebec (Q203). Elife 10, e71959. doi: 10.7554/eLife.71959
Yano, T., Kassovska-Bratinova, S., Teh, J. S., Winkler, J., Sullivan, K., Isaacs, A., et al. (2011). Reduction of clofazimine by mycobacterial type 2 NADH:Quinone oxidoreductase: A pathway for the generation of bactericidal levels of reactive oxygen species. J. Biol. Chem. 286 (12), 10276–10287. doi: 10.1074/jbc.M110.200501
Yano, T., Li, L. S., Weinstein, E., Teh, J. S., Rubin, H. (2006). Steady-state kinetics and inhibitory action of antitubercular phenothiazines on mycobacterium tuberculosis type-II NADH-menaquinone oxidoreductase (NDH-2). J. Biol. Chem. 281 (17), 11456–11463. doi: 10.1074/jbc.M508844200
Yao, R., Wang, B., Fu, L., Li, L., You, K., Li, Y. G., et al. (2022). Sudapyridine (WX-081), a novel compound against mycobacterium tuberculosis. Microbiol. Spectr. 10 (1), e0247721. doi: 10.1128/spectrum.02477-21
Yu, W., Chiwala, G., Gao, Y., Liu, Z., Sapkota, S., Lu, Z., et al. (2020). TB47 and clofazimine form a highly synergistic sterilizing block in a second-line regimen for tuberculosis in mice. BioMed. Pharmacother. 131, 110782. doi: 10.1016/j.biopha.2020.110782
Yu, W., Yusuf, B., Wang, S., Tian, X., Hameed, H. M. A., Lu, Z., et al. (2021). Sterilizing effects of novel regimens containing TB47, clofazimine, and linezolid in a murine model of tuberculosis. Antimicrob. Agents Chemother. 65 (10), e0070621. doi: 10.1128/AAC.00706-21
Zeng, S., Zhang, J., Sun, M., Zhang, X., Cook, G. M., Zhang, T. (2021). Nitric oxide-dependent electron transport chain inhibition by the cytochrome bc1 inhibitor and pretomanid combination kills mycobacterium tuberculosis. Antimicrob. Agents Chemother. 65 (9), e0095621. doi: 10.1128/AAC.00956-21
Zhang, Y. J., Ioerger, T. R., Huttenhower, C., Long, J. E., Sassetti, C. M., Sacchettini, J. C., et al. (2012). Global assessment of genomic regions required for growth in mycobacterium tuberculosis. PloS Pathog. 8 (9), e1002946. doi: 10.1371/journal.ppat.1002946
Zheng, H., Wang, Y., He, W., Li, F., Xia, H., Zhao, B., et al. (2022). In vitro activity of pretomanid against nontuberculous mycobacteria. Antimicrob. Agents Chemother. 66 (1), e0181021. doi: 10.1128/AAC.01810-21
Zhou, X., Gao, Y., Wang, W., Yang, X., Yang, X., Liu, F., et al. (2021). Architecture of the mycobacterial succinate dehydrogenase with a membrane-embedded rieske FeS cluster. Proc. Natl. Acad. Sci. U.S.A. 118 (15), e2022308118. doi: 10.1073/pnas.2022308118
Keywords: respiration, synthetic lethality, drug combinations, antibiotics, synergy
Citation: McNeil MB, Cheung C-Y, Waller NJE, Adolph C, Chapman CL, Seeto NEJ, Jowsey W, Li Z, Hameed HMA, Zhang T and Cook GM (2022) Uncovering interactions between mycobacterial respiratory complexes to target drug-resistant Mycobacterium tuberculosis. Front. Cell. Infect. Microbiol. 12:980844. doi: 10.3389/fcimb.2022.980844
Received: 29 June 2022; Accepted: 03 August 2022;
Published: 24 August 2022.
Edited by:
Helena Ingrid Boshoff, National Institutes of Health (NIH), United StatesReviewed by:
Bavesh Kana, University of the Witwatersrand, South AfricaKyle Rohde, University of Central Florida, United States
Copyright © 2022 McNeil, Cheung, Waller, Adolph, Chapman, Seeto, Jowsey, Li, Hameed, Zhang and Cook. This is an open-access article distributed under the terms of the Creative Commons Attribution License (CC BY). The use, distribution or reproduction in other forums is permitted, provided the original author(s) and the copyright owner(s) are credited and that the original publication in this journal is cited, in accordance with accepted academic practice. No use, distribution or reproduction is permitted which does not comply with these terms.
*Correspondence: Matthew B. McNeil, bWF0dGhldy5tY25laWxAb3RhZ28uYWMubno=; Gregory M. Cook, Z3JlZy5jb29rQG90YWdvLmFjLm56