- 1Max Planck Institute for Infection Biology, Berlin, Germany
- 2European Molecular Biology Laboratory, Hamburg Unit, Hamburg, Germany
- 3Centre for Structural Systems Biology, Hamburg, Germany
Malaria remains a leading cause of death and disease in many tropical and subtropical regions of the world. Due to the alarming spread of resistance to almost all available antimalarial drugs, novel therapeutic strategies are urgently needed. As the intracellular human malaria parasite Plasmodium falciparum depends entirely on the host to meet its nutrient requirements and the majority of its transmembrane transporters are essential and lack human orthologs, these have often been suggested as potential targets of novel antimalarial drugs. However, membrane proteins are less amenable to proteomic tools compared to soluble parasite proteins, and have thus not been characterised as well. While it had been proposed that P. falciparum had a lower number of transporters (2.5% of its predicted proteome) in comparison to most reference genomes, manual curation of information from various sources led to the identification of 197 known and putative transporter genes, representing almost 4% of all parasite genes, a proportion that is comparable to well-studied metazoan species. This transporter list presented here was compiled by collating data from several databases along with extensive literature searches, and includes parasite-encoded membrane-resident/associated channels, carriers, and pumps that are located within the parasite or exported to the host cell. It provides updated information on the substrates, subcellular localisation, class, predicted essentiality, and the presence or absence of human orthologs of P. falciparum transporters to quickly identify essential proteins without human orthologs for further functional characterisation and potential exploitation as novel drug targets.
Introduction
To sustain rapid growth within human red blood cells, Plasmodium falciparum requires sufficient nutrients and electrolytes for its active metabolism. Therefore, the parasite expresses a wide range of transport proteins to acquire substrates and efflux metabolites. As the majority of these carriers, channels, and pumps are predicted to be essential during intraerythrocytic stages (Martin, 2020) and have no identified human orthologs, these could be exploited as targets of novel drugs (Ludin et al., 2012). Due to the emergence of parasite resistance to most available antimalarials, new therapeutic strategies are urgently needed (Plowe, 2022). There are many reports on transporters associated with drug resistance (Cowell and Winzeler, 2019; Martin, 2020; Murithi et al., 2021; Shafik et al., 2022), and advances in the development of drugs that target solute transporters were recently reviewed (Belete, 2020; Monteiro Júnior et al., 2022). Here, an extended list of P. falciparum transport proteins is presented with many new additions and updated information on transporter localisation and essentiality based on experimental evidence and orthology inference.
The last two transporter lists were published in 2020 and 2016 and contained 117 (Martin, 2020) and 139 (Weiner and Kooij, 2016) proteins, corresponding to 2.2% and 2.6% of the predicted P. falciparum proteome, respectively. The localisation within the parasite-infected host cell was not indicated for all of these, as microscopic examination after endogenous tagging with fluorescent proteins or staining using specific antibodies was not conducted for all transporters. However, precise knowledge of the location of a transport protein and its orientation in the membrane is paramount for understanding its function and the dynamics of solute transport processes between cellular compartments. Therefore, the list presented here contains new information on subcellular localisation and function based on results from recent microscopy experiments (Edaye and Georges, 2015; Haase et al., 2021; Murithi et al., 2021; Wichers et al., 2021; Ahiya et al., 2022; Wichers et al., 2022), solubility assays, immunoprecipitation, proximity-dependent biotinylation or subcellular fractionation followed by immunoblot or proteomic analyses (Boucher et al., 2018; Balestra et al., 2021; Bullen et al., 2022), functional and structural studies (Shafik et al., 2020; Beck and Ho, 2021), the presence of targeting signals (Sayers et al., 2018; van Esveld et al., 2021), and Gene Ontology (GO) annotations (Blake et al., 2015). In addition, data on essentiality of P. falciparum genes are usually based on a large piggyBac screen (Zhang et al., 2018) that is known to contain some false-positive and false-negative results (Martin, 2020), highlighting the need for verification by other studies. Thus, results from the latest publications (Jiang et al., 2020; Swift et al., 2020; Oberstaller et al., 2021; Wichers et al., 2022) were included in the list along with information on the presence or absence of human orthologs, as this is important for therapeutic development and was not systematically specified previously. Of note, this mini review focuses mainly on asexual blood-stage parasites and also contains recent data on other stages, as transporters are likely important throughout the life cycle.
Plasmodium gene annotations are still incomplete with a large proportion of genes completely lacking characterisation of their function and localisation or only having sparse functional annotation deduced by orthology (Böhme et al., 2019). The lower number of genes representing the malaria transportome reported in earlier studies may be due to the lack of conventional transmembrane domains in some P. falciparum transporters (Desai, 2012) and difficult analysis by mass spectrometry. The reduced number of detected peptides (Lu et al., 2021) stems both from the typically low protein amounts extracted from parasite culture that are subjected to subcellular fractionation or immunoprecipitation and from the fact that membrane proteins such as transporters are less amenable to proteomics compared to soluble proteins. This has resulted in the conclusion that P. falciparum may have a reduced set of transporters compared to metazoan reference genomes (Weiner and Kooij, 2016; Martin, 2020).
Here, additional putative transporters were detected by compiling data from several databases (Aurrecoechea et al., 2009; Blake et al., 2015; Saier et al., 2016; Elbourne et al., 2017) and the literature. This mini review also covers newly identified putative calcium transporters (Balestra et al., 2021; Gupta et al., 2022), as calcium homeostasis is thought to be critical for all parasite stages (Brochet and Billker, 2016) and likely a promising drug target (Gupta et al., 2022). However, the molecular identity of most of the transporters involved in calcium transport has remained unclear (Lourido and Moreno, 2015), with contrasting results and conclusions regarding their substrates and subcellular localisation as well as the cellular compartment used for calcium storage (Brochet and Billker, 2016). The manually curated list of 197 transporter genes presented here represents almost 4% of 5720 P. falciparum 3D7 genes, of which 5318 are protein-coding (Aurrecoechea et al., 2009), a proportion that is comparable to the 3 – 5% reported for well-studied metazoan species (Elbourne et al., 2017). It includes the most recent published data and provides an updated overview on the substrates, localisation, function, classification, essentiality, and human orthologs of P. falciparum transporters and may serve as a basis for improved annotations of transporter genes and further functional characterisation of potential drug targets.
Approaches for Transport Protein Identification and Compilation of a Comprehensive List
Whole-genome sequencing, genome-wide searches and comparative genomics enabled the detection and fast annotation of many P. falciparum transporter genes by assigning functions that are computationally inferred from orthology across hundreds of species, facilitating functional characterisation at a large scale. However, molecular pathways and mechanisms that occur in parasites can differ tremendously from model organisms (Woo et al., 2015), and some known Plasmodium transporters are genus-specific and/or lack conventional transmembrane domains (Desai, 2012). Thus, function predictions based on the presence of protein features and on orthology inference harbour the possibility of incomplete or incorrect annotations. For example, PF3D7_1368200 was annotated as “ABC transporter E family member 1, putative (ABCE1)” due to its ATP-binding cassette that similar to that of ABC transporters (Koenderink et al., 2010). However, it is unlikely to be a transporter because of its function in RNA processing (Mather et al., 2007; Sinha et al., 2021), demonstrating the need for manual curation of GO terms and gene annotations.
The existing transporter list published in 2020 (Martin, 2020) was extended by collating data from various sources. Therefore, a table of 123 transport proteins from the P. falciparum strain 3D7 (genome version 3.0) with information on substrates, transporter classes and families was downloaded from http://www.membranetransport.org/transportDB2/index.html (Elbourne et al., 2017). Additional transporters associated with the GO term “transmembrane transporter activity” (GO:0022857) (Blake et al., 2015), mentioned on Malaria Parasite Metabolic Pathways (https://mpmp.huji.ac.il/maps/transporters.html) (Ginsburg and Tilley, 2011) or in research articles were included. For example, PfTMCO1 (transmembrane and coiled-coil domain-containing protein, PF3D7_1362300), identified based on orthology to proteins in other protozoan parasites (Gupta et al., 2022), was added. In contrast, glideosome-associated protein 40 (PfGAP40, PF3D7_0515700) and rhoptry protein PfROP14 (PF3D7_0613300) were removed, as new data on their function and localisation suggest that these are not transporters (Anantharaman et al., 2007; Zuccala et al., 2012; Ferreira et al., 2020).
As different names were sometimes used for the same protein (Weiner and Kooij, 2016; Staines et al., 2017; Martin, 2020), all alternative names found in the literature are mentioned in the table for clarification (Table 1). Transporter localisation, substrates and functions are indicated as in Martin, (2020) and predicted gene essentiality according to Zhang et al. (2018), unless stated otherwise. Transporter classes were assigned according to the Transport Classification Database (TCDB) (Saier et al., 2016) and if the transporter family was unknown, it was assigned according to the top TCDB blast hit (http://www.tcdb.org/progs/blast.php) based on sequence similarity to known transport proteins (Altschul et al., 1997). Data on the presence of human orthologs was retrieved from https://mpmp.huji.ac.il/maps/orth_hsap.html (Ginsburg and Tilley, 2011), a list compiled using recent publications. The existence of human orthologs was further verified using the TCDB protein blast.
In total, 197 transport proteins were identified (Table 1), with some of these forming a complex, e.g. the Plasmodium Translocon of EXported proteins (PTEX), consisting of three core components (de Koning-Ward et al., 2009; Beck and Ho, 2021). Protein complex components residing in or associated with the respective membrane that are required for substrate translocation were included, whereas accessory and auxiliary subunits were excluded. For clarity, only the likely site of active transport is indicated for each protein, although it might be detectable in other subcellular compartments during trafficking.
Calcium Transport Proteins as Potential Drug Targets
Calcium homeostasis was chosen as an example for illustrating transport pathways in the P. falciparum-infected erythrocyte (Figure 1), as Ca2+ signalling is known to be critical throughout the parasite life cycle (Brochet and Billker, 2016) and a link between Ca2+ uptake and virulence has been proposed in the related parasite Toxoplasma gondii (Pace et al., 2014). In fact, Ca2+ transporters such as PfATP6 (PF3D7_0106300) are currently under investigation as novel antimalarial drug targets (Gupta et al., 2022; Monteiro Júnior et al., 2022). While the concentration of free Ca2+ is ~1.8 mM in the blood plasma, mature erythrocytes only contain 30 – 60 nM Ca2+ (Brochet and Billker, 2016) due to active ion extrusion by the P-type plasma membrane Ca2+ ATPases (PMCA) 1 and 4 and slow Ca2+ uptake via several channels such as Piezo1, the erythroid N-methyl D-aspartate (NMDA) receptor, and the voltage-dependent anion channel (VDAC) (Kaestner et al., 2020).
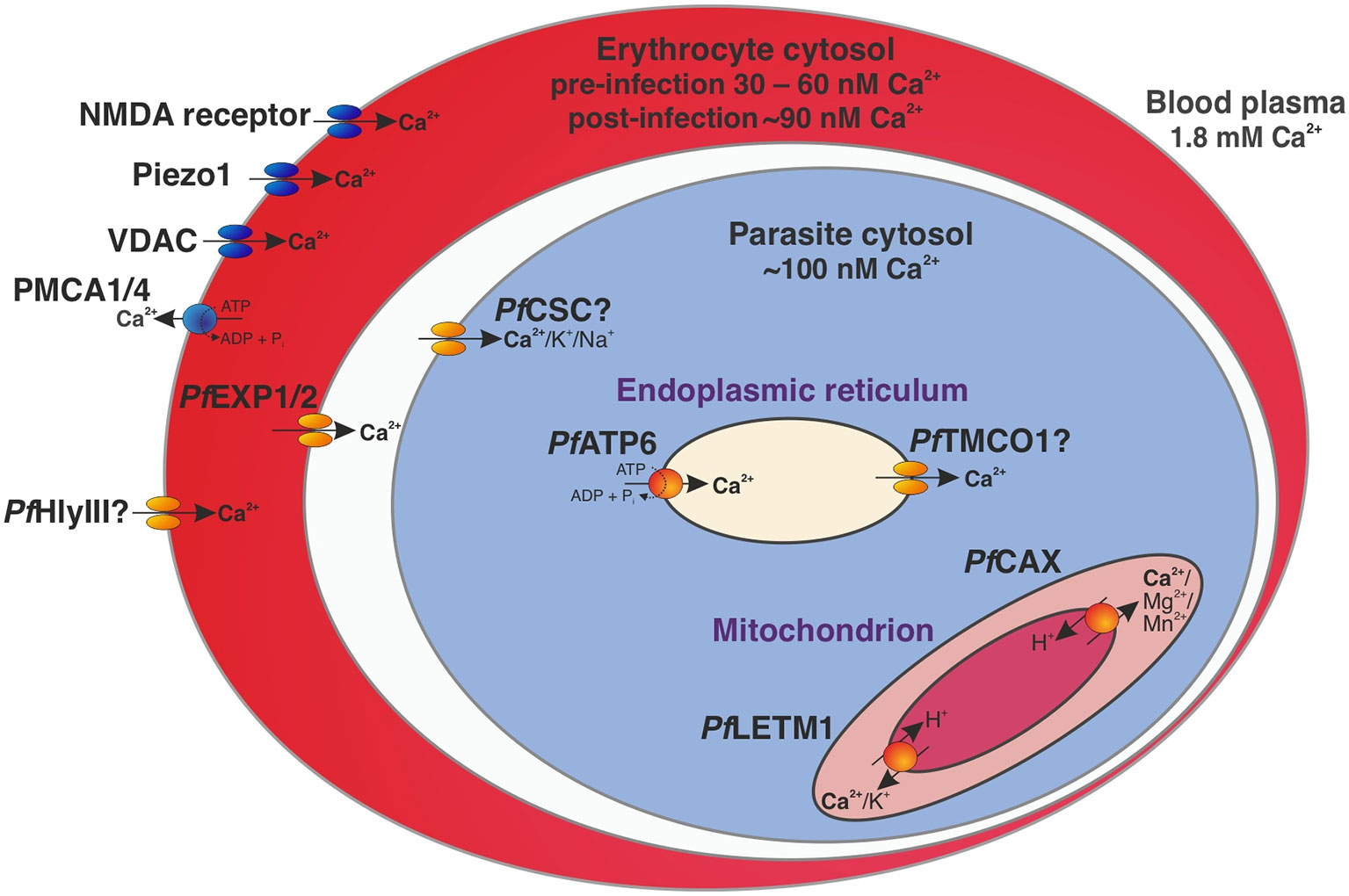
Figure 1 Calcium homeostasis in a trophozoite-stage P. falciparum-infected erythrocyte. Under resting conditions, the concentration of free Ca2+ is ~1.8 mM in the blood plasma, 30 – 60 nM in cytosol of an uninfected erythrocyte (Brochet and Billker, 2016), ~90 nM in the cytosol of the infected erythrocyte (Rohrbach et al., 2005), and ~100 nM in the cytosol of P. falciparum (Garcia et al., 1996). Transport proteins affecting intracellular calcium concentrations in the parasite-infected erythrocyte include the human P-type plasma membrane Ca2+-ATPases (PMCA) 1 and 4, human Piezo1, the erythroid N-methyl D-aspartate (NMDA) receptor, the voltage-dependent anion channel (VDAC) (Kaestner et al., 2020), and likely the parasite-encoded hemolysin III (PfHlyIII) (Moonah et al., 2014). A nutrient pore formed by PfEXP1 and PfEXP2 mediates passage through the parasitophorous vacuole membrane (Garten et al., 2018; Mesén-Ramírez et al., 2019) and the calcium-permeable stress-gated cation channel PfCSC may be responsible for Ca2+ entry into the parasite cytosol (Martin, 2020). The SERCA-type Ca2+-ATPase PfATP6 actively imports Ca2+ into the endoplasmic reticulum as an intracellular reservoir (Lourido and Moreno, 2015; Martin, 2020), while the putative calcium load-activated calcium channel PfTMCO1 (Gupta et al., 2022) may release ions back into the cytosol to avoid overload (Lourido and Moreno, 2015; Wang et al., 2016). Ca2+ efflux from the mitochondrion is likely mediated by the cation/H+ antiporters PfCAX (Rotmann et al., 2010) and PfLETM1 (Martin, 2020) via secondary active transport. Human-encoded transporters and channels are shown in blue and parasite-encoded proteins in orange.
A malaria parasite that resides within an erythrocyte maintains a cytosolic calcium level of approximately 100 nM by permeabilising its host cell and using a regulatory Ca2+ pool (Garcia et al., 1996). Extracellular Ca2+ is thought to first pass through a parasite-encoded channel in the erythrocyte plasma membrane (EPM) that is independent of PSAC (plasmodial surface anion channel), thereby increasing the intracellular Ca2+ concentration of the infected red blood cell (Zipprer et al., 2014). One candidate for this channel is hemolysin III (PfHlyIII, PF3D7_1455400), which forms an ion-permeable pore of approximately 3.2 nm in EPMs after its release from the parasite digestive vacuole (DV) upon merozoite egress (Moonah et al., 2014). Another potential route of Ca2+ entry into the infected erythrocyte is via enhanced activity of a host channel induced by the parasite, as suggested for VDAC (Bouyer et al., 2011).
Passage through the parasitophorous vacuole membrane (PVM) likely occurs via a nutrient pore for solutes < 1.4 kDa formed by PfEXP1 (PF3D7_1121600) and PfEXP2 (PF3D7_1471100) (Garten et al., 2018; Mesén-Ramírez et al., 2019). The ion may then enter the parasite cytosol via a parasite-encoded channel, one candidate being the calcium-permeable stress-gated cation channel PfCSC (PF3D7_1250200) that is activated by high external calcium levels (Martin, 2020). The localisation of this transporter at the PPM was inferred from an ancestral gene (Gaudet et al., 2011) and although this remains to be confirmed experimentally, it seems plausible due to the identification of this protein as an immunoreactive antigen with high serodominance in exposed individuals (Doolan et al., 2008). As PfCSC is highly expressed in sporozoites (Le Roch et al., 2003), its exposure to the immune system may occur at this parasite stage.
Calcium can then be stored in the endoplasmic reticulum upon active import by the SERCA-type Ca2+-ATPase PfATP6 (Lourido and Moreno, 2015; Martin, 2020). In case of Ca2+ overload of the ER, the putative calcium load-activated calcium channel PfTMCO1 (Gupta et al., 2022) may become active and release ions into the cytosol (Lourido and Moreno, 2015; Wang et al., 2016). Ca2+ efflux from the mitochondrion is likely mediated by the cation/H+ antiporters PfLETM1 (PF3D7_0417300) (Martin, 2020) and PfCAX/PfCHA (PF3D7_0603500) in exchange for protons that travel along the H+ gradient across the inner mitochondrial membrane (Rotmann et al., 2010).
Another putative intracellular Ca2+ pool may consist of acidocalcisomes – small electron-dense vesicles that are conserved from bacteria to humans and contain high concentrations of Ca2+, pyrophosphate, polyphosphate, iron, and zinc (Huang et al., 2014). Accordingly, acidocalcisome membranes contain a variety of specific transporters for these substrates across the tree of life (Huang et al., 2014). While many transporters were shown to reside in the acidocalcisome membrane in Trypanosoma brucei through proteomic studies and microscopy (Huang et al., 2014), no protein has been definitely localised to these organelles in P. falciparum (Magowan et al., 1997; Ruiz et al., 2004). Their low internal pH is likely required for the secondary active import of various ions and thought to be established and maintained by the plant-like H+-pump V-ATPase (Wunderlich et al., 2012; de Oliveira et al., 2021). This has yet to be verified experimentally, and there may be differences between parasite species. For example, PfVP1 (PF3D7_1456800), an ortholog of the acidocalcisome marker in T. brucei (Huang et al., 2014) and T. gondii (Rohloff et al., 2011), was previously suggested to localise to the parasite plasma membrane (PPM), DV and acidocalcisomes in P. falciparum, but could only be detected at the PPM by microscopy (Ahiya et al., 2022).
Other proteins that may translocate calcium and whose subcellular localisation has not yet been confirmed are PfATP9 (PF3D7_1348800), the putative calcium channel PF3D7_1331500, and PfICM1 (PF3D7_1231400). Elucidating their location and function is an important knowledge gap to be addressed (Kustatscher et al., 2022). Of the aforementioned putative Ca2+ transport proteins, PfICM1 and PfHlyIII may be worth exploring as drug targets due to their predicted essentiality and the absence of human counterparts.
Conclusions and Future Perspectives
This mini review consolidates data from various databases and provides an up-to-date overview of the subcellular localisation, function, predicted essentiality, and human orthologs of P. falciparum transporters for the fast identification of essential parasite transporters without human orthologs that may be promising novel targets for therapeutic development. Many of these candidates localise to the apicoplast, the mitochondrion, or the digestive vacuole, which are known to be “druggable” (Wunderlich et al., 2012; Oberstaller et al., 2021).
Moreover, the new transporter list will improve gene annotations and serve as a basis for further functional characterisation of the proteins. It will also be useful for systems biology approaches as it allows more reliable screening of e.g. genomic, transcriptomic, and proteomic data for P. falciparum transporters. The low coverage of the P. falciparum membrane proteome that complicates target profiling (Lu et al., 2021) may be overcome by large-scale culturing (Dalton et al., 2012) and more sensitive mass spectrometry techniques (McClure and Williams, 2018). Chemogenomic and transcriptional profiling of mutant-parasite libraries with altered drug sensitivities will further guide the determination of the mechanisms of drug action (Adjalley et al., 2015; Pradhan et al., 2015).
Author Contributions
The author confirms being the sole contributor of this work and has approved it for publication.
Funding
JW was supported by the Boehringer Ingelheim Foundation and the European Research Council under the European Union’s Horizon 2020 Research and Innovation Programme (grant agreement 759534).
Author Disclaimer
The funders had no role in study design, data collection, decision to publish, or preparation of the manuscript.
Conflict of Interest
The author declares that the research was conducted in the absence of any commercial or financial relationships that could be construed as a potential conflict of interest.
Publisher’s Note
All claims expressed in this article are solely those of the authors and do not necessarily represent those of their affiliated organizations, or those of the publisher, the editors and the reviewers. Any product that may be evaluated in this article, or claim that may be made by its manufacturer, is not guaranteed or endorsed by the publisher.
Acknowledgments
The author thanks Jan Strauss for providing many helpful tips and gratefully acknowledges Silvia Portugal and Eileen Devaney for critical reading of the manuscript.
References
Adjalley, S. H., Scanfeld, D., Kozlowski, E., Llinas, M., Fidock, D. A. (2015). Genome-Wide Transcriptome Profiling Reveals Functional Networks Involving the Plasmodium Falciparum Drug Resistance Transporters PfCRT and Pfmdr1. BMC Genomics 16, 1090. doi: 10.1186/s12864-015-2320-8
Agrawal, S., Striepen, B. (2010). More Membranes, More Proteins: Complex Protein Import Mechanisms Into Secondary Plastids. Protist 161 (5), 672–687. doi: 10.1016/j.protis.2010.09.002
Ahiya, A. I., Bhatnagar, S., Morrisey, J. M., Beck, J. R., Vaidya, A. B. (2022). Dramatic Consequences of Reducing Erythrocyte Membrane Cholesterol on Plasmodium Falciparum. Microbiol. Spectr. 10 (1), e0015822. doi: 10.1128/spectrum.00158-22
Altschul, S. F., Madden, T. L., Schäffer, A. A., Zhang, J., Zhang, Z., Miller, W., et al (1997). Gapped BLAST and PSI-BLAST: A New Generation of Protein Database Search Programs. Nucleic Acids Res. 25 (17), 3389–3402. doi: 10.1093/nar/25.17.3389
Anantharaman, V., Iyer, L. M., Balaji, S., Aravind, L. (2007). Adhesion Molecules and Other Secreted Host-Interaction Determinants in Apicomplexa: Insights From Comparative Genomics. Int. Rev. Cytol. 262, 1–74. doi: 10.1016/S0074-7696(07)62001-4
Aurrecoechea, C., Brestelli, J., Brunk, B. P., Dommer, J., Fischer, S., Gajria, B., et al. (2009). PlasmoDB: A Functional Genomic Database for Malaria Parasites. Nucleic Acids Res. 37 (Database issue), D539–DD43. doi: 10.1093/nar/gkn814
Balestra, A. C., Koussis, K., Klages, N., Howell, S. A., Flynn, H. R., Bantscheff, M., et al (2021). Ca2+ Signals Critical for Egress and Gametogenesis in Malaria Parasites Depend on a Multipass Membrane Protein That Interacts With PKG. Sci. Adv. 7 (13), eabe5396. doi: 10.1126/sciadv.abe5396
Beck, J. R., Ho, C. M. (2021). Transport Mechanisms at the Malaria Parasite-Host Cell Interface. PloS Pathog. 17 (4), e1009394. doi: 10.1371/journal.ppat.1009394
Belete, T. M. (2020). Recent Progress in the Development of New Antimalarial Drugs With Novel Targets. Drug Des. Devel. Ther. 14, 3875–3889. doi: 10.2147/DDDT.S265602
Blake, J. A., Christie, K. R., Dolan, M. E., Drabkin, H. J., Hill, D. P., Ni, L., et al(2015). Gene Ontology Consortium: Going Forward. Nucleic Acids Res. 43 (D1), D1049–D1D56. doi: 10.1093/nar/gku1179
Böhme, U., Otto, T. D., Sanders, M., Newbold, C. I., Berriman, M. (2019). Progression of the Canonical Reference Malaria Parasite Genome From 2002-2019. Wellcome Open Res. 4, 58. doi: 10.12688/wellcomeopenres.15194.1
Boucher, M. J., Ghosh, S., Zhang, L., Lal, A., Jang, S. W., Ju, A., et al (2018). Integrative Proteomics and Bioinformatic Prediction Enable a High-Confidence Apicoplast Proteome in Malaria Parasites. PloS Biol. 16 (9), e2005895. doi: 10.1371/journal.pbio.2005895
Bouyer, G., Cueff, A., Egée, S., Kmiecik, J., Maksimova, Y., Glogowska, E., et al (2011). Erythrocyte Peripheral Type Benzodiazepine Receptor/Voltage-Dependent Anion Channels are Upregulated by Plasmodium Falciparum. Blood 118 (8), 2305–2312. doi: 10.1182/blood-2011-01-329300
Brochet, M., Billker, O. (2016). Calcium Signalling in Malaria Parasites. Mol. Microbiol. 100 (3), 397–408. doi: 10.1111/mmi.13324
Bullen, H. E., Sanders, P. R., Dans, M. G., Jonsdottir, T. K., Riglar, D. T., Looker, O., et al (2022). The Plasmodium Falciparum Parasitophorous Vacuole Protein P113 Interacts With the Parasite Protein Export Machinery and Maintains Normal Vacuole Architecture. Mol. Microbiol 117 (5), 1245–1262. doi: 10.1111/mmi.14904
Cowell, A. N., Winzeler, E. A. (2019). Advances in Omics-Based Methods to Identify Novel Targets for Malaria and Other Parasitic Protozoan Infections. Genome Med. 11 (1), 63. doi: 10.1186/s13073-019-0673-3
Dalton, J. P., Demanga, C. G., Reiling, S. J., Wunderlich, J., Eng, J. W., Rohrbach, P. (2012). Large-Scale Growth of the Plasmodium Falciparum Malaria Parasite in a Wave Bioreactor. Int. J. Parasitol. 42 (3), 215–220. doi: 10.1016/j.ijpara.2012.01.001
Das, S., Lemgruber, L., Tay, C. L., Baum, J., Meissner, M. (2017). Multiple Essential Functions of Plasmodium Falciparum Actin-1 During Malaria Blood-Stage Development. BMC Biol. 15 (1), 70. doi: 10.1186/s12915-017-0406-2
de Koning-Ward, T. F., Gilson, P. R., Boddey, J. A., Rug, M., Smith, B. J., Papenfuss, A. T., et al (2009). A Newly Discovered Protein Export Machine in Malaria Parasites. Nature 459 (7249), 945–949. doi: 10.1038/nature08104
de Oliveira, L. S., Alborghetti, M. R., Carneiro, R. G., Bastos, I. M. D., Amino, R., Grellier, P., et al (2021). Calcium in the Backstage of Malaria Parasite Biology. Front. Cell Infect. Microbiol. 11, 708834. doi: 10.3389/fcimb.2021.708834
Desai, S. A. (2012). Ion and Nutrient Uptake by Malaria Parasite-Infected Erythrocytes. Cell Microbiol. 14 (7), 1003–1009. doi: 10.1111/j.1462-5822.2012.01790.x
Doolan, D. L., Mu, Y., Unal, B., Sundaresh, S., Hirst, S., Valdez, C., et al (2008). Profiling Humoral Immune Responses to P. Falciparum Infection With Protein Microarrays. Proteomics 8 (22), 4680–4694. doi: 10.1038/nature08104
Edaye, S., Georges, E. (2015). Characterization of Native PfABCG Protein in Plasmodium Falciparum. Biochem. Pharmacol. 97 (2), 137–146. doi: 10.1016/j.bcp.2015.06.035
Elbourne, L. D., Tetu, S. G., Hassan, K. A., Paulsen, I. T. (2017). TransportDB 2.0: A Database for Exploring Membrane Transporters in Sequenced Genomes From All Domains of Life. Nucleic Acids Res. 45 (D1), D320–D3D4. doi: 10.1093/nar/gkw1068
Ferreira, J. L., Heincke, D., Wichers, J. S., Liffner, B., Wilson, D. W., Gilberger, T. W. (2020). The Dynamic Roles of the Inner Membrane Complex in the Multiple Stages of the Malaria Parasite. Front. Cell Infect. Microbiol. 10, 611801. doi: 10.3389/fcimb.2020.611801
Friedrich, O., Reiling, S. J., Wunderlich, J., Rohrbach, P. (2014). Assessment of Plasmodium Falciparum PfMDR1 Transport Rates Using Fluo-4. J. Cell Mol. Med. 18 (9), 1851–1862. doi: 10.1111/jcmm.12313
Garcia, C., Dluzewski, A., Catalani, L., Burting, R., Hoyland, J., Mason, W. (1996). Calcium Homeostasis in Intraerythrocytic Malaria Parasites. Eur. J. Cell Biol. 71 (4), 409–413.
Garg, S., Agarwal, S., Kumar, S., Yazdani, S. S., Chitnis, C. E., Singh, S. (2013). Calcium-Dependent Permeabilization of Erythrocytes by a Perforin-Like Protein During Egress of Malaria Parasites. Nat. Commun. 4, 1736. doi: 10.1038/ncomms2725
Garten, M., Nasamu, A. S., Niles, J. C., Zimmerberg, J., Goldberg, D. E., Beck, J. R. (2018). EXP2 is a Nutrient-Permeable Channel in the Vacuolar Membrane of Plasmodium and is Essential for Protein Export via PTEX. Nat. Microbiol. 3 (10), 1090–1098. doi: 10.1038/s41564-018-0222-7
Gaudet, P., Livstone, M. S., Lewis, S. E., Thomas, P. D. (2011). Phylogenetic-Based Propagation of Functional Annotations Within the Gene Ontology Consortium. Brief Bioinform. 12 (5), 449–462. doi: 10.1093/bib/bbr042
Ginsburg, H., Tilley, L. (2011). Plasmodium Falciparum Metabolic Pathways (MPMP) Project Upgraded With a Database of Subcellular Locations of Gene Products. Trends Parasitol. 27 (7), 285–286. doi: 10.1016/j.pt.2011.03.001
Gupta, Y., Goicoechea, S., Pearce, C. M., Mathur, R., Romero, J. G., Kwofie, S. K., et al (2022). The Emerging Paradigm of Calcium Homeostasis as a New Therapeutic Target for Protozoan Parasites. Med. Res. Rev. 42 (1), 56–82. doi: 10.1002/med.21804
Haase, S., Condron, M., Miller, D., Cherkaoui, D., Jordan, S., Gulbis, J. M., et al (2021). Identification and Characterisation of a Phospholipid Scramblase in the Malaria Parasite Plasmodium Falciparum. Mol. Biochem. Parasitol. 243, 111374. doi: 10.1016/j.molbiopara.2021.111374
Hatin, I., Jambou, R., Ginsburg, H., Jaureguiberry, G. (1992). Single or Multiple Localization of ADP/ATP Transporter in Human Malarial Plasmodium Falciparum. Biochem. Pharmacol. 43 (1), 71–75. doi: 10.1016/0006-2952(92)90663-4
Hayashi, M., Yamada, H., Mitamura, T., Horii, T., Yamamoto, A., Moriyama, Y. (2000). Vacuolar H+-ATPase Localized in Plasma Membranes of Malaria Parasite Cells, Plasmodium Falciparum, is Involved in Regional Acidification of Parasitized Erythrocytes. J. Biol. Chem. 275 (44), 34353–34358. doi: 10.1074/jbc.M003323200
Huang, G., Ulrich, P. N., Storey, M., Johnson, D., Tischer, J., Tovar, J. A., et al (2014). Proteomic Analysis of the Acidocalcisome, an Organelle Conserved From Bacteria to Human Cells. PloS Pathog. 10 (12), e1004555. doi: 10.1371/journal.ppat.1004555
Jiang, Y., Wei, J., Cui, H., Liu, C., Zhi, Y., Jiang, Z., et al (2020). An Intracellular Membrane Protein GEP1 Regulates Xanthurenic Acid Induced Gametogenesis of Malaria Parasites. Nat. Commun. 11 (1), 1764. doi: 10.1038/s41467-020-15479-3
Kaestner, L., Bogdanova, A., Egee, S. (2020). Calcium Channels and Calcium-Regulated Channels in Human Red Blood Cells. Adv. Exp. Med. Biol. 1131, 625–648. doi: 10.1007/978-3-030-12457-1_25
Koenderink, J. B., Kavishe, R. A., Rijpma, S. R., Russel, F. G. (2010). The ABCs of Multidrug Resistance in Malaria. Trends Parasitol. 26 (9), 440–446. doi: 10.1016/j.pt.2010.05.002
Kustatscher, G., Collins, T., Gingras, A. C., Guo, T., Hermjakob, H., Ideker, T., et al (2022). Understudied Proteins: Opportunities and Challenges for Functional Proteomics. Nat. Methods. doi: 10.1038/s41592-022-01454-x
LaMonte, G., Lim, M. Y., Wree, M., Reimer, C., Nachon, M., Corey, V., et al (2016). Mutations in the Plasmodium Falciparum Cyclic Amine Resistance Locus (PfCARL) Confer Multidrug Resistance. mBio 7 (4), e00696–e00616. doi: 10.1128/mBio.00696-16
Le Roch, K. G., Zhou, Y., Blair, P. L., Grainger, M., Moch, J. K., Haynes, J. D., et al (2003). Discovery of Gene Function by Expression Profiling of the Malaria Parasite Life Cycle. Science 301 (5639), 1503–1508. doi: 10.1126/science.1087025
Lourido, S., Moreno, S. N. (2015). The Calcium Signaling Toolkit of the Apicomplexan Parasites Toxoplasma Gondii and Plasmodium Spp. Cell Calcium 57 (3), 186–193. doi: 10.1016/j.ceca.2014.12.010
Ludin, P., Woodcroft, B., Ralph, S. A., Mäser, P. (2012). In Silico Prediction of Antimalarial Drug Target Candidates. Int. J. Parasitol. Drugs Drug Resist. 2, 191–199. doi: 10.1016/j.ijpddr.2012.07.002
Lu, K. Y., Mansfield, C. R., Fitzgerald, M. C., Derbyshire, E. R. (2021). Chemoproteomics for Plasmodium Parasite Drug Target Discovery. Chembiochem 22 (16), 2591–2599. doi: 10.1002/cbic.202100155
Magowan, C., Brown, J. T., Liang, J., Heck, J., Coppel, R. L., Mohandas, N., et al (1997). Intracellular Structures of Normal and Aberrant Plasmodium Falciparum Malaria Parasites Imaged by Soft X-Ray Microscopy. Proc. Natl. Acad. Sci. U.S.A. 94 (12), 6222–6227. doi: 10.1073/pnas.94.12.6222
Maier, A. G., Rug, M., O'Neill, M. T., Brown, M., Chakravorty, S., Szestak, T., et al (2008). Exported Proteins Required for Virulence and Rigidity of Plasmodium Falciparum-Infected Human Erythrocytes. Cell 134 (1), 48–61. doi: 10.1016/j.cell.2008.04.051
Marapana, D. S., Dagley, L. F., Sandow, J. J., Nebl, T., Triglia, T., Pasternak, M., et al (2018). Plasmepsin V Cleaves Malaria Effector Proteins in a Distinct Endoplasmic Reticulum Translocation Interactome for Export to the Erythrocyte. Nat. Microbiol. 3 (9), 1010–1022. doi: 10.1038/s41564-018-0219-2
Marchesini, N., Luo, S., Rodrigues, C. O., Moreno, S. N., Docampo, R. (2000). Acidocalcisomes and a Vacuolar H+-Pyrophosphatase in Malaria Parasites. Biochem. J. 347 (Pt 1), 243–253. doi: 10.1042/bj3470243
Martin, R. E. (2020). The Transportome of the Malaria Parasite. Biol. Rev. Camb. Philos. Soc 95 (2), 305–332. doi: 10.1111/brv.12565
Mather, M. W., Henry, K. W., Vaidya, A. B. (2007). Mitochondrial Drug Targets in Apicomplexan Parasites. Curr. Drug Targets 8 (1), 49–60. doi: 10.2174/138945007779315632
McClure, R. A., Williams, J. D. (2018). Impact of Mass Spectrometry-Based Technologies and Strategies on Chemoproteomics as a Tool for Drug Discovery. ACS Med. Chem. Lett. 9 (8), 785–791. doi: 10.1021/acsmedchemlett.8b00181
Mesén-Ramírez, P., Bergmann, B., Tran, T. T., Garten, M., Stäcker, J., Naranjo-Prado, I., et al (2019). EXP1 is Critical for Nutrient Uptake Across the Parasitophorous Vacuole Membrane of Malaria Parasites. PloS Biol. 17 (9), e3000473. doi: 10.1371/journal.pbio.3000473
Monteiro Júnior, J. C., Krüger, A., Palmisano, G., Wrenger, C. (2022). Transporter-Mediated Solutes Uptake as Drug Target in Plasmodium Falciparum. Front. Pharmacol. 13, 845841. doi: 10.3389/fphar.2022.845841
Moonah, S., Sanders, N. G., Persichetti, J. K., Sullivan, D. J., Jr. (2014). Erythrocyte Lysis and Xenopus Laevis Oocyte Rupture by Recombinant Plasmodium Falciparum Hemolysin III. Eukaryot. Cell. 13 (10), 1337–1345. doi: 10.1128/EC.00088-14
Murithi, J. M., Deni, I., Pasaje, C. F. A., Okombo, J., Bridgford, J. L., Gnädig, N. F., et al (2021). The Plasmodium Falciparum ABC Transporter ABCI3 Confers Parasite Strain-Dependent Pleiotropic Antimalarial Drug Resistance. Cell Chem. Biol. 28, 1–16. doi: 10.1016/j.chembiol.2021.06.006
Nacer, A., Roux, E., Pomel, S., Scheidig-Benatar, C., Sakamoto, H., Lafont, F., et al (2011). Clag9 is Not Essential for PfEMP1 Surface Expression in non-Cytoadherent Plasmodium Falciparum Parasites With a Chromosome 9 Deletion. PloS One 6 (12), e29039. doi: 10.1371/journal.pone.0029039
Oberstaller, J., Otto, T. D., Rayner, J. C., Adams, J. H. (2021). Essential Genes of the Parasitic Apicomplexa. Trends Parasitol. 37 (4), 304–316. doi: 10.1016/j.pt.2020.11.007
Pace, D. A., McKnight, C. A., Liu, J., Jimenez, V., Moreno, S. N. (2014). Calcium Entry in Toxoplasma Gondii and its Enhancing Effect of Invasion-Linked Traits. J. Biol. Chem. 289 (28), 19637–19647. doi: 10.1074/jbc.M114.565390
Papalexis, V., Siomos, M. A., Campanale, N., Guo, X., Kocak, G., Foley, M., et al (2001). Histidine-Rich Protein 2 of the Malaria Parasite, Plasmodium Falciparum, is Involved in Detoxification of the by-Products of Haemoglobin Degradation. Mol. Biochem. Parasitol. 115 (1), 77–86. doi: 10.1016/S0166-6851(01)00271-7
Park, D. J., Lukens, A. K., Neafsey, D. E., Schaffner, S. F., Chang, H. H., Valim, C., et al (2012). Sequence-Based Association and Selection Scans Identify Drug Resistance Loci in the Plasmodium Falciparum Malaria Parasite. Proc. Natl. Acad. Sci. U.S.A. 109 (32), 13052–13067. doi: 10.1073/pnas.1210585109
Plowe, C. V. (2022). Malaria Chemoprevention and Drug Resistance: A Review of the Literature and Policy Implications. Malar. J. 21 (1), 104. doi: 10.1186/s12936-022-04115-8
Pradhan, A., Siwo, G. H., Singh, N., Martens, B., Balu, B., Button-Simons, K. A., et al (2015). Chemogenomic Profiling of Plasmodium Falciparum as a Tool to Aid Antimalarial Drug Discovery. Sci. Rep. 5, 15930. doi: 10.1038/srep15930
Rohloff, P., Miranda, K., Rodrigues, J. C., Fang, J., Galizzi, M., Plattner, H., et al (2011). Calcium Uptake and Proton Transport by Acidocalcisomes of Toxoplasma Gondii. PloS One 6 (4), e18390. doi: 10.1371/journal.pone.0018390
Rohrbach, P., Friedrich, O., Hentschel, J., Plattner, H., Fink, R. H., Lanzer, M. (2005). Quantitative Calcium Measurements in Subcellular Compartments of Plasmodium Falciparum-Infected Erythrocytes. J. Biol. Chem. 280 (30), 27960–27969. doi: 10.1074/jbc.M500777200
Rotmann, A., Sanchez, C., Guiguemde, A., Rohrbach, P., Dave, A., Bakouh, N., et al (2010). PfCHA is a Mitochondrial Divalent Cation/H+ Antiporter in Plasmodium Falciparum. Mol. Microbiol. 76 (6), 1591–1606. doi: 10.1111/j.1365-2958.2010.07187.x
Ruiz, F. A., Luo, S., Moreno, S. N., Docampo, R. (2004). Polyphosphate Content and Fine Structure of Acidocalcisomes of Plasmodium Falciparum. Microsc. Microanal. 10 (5), 563–567. doi: 10.1017/S1431927604040875
Saier, M. H., Jr., Reddy, V. S., Tsu, B. V., Ahmed, M. S., Li, C., Moreno-Hagelsieb, G. (2016). The Transporter Classification Database (TCDB): Recent Advances. Nucleic Acids Res. 44 (D1), D372–D3D9. doi: 10.1093/nar/gkv1103
Sassmannshausen, J., Pradel, G., Bennink, S. (2020). Perforin-Like Proteins of Apicomplexan Parasites. Front. Cell Infect. Microbiol. 10, 578883. doi: 10.3389/fcimb.2020.578883
Sayers, C. P., Mollard, V., Buchanan, H. D., McFadden, G. I., Goodman, C. D. (2018). A Genetic Screen in Rodent Malaria Parasites Identifies Five New Apicoplast Putative Membrane Transporters, One of Which is Essential in Human Malaria Parasites. Cell Microbiol. 20 (1), e12789. doi: 10.1111/cmi.12789
Schmidt, O., Pfanner, N., Meisinger, C. (2010). Mitochondrial Protein Import: From Proteomics to Functional Mechanisms. Nat. Rev. Mol. Cell Biol. 11 (9), 655–667. doi: 10.1038/nrm2959
Shafik, S. H., Cobbold, S. A., Barkat, K., Richards, S. N., Lancaster, N. S., Llinás, M., et al (2020). The Natural Function of the Malaria Parasite's Chloroquine Resistance Transporter. Nat. Commun. 11 (1), 3922. doi: 10.1038/s41467-020-17781-6
Shafik, S. H., Richards, S. N., Corry, B., Martin, R. E. (2022). Mechanistic Basis for Multidrug Resistance and Collateral Drug Sensitivity Conferred to the Malaria Parasite by Polymorphisms in PfMDR1 and PfCRT. PloS Biol. 20 (5), e3001616. doi: 10.1371/journal.pbio.3001616
Sheiner, L., Soldati-Favre, D. (2008). Protein Trafficking Inside Toxoplasma Gondii. Traffic 9 (5), 636–646. doi: 10.1111/j.1600-0854.2008.00713.x
Sinha, A., Baumgarten, S., Distiller, A., McHugh, E., Chen, P., Singh, M., et al (2021). Functional Characterization of the M6a-Dependent Translational Modulator PfYTH.2 in the Human Malaria Parasite. mBio 12 (2), e00661–e00621. doi: 10.1128/mBio.00661-21
Spork, S., Hiss, J. A., Mandel, K., Sommer, M., Kooij, T. W., Chu, T., et al (2009). An Unusual ERAD-Like Complex is Targeted to the Apicoplast of Plasmodium Falciparum. Eukaryot. Cell 8 (8), 1134–1145. doi: 10.1128/EC.00083-09
Staines, H. M., Moore, C. M., Slavic, K., Krishna, S. (2017). Transmembrane Solute Transport in the Apicomplexan Parasite Plasmodium. Emerg. Top. Life Sci. 1 (6), 553–561. doi: 10.1042/etls20170097
Swearingen, K. E., Lindner, S. E., Shi, L., Shears, M. J., Harupa, A., Hopp, C. S., et al (2016). Interrogating the Plasmodium Sporozoite Surface: Identification of Surface-Exposed Proteins and Demonstration of Glycosylation on CSP and TRAP by Mass Spectrometry-Based Proteomics. PloS Pathog. 12 (4), e1005606. doi: 10.1371/journal.ppat.1005606
Swift, R. P., Rajaram, K., Keutcha, C., Liu, H. B., Kwan, B., Dziedzic, A., et al (2020). The NTP Generating Activity of Pyruvate Kinase II is Critical for Apicoplast Maintenance in Plasmodium Falciparum. eLife 9, e50807. doi: 10.7554/eLife.50807.sa2
Taylor, C. J., McRobert, L., Baker, D. A. (2008). Disruption of a Plasmodium Falciparum Cyclic Nucleotide Phosphodiesterase Gene Causes Aberrant Gametogenesis. Mol. Microbiol. 69 (1), 110–118. doi: 10.1111/j.1365-2958.2008.06267.x
van der Velden, M., Rijpma, S. R., Russel, F. G., Sauerwein, R. W., Koenderink, J. B. (2015). PfMDR2 and PfMDR5 are Dispensable for Plasmodium Falciparum Asexual Parasite Multiplication But Change In Vitro Susceptibility to Anti-Malarial Drugs. Malar. J. 14, 76. doi: 10.1186/s12936-015-0581-y
van Dooren, G. G., Stimmler, L. M., McFadden, G. I. (2006). Metabolic Maps and Functions of the Plasmodium Mitochondrion. FEMS Microbiol. Rev. 30 (4), 596–630. doi: 10.1111/j.1574-6976.2006.00027.x
van Esveld, S. L., Meerstein-Kessel, L., Boshoven, C., Baaij, J. F., Barylyuk, K., Coolen, J. P. M., et al (2021). A Prioritized and Validated Resource of Mitochondrial Proteins in Plasmodium Identifies Unique Biology. mSphere 6 (5), e0061421. doi: 10.1128/mSphere.00614-21
Waller, K. L., McBride, S. M., Kim, K., McDonald, T. V. (2008). Characterization of Two Putative Potassium Channels in Plasmodium Falciparum. Malar. J. 7, 19. doi: 10.1186/1475-2875-7-19
Wang, Q. C., Zheng, Q., Tan, H., Zhang, B., Li, X., Yang, Y., et al (2016). TMCO1 Is an ER Ca2+ Load-Activated Ca2+ Channel. Cell 165 (6), 1454–1466. doi: 10.1016/j.cell.2016.04.051
Weiner, J., Kooij, T. W. (2016). Phylogenetic Profiles of All Membrane Transport Proteins of the Malaria Parasite Highlight New Drug Targets. Microb. Cell 3 (10), 511–521. doi: 10.15698/mic2016.10.534
Wichers, J. S., Mesén-Ramírez, P., Fuchs, G., Yu-Strzelczyk, J., Stäcker, J., von Thien, H., et al (2022). PMRT1, a Plasmodium-Specific Parasite Plasma Membrane Transporter, is Essential for Asexual and Sexual Blood Stage Development. mBio 13 (2), e0062322. doi: 10.1128/mbio.00623-22
Wichers, J. S., van Gelder, C., Fuchs, G., Ruge, J. M., Pietsch, E., Ferreira, J. L., et al (2021). Characterization of Apicomplexan Amino Acid Transporters (ApiATs) in the Malaria Parasite Plasmodium Falciparum. mSphere 6 (6), e0074321. doi: 10.1128/mSphere.00743-21
Wirth, C. C., Bennink, S., Scheuermayer, M., Fischer, R., Pradel, G. (2015). Perforin-Like Protein PPLP4 is Crucial for Mosquito Midgut Infection by Plasmodium Falciparum. Mol. Biochem. Parasitol. 201 (2), 90–99. doi: 10.1016/j.molbiopara.2015.06.005
Wirth, C. C., Glushakova, S., Scheuermayer, M., Repnik, U., Garg, S., Schaack, D., et al (2014). Perforin-Like Protein PPLP2 Permeabilizes the Red Blood Cell Membrane During Egress of Plasmodium Falciparum Gametocytes. Cell Microbiol. 16 (5), 709–733. doi: 10.1111/cmi.12288
Woo, Y. H., Ansari, H., Otto, T. D., Klinger, C. M., Kolisko, M., Michálek, J., et al (2015). Chromerid Genomes Reveal the Evolutionary Path From Photosynthetic Algae to Obligate Intracellular Parasites. eLife 4, e06974. doi: 10.7554/eLife.06974.033
Wunderlich, J., Rohrbach, P., Dalton, J. P. (2012). The Malaria Digestive Vacuole. Front. Biosci. (Schol. Ed.) 4, 1424–1448. doi: 10.2741/s344
Yang, A. S. P., O'Neill, M. T., Jennison, C., Lopaticki, S., Allison, C. C., Armistead, J. S., et al (2017). Cell Traversal Activity is Important for Plasmodium Falciparum Liver Infection in Humanized Mice. Cell Rep. 18 (13), 3105–3116. doi: 10.1016/j.celrep.2017.03.017
Zhang, M., Wang, C., Otto, T. D., Oberstaller, J., Liao, X., Adapa, S. R., et al (2018). Uncovering the Essential Genes of the Human Malaria Parasite Plasmodium Falciparum by Saturation Mutagenesis. Science 360 (6388), eaap7847. doi: 10.1126/science.aap7847
Zipprer, E. M., Neggers, M., Kushwaha, A., Rayavara, K., Desai, S. A. (2014). A Kinetic Fluorescence Assay Reveals Unusual Features of Ca++ Uptake in Plasmodium Falciparum-Infected Erythrocytes. Malar. J. 13, 184. doi: 10.1186/1475-2875-13-184
Zuccala, E. S., Gout, A. M., Dekiwadia, C., Marapana, D. S., Angrisano, F., Turnbull, L., et al (2012). Subcompartmentalisation of Proteins in the Rhoptries Correlates With Ordered Events of Erythrocyte Invasion by the Blood Stage Malaria Parasite. PloS One 7 (9), e46160. doi: 10.1371/journal.pone.0046160
Keywords: Plasmodium falciparum, malaria, drug target, transport pathway, transporters and channels, systems biology, calcium homeostasis, nutrient uptake
Citation: Wunderlich J (2022) Updated List of Transport Proteins in Plasmodium falciparum. Front. Cell. Infect. Microbiol. 12:926541. doi: 10.3389/fcimb.2022.926541
Received: 22 April 2022; Accepted: 23 May 2022;
Published: 24 June 2022.
Edited by:
Tania F. De Koning-Ward, Deakin University, AustraliaReviewed by:
Kevin Brown, University of Oklahoma Health Sciences Center, United StatesBenjamin Liffner, Indiana University, United States
Mikha Gabriela, The University of Melbourne, Australia
Copyright © 2022 Wunderlich. This is an open-access article distributed under the terms of the Creative Commons Attribution License (CC BY). The use, distribution or reproduction in other forums is permitted, provided the original author(s) and the copyright owner(s) are credited and that the original publication in this journal is cited, in accordance with accepted academic practice. No use, distribution or reproduction is permitted which does not comply with these terms.
*Correspondence: Juliane Wunderlich, d3VuZGVybGljaEBtcGlpYi1iZXJsaW4ubXBnLmRl