- 1Nano-Bioconjugate Chemistry Lab, Cluster Innovation Centre, University of Delhi, Delhi, India
- 2Department of Environmental Studies, University of Delhi, Delhi, India
- 3Department of Chemistry, University of Delhi, Delhi, India
Escherichia is a genus of prokaryotic gram-negative bacteria which forms a vital component of the gut microbiota of homeotherms including humans. Many members of this genus are commensals and pathogenic strains, which are responsible for some of the most common bacterial infections and can be fatal, particularly in the case of newborns and children. The fecal matter in wastewater treatment plants serves as major environmental sinks for the accumulation of Escherichia. The rise in antibiotic pollution and the lateral gene exchange of antibiotic-resistant genes have created antibiotic-resistant Escherichia strains that are often called superbugs. Antibiotic resistance has reached a crisis level that nowadays existing antibiotics are no longer effective. One way of tackling this emerging concern is by using nanomaterials. Punitively, nanomaterials can be used by conjugating with antibodies, biomolecules, and peptides to reduce antibiotic usage, whereas, preventatively, they can be used as either nano-antimicrobial additives or nano-photocatalytic sheets to reduce the microbial population and target the superbugs of environmental Escherichia. In this review, we have explored the threat posed by pathogenic Escherichia strains in the environment, especially in the context of antibiotic-resistant strains. Along with this, we have discussed some nanomaterial-mediated strategies in which the problem can be addressed by using nanomaterials as nanophotocatalytics, antimicrobial additives, drugs, and drug conjugates. This review also presents a brief overview of the ecological threats posed by the overuse of nanomaterials which warrants a balanced and judicious approach to the problem.
1. Introduction
Microbial pollution is the result of the increasing human population coupled with urbanization and animal husbandry. Among microbial pollutants, one of the primary representatives are from the genus Escherichia which are gram-negative, non-spore-forming, facultatively anaerobic, and rod-shaped bacteria. The species of the genus Escherichia are almost universal inhabitants of the gastrointestinal (GI) tract of homeotherms including humans, but unlike other genera of the same family such as Salmonella or Shigella, they are mostly non-pathogenic (Brock et al., 2003). The problem of microbial pollutants has been compounded by antibiotic micropollutants, which are also a by-product of the increasing population and originate both as effluents of pharmaceutical plants and cleared through the GI tract. Since the mobility of both gastrointestinal microbes, including opportunistic pathogens such as Escherichia coli and excreted micropollutants such as antibiotic residues, is waterborne and dictated by the water cycle, the solution must be at the point of concentration (sink) of both the microbe and antibiotic, which in this case are wastewater treatment plants. The anthropogenic concentration of Escherichia micropollutants and the proximity with antibiotic residues lead to the emergence of pathogenesis and antibiotic resistance which are a matter of great concern. In this review, we have focused on E. coli, which is one of the best-studied and characterized model organisms and a representative of its genus (Yu et al, 2021). In most cases, E. coli exists as a harmless commensal but can develop pathogenesis and lead to bacterium-related diarrhea, urinary tract infections, and meningitis (Palaniappan et al., 2006). The study of E. coli is also important as it is one of the bacteria which poses the greatest risk to human health due to its growing resistance to antibiotics and has been classified by the World Health Organization (WHO) along with the rest of the Enterobacteriaceae family as the 12 families of bacteria which pose the greatest threat to human health (Galindo-Méndez, 2020). Predictive statistical modeling of 23 pathogens has been used to estimate the global total deaths associated with and attributable to antimicrobial resistance of bacteria in 2019 to be 4.95 million and 1.27 million, respectively, with E. coli being responsible for most of the deaths with those associated with and attributable to antimicrobial resistant E. coli at 23.4% and 24.3%, respectively (Fernandez, 2022). Even without antimicrobial resistance, E. coli poses a significant health risk, as it is one of the major bacterial species contributing to diarrheal diseases and remains the second biggest killer of children below 5 years old after pneumonia, causing 0.437 million deaths annually (International Vaccine Access Center, 2020; Karambizi et al., 2021).
We have discussed the ecological role and changes of E. coli caused by the increased human population and also touched on the emergence of pathogenesis in the bacterium and how the problem is magnified in its artificial sink, wastewater treatment plants (WWTPs), where pathogenesis may provide survival benefit to the bacteria. In addition to pathogenesis, exposure to sublethal levels of antibiotics exerts a directional evolutionary selective pressure. Given the challenges, the use of nanoparticles (NPs) and associated nanomaterials has emerged as an attractive countermeasure either used in conjunction with antibiotics to reduce their effective dosage by increasing their efficiency or used on their own as nanotoxic additives or nanophotocatalytic sheets to neutralize microbial pollutants in their sink. The usage of nanomaterials is diversified in various sectors such as in industries, cosmetics, protective and self-cleaning materials, and most importantly, medicine. Recently, the use of nanoparticles showed remarkable antimicrobial properties. The properties of nanomaterials help in improving conjugation with antibiotics, providing better release, reducing the required dose, improving solubility, and enhancing better uptake and bioavailability. Novel nanomaterials are useful for both the source and the sink to address the problem. Nanoformulations can be used to increase the efficiency of antibiotic medication and, therefore, reduce their excretory load in wastewater. Also, their unique electro-optical and surface redox properties can help in reducing the micropollutant load in the sink, making them an ideal choice to address this concerning matter.
2. The ecological role of Escherichia coli
The gut microbiota accounts for 1010-1011 cells per gram of large intestinal content, consisting of more than 500 different species of bacterium (Tenaillon et al., 2010). Anaerobic bacteria outnumber E. coli 102–104 times; however, the latter can survive in environmental sediment, hence is most significant (Tenaillon et al., 2010). Escherichia coli is also one of the first microbes which colonize the human gut just after birth (Galindo-Méndez, 2020). The gut microbiota is a plastic ecosystem whose dynamics are determined by early microbial exposure, host genetics, and lifestyle (Fabbiano et al., 2017). The species E. coli presents an interesting ecological study due to the transition of the genus across mutualism, commensalism, and pathogenesis (Braz et al., 2020). In the mutualistic role, certain strains of E. coli are responsible for the production of vitamin K2 (menaquinone) which aids in clotting (Reygaert, 2017). The gut microbiota, including E. coli, contributes not only to host nutrition but also to host food metabolism, drug metabolism, and immune system development (Fabbiano et al., 2017). Gut microbiota including E. coli produces short-chain fatty acids (SCFA) which have been shown to play a role in the immune system and can reduce inflammation where chronic inflammation has been shown to play a role in colorectal cancer (Nakkarach et al., 2021). Healthy microbiota can also prevent the establishment and expansion of potentially pathogenic microorganisms in a process called colonization resistance either via bacteria–bacteria interventions or by triggering the host immune system (Sorbara & Pamer, 2019). Commensal strains of E. coli can provide colonization resistance against pathogenic serotypes and other genera such as Salmonella by resource competition for sugars present on the mucous layer or trace metals, respectively (Sorbara & Pamer, 2019). In its commensal role, E. coli still derives nutrition and protection, providing nothing significant in return. In the third form of interaction, pathogeny, certain strains of E. coli can cause serious illness, which may even lead to death.
Until recently, it was thought that E. coli is not able to survive and replicate for long outside a host; however, that has been challenged by the recent observation of the bacterium surviving for long periods in soil and sediment in tropical, subtropical, and temperate environments (Jang et al., 2017). As mentioned previously, the primary niche of E. coli is the colon of endotherms which includes cattle and humans from where it enters the environment with feces in the form of manure and sewage. Once in the environment, E. coli can survive for periods of time depending on a multitude of factors, including bacterial load, strain type, temperature, moisture, pH, oxygen, soil texture, and dissolved nutrients, particularly carbon (Van Elsas et al., 2011; Chekabab et al., 2013). Certain strains of the bacterium can respond in different ways including reducing cell size to increase surface area ratio or adopting a transport system with greater nutrient affinity; therefore, improving nutrient scavenging, morphological changes improving adhesion and colonization, and secretion of toxins as seen in certain pathotypes can also improve environmental survival and therefore be favored by natural selection (Van Elsas et al., 2011; Chekabab et al., 2013). Given that a high E. coli load is present in feces, the sewage system and wastewater treatment plants naturally serve as an artificial sink for the bacterium as it caters to highly dense habitations of humans in towns and cities. The sewage system and wastewater treatment plants provide many of the conditions which improve the survival of E. coli including an anaerobic environment rich in dissolved nutrients.
Figure 1 is a representation of the environmental cycle of E. coli and how it encounters antibiotic residue. As stated earlier, E. coli is mostly an intestinal commensal of warm-blooded animals, which includes in this context humans and livestock. Antibiotics are consumed by humans for therapeutic purposes and are administered to livestock for both therapeutic and prophylactic purposes to maximize economic productivity. In both cases, E. coli is excreted daily in the form of feces, where it may already be exposed to some antibiotic residues. Large quantities of livestock wastes are used as fertilizers, from where the E. coli can enter freshwater via surface runoff. On the other hand, human wastes, in the form of sewage, end up in wastewater treatment plants, where wastewater is mainly treated to separate debris and reduce organic matter. In a conventional wastewater treatment plant, the wastewater is first subjected to a grit and sand filter to remove debris. Then, it goes to the primary settling chamber to remove unstable colloidal contaminants. This is followed by an aeration tank to oxidize any organic matter into carbon dioxide and simpler organic compounds. Then, secondary settling is done with microbial additives, which feed on the simpler organic matter and in turn increase in bulk and create activated sludge, which is later reused and recycled. The final step includes disinfection with chlorination, ozonation, or UV radiation exposure, and finally, the cleaned water is released back into natural water. The problem with this method is that while it is good for the treatment of biological organic wastes, it is not purposely directed at treating specific ligands such as antibiotics. Micropollutants are often resistant to degradation due to their closed ring structure, non-biodegradability, and stability. Pharma micropollutants are present in low concentrations but are dangerous due to their persistence and, thus, possible ability to affect an organism’s entire life cycle (Petrovic et al., 2004; Gavrilescu et al., 2015). It is in the settling tanks of wastewater treatment plants where a large population of microbes including E. coli is brought in contact with antibiotic residues and organic matter. The microbial population is subjected to both directional evolutionary pressures as well as lateral gene transfer by mobile genetic elements and may develop antibiotic resistance. From here, the microbes and antibiotic residue may enter freshwater either via release and ineffective sterilization or through leakage. Freshwater with both microbial and antibiotic contamination is used for irrigation and drinking for both humans and livestock, whereas sludge from a wastewater treatment plant that contains microbial biomass is used as fertilizer on crops. The usage of both contaminated water and sludge for drinking, irrigation, and fertilizer and eating contaminated animal products may lead to bioaccumulation of antibiotic residues as well as exposure to antibiotic-resistant bacteria including E. coli. Animal husbandry and agriculture require large amounts of water to produce and process their products. Raw meat and vegetables are susceptible to carry large numbers of bacteria if processed with inadequately treated water (Rasheed et al., 2014). It is important to keep in mind that this is a multidirectional process.
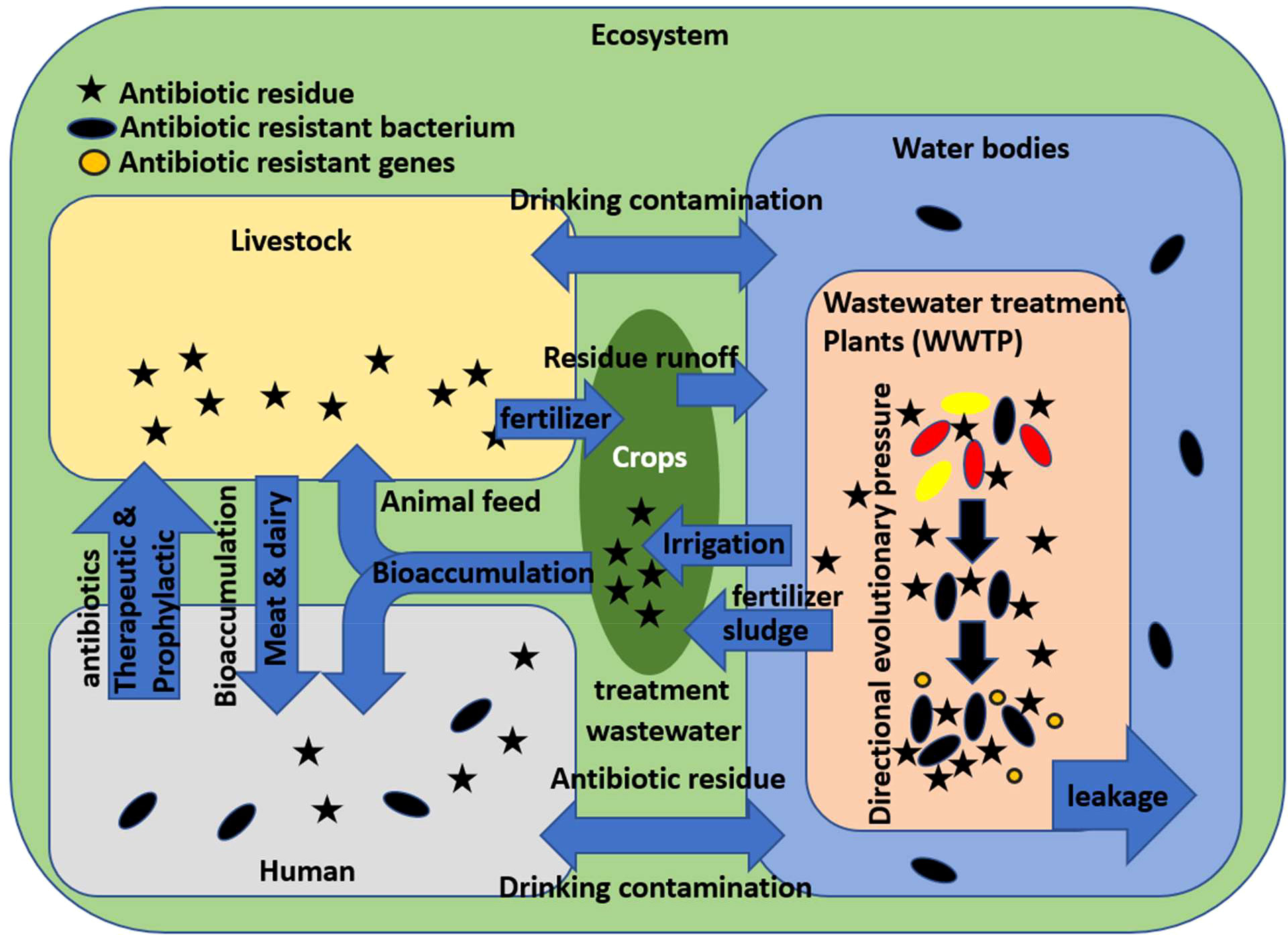
Figure 1 Ecological cycle of antibiotic resistance development in Escherichia coli and human exposure.
3. Pathogenesis in Escherichia coli and the role of antibiotics
The path to pathogenesis is rooted in the genome of E. coli which contains all the tools that the bacterium uses to adapt and survive in its microhabitat. Genomic evolution is driven by a multitude of internal and external forces including mutation, recombination, horizontal gene transfer (HGT), and directional evolutionary pressures. One reason behind the pathogenesis of E. coli is the substantial genetic plasticity of its pan-genome which may be thought of as a mosaic with a set of core and accessory genome, virulence genes, and antibiotic resistance in transmissible genetic elements which can easily be shared among different bacteria (Braz et al., 2020). These pathotypes have diversified their niche beyond the colon and into the small intestine, urinary tract, and meninges leading to diarrheal diseases, urinary tract infection (UTI), meningitis, and sepsis. Pathotypes have several morphological adaptations which aid in colonization and adhesion which help them establish themselves (Lawal et al., 2022). Escherichia coli is the most frequent cause of urinary tract infections and has been identified as the causative agent behind the disease in every anatomical site of the body leading to appendicitis, pneumonia, gastrointestinal infections, skin abscesses, endocarditis, meningitis, and intra-amniotic and puerperal infections in pregnant women (Galindo-Méndez, 2020).
A basic schematic of E. coli pathotypes is given in Figure 2. Based on the virulence factors present, pathogenic E. coli can be classified into the following: enteropathogenic E. coli (EPEC) which causes diarrhea in children and animals; enterohemorrhagic E. coli (EHEC) which causes hemorrhagic colitis and hemolytic-uremic syndrome; entero-invasive E. coli (EIEC) responsible for inflammatory diarrhea; enterotoxigenic E. coli (ETEC) which causes traveler’s, porcine, and bovine diarrhea; entero-aggravative E. coli (EAEC) which causes persistent diarrhea; diffusively adherent E. coli (DAEC) which is also responsible for diarrhea in children; entero-invasive E. coli which causes watery diarrhea and dysentery; uropathogenic E. coli (UPEC) which causes urinary tract infections; and neonatal meningitis E. coli (NMEC) which is responsible for meningitis and sepsis (Palaniappan et al., 2006; Kang et al., 2013; Rojas-Lopez et al., 2018).
This problem of E. coli microbial pollution is compounded by the fact that they are also exposed to antibiotic residues in sewage and wastewater treatment facilities. The consumption of antibiotics has increased to an estimated 14.2 defined daily doses (DDD) per 1,000 per day in 2018, which is a 46% increase from 2000 (Browne et al., 2021). Applying the principle of what goes in must also come out, undigested antibiotics, as well as residue molecules from not only humans but also cattle, are released into the sewage system. Antibiotics apply a directional evolutionary pressure on the E. coli microbes and lead to the development of antibiotic resistance genes which along with virulence-causing elements can be exchanged among E. coli microbes. The development of pathogenesis and antibiotic resistance poses a compounding risk of antibiotic-resistant pathogenic E. coli which poses a serious threat to human health. Antibiotic resistance may be a result of the bacterium’s inherent makeup, acquired traits, or active measures. Recent advances in whole genome sequencing have shed light on the genetic basis of the concern. Escherichia coli has a pan-genome of about 18,000 genes, with the average and core genomes of about 4,700 and 2,000 genes, and evolutionary pressures and horizontal gene transfer of genetic elements are the major reasons behind virulence and antibiotic resistance (Van Elsas et al., 2011; Poirel et al., 2018). Active measures which can be used by E. coli against drugs include limiting the uptake of the drug, modifying the drug target, drug inactivation, and drug efflux (Reygaert, 2017). One way to overpower bacterial countermeasures and effectively neutralize them before they enter the environment and cause diseases is via using nanomaterials. Nanomaterials can be used either as nanophotocatalysts that use solar or artificial radiation to physically lyse the cell or as nanotoxic additives for microbes or as conjugates to antibiotics to reduce their needed amount or improve their efficiency.
4. Threats posed by antibiotic-resistant Escherichia coli
As previously mentioned, the use of antibiotics has gone up considerably in the past few decades, especially in the developing world, for both human and livestock applications. This translates into a higher concentration of antibiotic residue in wastewater. Escherichia coli is a very common commensal organism, which inhabits the gastrointestinal tract of warm-blooded animals including humans and thus gets excreted into the sewage system with feces. The bacterium is subject to directional evolutionary pressure from these antibiotic residues, and using its substantial pan-genome, it is able to mobilize antibiotic resistance phenotypes. Antibiotics and antibiotic resistance are part of the natural arm’s race of the microbial world, which has been ongoing for millions of years; thus, microbes have developed antibiotic resistance, and new types of antibiotics have been developed to overcome them. Generally, the cytological and biochemical mechanism of antibiotic resistance can be divided into the following: antibiotic modification or degradation using special enzymes, antibiotic efflux using efflux pumps, and antibiotic sequestration using antibiotic binding proteins, target modification, bypass, or protection which includes strategies from methylation of the target macromolecule to increasing the production of macromolecule variants, which have much less binding affinity to the antibiotics (Peterson et al., 2018). In the case of gram-negative bacterium, two major contributors to intrinsic resistance are the outer membrane and the presence of numerous efflux pumps, which not only prevent the entry of many molecules but also rapidly reduce their intercellular concentration (Galindo-Méndez, 2020).
Data show that E. coli presents antibiotic resistance against drugs, which have been used for the longest time, as evidenced by the high degree of resistance against sulfonamides, which have been used globally since the 1930s, and resistant clones were first isolated as early as the 1950s (Galindo-Méndez, 2020). It is also not a coincidence that low- to mid-income countries, which have the highest consumption rates of antibiotics, have a higher rate of antibiotic resistance than high-income countries (Galindo-Méndez, 2020). Over the past decades, E. coli has resistance to major classes of antibiotics such as β-lactams, quinolones, aminoglycosides, sulfonamides, and fosfomycin. Unfortunately, this resistance has spread to the last resources of antibiotic classes such as polymyxins and carbapenems (Galindo-Méndez, 2020).
5. Nanophotocatalytic sheets for reduction in Escherichia coli load
Nanomaterials lie in a transitional realm between bulk and quantum phases of matter and are therefore endowed with special properties, one of them being photocatalysm. In a bulk crystalline conductor, the charge carriers are free flowing; thus, the electronic properties are indistinct. However, in the case where the size of the crystalline material decreases and reaches below the De Broglie electron wavelength, the charge-carrying electrons become confined, leading to unique properties (Daniel & Astruc, 2004; Neikov, 2009). One of these unique properties is called the quantum size effect (QSE) which arises due to the previously mentioned confinement of electrons which causes the edges of the valence band (VB) and conduction band (CB) to split, separated by an energy or band gap (Eg). The CB is electron-filled and of lower energy, whereas the VB is electron empty and of higher energy (Ajiboye et al., 2021). When a photon of sufficient energy equal to or greater than the band gap (Eg ≤ hv) is absorbed by the nanomaterial, electron(s) can be promoted from a lower energy valence band to a higher energy conduction band, leaving behind electron holes (Ajiboye et al., 2021). The exciton pair, electron, and electron hole can cause redox reactions either directly or via the formation of intermediate reactive oxygen species (ROS). Various nanomaterials include noble metals (Ag, Au, Pd), metal oxides, metal halides, metal sulfides, metal phosphides, non-metallic nanoparticles such as 3D-reduced graphene oxide (R-GO), doped metal nanomaterials by either other metals or non-metals, and heterojunction nanomaterials which combine wide and narrow band materials (Ajiboye et al., 2021). In addition to nanophotocatalysis, the nanomaterials themselves may show antimicrobial activity due to a multitude of reasons. The exact mechanism behind antimicrobial action is known but has been theorized to include ROS generation, metal ion leaching which can inhibit various biochemical and cytological processes, membrane destabilization, and altering of the cellular permeability (Rajak et al., 2020). Mechanical abrasion and damage of the cell membrane may be another mechanism of antimicrobial activity.
There are three possible ways theorized for the nano-inactivation of bacteria: DNA destruction, oxidative damage of the respiratory enzyme coenzyme A, and damage to the cell wall leading to cytolysis (Ajiboye et al., 2021). In the context of nanophotocatalysis when we are considering nanophotocatalytic sheets and surface reactions, the mechanism of cell membrane destruction is most relevant. The cell wall of E. coli is made up of a thick layer of proteins and sugar which prevents cytolysis (Ajiboye et al., 2021). Escherichia coli is a gram-negative bacterium which means that its cell wall consists of two lipid membranes with an intermediate peptidoglycan layer.
Damage to the bacterial cell wall may be further subdivided into mechanical damage, involving abrasion of the cell onto the nanostructures leading to physical ruptures and photocatalytic damage which depends on the spectra and intensity of incident radiation, concentration, size, morphology, and constitution of the nanophotocatalytic material. The characteristics of an ideal nanophotocatalyst are as follows: it is non-toxic by itself, is chemically and ecologically inert, and has a moderate band gap. Such nanomaterials can either be added in wastewater treatment plants or immobilized on a substratum and exposed to sunlight or artificial light. Figure 3 provides a representation of both a simplified gram-negative cell wall as well as the photocatalytic mode of destabilization of the cell membrane where minimal damage is done by contact alone if the nanomaterial has a smooth surface, and it is only on incident radiation and ROS generation that significant cell membrane destabilization and delamination occur.
Table 1 provides a summary of some nanophotocatalytic materials used for E. coli neutralization. Immobilization of nanomaterials onto substrates to form nanophotocatalytic screens can be broadly classified as non-covalent and covalent techniques. Non-covalent immobilization mainly involves the electrostatic attraction and adherence of nanomaterials onto a substratum by virtue of opposition of their surface charge. Nano-electrodeposition is a type of electrodeposition involving nanomaterials. The process has many advantages such as direct attachment or growth of nanomaterials on the substratum without a linker and more control over particle size, crystalline orientation, film thickness, and morphology (Mohanty, 2011).
An electrodeposition setup consists of the following components: an anode which may be the nanomaterial precursor or inert, a cathode which is the working electrode or where deposition is to be done, an electrolytic bath or solution, a voltage source, and a power management device. On application of a potential difference, electrons are replaced from the anode to the cathode, nanomaterial precursor ligand cations migrate to the cathode under the electric field, but reaction with a reductant present in the solution can cause agglomeration into nascent nanomaterial clusters, followed by growth and/or immobilization on the cathode (Cheon et al., 2011). Electrodeposition properties such as electrolytic bath constituents and concentration, working electrode surface area, temperature, time, current/voltage, and whether it is continuous or pulsed can be altered to fine-tune the morphology and size of deposited nanomaterials. Nanocomposites can be created depending on the electrodeposition in the presence of several nanomaterial precursor chemicals. If the nanomaterial has been prepared beforehand, then using the same technique can be electrodeposited on the substratum also.
Physical deposition techniques are cheaper and facile and employ dip or spin coating whereby the nanomaterial is suspended in a suitable solvent and the substratum is either dipped into the solvent or fixed on a rotating platform and a drop of solvent is placed followed by spinning. This results in a thin film of solvent and nanomaterials to be deposited on the substratum, and the film thickness can be controlled by the nanomaterial concentration in the solution and RPM. Vapor deposition is another technique that can be used for fabricating controlled nanolayers on a substratum. Vapor deposition can be further subdivided into chemical vapor deposition (CVD) and physical vapor deposition (PVD). In chemical vapor deposition, the nanomaterial precursor chemical is present in the gaseous phase (vapor) and is activated via heat, light, and plasma and then exposed to the substratum where it reacts to form stable solid nanostructures (Choy, 2003). PVD is performed in a high-vacuum environment where the target or precursor nanomaterial is present in a condensed form and is bombarded by a sputtering gas, detached atoms, under the influence of an electrical field deposit on the substratum (Safavi et al., 2021). An additional step of calcination can also be employed where the substratum with an overlaying thin film is subjected to high temperature for several hours which results in the evaporation of the solvent, any volatile impurities, and adhesion between the nanomaterial and underlying substratum.
Covalent immobilization techniques involve the covalent chemical linking of nanoparticles onto the substrate. The benefit of this method is that the nanomaterial can be synthesized and stored separately, and covalent bonds are strong, hence resistant to detachment on extreme changes in temperature, pH, electrical fields, and mechanical abrasion. Several crosslinking chemical reactions including NHS-EDC and biotinylation can be used. NHS-EDC crosslinking involves the formation of a stable NHS ester intermediate and ultimately links the carboxylic group with amine. Biotinylation is another crosslinking process involving the linking of biotin with another molecule such as streptavidin which binds it with high affinity. The requirement of such techniques is that the prerequisite chemical groups must be present on the surface of the nanomaterial and substratum. If not, then the nanomaterial or substratum can be coated with a suitable ligand to make those groups available for conjugation. The benefit of using nanophotocatalytic sheets instead of the addition of nanomaterials into wastewater directly is that it would limit nanomaterial discharge into the wastewater which would eventually end up in the hydrosphere and ecosystem.
6. Role of antimicrobial nanoparticles in mitigating Escherichia coli pollution
In the turbid conditions of wastewater treatment plants, nanophotocatalysts reduce in efficiency because of lower availability of light. In such cases, another property of nanomaterials, that is nanotoxicity or the antimicrobial nature of certain nanoformulations, can be used to address the issue. Certain properties of nanoparticles already prove its competency in various fields like drug delivery and sensing. The cell wall of the bacteria provides strength, stiffness, and structure to the cell, while it also helps against osmotic rupture and mechanical damage. The cell wall of any bacteria plays an important role in the diffusion of the nanoparticles in the cytoplasm of the cell. Several other factors can alter the bacteria’s susceptibility or tolerance toward nanoparticles such as pH, concentration, shape, size, charge, and chemical property. Copper oxide (CuO) NPs are extremely sensitive to E. coli, but Staphylococcus aureus and Bacillus subtilis are less susceptible. In another case, B. subtilis and S. aureus are more sensitive to ZnO NPs and NiO NPs than E. coli (Baek & An, 2011).
The mechanism of action and cellular response due to nanoparticle treatment are still areas of exploration. Nanoparticles adhere or attach to the cell membrane of the bacteria by electrostatic attraction and disrupt the membrane integrity which is shown in Figure 4. The nanoparticles and bacteria can interact directly by physical contact with the cell membrane or by inducing the irregular pits on the cell wall for the entry of ions in the cytoplasm. It was reported by Vazquez Munoz et al. that nanoparticle interaction with the cell wall can alter the potential of the cell membrane which enhances its permeability for foreign particles (Vazquez-Muñoz et al., 2019). The metallic nanoparticles destabilize the plasma membrane potential and lead to outer membrane destabilization and ATP depletion (Lok et al., 2006).
The properties of nanoparticles such as shape, size, and charge affect the tendency of transfection. Small-size nanoparticles have a higher internalization tendency in bacterial cells, and due to the high surface area to mass ratio, it can affect the bacterial function much predominantly as compared with larger nanoparticles. Other than the size of nanoparticles, shape also plays a vital role in interaction with cells. Among the other shapes of nanoparticles such as rod, sphere, tube, cube, plate, sheet, star, and triangle, the spherical ones are the most used shapes (Slavin et al., 2017). According to the study by Wang et al., the rod- and cube-shaped nanoparticles are more effective due to their high number of crystal facets (Zhipeng Wang et al., 2010). The higher facets with the atom density, the less energy it takes to produce oxygen vacancies, and the stronger the antibacterial action will be. In addition to this, the wire- and rod-shaped nanoparticles show high penetration power in bacterial cell wall as compared with the spherical ones. Talebian et al. reported a greater antibacterial tendency of the flower-shaped nanoparticles as compared with the spherical nanoparticles (Talebian et al., 2013). The charge of the nanoparticles is another factor that affects the transfection of nanoparticles inside the bacterial cell wall. The bacterial cell wall is mostly negatively charged, and the positively charged nanoparticles adhered securely on the bacterial surface before fusing with the cell wall, whereas the negatively charged nanoparticles show no attachment (Ivask et al., 2014). The cationic nanoparticles induce cell membrane damage by penetrating inside it and interfere with and alter the function of the electron transport system of the bacterial cell (Slavin et al., 2017). However, some studies reveal that surface modification of nanoparticles via using biocompatible polyethylene glycol, chitosan, polyethylene imine, and 3-aminopropyltriethoxysilane enhances cellular transfection and reduces the chance of rejection (Tamara et al., 2018).
7. Possible mechanisms of antibacterial nanoparticles
Several nanosystems showed antimicrobial tendency without the involvement of antimicrobial drugs. Nanosystems show antibacterial property through membrane disruption, ATP depletion, ROS generation, and DNA replication inhibition (Slavin et al., 2017; Tamara et al., 2018). Among various nanomaterials, the metal-based chitosan nanoparticles and surfactant-modified nanoparticles are used frequently for antibacterial action. After the uptake of nanoparticles, these internalized nanoparticles cause nanotoxicity by ROS production which results in the formation of oxidative stress, and it affects various cellular functions which are shown in Figure 5.
The mitochondria of the cell produce ATP by the reduction of molecular oxygen to water via a series of proton and electron transfer reactions as described in Figure 6. Sometimes, the oxygen is not completely reduced and forms harmful radicals such as superoxide, hydroxyl, hydrogen peroxide, and other oxygen radicals. The ROS plays a beneficial role in mitogenic responses and cellular signaling, but in the case of overproduction of ROS, it is hard for the cell to maintain normal physiological redox-based functions. It has been found from various studies that oxidative stress was the key reason in the case of inflammation, Parkinson’s disease, cardiovascular disease, Alzheimer’s disease, arthritis, amyotrophic lateral sclerosis, diabetes, and cancer (Halliwell, 1989; Bodamyali et al., 2000; Sachidanandam et al., 2005; Valko et al., 2007; Yin et al., 2009). The excessive ROS production by the nanoparticles inside the bacterial cytoplasm causes genotoxicity; oxidative DNA, lipid, and protein damage; mutagenesis; and carcinogenesis in humans (Abo-zeid and Williams, 2020).
The nanotoxicity caused by the overproduction of ROS and the extent of nanotoxicity caused by nanoparticles depend on its chemical nature, structure, shape, and size (Gonzalez et al., 2008). Compared with their bulk counterparts, nanoparticles have much higher surface area and reactivity which lead to high ROS generation leading to genotoxicity and cytotoxicity (Oberdörster et al., 2005). In a study, ZnO nanoparticles easily absorb UV light and produce hydrogen peroxide and superoxide anion, and in the case of TiO2, produce radicals after UV irradiation. The produced reactive species can easily diffuse inside the cell and cause cell death (Brunet et al., 2009; Sirelkhatim et al., 2015). Various nanoparticles used against E. coli are summarized in Table 2.
An additional benefit of using nanomaterials as either photocatalytic or toxicity-mediated bacteriostatic or bactericidal agents is that they can destroy microbes, which have already acquired antibiotic resistance and thus post an immediate threat of release and contamination. Microbes are also capable of acquiring resistance against nanomaterials but that would take multiple mutations and would be achieved through reducing membrane permeability for nanomaterials by more efficient efflux pumps (Lee et al., 2019). Therefore, by combining current therapeutics with nanomaterials, the number of mutations for effective resistance is increased, and thus, the chances that it would happen are reduced. A study involving exposure of ampicillin-resistant bacteria to AuNPs functionalized with ampicillin (AuNPs@Amp) showed that it had bactericidal effects (TA and Lu, 2012). This suggested that the nanoparticles and the antibiotics synergistically were able to overcome microbial antibiotic resistance. Nanomaterials are bacteriostatic or bactericidal in contact with microbes via photocatalytic or mechanical damage. Since these methods are non-specific, they would require significant membrane or cell wall modification to mitigate. Nanomaterial-mediated membrane damage is capable of destroying even multiple drug-resistant bacteria (B. Das et al., 2017).
8. Nanophotocatalytic destruction of antibiotic residues
Until now, we have discussed about the antimicrobial effects of nanomaterials; however, in order to address the issue of development and proliferation of antibiotic-resistant genes (ARGs) and antibiotic-resistant bacteria (ARBs) holistically, the antibiotic residues in wastewater as well as the genetic elements responsible for the lateral transmission of resistance also have to be addressed. As mentioned earlier, antibiotics are water-soluble and non-biodegradable, contributing to their persistence in the wastewater environment. Globally, some of the most used antibiotic classes are penicillins followed by cephalosporins (Klein et al., 2019) with about a quarter of the antibiotics being consumed in S. Asia led by India and about 10th by China (Browne et al., 2021). CdS-TiO2 nanoparticles were used.
Some studies using various nanophotocatalytic materials for the degradation of antibiotic residues in water are provided in Table 3. The efficiency of mineralization depends on several factors including the nature of the nanophotocatalyst and its concentration, the strength (wattage) and nature of incident radiation which reaches the nanophotocatalyst, and the concentration and type of antibiotic residue. An ideal nanophotocatalyst should have a suitable band gap to be able to use the visible spectra of light irradiated by the sun and not absorbed by the ozone layer. There are four distinct steps involved in nanophotocatalysis: first is the temporary adsorption of the pollutant or, in this case, antibiotic residue onto the nanophotocatalyst surface either due to random collision or electrostatic attraction. This is followed by exciton generation by the nanophotocatalyst, that is the generation of electron and hole pair after absorbing photonic radiation. The next step involves the migration of electron from the valence to the conduction band which is followed by effecting the redox degradation of the antibiotic residue either directly or through ROS intermediates which are formed by splitting of water molecules. The final step involves the desorption of antibiotic residues from the nanophotocatalyst surface. Complete mineralization involves the breaking down of antibiotic residues into carbon dioxide and water.
9. Nanophotocatalytic destruction of mobile genetic elements
As discussed previously, antibiotic resistance is developed in microbes including E. coli by directional evolution and stored as genetic instructions. These instructions can be exchanged in a process called horizontal gene transfer which uses extracellular naked DNA to transfer resistance genes to microbes which undergo transformation. Escherichia coli is not generally thought to undergo transformation naturally; however, under certain conditions where it is subject to stresses, it may become susceptible to transformation, which mainly involves the formation of membrane pores for the uptake of DNA (Hasegawa et al., 2018). DNA is a polyanion which is resistant to many environmental degradative effects by virtue of its strong phosphate backbone; therefore, nanophotocatalytic advanced oxidative processes are attractive in their active degradation.
Some studies of the degradation of genetic elements using nanophotocatalysis are provided in Table 4. As in the case of antibiotic degradation, the extent of degradation depends on the nanophotocatalyst used, its band gap and adsorptive capacity, and the incident radiation both in strength and spectral distribution, as well as the nature of the genetic element. It has been reported that the GC ratio is directly proportional to the rate of destruction (Ren et al., 2018). DNA degradation occurs on the phosphate backbone which converts plasmids into ssDNA and further on smaller oligonucleotides. DNA is a polyanion; hence, having a positively charged nanoparticle would increase electrostatic attraction.
10. Nanoparticle–antibiotic conjugates
The use of nanophotocatalysts is discussed in external environmental applications as nanophotocatalytic sheets where they would cause the mitigation of ARBs in wastewater. However, nanomaterials can also be used in conjugation with antibiotics to reduce their load on the environment. Nanomaterials alone are a good alternative for mitigating environmental pathogens. However, they lack specificity for microorganisms, thus causing various adverse effects on non-target eukaryotes (Bondarenko et al., 2013; Heim et al., 2015). According to studies in mice and rats, NPs, for example silver NPs, can trigger unexpected alterations in microbes, resulting in dysbiosis (Van Den Brûle et al., 2015; Williams et al., 2015). Therefore, one of the obstacles facing the development and deployment of NP-based treatments is the issue of specificity and the eradication of adverse effects. As compared with antibiotics, the benefit of using NPs is their capacity to negatively influence pathogens through numerous routes at the same time as discussed in the previous section, preventing bacterial resistance from developing (Wang et al., 2017). However, studies have reported for E. coli and Pseudomonas aeruginosa developing resistance to Ag NPs by producing flagellin, an adhesive protein, which causes NPs to aggregate and to lose their antibacterial effect (Panáček et al., 2018). Overall, bacterial resistance to NPs has not been well investigated, and further research is needed. Despite this, it can be hypothesized that bacterial resistance to NPs is certain because of selective pressure, even if it is not attributable to genetic alterations (Smerkova et al., 2020). A novel method of addressing this issue would be combining the antimicrobial properties of nanomaterials and antibiotics in a synergistic manner.
The rational encapsulation or conjugation of NPs with antibiotics has been one of the potential strategies for minimizing the detrimental effects of NPs and antibiotics on bacterial equilibrium and improving the selectivity of NPs toward specific pathogens. Antibiotics administered as antibiotic–NP conjugate forms at the same concentration as that of the free antibiotics had better inhibitory effects on bacterial growth, which might be explained by the enhanced penetration across the bacterial cell membrane, in-vivo stability, and specificity for intracellular targets (Christopher et al., 2021). Along with this, a higher concentration of antibiotics can be delivered inside the cells using these conjugates, which in turn limits the requirement of higher antibiotic dosage, thereby restricting its side effects (Kollef et al., 2011). In addition to this, pathogens that are multidrug-resistant pose the greatest threat to all antibiotics. The synergistic antibiotic release from NPs or several antibiotics conjugated or encapsulated on NPs provides further benefits in terms of bacterial killing effectiveness and speed.
The specific surface area of the antibiotic–NP system escalates as the size of the NPs decreases, allowing more antibiotic molecules to be functionalized onto the NP’s surface. The antibiotics can be linked physically or chemically to the NPs, without forming any core–corona structures. Hydrophobic, host–guest, avidin–biotin, and electrostatic interactions are all examples of physical interactions between antibiotics and NPs. In case of chemical interactions, different functional groups show conjugation with antibiotics by various means such as the following: amine-functionalized NP is conjugated with antibiotics that either contain a succinimidyl ester group or an isothiocyanate group, aldehyde-functionalized NP is conjugated with antibiotics that contain a hydrazide group, and active hydrogen-functionalized NP is conjugated with antibiotics that contain an amine group. AuNPs are conjugated with antibiotics that contain a sulfhydryl group, carboxyl acid-functionalized NPs are conjugated with antibiotics utilizing hexamethylene diisocyanate as a coupling agent and polyethylene glycol as a linker, alkyne-functionalized NPs are conjugated with antibiotics that contain an azide group, and tetrazine-functionalized NPs are conjugated with antibiotics that contain a trans-cyclooctene group (Jelinkova et al., 2019).
The antibiotic–nanomaterial system allows the desired amount of antibiotics to be delivered to the target sites with enhanced efficacy in cells. This can be accomplished via the following processes: i) antibiotic compounds can be hidden in antibiotic–nanomaterial conjugates during cellular penetration; ii) when antibiotics are administered at a high particle number concentration, bacteria are less likely to expel them through the efflux pumps; iii) when antibiotics are delivered in nanoscale form, they can be delivered more selectively and at a faster pace to the infection site. Due to differences in drug release kinetics, lower dosages of nanomaterial-conjugated multiple antibiotics are more efficacious than their molecular counterparts; iv) in infected cells, certain polymeric NPs have a higher biodegradability. Polymeric NPs can penetrate various membranes, break down inside the infected cell, and preferentially attach to contaminated components including the cytoplasm, macrophages, and phagolysosomes once delivered; v) inside cells, nanomaterials can preferentially accumulate and can capture microorganisms and prevent extracellular bacterial entrance into macrophages, reducing the severity of infections; vi) temperature, pH, light, ultrasound, magnetism, oxygen or carbon dioxide levels, ionic strength, and other stimuli-response characteristics of NP can be modified; and vii) multiple antibacterial pathways can be activated simultaneously by nanomaterials. A microbe’s ability to mutate is extremely unlikely. As a result, bacteria exposed to antibiotic–nanomaterial conjugates are less likely to acquire resistance (Mamun et al., 2021).
The antibiotic–nanomaterial conjugates work by combining the antibacterial property of the antibiotics with the advantages of the NPs providing a much better system for mitigating the development of drug-resistant pathogens. The mode of action for these antibiotic–nanomaterial conjugates for treating pathogens is very much similar to that of the NPs alone as discussed in the earlier sections. They mainly work by causing physical destruction of microbial cell structures, protein malfunction, membrane disintegration, ROS production and antioxidant depletion, and change of signal transduction through dephosphorylation of peptide substrates on tyrosine residues, resulting in signal transduction inhibition and bacterial growth suppression (Muzammil et al., 2018). Different examples of these antibiotic–nanomaterial conjugates used effectively for mitigating E. coli infection are given in Table 5.
11. Antibiotic–NP conjugates at the industrial level or in clinical trials
Vaccinations were initially viewed as a promising preventative strategy and an inhibitory tool against microbial infections. Vaccines have the tendency to reduce the need for antibiotics and the illness symptoms (Baker et al., 2018). The development of a glycoconjugate and recombinant DNA technique makes the vaccination more effective against resistant pathogens such as Pneumococcus, Haemophilus influenzae type B, Meningococcus, group B Streptococcus, Shigella, and E. coli (Bilukha & Rosenstein, 2005; Delany et al., 2014). In recent years, the effective usage of nano-antibiotics in medicine has been noted. A proprietary formulation known as liposomal amikacin for inhalation (ARIKAYCE®, created by Insmed Incorporated) wraps aqueous amikacin in charged neutral liposomes made of dipalmitoyl phosphatidylcholine and cholesterol. The USFDA has authorized this nanoformulation for the treatment of patients with Mycobacterium avium complex lung illness, who have failed conventional therapy and have few or no other therapeutic options (Cipolla et al., 2016). Commercially accessible liposomal-encapsulated ciprofloxacin (Lipoquin™ or ARD-3100 and Pulmaquin™ or ARD-3150), both produced by Aradigm Corporation for non-cystic fibrosis bronchiectasis colonized with P. aeruginosa, is also available (Cipolla et al., 2016). Other than this, numerous nano-antibiotics, including mupirocin, gentamicin, polymyxin B, fluconazole, and others, are also being used in therapeutic settings. Prevnar is a pneumococcal vaccine created by Wyeth that contains saccharides of seven serotypes of the capsular antigens of Streptococcus pneumoniae coupled to mutant CRM197 diphtheria toxoid (Bricks & Berezin, 2006; Chakraborty et al., 2022). Other than these commercial nanoformulations, there are various ongoing studies for the development of nanoformulations having antibacterial and antimicrobial properties. Many studies have been reported for the development of antibiotic-conjugated nanomaterials that can be effectively used to mitigate bacterial pollution. For example, M. Martínez-Carmona et al. reported a novel antibiotic–nanomaterial conjugate based on mesoporous silica nanoparticles (MSNs) loaded with the antibiotic levofloxacin (LEVO) and coated with the lectin concanavalin A (ConA) (Martínez-Carmona et al., 2019). The stable MSNs having an average size of 150 nm were synthesized and loaded with LEVO. The ConA was then covalently attached to the MSN for easy penetration into the gram-negative bacteria biofilms, which in turn escalated the efficacy of LEVO to a great extent. These LEVO-loaded MSNs showed a significant increase in the antimicrobial property of LEVO against bacterial biofilms (Martínez-Carmona et al., 2019). In another study, A. Aguilar-Colomer et al. reported the antimicrobial activity of antibiotic cargo produced by MSNs against several bacterial strains, including E. coli and S. aureus, in detail (Aguilar-Colomer et al., 2021). The MSNs loaded with three different antibiotics, gentamicin, levofloxacin, and rifampin, separately were used to study their released dosages’ biological activity as well as their impact on bacterial biofilm. The study showed that MSNs loaded with gentamicin had an uninterrupted release kinetics but minimal antibiofilm impact. On the other hand, levofloxacin and rifampin revealed release profiles that included an early burst effect followed by a continuous release, with active dosages capable of reducing up to 99.9% bacterial biofilm and remaining active for 72 h. This study paves the way for the development of tailored MSN-based nanotherapies to treat chronic bone infection (Aguilar-Colomer et al., 2021). J. Aguilera-Correa et al. reported a nanomedicine capable of eradicating E. coli-related bone infections, engineered using MSNs as a platform (Aguilera-Correa et al., 2022). These MSNs were loaded with moxifloxacin and were further modified with Arabic gum and colistin. This antibiotic–Np system displayed a significant affinity for the E. coli biofilm matrix and a pronounced antibacterial effect because of the bactericidal impact of moxifloxacin and the disaggregating effect of colistin. The results showed that, in vitro, the antibiotic–nanomaterial system was able to mitigate an infection on a trabecular bone, and in vivo, it demonstrated substantial antibacterial activity against E. coli-induced osteomyelitis. The antibiotic–nanomaterial system was found to be preferably non-cytotoxic, having a non-significant effect on cell proliferation and a slight hepatotoxicity, possibly due to the characteristics of both antibiotics (Aguilera-Correa et al., 2022).
12. Econanotoxicity
Nanotoxicology is a distinct and relatively recent branch of study, as the behavior of materials at the nano level can differ from the bulk counterpart and enable unique properties for uptake or tissue interactions (Nel et al., 2006). Nanotoxicology of a particular material depends on several factors ranging from size, shape, constituent material, surface structure, surface charge, electronic structure, surface ligands, and solubility (Nel et al., 2006; Cassano et al., 2017). Human exposure to nanomaterials is nothing new, as natural nanomaterials are found mostly in the form of particulate matter in the air. The source of such nanomaterials are mostly volcanic, dust storms, cosmic, and some organisms (Kumar et al., 2021). Nanomaterials have several avenues from which they can enter the body, specifically via respiratory, dermal, and gastrointestinal routes. Since nanomaterials for the treatment of wastewater are mostly immobilized by the hydrosphere, only the dermal and gastrointestinal exposure routes are relevant. In a study, it was noted that neutral and positively charged latex nanoparticles in the 50-500-nm range could not penetrate the dermis, while negative ones could (Kumar et al., 2021). In another study, it was found that nanoparticles of 18 nm are absorbed by the GI walls of a murine model. Furthermore, it was reported that positively charged and smaller nanoparticles are more readily absorbed by the GI tract (Kumar et al., 2021). A study using ZnO and TiO2 nanoparticles on skin penetration showed low toxicity, but it varies with the chemical nature, shape, and an increase in size with some exceptions (Nohynek et al., 2007). Positively charged nanoparticles seem to pass through the cell membranes more easily than negatively charged or neutral nanoparticles. One explanation for this would be that positively charged nanoparticles aid in the adsorption of proteins forming a corona, which promotes opsonization (Garcés et al., 2021). Once the nanoparticles enter the skin, respiratory system, or GI tract, they can enter the lymph nodes and finally into the systemic circulation, where they can accumulate in various organs along with some particles being able to cross the blood–brain barrier. A study of various nanoparticles such as TiO2, ZnO, Fe2O3, Fe3O4, and MgO showed that the mode of toxicity included cardiorespiratory damage at the organ level as well as ROS and DNA damage at the cellular level (Wang and Tang, 2021). As previously stated, humans and other organisms have co-evolved with natural nanoparticles; however, their exposure to engineered nanoparticles is relatively very recent; hence, there is no evolutionary defense mechanism. Nanotoxicity in aquatic ecosystems is a major and recent concern, as increasing amounts of engineered nanoparticles are being used and finding their way into the hydrosphere. Nanoparticles with redox or dissolving properties have the potential to disrupt cellular membrane stability (Khezerlou et al., 2018; Chakraborty et al., 2022). If they enter the cell, they cause oxidative damage, so in general, they have a bacteriostatic effect and, in large enough concentrations, bactericidal effects. Algae is a cornerstone of aquatic ecosystems, acting as producers and supporting the food web. Nanoparticles have been demonstrated to adhere to algal cell walls and internalized by endocytosis and then move up the trophic level from there via ecological bioaccumulation. The main effects on algae on exposure to diverse engineered nanoparticles include membrane damage, reduced chlorophyll content, oxidative stress, mitochondrial depolarization, and altered gene expression. It was found that exposure of silver nanoparticles at 0.4 - 6.4 mg/L to Dunaliella tertiolecta and Skeletonema costatum reduced growth by half (Abbas et al., 2020). Engineered nanoparticles are also uptaken and accumulated in the food vacuole of protozoans via endocytosis and phagocytosis, and they can enter the food chain from there, leading to biomagnification. Exposure of engineered nanoparticles in plants can also lead to a host of reactions, which depends on their mode of uptake and translocation throughout different parts of the plant. Since sludge from wastewater treatment plants is also used as fertilizers, this is particularly relevant. Exposure of Brassica oleracea and Lactuca sativa to 10 and 250 mg/plant of CuO nanoparticles led to a reduction in plant growth and photosynthesis, whereas CuO nanoparticles when exposed to Oryza sativa at 50–1,000 mg/kg also led to negative effects to plant growth (Abbas et al., 2020). Exposure of Ipomoea batatas to CuO, CeO2, and ZnO nanoparticles at 100-1,000 mg/kg again led to a reduction in biomass and bioaccumulation (Abbas et al., 2020). Select metal oxide nanoparticles such as TiO2, Al2O3, and ZnO were detected in Daphnia gut kept in a nanosuspension for 48 h with TiO2, showing a rapid accumulation in 12 h and slow excretion and still remaining in the body in 72 h (Krysanov et al., 2010). TiO2 nanoparticles did not accumulate in the internal organs of rainbow trout; however, accumulation could be seen in the muscles and liver, when kept in a nanosolution (Krysanov et al., 2010). Tin was detected in the gills, gut, and spleen of guppy kept in a nanosolution of SnO2, whereas zebrafish kept in ZnO nanosolution did not accumulate the same in the gills, liver, brain, and kidney, but when exposed to CeO2 nanoparticles, they could be detected in the liver (Krysanov et al., 2010). The elimination and biodegradation of NPs through urine and biliary channel is very low. This allows the NPs to remain in the tissues for a longer period of time and accumulate in the liver, lung, spleen, bone marrow, colon, and lymphatic system; other than this, the harmful by-products from NPs may lyse RBCs, cause DNA damage, and obstruct blood coagulation pathways and become more hazardous (Lei et al., 2008; Ansari et al., 2014; Ivask et al., 2014; Kandi & Kandi, 2015; Lin, 2015; Zaidi et al., 2017). Therefore, the effects of exposure to engineered nanoparticles on the environment on a macro level can be broadly said to reduce productivity and cause bioaccumulation.
As previously mentioned, biota have no evolutionary defense against engineered nanoparticles; hence, the toxicity of NPs is a matter of recent concern; however, not all studies have been conducted at environmentally realistic concentrations. Also, the same properties that make nanoparticles useful and their small and active nature also contribute to their removal from the environment. The possible fate of such nanomaterials in wastewater treatment plants includes adsorption onto large debris which are separated by grit filters, aggregation and settling out in primary or secondary settling chambers, interaction and removal by interaction with organic and microbial matter, and oxidative removal in advanced disinfection processes (Brar et al., 2010). Removal by adsorption of organic or microbial matter, which is a major constituent of sludge, is an important fate of nanomaterials in wastewater treatment plants; hence, sludge resultant immediately after any nanomaterial application can be collected and dried, and after incineration, the nanomaterial is recycled. Natural aquatic environments are a very complex system of water, salts, humic acids, sediments, microorganisms, substrata, etc. Nanoparticles with high surface charge reduce homoagglomeration but promote hetero-agglomeration due to electrostatic attraction. Engineered nanoparticles collide in aquatic media and stick to solid surfaces which slows their transportation and results in sedimentation (Bathi et al., 2021). Despite this, active measures should be taken to reduce exposure and nanotoxicity to a bare minimum. Econanotoxicity can be minimized in broadly two ways which can be classified under quantitative and qualitative parameters. Qualitative parameters include the manufacture of nanoparticles which are least damaging to the environment by factoring in parameters which in general contribute to their nanotoxicity. Engineered nanoparticles should have the size and charge or combined surface charge density so that they are impermeable to the dermis as well as the GI tract. If possible, they should be biodegradable so that they do not cause nanobioaccumulation. Nanoparticles should be chemically stable, i.e., on unintentional release they should permanently stay as sediment and mineralize. They should not leach pollutants or transform into secondary pollutants. To achieve this, their constituents should be as beguine as possible. The morphology of nanoparticles should also be kept non-abrasive so as to not cause membrane damage if they are unintentionally released and encounter microbiota. Green nanosynthesis using phytoextracts can also be used to minimize ecological footprint (Sarkar et al., 2021). As previously mentioned, since nanoparticles contain a surface charge, they can be separated using an electrostatic screen. There is also the option of using magnetic-engineered nanoparticles which can be separated using an applied magnetic field (Nasrollahi, 2020). Quantitative methods include the minimization of the amount of total engineered nanomaterials used; in this regard, nanomaterials have an advantage because of their extremely high surface area to volume or mass ratio, which means that relatively small amounts of the material are required. Secondly, since nanoparticle-mediated activity depends on the surface properties and not on bulk volume, the engineered nanoparticles can be immobilized as a unilayer on a screen substratum using electroplating or sputtering, thereby using a little material as possible. Minimizing of nanomaterial used and retrieval is particularly useful in the case of antimicrobial nanomaterials, which by virtue of their function must be nanotoxic. As mentioned previously, another reason for the minimization of nanomaterial usage is the fact that nanoparticles adhere to the cell wall or membrane and destabilize it, making it more permeable. This can in turn promote the transfection of mobile genetic elements containing antibiotic resistance. As revealed in a previous study, exposure to sublethal concentrations of ZnO nanoparticles was shown to greatly enhance horizontal gene transfer from E. coli, hence highlighting the need for their judicial use (Wang et al., 2018b).
13. Conclusion
Escherichia coli is a very common gram-negative commensal which mostly lives in the large intestine of warm-blooded animals without causing any harm. However, under certain circumstances, it may acquire traits that make it pathogenic. The threat is compounded when one considers excreted E. coli in conjunction with antibiotic pollution which ends up in the same sink, that is wastewater treatment plants. Here, E. coli has been found to survive under directional evolutionary stress caused by these antibiotics by developing select pathogenic traits and, as a result, may develop and transfer antibiotic resistance via mobile genetic units which pose the risk of drug-resistant E. coli strains. These drug-resistant strains or “superbugs” can lead to diseases and epidemics which are resistant or untreatable by antibiotics conventionally available and stockpiled. Nanomaterials present a novel method in which this issue can be mitigated. Nanomaterials can be used preventatively as antibiotic conjugates, increasing their efficiency and reducing their need and thus discharge into the environment. They can also be used punitively as nanophotocatalytic agents either to lyse the cell wall using artificial or natural sunlight or to disintegrate antibiotic residues and mobile genetic elements in the environment. They can also be used as antimicrobial additives to decrease microbial population in the sink and, thus, the threat posed to humans via several avenues. However, it is important to remember that ecological threats posed by excessive discharge of engineered nanomaterials are probable but poorly understood; hence, nanomaterials should be used judiciously after considering all aspects of efficiency, human health, and environmental integrity.
Author contributions
All authors listed have made a substantial, direct, and intellectual contribution to the work and approved it for publication.
Acknowledgments
MK is thankful for the research and development grant by the University of Delhi, Delhi, India. NS, PK, and AS are grateful for the following fellowships: UGC-CSIR fellowship no. F.16-6(DEC 2016)/2017(NET) (NS), CSIR fellowship no. 09/045(1646)/2019-EMR-1 (PK), and fellowship from the Dept. of Chemistry, Delhi University (AS).
Conflict of interest
The authors declare that the research was conducted in the absence of any commercial or financial relationships that could be construed as a potential conflict of interest.
Publisher’s note
All claims expressed in this article are solely those of the authors and do not necessarily represent those of their affiliated organizations, or those of the publisher, the editors and the reviewers. Any product that may be evaluated in this article, or claim that may be made by its manufacturer, is not guaranteed or endorsed by the publisher.
References
Abbas, Q., Yousaf, B., Ullah, H., Ali, M. U., Ok, Y. S., Rinklebe, J. (2020). Environmental transformation and nano-toxicity of engineered nano-particles (ENPs) in aquatic and terrestrial organisms. Crit. Rev. Environ. Sci. Technol. 50 (23), 2523–2581. doi: 10.1080/10643389.2019.1705721
Abo-zeid, Y., Williams, G. R. (2020). The potential anti-infective applications of metal oxide nanoparticles: A systematic review. Wiley Interdiscip. Reviews: Nanomed Nanobiotechnol 12 (2), e1592.
Adhikari, S., Banerjee, A., Eswar, N. K., Sarkar, D., Madras, G. (2015). Photocatalytic inactivation of e. coli by ZnO–Ag nanoparticles under solar radiation. RSC Adv. 5 (63), 51067–51077.
Aguilar-Colomer, A., Colilla, M., Izquierdo-Barba, I., Jimenez-Jimenez, C., Mahillo, I., Esteban, J., et al. (2021). Impact of the antibiotic-cargo from MSNs on gram-positive and gram-negative bacterial biofilms. Microporous Mesoporous Materials 311, 110681. doi: 10.1016/j.micromeso.2020.110681
Aguilera-Correa, J., Gisbert-Garzarán, M., Mediero, A., Carias-Cálix, R., Jiménez-Jiménez, C., Esteban, J., et al. (2022). Arabic Gum plus colistin coated moxifloxacin-loaded nanoparticles for the treatment of bone infection caused by escherichia coli. Acta Biomaterialia 137, 218–237. doi: 10.1016/j.actbio.2021.10.014
Ajiboye, T. O., Babalola, S. O., Onwudiwe, D. C. (2021). Photocatalytic inactivation as a method of elimination of e. coli from drinking water. Appl. Sci. 11 (3), 1313.
Al-Nabulsi, A., Osaili, T., Sawalha, A., Olaimat, A. N., Albiss, B. A., Mehyar, G., et al. (2020). Antimicrobial activity of chitosan coating containing ZnO nanoparticles against e. coli O157: H7 on the surface of white brined cheese. Int. J. Food Microbiol. 334, 108838.
Alzubaidy, M. W. M. (2022). Silver nanoparticles prepared by sol-gel method effect on escherichia coli as antimicrobial. Web Scientist: Int. Sci. Res. J. 3 (3), 100–113.
Ansari, M. A., Khan, H. M., Khan, A. A., Ahmad, M. K., Mahdi, A. A., Pal, R., et al. (2014). Interaction of silver nanoparticles with escherichia coli and their cell envelope biomolecules. J. basic Microbiol. 54 (9), 905–915. doi: 10.1002/jobm.201300457
Baaloudj, O., Assadi, A. A., Azizi, M., Kenfoud, H., Trari, M., Amrane, A., et al. (2021). Synthesis and characterization of ZnBi2O4 nanoparticles: photocatalytic performance for antibiotic removal under different light sources. Appl. Sci. 11 (9), 3975. doi: 10.3390/app11093975
Baek, Y.-W., An, Y.-J. (2011). Microbial toxicity of metal oxide nanoparticles (CuO, NiO, ZnO, and Sb2O3) to escherichia coli, bacillus subtilis, and streptococcus aureus. Sci. total Environ. 409 (8), 1603–1608. doi: 10.1016/j.scitotenv.2011.01.014
Baker, S. J., Payne, D. J., Rappuoli, R., De Gregorio, E. (2018). Technologies to address antimicrobial resistance. Proc. Natl. Acad. Sci. 115 (51), 12887–12895. doi: 10.1073/pnas.1717160115
Banoee, M., Seif, S., Nazari, Z. E., Jafari-Fesharaki, P., Shahverdi, H. R., Moballegh, A., et al. (2010). ZnO nanoparticles enhanced antibacterial activity of ciprofloxacin against staphylococcus aureus and escherichia coli. J. Biomed. Materials Res. Part B: Appl. Biomaterials 93 (2), 557–561. doi: 10.1002/jbm.b.31615
Bathi, J. R., Moazeni, F., Upadhyayula, V. K., Chowdhury, I., Palchoudhury, S., Potts, G. E., et al. (2021). Behavior of engineered nanoparticles in aquatic environmental samples: Current status and challenges. Sci. total Environ. 793, 148560. doi: 10.1016/j.scitotenv.2021.148560
Bellio, P., Luzi, C., Mancini, A., Cracchiolo, S., Passacantando, M., Di Pietro, L., et al. (2018). Cerium oxide nanoparticles as potential antibiotic adjuvant. effects of CeO2 nanoparticles on bacterial outer membrane permeability. Biochim. Biophys. Acta (BBA)-Biomembranes 1860 (11), 2428–2435.
Benrighi, Y., Nasrallah, N., Chaabane, T., Sivasankar, V., Darchen, A., Baaloudj, O. (2021). Photocatalytic performances of ZnCr2O4 nanoparticles for cephalosporins removal: Structural, optical and electrochemical properties. Optical Materials 115, 111035. doi: 10.1016/j.optmat.2021.111035
Bhattacharya, P., Neogi, S. (2017). Gentamicin coated iron oxide nanoparticles as novel antibacterial agents. Materials Res. Express 4 (9), 095005. doi: 10.1088/2053-1591/aa8652
Bilukha, O., Rosenstein, N. E. (2005). Prevention and control of meningococcal disease: recommendations of the advisory committee on immunization practices (ACIP). Centers for Disease Control and Prevention 54, RR–7.
Bodamyali, T., Stevens, C. R., Blake, D. R., Winyard, P. G. (2000). “Reactive oxygen/nitrogen species and acute inflammation: a physiological process,” In Free radicals and inflammation (Birkhäuser, Basel: Springer), 11–16. doi: 10.1007/978-3-0348-8482-2_2
Bondarenko, O., Juganson, K., Ivask, A., Kasemets, K., Mortimer, M., Kahru, A. (2013). Toxicity of Ag, CuO and ZnO nanoparticles to selected environmentally relevant test organisms and mammalian cells in vitro: a critical review. Arch. Toxicol. 87 (7), 1181–1200. doi: 10.1007/s00204-013-1079-4
Brar, S. K., Verma, M., Tyagi, R., Surampalli, R. (2010). Engineered nanoparticles in wastewater and wastewater sludge–evidence and impacts. Waste Manage. 30 (3), 504–520. doi: 10.1016/j.wasman.2009.10.012
Braz, V. S., Melchior, K., Moreira, C. G. (2020). Escherichia coli as a multifaceted pathogenic and versatile bacterium. Front. Cell. Infection Microbiol. 10, 548492. doi: 10.3389/fcimb.2020.548492
Bresee, J., Bond, C. M., Worthington, R. J., Smith, C. A., Gifford, J. C., Simpson, C. A., et al. (2014). Nanoscale structure–activity relationships, mode of action, and biocompatibility of gold nanoparticle antibiotics. J. Am. Chem. Soc. 136 (14), 5295–5300. doi: 10.1021/ja408505n
Bricks, L. F., Berezin, E. (2006). Impact of pneumococcal conjugate vaccine on the prevention of invasive pneumococcal diseases. Jornal Pediatria 82, s67–s74. doi: 10.2223/JPED.1475
Brock, T. D., Madigan, M. T., Martinko, J. M., Parker, J. (2003). Brock biology of microorganisms. Upper Saddle River (NJ): Prentice-Hall.
Browne, A. J., Chipeta, M. G., Haines-Woodhouse, G., Kumaran, E. P., Hamadani, B. H. K., Zaraa, S., et al. (2021). Global antibiotic consumption and usage in human 2000–18: a spatial modelling study. Lancet Planetary Health 5 (12), e893–e904. doi: 10.1016/S2542-5196(21)00280-1
Brunet, L., Lyon, D. Y., Hotze, E. M., Alvarez, P. J., Wiesner, M. R. (2009). Comparative photoactivity and antibacterial properties of C60 fullerenes and titanium dioxide nanoparticles. Environ. Sci. Technol. 43 (12), 4355–4360. doi: 10.1021/es803093t
Capeletti, L. B., de Oliveira, L. F., Goncalves, K., d., A., de Oliveira, J. F. A., Saito, A., et al. (2014). Tailored silica–antibiotic nanoparticles: overcoming bacterial resistance with low cytotoxicity. Langmuir 30 (25), 7456–7464. doi: 10.1021/la4046435
Carver, J. A., Simpson, A. L., Rathi, R. P., Normil, N., Lee, A. G., Force, M. D., et al. (2020). Functionalized single-walled carbon nanotubes and nanographene oxide to overcome antibiotic resistance in tetracycline-resistant escherichia coli. ACS Appl. Nano Materials 3 (4), 3910–3921. doi: 10.1021/acsanm.0c00677
Cassano, D., Pocoví-Martínez, S., Voliani, V. (2017). Ultrasmall-in-nano approach: Enabling the translation of metal nanomaterials to clinics. Bioconjugate Chem. 29 (1), 4–16.
Chakraborty, N., Jha, D., Roy, I., Kumar, P., Gaurav, S. S., Marimuthu, K., et al. (2022). Nanobiotics against antimicrobial resistance: harnessing the power of nanoscale materials and technologies. J. nanobiotechnology 20 (1), 1–25. doi: 10.1186/s12951-022-01573-9
Chekabab, S. M., Paquin-Veillette, J., Dozois, C. M., Harel, J. (2013). The ecological habitat and transmission of escherichia coli O157: H7. FEMS Microbiol. Lett. 341 (1), 1–12.
Cheon, J. M., Lee, J. H., Song, Y., Kim, J. (2011). Synthesis of Ag nanoparticles using an electrolysis method and application to inkjet printing. Colloids Surfaces A: Physicochemical Eng. Aspects 389 (1-3), 175–179. doi: 10.1016/j.colsurfa.2011.08.032
Choy, K. (2003). Chemical vapour deposition of coatings. Prog. materials Sci. 48 (2), 57–170. doi: 10.1016/S0079-6425(01)00009-3
Christopher, K. C., Rita, W. Y., Leung, S. S., Mamie, H. U. I., Ip, M. (2021). Overcoming the rising incidence and evolving mechanisms of antibiotic resistance by novel drug delivery approaches–an overview. Advanced Drug Delivery Rev. 181, 114078. doi: 10.1016/j.addr.2021.114078
Cipolla, D., Blanchard, J., Gonda, I. (2016). Development of liposomal ciprofloxacin to treat lung infections. Pharmaceutics 8 (1), 6. doi: 10.3390/pharmaceutics8010006
Daniel, M.-C., Astruc, D. (2004). Gold nanoparticles: Assembly, supramolecular chemistry, quantum-size-related properties, and applications toward biology, catalysis, and nanotechnology. Chem. Rev. 104 (1), 293–346. doi: 10.1021/cr030698+
Das, S., Sinha, S., Das, B., Jayabalan, R., Suar, M., AmritaMishra, A., et al. (2017). Disinfection of multidrug resistant escherichia coli by solar-photocatalysis using fe-doped ZnO nanoparticles. Sci. Rep. 7, 104. doi: 10.1038/s41598-017-00173-0
Delany, I., Rappuoli, R., De Gregorio, E. (2014). Vaccines for the 21st century. EMBO Mol. Med. 6 (6), 708–720. doi: 10.1002/emmm.201403876
Denisova, V., Mezule, L., Juhna, T. (2022). The effect of chitosan nanoparticles on escherichia coli viability in drinking water disinfection. Water Pract. Technol. 17 (2), 537–543. doi: 10.2166/wpt.2022.012
De Vietro, N., Tursi, A., Beneduci, A., Chidichimo, F., Milella, A., Fracassi, F., et al. (2019). Photocatalytic inactivation of escherichia coli bacteria in water using low pressure plasma deposited TiO2 cellulose fabric. Photochem Photobiol Sci. 18 (9), 2248–2258. doi: 10.1039/c9pp00050j
Djellabi, R., Ali, J., Zhao, X., Saber, A. N., Yang, B. (2020). CuO NPs incorporated into electron-rich TCTA@ PVP photoactive polymer for the photocatalytic oxidation of dyes and bacteria inactivation. J. Water Process Eng. 36, 101238. doi: 10.1016/j.jwpe.2020.101238
Durán, N., Marcato, P. D., Conti, R. D., Alves, O. L., Costa, F., Brocchi, M. (2010). Potential use of silver nanoparticles on pathogenic bacteria, their toxicity and possible mechanisms of action. J. Braz. Chem. Soc. 21 (6), 949–959. doi: 10.1590/S0103-50532010000600002
Evans, P., Sheel, D. (2007). Photoactive and antibacterial TiO2 thin films on stainless steel. Surface Coatings Technol. 201 (22-23), 9319–9324. doi: 10.1016/j.surfcoat.2007.04.013
Fabbiano, S., Suárez-Zamorano, N., Trajkovski, M. (2017). Host–microbiota mutualism in metabolic diseases. Front. Endocrinol. 267. doi: 10.3389/fendo.2017.00267
Fayaz, A. M., Balaji, K., Girilal, M., Yadav, R., Kalaichelvan, P. T., Venketesan, R. (2010). Biogenic synthesis of silver nanoparticles and their synergistic effect with antibiotics: a study against gram-positive and gram-negative bacteria. Nanomed: Nanotechnol Biol. Med. 6 (1), 103–109. doi: 10.1016/j.nano.2009.04.006
Fernandez, G. (2022). “Turning the juggernaut,” in Lancet Planetary Health .6. (LANGFORD LANE, KIDLINGTON, OXFORD OX5 1GB: ELSEVIER SCI LTD THE BOULEVARD), E75–E75.
Fernandez-Ibanez, P., Polo-López, M., Malato, S., Wadhwa, S., Hamilton, J., Dunlop, P., et al. (2015). Solar photocatalytic disinfection of water using titanium dioxide graphene composites. Chem. Eng. J. 261, 36–44. doi: 10.1016/j.cej.2014.06.089
Gabrielyan, L., Hakobyan, L., Hovhannisyan, A., Trchounian, A. (2019). Effects of iron oxide (Fe3O4) nanoparticles on escherichia coli antibiotic-resistant strains. J. Appl. Microbiol. 126 (4), 1108–1116. doi: 10.1111/jam.14214
Gaim, Y. T., Yimanuh, S. M., Kidanu, Z. G. (2022). Enhanced photocatalytic degradation of amoxicillin with Mn-doped Cu2O under sunlight irradiation. J. Composites Sci. 6 (10), 317. doi: 10.3390/jcs6100317
Galindo-Méndez, M. (2020). ntimicrobial resistance in Escherichia coli. E. Coli Infections-Importance of Early Diagnosis and Efficient Treatment, 1–20. London, UK: InTech Open.
Gankhuyag, S., Bae, D. S., Lee, K., Lee, S. (2021). One-pot synthesis of SiO2@ Ag mesoporous nanoparticle coating for inhibition of escherichia coli bacteria on various surfaces. Nanomaterials 11 (2), 549. doi: 10.3390/nano11020549
Garcés, M., Cáceres, L., Chiappetta, D., Magnani, N., Evelson, P. (2021). Current understanding of nanoparticle toxicity mechanisms and interactions with biological systems. New J. Chem. 45 (32), 14328–14344. doi: 10.1039/D1NJ01415C
Gavrilescu, M., Demnerová, K., Aamand, J., Agathos, S., Fava, F. (2015). Emerging pollutants in the environment: present and future challenges in biomonitoring, ecological risks and bioremediation. New Biotechnol. 32 (1), 147–156. doi: 10.1016/j.nbt.2014.01.001
Gonzalez, L., Lison, D., Kirsch-Volders, M. (2008). Genotoxicity of engineered nanomaterials: a critical review. Nanotoxicology 2 (4), 252–273. doi: 10.1080/17435390802464986
Gounani, Z., Asadollahi, M. A., Pedersen, J. N., Lyngsø, J., Pedersen, J. S., Arpanaei, A., et al. (2019). Mesoporous silica nanoparticles carrying multiple antibiotics provide enhanced synergistic effect and improved biocompatibility. Colloids Surfaces B: Biointerfaces 175, 498–508. doi: 10.1016/j.colsurfb.2018.12.035
Halliwell, B., Gutteridge, J. M. C. (1989). The chemistry of oxygen radicals and other derived species. In: Halliwell, B., Gutteridge, J. M. C. Eds. Free Radicals in Biology and Medicine. 2nd edn. Oxford: Clarendon Press, 22–85.
Hasegawa, H., Suzuki, E., Maeda, S. (2018). Horizontal plasmid transfer by transformation in escherichia coli: environmental factors and possible mechanisms. Front. Microbiol. 9, 2365. doi: 10.3389/fmicb.2018.02365
Heim, J., Felder, E., Tahir, M. N., Kaltbeitzel, A., Heinrich, U. R., Brochhausen, C., et al. (2015). Genotoxic effects of zinc oxide nanoparticles. Nanoscale 7 (19), 8931–8938. doi: 10.1039/C5NR01167A
Hetrick, E. M., Shin, J. H., Paul, H. S., Schoenfisch, M. H. (2009). Anti-biofilm efficacy of nitric oxide-releasing silica nanoparticles. Biomaterials 30 (14), 2782–2789. doi: 10.1016/j.biomaterials.2009.01.052
International Vaccine Access Center (IVAC), Johns Hopkins Bloomberg School of Public Health (2020). Pneumonia and diarrhea pro-gress report 2020. Available at: http://www.jhsph.edu/ivac/wp-content/uploads/2020/11/IVAC_PDPR_2020.pdf. Accessed 2021 Jan 11.
Ivask, A., ElBadawy, A., Kaweeteerawat, C., Boren, D., Fischer, H., Ji, Z., et al. (2014). Toxicity mechanisms in escherichia coli vary for silver nanoparticles and differ from ionic silver. ACS nano 8 (1), 374–386. doi: 10.1021/nn4044047
Jang, J., Hur, H. G., Sadowsky, M. J., Byappanahalli, M., Yan, T., Ishii, S. (2017). Environmental escherichia coli: ecology and public health implications–a review. J. Appl. Microbiol. 123 (3), 570–581. doi: 10.1111/jam.13468
Jelinkova, P., Mazumdar, A., Sur, V. P., Kociova, S., Dolezelikova, K., Jimenez, A. M. J., et al. (2019). Nanoparticle-drug conjugates treating bacterial infections. J. Controlled release 307, 166–185. doi: 10.1016/j.jconrel.2019.06.013
Jiang, W., Mashayekhi, H., Xing, B. (2009). Bacterial toxicity comparison between nano-and micro-scaled oxide particles. Environ. pollut. 157 (5), 1619–1625. doi: 10.1016/j.envpol.2008.12.025
Kalita, S., Kandimalla, R., Sharma, K. K., Kataki, A. C., Deka, M., Kotoky, J. (2016). Amoxicillin functionalized gold nanoparticles reverts MRSA resistance. Materials Sci. Engineering: C 61, 720–727. doi: 10.1016/j.msec.2015.12.078
Kandi, V., Kandi, S. (2015). Antimicrobial properties of nanomolecules: Potential candidates as antibiotics in the era of multi-drug resistance. Epidemiol. Health 37, e2015020. doi: 10.4178/epih/e2015020
Kang, G., Hart, C. A., Shears, P. (2013). Bacterial enteropathogens. In: Manson’s Tropical Diseases. 23rd edn. Edited by Farrar, J., Hotez, P., Junghanss, T., Kang, G., Lalloo, D., White, N. J.. Elsevier Health Sciences, 398–399.
Karambizi, N. U., McMahan, C. S., Blue, C. N., Temesvari, L. A. (2021). Global estimated disability-adjusted life-years (DALYs) of diarrheal diseases: A systematic analysis of data from 28 years of the global burden of disease study. PloS One 16 (10), e0259077. doi: 10.1371/journal.pone.0259077
Karaolia, P., Michael-Kordatou, I., Hapeshi, E., Drosou, C., Bertakis, Y., Christofilos, D., et al. (2018). Removal of antibiotics, antibiotic-resistant bacteria and their associated genes by graphene-based TiO2 composite photocatalysts under solar radiation in urban wastewaters. Appl. Catalysis B: Environ. 224, 810–824. doi: 10.1016/j.apcatb.2017.11.020
Kaushal, S., Kumar, A., Bains, H., Singh, P. P. (2022). Photocatalytic degradation of tetracycline antibiotic and organic dyes using biogenic synthesized CuO/Fe2O3 nanocomposite: Pathways and mechanism insights. Environ. Sci. Pollut. Res. 1–17. doi: 10.21203/rs.3.rs-1743967/v2
Khezerlou, A., Alizadeh-Sani, M., Azizi-Lalabadi, M., Ehsani, A. (2018). Nanoparticles and their antimicrobial properties against pathogens including bacteria, fungi, parasites and viruses. Microbial pathogenesis 123, 505–526. doi: 10.1016/j.micpath.2018.08.008
Klein, E. Y., Tseng, K. K., Pant, S., Laxminarayan, R. (2019). Tracking global trends in the effectiveness of antibiotic therapy using the drug resistance index. BMJ Global Health 4 (2), e001315. doi: 10.1136/bmjgh-2018-001315
Kollef, M. H., Golan, Y., Micek, S. T., Shorr, A. F., Restrepo, M. I. (2011). Appraising contemporary strategies to combat multidrug resistant gram-negative bacterial infections–proceedings and data from the gram-negative resistance summit. Clin. Infect. Dis. 53 (suppl_2), S33–S55. doi: 10.1093/cid/cir475
Krysanov, E. Y., Pavlov, D., Demidova, T., Dgebuadze, Y. (2010). Effect of nanoparticles on aquatic organisms. Biol. Bull. 37 (4), 406–412. doi: 10.1134/S1062359010040114
Kumar, V., Guleria, P., Ranjan, S., Dasgupta, N., Lichtfouse, E. (2021) Nanotoxicology and Nanoecotoxicology Vol. 159.
Lam, S.-M., Jaffari, Z. H., Sin, J.-C., Zeng, H., Lin, H., Li, H., et al. (2021). Surface decorated coral-like magnetic BiFeO3 with au nanoparticles for effective sunlight photodegradation of 2, 4-d and e. coli inactivation. J. Mol. Liquids 326, 115372. doi: 10.1016/j.molliq.2021.115372
Lawal, O. U., Parreira, V. R., Goodridge, L. (2022). The Biology and the Evolutionary Dynamics of Diarrheagenic Escherichia coli Pathotypes. intechopen 1–37. doi: 10.5772/intechopen.101567
Lee, N., Ko, W., Hsueh, P. (2019). Nanoparticles in the treatment of infections caused by multidrug-resistant organisms. Front Pharmacol. 10, 1153. doi: 10.3389/fphar.2019.01153
Lei, R., Wu, C., Yang, B., Ma, H., Shi, C., Wang, Q., et al. (2008). Integrated metabolomic analysis of the nano-sized copper particle-induced hepatotoxicity and nephrotoxicity in rats: a rapid in vivo screening method for nanotoxicity. Toxicol. Appl. Pharmacol. 232 (2), 292–301. doi: 10.1016/j.taap.2008.06.026
Lin, Z., Monteiro-Riviere, N. A., Riviere, J. E. (2015). Pharmacokinetics of metallic nanoparticles. Wiley Interdiscip. Reviews: Nanomed Nanobiotechnol 7 (2), 189–217. doi: 10.1002/wnan.1304
Li, Y., Yang, D., Wang, S., Li, C., Xue, B., Yang, L., et al. (2018). The detailed bactericidal process of ferric oxide nanoparticles on e. coli. Molecules 23 (3), 606. doi: 10.3390/molecules23030606
Lin, Z., Monteiro‐Riviere, N. A., Riviere, J. E.. (2015). Pharmacokinetics of metallic nanoparticles. Wiley Interdisciplinary Reviews: Nanomedicine and Nanobiotechnology 7 (2), 189–217. doi: 10.1002/wnan.1304
Lok, C.-N., Ho, C.-M., Chen, R., He, Q.-Y., Yu, W.-Y., Sun, H., et al. (2006). Proteomic analysis of the mode of antibacterial action of silver nanoparticles. J. Proteome Res. 5 (4), 916–924. doi: 10.1021/pr0504079
Luo, Z., Wu, Q., Zhang, M., Li, P., Ding, Y. (2011). Cooperative antimicrobial activity of CdTe quantum dots with rocephin and fluorescence monitoring for escherichia coli. J. colloid Interface Sci. 362 (1), 100–106. doi: 10.1016/j.jcis.2011.06.039
Mamun, M. M., Sorinolu, A. J., Munir, M., Vejerano, E. P. (2021). Nanoantibiotics: Functions and properties at the nanoscale to combat antibiotic resistance. Front. Chem. 9, 348. doi: 10.3389/fchem.2021.687660
Martínez-Carmona, M., Izquierdo-Barba, I., Colilla, M., Vallet-Regí, M. (2019). Concanavalin a-targeted mesoporous silica nanoparticles for infection treatment. Acta Biomaterialia 96, 547–556. doi: 10.1016/j.actbio.2019.07.001
Marugan, J., van Grieken, R., Sordo, C., Cruz, C. (2009). Corrigendum to “Kinetics of the photocatalytic disinfection of escherichia coli suspensions”[Appl. catal. b: Environ. 82 (2008) 27–36]. Appl. Catalysis B: Environ. 88 (3-4), 582–583.
Matboo, S. A., Nazari, S., Niapour, A., Niri, M. V., Asgari, E., Mokhtari, S. (2022). Antibacterial effect of TiO2 modified with poly-amidoamine dendrimer–G3 on S. aureus and E. coli in aqueous solutions. Water Sci. Technol 85 (2), 605–616. doi: 10.2166/wst.2022.007
Mirzaei, A., Chen, Z., Haghighat, F., Yerushalmi, L. (2019). Magnetic fluorinated mesoporous g-C3N4 for photocatalytic degradation of amoxicillin: transformation mechanism and toxicity assessment. Appl. Catalysis B: Environ. 242, 337–348. doi: 10.1016/j.apcatb.2018.10.009
Mohanty, U. (2011). Electrodeposition: a versatile and inexpensive tool for the synthesis of nanoparticles, nanorods, nanowires, and nanoclusters of metals. J. Appl. electrochemistry 41 (3), 257–270. doi: 10.1007/s10800-010-0234-3
Morones, J. R., Elechiguerra, J. L., Camacho, A., Holt, K., Kouri, J. B., Ramírez, J. T., et al. (2005). The bactericidal effect of silver nanoparticles. Nanotechnology 16 (10), 2346. doi: 10.1088/0957-4484/16/10/059
Muzammil, S., Hayat, S., Fakhar-E-Alam, M., Aslam, B., Siddique, M. H., Nisar, M. A., et al. (2018). Nanoantibiotics: Future nanotechnologies to combat antibiotic resistance. Front. Biosci. 10, 352–374. doi: 10.2741/e827
Nakkarach, A., Foo, H. L., Song, A. A.-L., Mutalib, N. E. A., Nitisinprasert, S., Withayagiat, U. (2021). Anti-cancer and anti-inflammatory effects elicited by short chain fatty acids produced by escherichia coli isolated from healthy human gut microbiota. Microbial Cell factories 20 (1), 1–17. doi: 10.1186/s12934-020-01477-z
Naqvi, S. Z. H., Kiran, U., Ali, M. I., Jamal, A., Hameed, A., Ahmed, S., et al. (2013). Combined efficacy of biologically synthesized silver nanoparticles and different antibiotics against multidrug-resistant bacteria. Int. J. nanomedi 8, 3187. doi: 10.2147/IJN.S49284
Nasrollahi, Z. (2020). Wastewater treatment using magnetic nanoparticles and nanocomposites. SRPH J. Fundam. Sci. Technol. 2 (4), 8–10. doi: 10.47176/sjfst.2.4.8
Neikov, O. D. (2009). “Nanopowders,” In Handbook of non-ferrous metal powders, 80–101. doi: 10.1016/B978-1-85617-422-0.00004-5
Nel, A., Xia, T., Madler, L., Li, N. (2006). Toxic potential of materials at the nanolevel. science 311 (5761), 622–627.
Nohynek, G. J., Lademann, J., Ribaud, C., Roberts, M. S. (2007). Grey goo on the skin? nanotechnology, cosmetic and sunscreen safety. Crit. Rev. Toxicol. 37 (3), 251–277. doi: 10.1080/10408440601177780
Oberdörster, G., Oberdörster, E., Oberdörster, J. (2005). Nanotoxicology: an emerging discipline evolving from studies of ultrafine particles. Environ. Health Perspect. 113, 823–839. doi: 10.1289/ehp.7339
Palaniappan, R. U., Zhang, Y., Chiu, D., Torres, A., DebRoy, C., Whittam, T. S., et al. (2006). Differentiation of escherichia coli pathotypes by oligonucleotide spotted array. J. Clin. Microbiol. 44 (4), 1495–1501. doi: 10.1128/JCM.44.4.1495-1501.2006
Panáček, A., Kvítek, L., Smékalová, M., Večeřová, R., Kolář, M., Röderová, M., et al. (2018). Bacterial resistance to silver nanoparticles and how to overcome it. Nat. nanotechnol 13 (1), 65–71.
Pant, H. R., Pant, B., Sharma, R. K., Amarjargal, A., Kim, H. J., Park, C. H., et al. (2013). Antibacterial and photocatalytic properties of Ag/TiO2/ZnO nano-flowers prepared by facile one-pot hydrothermal process. Ceramics Int. 39 (2), 1503–1510. doi: 10.1016/j.ceramint.2012.07.097
Pant, B., Park, M., Lee, J. H., Kim, H.-Y., Park, S.-J. (2017). Novel magnetically separable silver-iron oxide nanoparticles decorated graphitic carbon nitride nano-sheets: A multifunctional photocatalyst via one-step hydrothermal process. J. colloid Interface Sci. 496, 343–352. doi: 10.1016/j.jcis.2017.02.012
Patra, P., Mitra, S., Debnath, N., Pramanik, P., Goswami, A. (2014). Ciprofloxacin conjugated zinc oxide nanoparticle: A camouflage towards multidrug resistant bacteria. Bull. Materials Sci. 37 (2), 199–206. doi: 10.1007/s12034-014-0637-6
Pattnaik, S. P., Behera, A., Martha, S., Acharya, R., Parida, K. (2019). Facile synthesis of exfoliated graphitic carbon nitride for photocatalytic degradation of ciprofloxacin under solar irradiation. J. materials Sci. 54 (7), 5726–5742. doi: 10.1007/s10853-018-03266-x
Paudel, S., Peña-Bahamonde, J., Shakiba, S., Astete, C. E., Louie, S. M., Sabliov, C. M., et al. (2021). Prevention of infection caused by enteropathogenic e. coli O157: H7 in intestinal cells using enrofloxacin entrapped in polymer based nanocarriers. J. Hazardous Materials 414, 125454.
Perni, S., Piccirillo, C., Pratten, J., Prokopovich, P., Chrzanowski, W., Parkin, I. P., et al. (2009). The antimicrobial properties of light-activated polymers containing methylene blue and gold nanoparticles. Biomaterials 30 (1), 89–93. doi: 10.1016/j.biomaterials.2008.09.020
Peterson, A., Aas, S., Wasserman, D. (2018). “Selected Abstracts From the 2018 International Neuroethics Society Annual Meeting,” In Annual Meeting, AJOB Neuroscience 9 (4), W1–W21. doi: 10.1080/21507740.2018.1553906
Petrovic, M., Eljarrat, E., Lopez de Alda, M., Barceló, D. (2004). Endocrine disrupting compounds and other emerging contaminants in the environment: a survey on new monitoring strategies and occurrence data. Analytical Bioanalytical Chem. 378 (3), 549–562. doi: 10.1007/s00216-003-2184-7
Poirel, L., Madec, J.-Y., Lupo, A., Schink, A.-K., Kieffer, N., Nordmann, P., et al. (2018). Antimicrobial resistance in escherichia coli. Microbiol. Spectr. 6 (4), 6.4. 14. doi: 10.1128/microbiolspec.ARBA-0026-2017
Radovic-Moreno, A. F., Lu, T. K., Puscasu, V. A., Yoon, C. J., Langer, R., Farokhzad, O. C. (2012). Surface charge-switching polymeric nanoparticles for bacterial cell wall-targeted delivery of antibiotics. ACS nano 6 (5), 4279–4287. doi: 10.1021/nn3008383
Rajak, B. L., Kumar, R., Gogoi, M., Patra, S. (2020). “Antimicrobial activity of nanomaterials,” in Nanoscience in medicine. (Cham: Springer) 1, 147–185. doi: 10.1007/978-3-030-29207-2_5
Rasheed, M. U., Thajuddin, N., Ahamed, P., Teklemariam, Z., Jamil, K. (2014). Resistência microbiana a drogas em linhagens de escherichia coli isoladas de fontes alimentares. Rev. do Instituto Medicina Trop. São Paulo 56, 341–346.
Reddy, M. P., Venugopal, A., Subrahmanyam, M. (2007). Hydroxyapatite-supported Ag–TiO2 as escherichia coli disinfection photocatalyst. Water Res. 41 (2), 379–386. doi: 10.1016/j.watres.2006.09.018
Ren, S., Boo, C., Guo, N., Wang, S., Elimelech, M., Wang, Y. (2018). Photocatalytic reactive ultrafiltration membrane for removal of antibiotic resistant bacteria and antibiotic resistance genes from wastewater effluent. Environ. Sci. Technol. 52 (15), 8666–8673. doi: 10.1021/acs.est.8b01888
Reygaert, W. C. (2017). Antimicrobial mechanisms of escherichia coli Vol. 5 (London, UK: IntechOpen).
Rispoli, F., Angelov, A., Badia, D., Kumar, A., Seal, S., Shah, V. (2010). Understanding the toxicity of aggregated zero valent copper nanoparticles against escherichia coli. J. Hazardous Materials 180 (1-3), 212–216. doi: 10.1016/j.jhazmat.2010.04.016
Rojas-Lopez, M., Monterio, R., Pizza, M., Desvaux, M., Rosini, R. (2018). Intestinal pathogenic escherichia coli: insights for vaccine development. Front. Microbiol. 9, 440. doi: 10.3389/fmicb.2018.00440
Sachidanandam, K., Fagan, S. C., Ergul, A. (2005). Oxidative stress and cardiovascular disease: antioxidants and unresolved issues. Cardiovasc. Drug Rev. 23 (2), 115–132. doi: 10.1111/j.1527-3466.2005.tb00160.x
Safavi, M. S., Walsh, F. C., Surmeneva, M. A., Surmenev, R. A., Khalil-Allafi, J. (2021). Electrodeposited hydroxyapatite-based biocoatings: Recent progress and future challenges. Coatings 11 (1), 110. doi: 10.3390/coatings11010110
Sarkar, N., Sharma, R. S., Kaushik, M. (2021). Innovative application of facile single pot green synthesized CuO and CuO@ APTES nanoparticles in nanopriming of vigna radiata seeds. Environ. Sci. pollut. Res. 28 (11), 13221–13228. doi: 10.1007/s11356-020-11493-6
Shaikh, S., Rizvi, S. M. D., Shakil, S., Hussain, T., Alshammari, T. M., Ahmad, W., et al. (2017). Synthesis and characterization of cefotaxime conjugated gold nanoparticles and their use to target drug-resistant CTX-M-Producing bacterial pathogens. J. Cell. Biochem. 118 (9), 2802–2808. doi: 10.1002/jcb.25929
Shen, X.-C., Zhang, Z.-L., Zhou, B., Peng, J., Xie, M., Zhang, M., et al. (2008). Visible light-induced plasmid DNA damage catalyzed by a CdSe/ZnS-photosensitized nano-TiO2 film. Environ. Sci. Technol. 42 (14), 5049–5054. doi: 10.1021/es800668g
Sirelkhatim, A., Mahmud, S., Seeni, A., Kaus, N. H. M., Ann, L. C., Bakhori, S. K. M., et al. (2015). Review on zinc oxide nanoparticles: antibacterial activity and toxicity mechanism. Nano-micro Lett. 7 (3), 219–242. doi: 10.1007/s40820-015-0040-x
Slavin, Y. N., Asnis, J., Häfeli, U. O., Bach, H. (2017). Metal nanoparticles: understanding the mechanisms behind antibacterial activity. J. nanobiotechnology 15 (1), 1–20. doi: 10.1186/s12951-017-0308-z
Smekalova, M., Aragon, V., Panacek, A., Prucek, R., Zboril, R., Kvitek, L. (2016). Enhanced antibacterial effect of antibiotics in combination with silver nanoparticles against animal pathogens. Veterinary J. 209, 174–179. doi: 10.1016/j.tvjl.2015.10.032
Smerkova, K., Dolezelikova, K., Bozdechova, L., Heger, Z., Zurek, L., Adam, V. (2020). Nanomaterials with active targeting as advanced antimicrobials. Wiley Interdiscip. Reviews: Nanomed Nanobiotechnol 12 (5), e1636.
Sorbara, M. T., Pamer, E. G. (2019). Interbacterial mechanisms of colonization resistance and the strategies pathogens use to overcome them. Mucosal Immunol. 12 (1), 1–9.
Ta, B. A. S. K. S., Lu, J. (2012). Obare SO Scott ME. Appl. Environ. Microbiol 78, 2768–2774. doi: 10.1128/AEM.06513-11
Talebian, N., Amininezhad, S. M., Doudi, M. (2013). Controllable synthesis of ZnO nanoparticles and their morphology-dependent antibacterial and optical properties. J. Photochem. Photobiol. B: Biol. 120, 66–73. doi: 10.1016/j.jphotobiol.2013.01.004
Tamara, F. R., Lin, C., Mi, F.-L., Ho, Y.-C. (2018). Antibacterial effects of chitosan/cationic peptide nanoparticles. Nanomaterials 8 (2), 88. doi: 10.3390/nano8020088
Tenaillon, O., Skurnik, D., Picard, B., Denamur, E. (2010). The population genetics of commensal escherichia coli. Nat. Rev. Microbiol. 8 (3), 207–217. doi: 10.1038/nrmicro2298
Tiwari, D., Lee, S.-M., Kim, D.-J. (2022). Photocatalytic degradation of amoxicillin and tetracycline by template synthesized nano-structured Ce3+@ TiO2 thin film catalyst. Environ. Res. 210, 112914. doi: 10.1016/j.envres.2022.112914
Tsuang, Y. H., Sun, J. S., Huang, Y. C., Lu, C. H., Chang, W. H. S., Wang, C. C. (2008). Studies of photokilling of bacteria using titanium dioxide nanoparticles. Artif. Organs 32 (2), 167–174. doi: 10.1111/j.1525-1594.2007.00530.x
Valko, M., Leibfritz, D., Moncol, J., Cronin, M. T., Mazur, M., Telser, J. (2007). Free radicals and antioxidants in normal physiological functions and human disease. Int. J. Biochem. Cell Biol. 39 (1), 44–84. doi: 10.1016/j.biocel.2006.07.001
Van Den Brûle, S., Ambroise, J., Lecloux, H., Levard, C., Soulas, R., De Temmerman, P.-J., et al. (2015). Dietary silver nanoparticles can disturb the gut microbiota in mice. Particle fibre Toxicol. 13 (1), 1–16.
Van Elsas, J. D., Semenov, A. V., Costa, R., Trevors, J. T. (2011). Survival of escherichia coli in the environment: fundamental and public health aspects. ISME J. 5 (2), 173–183. doi: 10.1038/ismej.2010.80
Vazquez-Muñoz, R., Meza-Villezcas, A., Fournier, P., Soria-Castro, E., Juarez-Moreno, K., Gallego-Hernández, A., et al. (2019). Enhancement of antibiotics antimicrobial activity due to the silver nanoparticles impact on the cell membrane. PloS One 14 (11), e0224904. doi: 10.1371/journal.pone.0224904
Wang, X., Deng, A., Cao, W., Li, Q., Wang, L., Zhou, J., et al. (2018a). Synthesis of chitosan/poly (ethylene glycol)-modified magnetic nanoparticles for antibiotic delivery and their enhanced anti-biofilm activity in the presence of magnetic field. J. materials Sci. 53 (9), 6433–6449. doi: 10.1007/s10853-018-1998-9
Wang, W., Fang, J., Shao, S., Lai, M., Lu, C. (2017). Compact and uniform TiO2@ g-C3N4 core-shell quantum heterojunction for photocatalytic degradation of tetracycline antibiotics. Appl. Catalysis B: Environ. 217, 57–64. doi: 10.1016/j.apcatb.2017.05.037
Wang, L., Hu, C., Shao, L. (2017). The antimicrobial activity of nanoparticles: present situation and prospects for the future. Int. J. nanomedi 121227. doi: 10.2147/IJN.S121956
Wang, Z., Lee, Y.-H., Wu, B., Horst, A., Kang, Y., Tang, Y. J., et al. (2010). Anti-microbial activities of aerosolized transition metal oxide nanoparticles. Chemosphere 80 (5), 525–529. doi: 10.1016/j.chemosphere.2010.04.047
Wang, S., Liu, D., Yu, J., Zhang, X., Zhao, P., Ren, Z., et al. (2021). Photocatalytic penicillin degradation performance and the mechanism of the fragmented TiO2 modified by CdS quantum dots. ACS omega 6 (28), 18178–18189. doi: 10.1021/acsomega.1c02079
Wang, Z., Tang, M. (2021). Research progress on toxicity, function, and mechanism of metal oxide nanoparticles on vascular endothelial cells. J. Appl. Toxicol. 41 (5), 683–700. doi: 10.1002/jat.4121
Wang, X., Yang, F., Zhao, J. (2018b). Bacterial exposure to ZnO nanoparticles facilitates horizontal transfer of antibiotic resistance genes. Nano Impact 10, 61–67. doi: 10.1016/j.impact.2017.11.006
Williams, K., Milner, J., Boudreau, M. D., Gokulan, K., Cerniglia, C. E., Khare, S. (2015). Effects of subchronic exposure of silver nanoparticles on intestinal microbiota and gut-associated immune responses in the ileum of sprague-dawley rats. Nanotoxicology 9 (3), 279–289. doi: 10.3109/17435390.2014.921346
Woźniak-Budych, M. J., Przysiecka, Ł., Langer, K., Peplińska, B., Jarek, M., Wiesner, M., et al. (2017). Green synthesis of rifampicin-loaded copper nanoparticles with enhanced antimicrobial activity. J. Materials Science: Materials Med. 28 (3), 1–16. doi: 10.1007/s10856-017-5857-z
Yang, X., Wang, Y. (2008). Photocatalytic effect on plasmid DNA damage under different UV irradiation time. Building Environ. 43 (3), 253–257. doi: 10.1016/j.buildenv.2006.02.025
Yin, J.-J., Lao, F., Fu, P. P., Wamer, W. G., Zhao, Y., Wang, P. C., et al. (2009). The scavenging of reactive oxygen species and the potential for cell protection by functionalized fullerene materials. Biomaterials 30 (4), 611–621. doi: 10.1016/j.biomaterials.2008.09.061
Yu, D., Banting, G., Neumann, N. F. (2021). A review of the taxonomy, genetics, and biology of the genus escherichia and the type species escherichia coli. Can. J. Microbiol. 67 (8), 553–571. doi: 10.1139/cjm-2020-0508
Zaidi, S., Misba, L., Khan, A. U. (2017). Nano-therapeutics: a revolution in infection control in post antibiotic era. Nanomed: Nanotechnol Biol. Med. 13 (7), 2281–2301.
Zhang, J., Wang, J., Xu, H., Lv, X., Zeng, Y., Duan, J., et al. (2019). The effective photocatalysis and antibacterial properties of AgBr/AgVO 3 composites under visible-light. RSC Adv. 9 (63), 37109–37118. doi: 10.1039/C9RA06810D
Keywords: Escherichia pollution, microbial pollution, nanomaterial for mitigation, nano-intervention, antimicrobial nanoparticles, E. coli pollutants, antibiotic resistance, superbugs
Citation: Kaushik M, Sarkar N, Singh A and Kumar P (2023) Nanomaterials to address the genesis of antibiotic resistance in Escherichia coli. Front. Cell. Infect. Microbiol. 12:946184. doi: 10.3389/fcimb.2022.946184
Received: 17 May 2022; Accepted: 08 December 2022;
Published: 04 January 2023.
Edited by:
Xiumin Wang, Feed Research Institute (CAAS), ChinaReviewed by:
Ashish Kumar Singh, Jagatpur Post Graduate College, IndiaJingfeng Wang, Tianjin Institute of Environmental and Operational Medicine, China
Copyright © 2023 Kaushik, Sarkar, Singh and Kumar. This is an open-access article distributed under the terms of the Creative Commons Attribution License (CC BY). The use, distribution or reproduction in other forums is permitted, provided the original author(s) and the copyright owner(s) are credited and that the original publication in this journal is cited, in accordance with accepted academic practice. No use, distribution or reproduction is permitted which does not comply with these terms.
*Correspondence: Mahima Kaushik, bWthdXNoaWtAY2ljLmR1LmFjLmlu; a2F1c2hpa21haGltYTIwMTFAZ21haWwuY29t
†These authors have contributed equally to this work