- 1Department of Parasitology and Tropical Medicine, Graduate School of Medical and Dental Sciences, Tokyo Medical and Dental University, Tokyo, Japan
- 2Department of Molecular Protozoology, Research Institute for Microbial Diseases, Osaka University, Osaka, Japan
The emergence and spread of drug-resistant Plasmodium falciparum have compromised antimalarial efficacy and threatened the global malaria elimination campaign using artemisinin combination therapies. The impacts of amino acid substitutions in antimalarial drug resistance-associated genes on drug susceptibility have been investigated; however, the effects of amplification of those genes remain unexplored due to the lack of robust genetic approaches. Here, we generated transgenic P. falciparum parasites with an additional copy of a drug resistance-associated gene using the highly efficient CRISPR/Cas9 system and investigated their drug response. Insertion of a drug resistance-associated gene expression cassette in the genome resulted in a roughly twofold increase of mRNA levels of the target gene mdr1, which encodes multidrug resistance protein 1. The gene duplication event contributed to resistance to mefloquine, lumefantrine, and dihydroartemisinin, while the duplication of a genomic region encoding plasmepsin 2 and plasmepsin 3 did not affect resistance to antimalarial drugs, including piperaquine. We further demonstrated that mdr1 mRNA expression levels are strongly associated with mefloquine resistance in several field-derived P. falciparum lines with various genetic backgrounds. This study provides compelling evidence that mdr1 could serve as a molecular marker for the surveillance of mefloquine-resistant parasites. Long DNA integration into parasite genomes using the CRISPR/Cas9 system provides a useful tool for the evaluation of the effect of copy number variation on drug response.
Introduction
The WHO estimated that there were 241 million cases and 627,000 deaths due to malaria in 2020 (World Malaria Report, 2021). The emergence of drug-resistant parasites makes malaria control difficult, as evidenced by the fact that chloroquine-resistant Plasmodium falciparum is now spread globally (Ecker et al., 2012). Currently, the WHO recommends artemisinin-based combination therapies (ACTs), in which artemisinin is used as a first-line drug along with partner drugs such as mefloquine, lumefantrine, piperaquine, and amodiaquine. ACTs have greatly contributed to the decrease in malarial deaths (Bhatt et al., 2015; World Malaria Report, 2021). However, recent epidemiological studies indicate that ACT treatment failures are increasing worldwide, particularly in the Greater Mekong Subregion (GMS) (Ashley et al., 2014; Amato et al., 2017; Witkowski et al., 2017; Ikeda et al., 2018). One possible reason for ACT failures is the emergence of strains with mutations in kelch13, which are associated with artemisinin resistance (Ariey et al., 2014; van der Pluijm et al., 2019; Balikagala et al., 2021). In addition, ACT failure may result from resistance acquired from partner drugs (Leang et al., 2013; Ashley et al., 2014; Nsanzabana, 2019). Triple ACTs (TACTs), which add another partner drug with a conventional ACT, were introduced and have increased antimalarial efficacy (Dini et al., 2018; Okell et al., 2018; van der Pluijm et al., 2020). The selection of effective partner drugs is important for successful treatment by ACTs; the identification of suitable molecular markers associated with partner drug resistance is required for optimal ACT design and implementation.
Recent epidemiological studies suggested that partner drug resistance is due to amino acid substitutions or copy number variations of several genes. Mefloquine resistance is considered to be conferred by an increase in the copy number of the gene that encodes multidrug resistance protein 1 (PF3D7_0523000, mdr1), a protein homologous to the human drug efflux pump (Price et al., 2004). Piperaquine resistance is reported to be correlated with increased copy number of plasmepsin 2 (PF3D7_148000, pm2) and plasmepsin 3 (PF3D7_148100, pm3), based on genome-wide association studies (Amato et al., 2017; Witkowski et al., 2017). The relationship between specific amino acid substitutions and drug resistance has been analyzed by allelic replacement using plasmid integration via a single crossover, as well as recently developed genome editing methods such as zinc-finger nuclease or CRISPR/Cas9 (Triglia et al., 1998; Sidhu et al., 2005; Veiga et al., 2016; Dhingra et al., 2017; Ross et al., 2018). In contrast, the association between gene copy number variations and drug sensitivity has been not well examined due to the technical limitations of genetic methods (Sidhu et al., 2006; Loesbanluechai et al., 2019).
We recently utilized the CRISPR/Cas9 genome editing system to integrate a large gene expression cassette (Nishi et al., 2021). Here, we applied this technology to generate transgenic parasites in which an additional copy of mdr1, pm2, or pm3 was integrated to examine the effect of their amplification on antimalarial drug sensitivities. Our results demonstrated that increased mRNA expression of mdr1 reduced the sensitivity to mefloquine and slightly to lumefantrine and dihydroartemisinin. In contrast, duplication of the genomic region containing pm2 and pm3 did not change the sensitivity to known partner drugs, including piperaquine. We further demonstrated that mdr1 expression levels are associated with mefloquine resistance using field-derived strains, and we illustrated that mdr1 expression and/or copy number could be a useful marker for the surveillance of mefloquine-resistant parasites. This is the first report of the generation of transgenic parasites with an additional copy of a drug resistance-associated gene. Our robust genome editing protocol to introduce gene amplification could be an effective tool to evaluate the association between copy number variation and drug resistance.
Materials and Method
Ethical Clearance
Ethical approval for the use of human red blood cells (RBCs) and plasma from the Japanese Red Cross Tokyo Blood Center was obtained from the Medical Research Ethical Committee of the Tokyo Medical and Dental University.
Parasite Strains and Culture
All laboratory strains of P. falciparum were obtained from the MR4 repository (www.beiresources.org), namely, 3D7 (MRA-102), Dd2 (MRA-150), IPC_5188 (MRA-1239), IPC_5202 (MRA-1240), 7G8 (MRA-125), and FCR3 (MRA-736). The pfcas9 parasite line was used for the derivation of the transgenic parasites in this study. This line was generated from the P. falciparum strain 3D7 in our previous study (Nishi et al., 2021) and possesses a cas9 expression cassette integrated within the knob-associated histidine-rich protein locus (PF3D7_0202000, kahrp). All parasite strains were cultivated with human type O RBCs (obtained from the Japanese Red Cross Tokyo Blood Center) at 2% hematocrit in a complete medium, which consists of RPMI-1640 medium containing 2.5% human serum (obtained from the Japanese Red Cross Tokyo Blood Center), 2.5% AlbuMAX II (Life Technologies, Carlsbad, CA, USA), 25 mM of HEPES, 0.225% sodium bicarbonate, and 0.38 mM of hypoxanthine supplemented with 10 μg/ml gentamicin. Parasite cultures were incubated under low-oxygen conditions (90% N2, 5% CO2, and 5% O2) as described (Nishi et al., 2021).
Construction of an SgRNA-Expressing Plasmid and Preparation of Donor Template DNA
SgRNA-expressing plasmids and donor template DNA were generated as described below and used for the derivation of transgenic parasites with an additional copy of mdr1, pm2, pm3, or both pm2 and pm3, respectively named pfcas9-mdr1, pfcas9-pm2, pfcas9-pm3, and pfcas9-pm2/3. The gRNA was designed as described (Nishi et al., 2021). Briefly, a 19-bp gRNA target sequence, which is predicted to have no off-target candidates, was designed using the CHOPCHOP program (https://chopchop.cbu.uib.no/). To generate the plasmid expressing sgRNA, an oligonucleotide pair was annealed and cloned into the pf-gRNA plasmid as described. The plasmid expressing sgRNA was modified by a csp-sgRNA_1F and csp-sgRNA_1R primer set to integrate within the circumsporozoite protein locus (csp, PF3D7_0304600) and named psgRNA-csp. To integrate the mdr1 promoter region within the csp locus, the plasmid expressing sgRNA to recognize csp-HR1 was generated with the csp-gRNA_2F and csp-sgRNA_2R primer set, and the resulting plasmid was named psgRNA-cspHR1. To integrate the pm3 region within the csp locus of pfcas9-pm2, the plasmid expressing sgRNA to recognize csp-HR2 was generated with the csp-gRNA_3F and csp-sgRNA_3R primer set, and the resulting plasmid was named psgRNA-cspHR2. The donor DNA plasmid pDonor_mdr1 and PCR-fragment were used for the generation of the pfcas9-mdr1 parasite. First, the mdr1 fragment including ORF and 3′ untranslated regions (UTRs) was inserted using an In-Fusion HD cloning kit into the EcoRI and HindIII sites of the donor template DNA plasmid, to replace the GFP coding sequence. Next, to add the 3-kbp promoter of mdr1 to the csp locus, the donor DNA fragment was used in which the homologous region of the csp locus and mdr1 region containing the promoter and 700-bp ORF was fused by overlap PCR. To prevent re-cleavage by the Cas9–sgRNA complex, the donor DNA lacked 20 bp of the csp sequence in the homologous region. The donor DNA plasmids pDonor_pm2 and pDonor_pm3 were used for the generation of pfcas9-pm2 or pfcas9-pm3 parasites, respectively. The donor DNA plasmids were modified by integrating the pm2 fragment including promoter and 3′-UTR and amplified using the primer set pm2_HindIII-F and pm2_EcoRI-R, or the pm3 fragment amplified using the primer set pm3_HindIII-F and pm3_EcoRI-R as described above. The donor DNA to generate the pfcas9-pm2/3 parasite was a fragment that fused the 3′-UTR of pm2 with the pm3 expression cassette by overlap PCR. All donor DNA plasmids were linearized for transfection. The sequences of oligonucleotides used for sgRNA-expressing plasmid or donor DNA constructions are listed in Supplementary Table 1.
Generation of Transgenic Parasites
Transfection of P. falciparum by CRISPR/Cas9 was performed as described (Mohring et al., 2019; Morita et al., 2021). Tightly synchronized mature schizonts were transfected using the FP-158 program on a Nucleofector 4D device (LONZA, Basel, Switzerland). First, for transfections to integrate the target gene into the csp locus, the psgRNA-csp and linear donor DNA were used. Next, the psgRNA-cspHR1 and linear donor DNA were used to add the mdr1 promoter region. To generate the pfcas9-pm2/3 line, the pfcas9-pm2 line was transfected with linear donor DNA and the psgRNA-cspHR2. Each linear repair DNA template (25 µg) plus plasmid containing the sgRNA (25 µg) was dissolved in 100 µl of P3 Primary Cell 4D-Nucleofector™ X Kit (LONZA) and were mixed with purified schizonts (1 × 108). Pyrimethamine-mediated (25 ng/ml) selection of the transgenic parasites was initiated 72 h after transfection and continued for 10 days. The emergence of transgenic parasites was monitored using a diagnostic PCR assay, followed by 1 µM of 5-fluorocytosine treatment for 5 days to eliminate plasmid-containing parasites. Parasite clones were further selected by limiting dilution, in which by diagnostics the target gene was integrated. The diagnostic PCR products were sequenced by standard Sanger sequencing to confirm the absence of undesired mutations in the target gene coding regions. To generate pfcas9-mdr1 and pfcas9-pm2-3, synchronized mature schizonts of the plasmid-free primary transgenic parasite clone were used for the secondary transfection. Removal of the sgRNA plasmid from the primary transgenic parasites was confirmed by the restoration of pyrimethamine susceptibility. The sequences of primers used for diagnostic PCR are listed in Supplementary Table 1.
Transcription of Target Genes
To quantify mdr1 transcript levels, total RNA was purified from parasites 6 to 10 h post-infection (hpi), when the expression of mdr1 reached the maximum level (https://plasmodb.org/plasmo). For assay of pm2 and pm3 transcript levels, total RNAs were purified from parasites 26 to 30 hpi. Total RNA was isolated using TRIzol™ (Thermo Fisher Scientific, Waltham, MA, USA) and purified from three biological independent samples for each parasite strain. The cDNAs were synthesized from 1 µg of each purified total RNA using a PrimeScript RT reagent kit with a gDNA Eraser according to the manufacturer’s instructions (TAKARA Bio, Kusatsu, Japan). Quantitative reverse transcription PCR assays were performed in triplicate using cDNA as a template with Power SYBR® Green Master Mix (Thermo Fisher Scientific) and a StepOne Plus device (Thermo Fisher Scientific). Target gene expression levels were calculated based on the CT values using the ddCt method. All real-time PCRs were performed using the seryl tRNA synthetase gene (PF3D7_0717700) as an internal control and in at least two independent experiments. Primers are listed in Supplementary Table 1.
Southern Blotting
Southern hybridization analysis was performed as described with some modifications (Shinzawa et al., 2020; Nishi et al., 2021). Briefly, to detect the mdr1 locus, genomic DNA was extracted from blood-stage parasites (2 µg) and digested with the restriction enzyme ScaI. The primers mdr1-probe-F and mdr1-probe-R were used to generate the DNA probe. To detect the pm2 locus, parasite genomic DNA was digested with the restriction enzymes ScaI and SalI. For detecting the pm3 locus, parasite genomic DNA was digested with the restriction enzymes ScaI, SalI, and BamHI. The fragments were separated on a 0.8% agarose gel and transferred to nylon membranes. DNA probes to characterize the pm2 and pm3 loci were generated with the following respective primer pairs: pm2-probe-F and pm2-probe-R, and pm3-probe-F and pm3-probe-R. The positive control was the plasmid used to generate the transgenic parasites in this study. The PCR products were labeled with digoxigenin (DIG) and used as hybridization probes. Chemiluminescence signals were detected using ChemiDoc MP (Bio-Rad, Hercules, CA, USA).
Fitness Assay
Fitness assays were performed as described with some modifications (Nishi et al., 2021). Tightly sorbitol-synchronized ring-stage parasites were inoculated at 0.1% parasitemia and 2% hematocrit in a drug-free complete medium. The culture medium was changed, and the parasitemia was monitored by Giemsa stain daily for 4 days.
Preparation of Antimalarial Drug Stock Solutions
Mefloquine, piperaquine, lumefantrine, and dihydroartemisinin were purchased from Tokyo Chemical Industry Co., Ltd. Tokyo, Japan. Stock solutions of 50 mM of mefloquine, 2 mM of lumefantrine, and 100 mM of dihydroartemisinin were prepared in dimethyl sulfoxide (Wako, Tokyo, Japan) and 1 mM of piperaquine in 0.5% lactic acid (Wako), with ultrasonic treatment and warming at 60°C to dissolve. The stock solutions were aliquoted and stored at −20°C until use.
Measurement of the IC50 Values of Antimalarial Drugs
Parasites were synchronized by the sorbitol-based method, and 48 h later, the parasitemia was adjusted to 0.5% in blood at 2% hematocrit. The final drug concentrations tested ranged from 0 to 800 nM for mefloquine, 0 to 100 nM for piperaquine, 0 to 1,600 nM for lumefantrine, and 0 to 80 nM for dihydroartemisinin. Parasite culture (50 µl) and diluted drug medium (50 µl) were dispensed at a final parasitemia of 0.25% in quadruplicate to wells of a flat-bottom 96-well plate. Piperaquine and lumefantrine were incubated for 96 h, mefloquine for 144 h due to its longer half-life, and dihydroartemisinin for 48 h due to its rapid loss of drug activity. Parasite growth in each well was assessed using SYBR Green I (Invitrogen, Waltham, MA, USA) with lysis buffer (20 mM of Tris-HCl, pH 7.5, 5 mM EDTA, 0.008% saponin, and 0.08% Triton X-100). The plates were shaken until all RBCs were lysed and were then placed at room temperature in the dark for 30 min. SYBR Green I fluorescence was measured using a BioTek Synergy LX microplate reader (Agilent Technologies, Santa Clara, CA, USA) with excitation and emission wavelengths at 485 and 535 nm, respectively. The IC50 values were calculated based on the detected fluorescence intensities using GraphPad Prism 9 software. Briefly, their survival rates were plotted against the logarithm of drug concentration, and the curve fittings were performed by non-linear regression to yield the drug concentration (IC50 values) that produced a 50% survival rate.
Piperaquine Survival Assay
In vitro piperaquine survival assays (PSAs) were performed as described (Duru et al., 2015). Briefly, early ring-stage parasites at 0 to 3 hpi were prepared using 70% Percoll gradients (GE Healthcare, Chicago, IL, USA) and 5% sorbitol treatment. The resulting tightly synchronized parasites with 0.5% parasitemia and 2% hematocrit were cultured in 200 nM of piperaquine or 0.5% lactic acid medium for 48 h in 48-well plates. The parasites were washed with an incomplete medium and further cultured for 24 h without drug treatment. Parasitemias were determined via SYBR Green I fluorescence, as described above for the in vitro IC50 drug susceptibility assays. Good concordance was observed between cell count values by a SYBR Green assay versus microscopy-based counting of Giemsa-stained thin blood smears. Assays were repeated in duplicate at least twice.
Copy Number Variation Analysis of mdr1
To quantify mdr1 copy numbers, total genomic DNAs were purified from the transgenic parasites as described above. Real-time genomic PCR assays were performed in triplicate with Power SYBR® Green Master Mix (Thermo Fisher Scientific) and a StepOne Plus device (Thermo Fisher Scientific) using genomic DNA as a template. To calculate the mdr1 copy number, PF3D7_0523800 was used as an internal control. The primer sets for mdr1 and PF3D7_0523800 were validated using pfcas9 and pfcasp-mdr1 genomic DNA. For laboratory strains, the reactions of real-time genomic PCR were performed using 0.1 ng of genomic DNA. Quantification of mdr1 copy numbers was determined by ddCt methods. All real-time PCRs were performed in two independent experiments. Primers are listed in Supplementary Table 1.
Statistical Analysis
All experiments were statistically analyzed from at least three biological replicates using an unpaired Student’s t-test within GraphPad Prism 9.0 (GraphPad Software Inc.).
Results
Generation of Transgenic Plasmodium falciparum Parasites With an Additional Copy of Drug Resistance-Associated Genes
To investigate a possible direct causal association of drug resistance with copy number amplification of mdr1, pm2, and pm3, we generated transgenic P. falciparum parasites with an additional copy of each gene by CRISPR/Cas9-mediated knocking-in of long genomic regions. The introduced expression cassettes contained the target gene coding regions together with the promoter and 3′-UTRs, predicted by amplification-free RNA-seq data to mimic its original expression pattern (Chappell et al., 2020). The above resistance-associated genes were introduced into the circumsporozoite protein (csp) locus of a Streptococcus pyogenes Cas9-expressing 3D7 parental strain (pfcas9; parental). The csp locus is considered to be dispensable for asexual parasite proliferation, with no known knock-out phenotype (Nishi et al., 2021). To knock-in mdr1, the fragment corresponding to the coding region and 3′-UTR was introduced into the csp locus, followed by the insertion of an upstream 3-kb promoter region (pfcas9-mdr1; Figure 1A). For pm2 and pm3, transgenic parasites were generated with an additional copy of pm2 or pm3 (pfcas9-pm2 or pfcas9-pm3; Figure 1A). To investigate a possible synergistic effect of pm2 and pm3, an expression cassette of pm3 was introduced into the genome of pfcas9-pm2 downstream of the inserted pm2, to generate transgenic parasites containing additional copies of both pm2 and pm3 (pfcas9-pm2/3; Figure 1A). Genotyping PCR and Southern hybridization analyses showed the correct integration of the additional gene copy at the csp locus in the respective transgenic parasites (Figures 1B–E). There were no undesired mutations in the coding regions of the inserted genes (data not shown), supporting that the additional copies were correctly introduced.
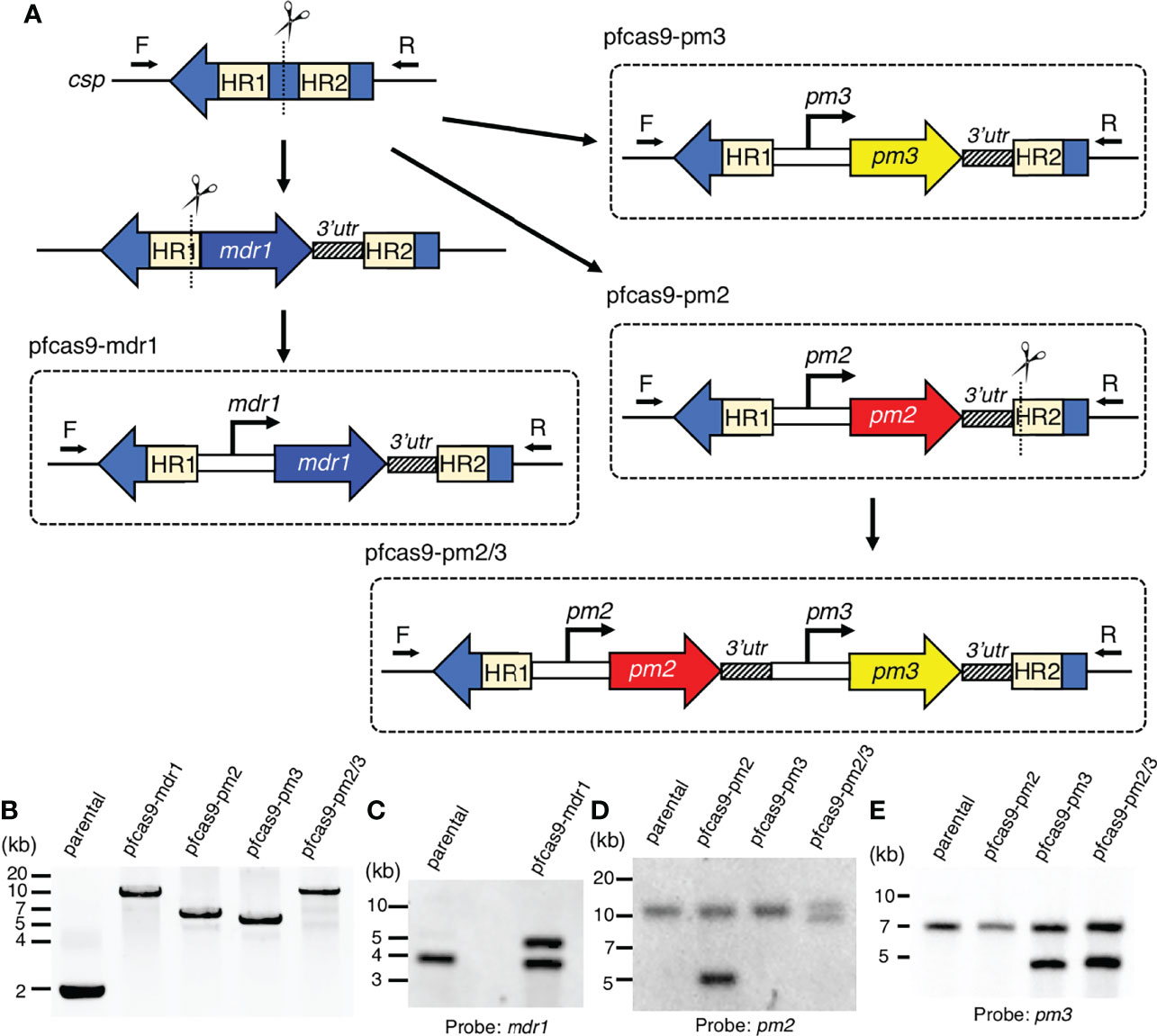
Figure 1 Generation of transgenic parasites with an additional copy of drug resistance-associated genes by CRISPR/Cas9-based genome editing. (A) Schematic representation of a strategy to derive transgenic parasites with an additional copy of drug resistance-associated genes. The pfcas9 parasite line was transfected with an sgRNA expression vector and a linear form of the donor DNA template. The drug resistance-associated gene expression cassette was integrated into the csp locus via homology-directed repair. (B) Genotyping PCR was performed using csp-check-F and csp-check-R primers. For each transgenic parasite, the integration of an additional copy into the csp locus was confirmed. (C–E) Southern hybridization analyses of transgenic parasites were carried out using partial DNA fragments of mdr1 (C), pm2 (D), and pm3 (E) open reading frames as the DNA probes. A double signal indicating gene amplification was detected in each transgenic parasite.
Increase in mRNA Expression of Target Genes Following Copy Number Amplification
To evaluate the relationship between copy number amplification and mRNA expression, the transcript levels of each gene were compared between parental and transgenic parasites. The mdr1 transcript levels were examined by quantitative reverse transcription PCR at the ring stage (6–10 hpi), and those of pm2 and pm3 were examined at the trophozoite stage (26–30 hpi), at their highest transcription timing according to the transcriptomics data (Toenhake et al., 2018; Wichers et al., 2019; Chappell et al., 2020). The results demonstrated that the mdr1 transcript level in ring-stage parasites of the pfcas9-mdr1 line was about 2-fold higher than that of parental parasites (Figure 2A and Supplementary Table 2). The insertion of each pm2 or pm3 expression cassette, or both pm2 and pm3 increased their target gene mRNA levels by 1.5- to 2-fold compared to parental parasites (Figures 2B, C and Supplementary Table 2). All transgenic strains appeared to be normal with respect to intra-erythrocytic development (Figure 2D). The results were consistent for two independent clones of each transgenic parasite. In summary, these results demonstrated that an additional copy of a drug resistance-associated gene within the csp locus increased the respective mRNA expression about twofold without any transcriptional suppression, and the increased expression did not affect the asexual stage development.
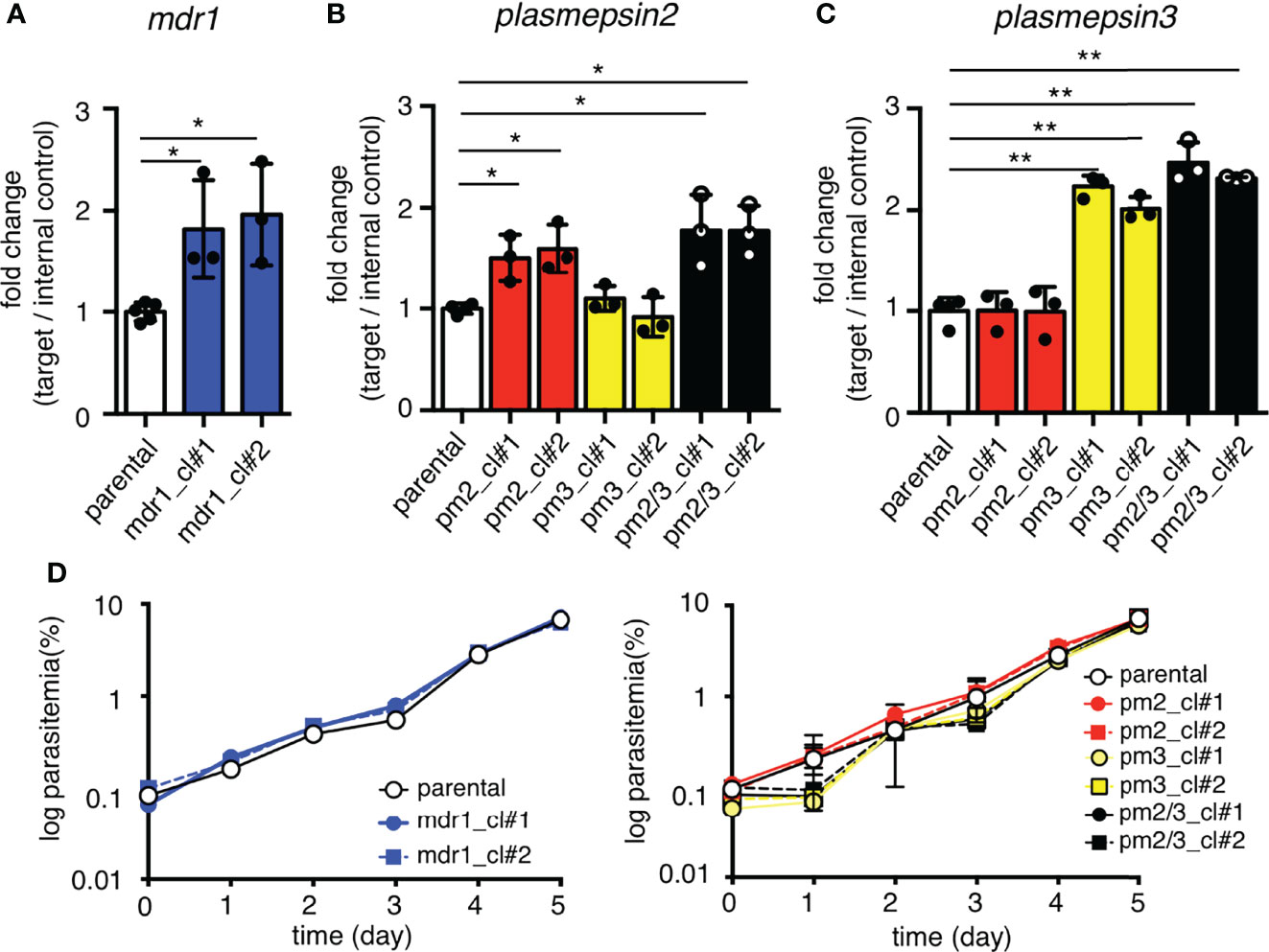
Figure 2 Increased mRNA expression by an additional copy of drug resistance-associated genes. (A) The mRNA expression of mdr1 was measured by quantitative reverse transcription PCR. The two clones of transgenic parasites with integrated mdr1 cassette (blue) had approximately 2-fold increased mRNA expression compared to the parental strain (white). pfcas9-mdr1 clones are labeled mdr1_cl#1 and mdr1_cl#2. (B, C) The mRNA expressions of pm2 or pm3 were measured by quantitative reverse transcription PCR. The pm2, pm3, or both-integrated parasites (red, yellow, and black) had approximately 2-fold increased mRNA expression of integrated genes compared to the parental strain. pfcas9-pm2, pfcas9-pm3, and pfcas9-pm2/3 clones are labeled pm2_cl#1 and pm2_cl#2, pm3_cl#1 and pm3_cl#2, and pm2/3_cl#1 and pm2/3_cl#2, respectively. (D) Growth of the transgenic parasites during asexual developmental cycle was comparable to the parental strain (left, mdr1; right, pm2, pm3, or both). N, n = 3–5 (Supplementary Table 2). Significance was determined using Student’s t-tests. *p < 0.01; **p < 0.001.
Drug Response of the Transgenic Parasites With Amplification of Drug Resistance-Associated Genes
To examine the effects of an additional copy of mdr1 on drug susceptibility, we measured the 50% inhibitory concentration (IC50) values of pfcas9-mdr1 for the common ACT partner drugs mefloquine, lumefantrine, and piperaquine, and an artemisinin derivative, dihydroartemisinin (Table 1 and Figure 3). The IC50 values of mefloquine for two clones of pfcas9-mdr1 were 38.7 and 37.8 nM, which were ~1.8-fold higher than those of parental parasites (IC50 value; 21.0 nM; Figure 3A). The pfcas9-mdr1 line survived with no morphological changes, even at a mefloquine concentration that killed the parental line (Supplementary Figure 1). For lumefantrine, the IC50 values of the two clones were 30.3 and 30.1 nM. These values were ~1.4-fold increase compared to the parental line (IC50; 21.2 nM; Figure 3B). Although the increased expression of mdr1 mediated by an additional copy insertion confers resistance to mefloquine and lumefantrine, parental and transgenic parasites were not significantly different for piperaquine sensitivity (Figure 3C). In addition, the amplification of mdr1 slightly increased the IC50 values for dihydroartemisinin (Figure 3D).
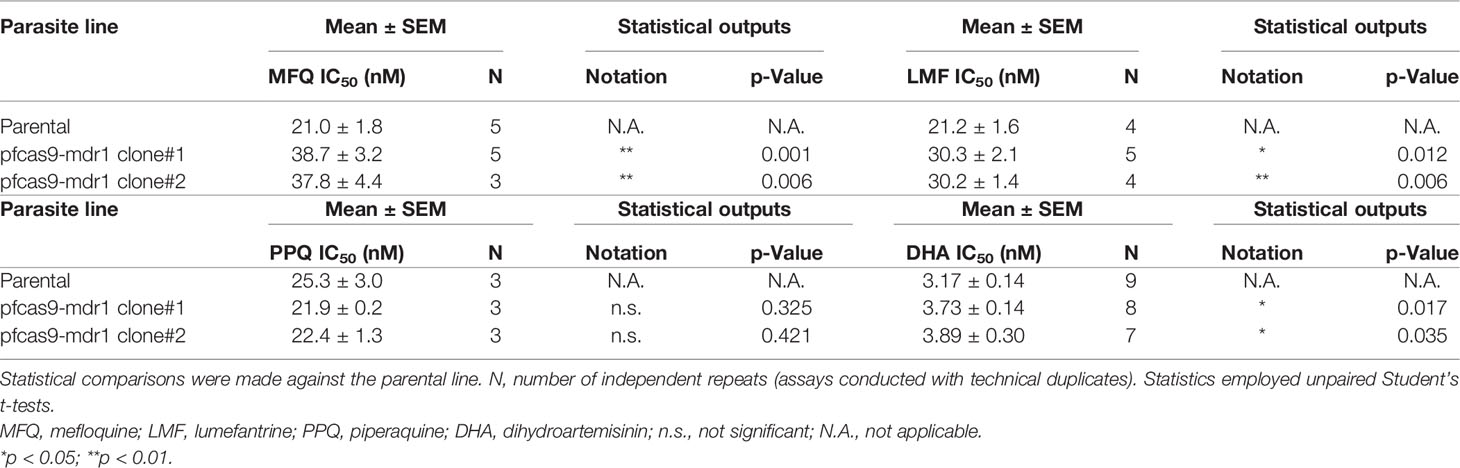
Table 1 IC50 values of mefloquine, piperaquine, lumefantrine, and dihydroartemisinin with pfcas9-mdr1.
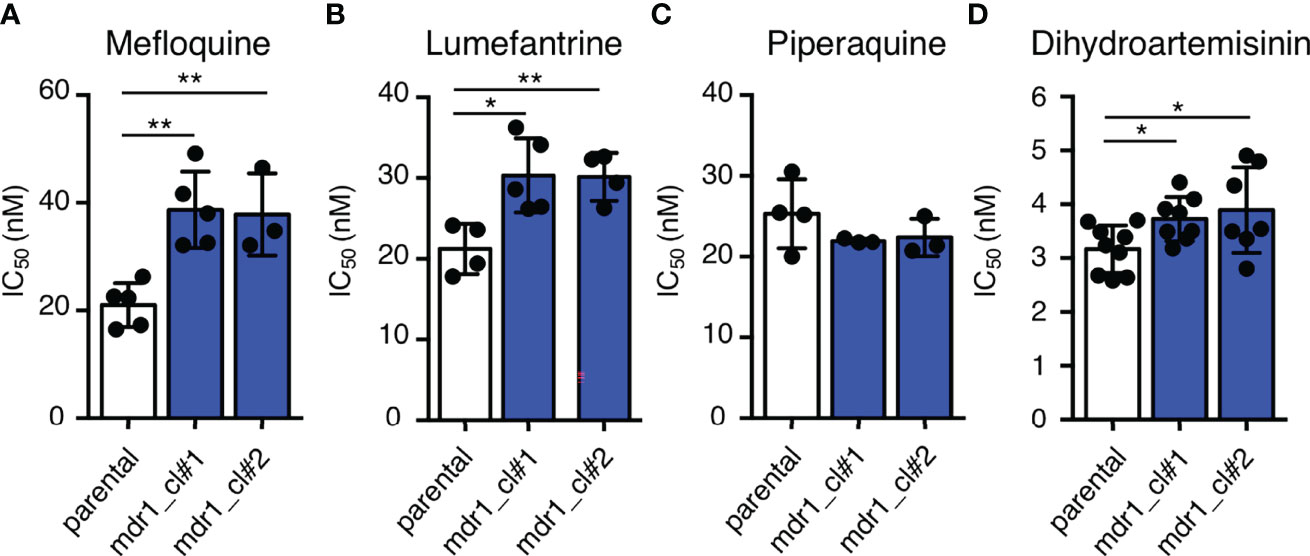
Figure 3 An additional copy of mdr1 modified susceptibility to multiple antimalarial drugs. (A–D) The IC50 values were determined for the antimalarial drugs mefloquine, lumefantrine, piperaquine, and dihydroartemisinin with pfcas9-mdr1 parasites (mdr1_cl#1 and mdr1_cl#2) and the pfcas9 parental strain (parental). IC50 values of mefloquine (A), lumefantrine (B), and dihydroartemisinin (D) with transgenic parasites were higher than those of the parental strain, but the IC50 values of piperaquine (C) were not significantly different. Mean ± SEM IC50 values (Table 1) were calculated three to nine times. Statistical evaluations comparing the transgenic parasites and parental strain were performed using Student’s t-tests. *p < 0.05; **p < 0.01.
We then analyzed the effects of increased copy numbers of pm2 and pm3 on ACT-component drugs by measuring IC50 (Table 2). Insertion of pm2 or pm3 had no effect on the resistance of ACT partner drugs, namely, mefloquine, lumefantrine, and piperaquine, nor on an artemisinin derivative, dihydroartemisinin (Figures 4A–D). These results were consistent with a report demonstrating that episomal overexpression of pm2 or pm3 did not confer drug resistance (Loesbanluechai et al., 2019). Furthermore, no significant changes were observed in drug resistance by insertion of both pm2 and pm3 (Figures 4A–D). The amplification of the pm2 and pm3 region has been reported to be associated with reduced susceptibility of piperaquine using a PSA (Witkowski et al., 2017). Therefore, we performed a PSA using pfcas9-pm2/3 transgenic parasites and found that an additional copy of both pm2 and pm3 had no impact on drug response compared to parental parasites (Figures 4E, F and Table 2). These results indicate that, at least in the genetic background of the P. falciparum reference strain 3D7, a 1.5- to 2-fold increase in pm2 and pm3 expression levels is not sufficient to confer detectable drug resistance to piperaquine and other drugs including artemisinin.
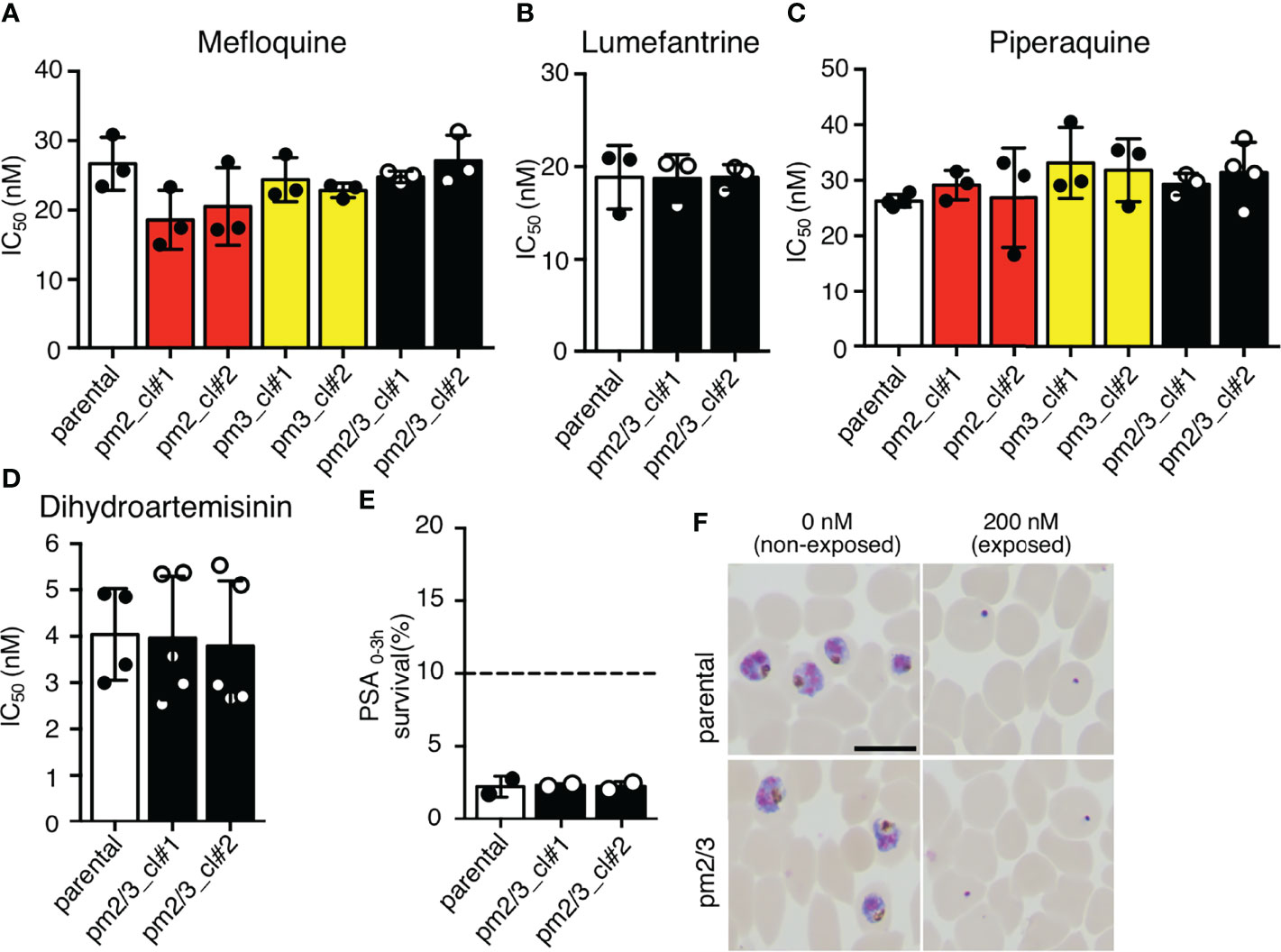
Figure 4 The effect of an additional copy of pm2 and pm3 on drug sensitivity. (A–D) IC50 values were determined for the antimalarial drugs mefloquine, lumefantrine, piperaquine, and dihydroartemisinin with transgenic parasites harboring an additional copy of pm2 and/or pm3 (pm2_cl#1, pm2_cl#2, pm3_cl#1, pm3_cl#2, pm2/3_cl#1, and pm2/3_cl#2) versus the pfcas9 parental strain (parental). None of the IC50 values for these transgenic parasites were significant versus the parental strain. IC50 values (Table 2) were calculated three to five times. (E, F) In vitro piperaquine survival assays (PSA0–3 h) were performed for the pfcas9-pm2/3 and the parental strain. (E) Survival rates were determined as a percentage of the parasitemia obtained with treated versus drug-off and were not significant comparing transgenic parasites and parental. The dashed line represents the 10% survival rate cutoff that distinguishes piperaquine-resistant (≥10%) from piperaquine-sensitive (<10%) parasites in PSAs. (F) Thin blood smears stained with Giemsa reagent were prepared 24 h post-drug withdrawal. pfcas9-pm2/3 was killed by piperaquine treatment, as was the parental strain. N, n = 2 (Table 2). A scale bar indicates 10 µm. Significance was determined using Student’s t-tests.
mdr1 Transcript Levels Correlate With Mefloquine Resistance, But Not Lumefantrine and Dihydroartemisinin
Copy number variation analysis of patient-derived P. falciparum DNA has shown that mdr1 is associated with antimalarial drug susceptibility (Price et al., 2004; Price et al., 2006; Phompradit et al., 2014; Phyo et al., 2016; Montenegro et al., 2021). We demonstrated that an increase in mdr1 mRNA expression reduced the susceptibility for mefloquine, lumefantrine, and dihydroartemisinin (Figure 3). We next examined whether mdr1 transcript levels directly correlate with drug resistance using multiple laboratory strains. We used 3D7 and 7G8 as mdr1 single-copy reference strains and Dd2 and FCR3 lines as mdr1 multiple copy reference strains (Price et al., 2004; Veiga et al., 2012). The Cambodian IPC_5188 and IPC_5202 isolates, which respectively show susceptible and resistance phenotypes in a ring-survival assay with artemisinin, were used as strains in which the mdr1 copy number was unknown (Ariey et al., 2014). First, a real-time genomic PCR assay was developed to determine mdr1 genome copy number by the ddCt method, with PF3D7_0523800 as an internal control gene, which locates on the same chromosome as mdr1 but outside of the genomic multiplication region of known drug-resistant strains. The results showed that when 3D7, which has one copy of mdr1, was used as a control, 7G8 had a single copy of mdr1, and Dd2 and FCR3 had multiple copies as reported (Supplementary Table 3). The Cambodian isolates IPC_5188 and IPC_5202 were found to contain single copies and multiple copies, respectively (Figure 5A and Supplementary Table 3). The subsequent quantitative reverse transcription PCR assays revealed that the strains with multi-copy mdr1 showed higher expression levels than lines with a single copy of mdr1 (Figures 5B–D and Supplementary Table 3). We then examined whether there were significant correlations between the mdr1 expression levels of these laboratory strains and their susceptibility to mefloquine, lumefantrine, or dihydroartemisinin (Figures 5B–D). The results indicated that mefloquine resistance is strongly correlated with mdr1 expression levels (R2 = 0.91), while factors other than mdr1 expression could have larger impacts on resistance to lumefantrine and dihydroartemisinin (Figures 5C, D).
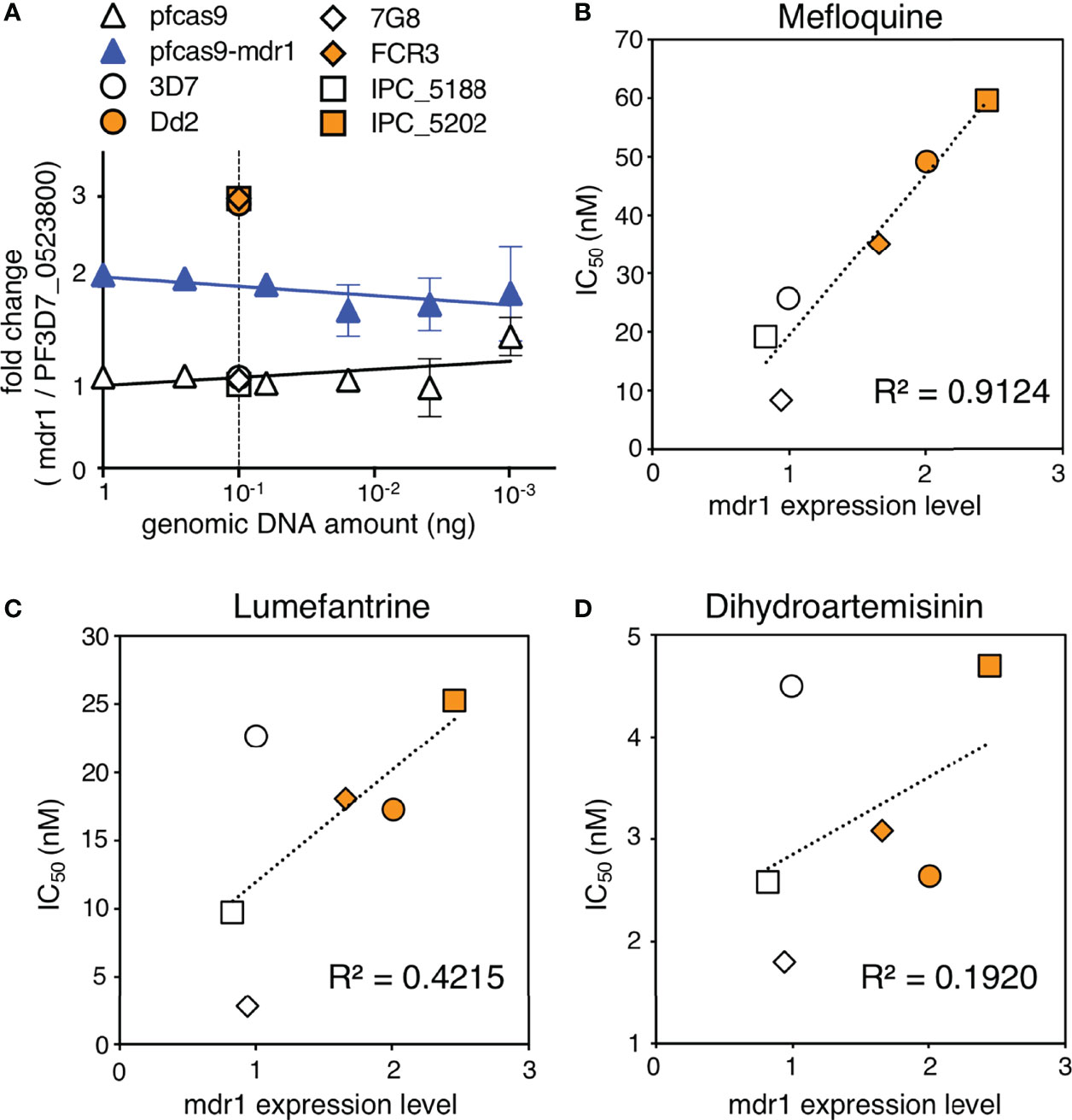
Figure 5 Association of mdr1 copy number and antimalarial drug sensitivity in laboratory strains. (A) The mdr1 copy number of laboratory strains and transgenic parasites were detected by real-time genomic PCR. Dd2 and FCR3 were used as mdr1 multicopy reference parasites, and 3D7 and 7G8 were used as mdr1 single-copy reference parasites. Cambodian field isolates IPC_5188 and IPC_5202 were found to have single and multiple copies, respectively. N, n = 3–4 (Supplementary Table 3). (B–D) The laboratory strains were assayed for mdr1 expression levels and IC50 values for mefloquine (B), lumefantrine (C), and dihydroartemisinin (D). N, n = 3–9 (Supplementary Table 3). There was a linear correlation between the mdr1 expression levels and mefloquine resistance (R2 = 0.91). The association between mdr1 expression levels and lumefantrine or dihydroartemisinin resistance was unclear (R2 = 0.42, 0.19, respectively). Data are shown as mean ± SD. The coefficient of determination, R2, was defined using linear regression modeling. The strains with multiple copied mdr1 are indicated with orange-filled markers.
Discussion
Recent studies show that the evolution of P. falciparum drug resistance has rapidly compromised the clinical efficacy of ACTs (Ashley et al., 2014; Amato et al., 2017; Witkowski et al., 2017). The design of effective ACT strategies requires an understanding of the local spread of drug-resistant parasites, especially regional resistance to partner drugs. In this report, we determined if duplications of mdr1, pm2, or pm3, which were observed in field-derived drug-resistant parasites, could reduce the susceptibility against ACT partner drugs. By applying the CRISPR/Cas9 genome editing system, we successfully generated transgenic parasites containing additional copies of mdr1, pm2, pm3, or a combination of pm2 and pm3; these gene duplications resulted in approximately twofold increases in target gene transcript levels. We proved by using transgenic parasites that an increased mRNA expression level of mdr1 directly decreased sensitivity to mefloquine, lumefantrine, and dihydroartemisinin (Figure 3). In various genetic backgrounds, the high correlation between the expression level of mdr1 and the IC50 values of mefloquine indicated that the mdr1 copy number was the most important genetic factor contributing to mefloquine resistance. However, mdr1 mRNA levels do not correlate with copy number, and this indicates the role of additional aspects that determine mdr1 expression levels, such as transcription-related factors. Expression levels of mdr1 were not tightly correlated with sensitivity to lumefantrine and dihydroartemisinin, although insertion of an additional copy of mdr1 resulted in decreased sensitivities. These results suggest that the sensitivity to those drugs is affected by other factors in the genetic background rather than mdr1 expression. Our findings are consistent with the epidemiology-based copy number variation analyses of mdr1, which reported that mefloquine resistance was strongly associated with mdr1 copy number (Price et al., 2004; Veiga et al., 2012; Phompradit et al., 2014). The mRNA expression level of mdr1 should be determined to judge mefloquine-resistant parasites; however, measuring transcript levels is difficult using patient blood due to RNA instability. Therefore, the mdr1 copy number remains an important marker for surveillance of drug-resistant parasites, and epidemiological investigations should continue.
We also clearly demonstrated that insertion of an additional pm2 or pm3 copy into the P. falciparum 3D7 strain had no impact on its antimalarial drug sensitivity. These observations agree with the determination that increased expression of pm2 or pm3 via episomal plasmids had no effect on piperaquine susceptibility (Loesbanluechai et al., 2019). The correlation between piperaquine resistance and pm2/3 amplification has been reported only in Southeast Asia (Huang et al., 2020). Therefore, increasing pm2/3 expression in the genetic background of strains of Southeast Asian origin may confer piperaquine resistance. In addition, mutations in the chloroquine resistance transporter that differ from mutations conferring chloroquine resistance have been shown to reduce piperaquine sensitivity (Duru et al., 2015; Agrawal et al., 2017; Dhingra et al., 2017; Ross et al., 2018). Also, many parasites with a single copy of pm2 and pm3 have been isolated, which have failed dihydroartemisinin/piperaquine combination treatment in epidemiological studies (Huang et al., 2020; Si et al., 2021). Currently, the pm2/3 copy number variation is being used as a molecular surveillance resistance marker, but further studies are needed to discover the molecular basis of piperaquine resistance and epistatic interactions.
Epidemiology studies have predicted relationships between antimalarial drug resistance and various genetic factors. Genome editing in combination with Cas9-constitutive-expressing parasites and linearized-donor DNA templates enables the knock-in of large-sized DNAs, such as gene coding sequences including regulatory regions (Nishi et al., 2021). This system is useful to evaluate the causality between copy numbers of drug resistance-associated genes and drug resistance. Additional asexually dispensable gene loci are available for the generation of transgenic parasites with higher copy numbers of target genes and subsequent analysis of correlation with drug resistance, of importance since field isolates with three or more copies of specific genomic regions have been found (Ahouidi et al., 2021). Improvement of this system could allow us to find novel associations between gene copy number and antimalarial drug resistance by further copy number variation analysis in field studies. Identifying the relevance of the association through a genome editing-based gene amplification such as used in this study would provide important information for determining drug strategies for malaria control.
Data Availability Statement
The original contributions presented in the study are included in the article/Supplementary Material. Further inquiries can be directed to the corresponding author.
Ethics Statement
Ethical approvals for the use of human red blood cells (RBCs) and plasma from the Japanese Red Cross Tokyo Blood Center were obtained from the Medical Research Ethical Committee of the Tokyo Medical and Dental University. Written informed consent from the patients/ participants or patients/participants legal guardian/next of kin was not required to participate in this study in accordance with the national legislation and the institutional requirements.
Author Contributions
RK, SI, and NS conceived and designed experiments. RK and NS conducted experiments and analyzed the data. All authors wrote the manuscript and contributed to the article and approved the submitted version.
Funding
This work was supported in part by the e-ASIA Joint Research Program (20jm0210061) under Grant number 21wm0225014, supported by the Japan Agency for Medical Research and Development (AMED); Grants-in-Aid for Scientific Research (18K07084 and 19H03459), which was funded by the Japan Society for the Promotion of Science (JSPS); and grants from Mochida Memorial Foundation for Medical and Pharmaceutical Research, SENSHIN Medical Research Foundation and Ohyama Health Foundation. The funders had no role in the study design, data collection, and analysis; decision to publish; or preparation of the manuscript.
Conflict of Interest
The authors declare that the research was conducted in the absence of any commercial or financial relationships that could be construed as a potential conflict of interest.
Publisher’s Note
All claims expressed in this article are solely those of the authors and do not necessarily represent those of their affiliated organizations, or those of the publisher, the editors and the reviewers. Any product that may be evaluated in this article, or claim that may be made by its manufacturer, is not guaranteed or endorsed by the publisher.
Acknowledgments
We appreciate the Japanese Red Cross Society for providing human RBCs and plasma. We are grateful to the members of the Department of Parasitology and Tropical Medicine, Tokyo Medical and Dental University for their support. We also would like to thank Dr. Thomas Templeton for the critical reading of the manuscript.
Supplementary Material
The Supplementary Material for this article can be found online at: https://www.frontiersin.org/articles/10.3389/fcimb.2022.915656/full#supplementary-material
Abbreviations
mdr1, multidrug resistance protein 1; pm2, plasmepsin 2; pm3, plasmepsin 3.
References
Agrawal, S., Moser, K. A., Morton, L., Cummings, M. P., Parihar, A., Dwivedi, A., et al. (2017). Association of a Novel Mutation in the Plasmodium falciparum Chloroquine Resistance Transporter With Decreased Piperaquine Sensitivity. J. Infect. Dis. 216 (4), 468–476. doi: 10.1093/infdis/jix334
Ahouidi, A., Ali, M., Almagro-Garcia, J., Amambua-Ngwa, A., Amaratunga, C., Amato, R., et al. (2021). An Open Dataset of Plasmodium Genome Variation in 7,000 Worldwide Samples. Wellcome. Open Res. 6, 42. doi: 10.12688/wellcomeopenres.16168.2
Amato, R., Lim, P., Miotto, O., Amaratunga, C., Dek, D., Pearson, R. D., et al. (2017). Genetic Markers Associated With Dihydroartemisinin-Piperaquine Failure in Plasmodium falciparum Malaria in Cambodia: A Genotype-Phenotype Association Study. Lancet Infect. Dis. 17 (2), 164–173. doi: 10.1016/S1473-3099(16)30409-1
Ariey, F., Witkowski, B., Amaratunga, C., Beghain, J., Langlois, A. C., Khim, N., et al. (2014). A Molecular Marker of Artemisinin-Resistant Plasmodium falciparum Malaria. Nature 505 (7481), 50–55. doi: 10.1038/nature12876
Ashley, E. A., Dhorda, M., Fairhurst, R. M., Amaratunga, C., Lim, P., Suon, S., et al. (2014). Spread of Artemisinin Resistance in Plasmodium falciparum Malaria. N. Engl. J. Med. 371 (5), 411–423. doi: 10.1056/NEJMoa1314981
Balikagala, B., Fukuda, N., Ikeda, M., Katuro, O. T., Tachibana, S. I., Yamauchi, M., et al. (2021). Evidence of Artemisinin-Resistant Malaria in Africa. N. Engl. J. Med. 385 (13), 1163–1171. doi: 10.1056/NEJMoa2101746
Bhatt, S., Weiss, D. J., Cameron, E., Bisanzio, D., Mappin, B., Dalrymple, U., et al. (2015). The Effect of Malaria Control on Plasmodium falciparum in Africa Between 2000 and 2015. Nature 526 (7572), 207–211. doi: 10.1038/nature15535
Chappell, L., Ross, P., Orchard, L., Russell, T. J., Otto, T. D., Berriman, M., et al. (2020). Refining the Transcriptome of the Human Malaria Parasite Plasmodium falciparum Using Amplification-Free RNA-Seq. BMC Genomics 21 (1), 395. doi: 10.1186/s12864-020-06787-5
Dhingra, S. K., Redhi, D., Combrinck, J. M., Yeo, T., Okombo, J., Henrich, P. P., et al. (2017). A Variant PfCRT Isoform Can Contribute to Plasmodium falciparum Resistance to the First-Line Partner Drug Piperaquine. mBio 8 (3), e00303–17. doi: 10.1128/mBio.00303-17
Dini, S., Zaloumis, S., Cao, P., Price, R. N., Fowkes, F. J. I., van der Pluijm, R. W., et al. (2018). Investigating the Efficacy of Triple Artemisinin-Based Combination Therapies for Treating Plasmodium falciparum Malaria Patients Using Mathematical Modeling. Antimicrob. Agents Chemother. 62 (11), e01068–18. doi: 10.1128/AAC.01068-18
Duru, V., Khim, N., Leang, R., Kim, S., Domergue, A., Kloeung, N., et al. (2015). Plasmodium falciparum Dihydroartemisinin-Piperaquine Failures in Cambodia are Associated With Mutant K13 Parasites Presenting High Survival Rates in Novel Piperaquine In Vitro Assays: Retrospective and Prospective Investigations. BMC Med. 13, 305. doi: 10.1186/s12916-015-0539-5
Ecker, A., Lehane, A. M., Clain, J., Fidock, D. A. (2012). Pfcrt and Its Role in Antimalarial Drug Resistance. Trends Parasitol. 28, 504–514. doi: 10.1016/j.pt.2012.08.002
Huang, F., Shrestha, B., Liu, H., Tang, L. H., Zhou, S. S., Zhou, X. N., et al. (2020). No Evidence of Amplified Plasmodium falciparum Plasmepsin II Gene Copy Number in an Area With Artemisinin-Resistant Malaria Along the China-Myanmar Border. Malar. J. 19 (1), 334. doi: 10.1186/s12936-020-03410-6
Ikeda, M., Kaneko, M., Tachibana, S. I., Balikagala, B., Sakurai-Yatsushiro, M., Yatsushiro, S., et al. (2018). Artemisinin-Resistant Plasmodium falciparum With High Survival Rates, Uganda 2014-2016. Emerg. Infect. Dis. 24 (4), 718–726. doi: 10.3201/eid2404.170141
Leang, R., Barrette, A., Bouth, D. M., Menard, D., Abdur, R., Duong, S., et al. (2013). Efficacy of Dihydroartemisinin-Piperaquine for Treatment of Uncomplicated Plasmodium falciparum and Plasmodium vivax in Cambodia 2008 to 2010. Antimicrob. Agents Chemother. 57 (2), 818–826. doi: 10.1128/AAC.00686-12
Loesbanluechai, D., Kotanan, N., de Cozar, C., Kochakarn, T., Ansbro, M. R., Chotivanich, K., et al. (2019). Overexpression of Plasmepsin II and Plasmepsin III Does Not Directly Cause Reduction in Plasmodium falciparum Sensitivity to Artesunate, Chloroquine and Piperaquine. Int. J. Parasitol. Drugs Drug Resist. 9, 16–22. doi: 10.1016/j.ijpddr.2018.11.004
Mohring, F., Hart, M. N., Rawlinson, T. A., Henrici, R., Charleston, J. A., Diez Benavente, E., et al. (2019). Rapid and Iterative Genome Editing in the Malaria Parasite Plasmodium knowlesi Provides New Tools for P. vivax Research. Elife 8, e45829. doi: 10.7554/eLife.45829
Montenegro, L. M., de Las Salas, B., Neal, A. T., Tobon-Castaño, A., Fairhurst, R. M., Lopera-Mesa, T. M. (2021). State of Artemisinin and Partner Drug Susceptibility in Plasmodium falciparum Clinical Isolates From Colombia. Am. J. Trop. Med. Hyg. 104 (1), 263–270. doi: 10.4269/ajtmh.20-0148
Morita, M., Kanoi, B. N., Shinzawa, N., Kubota, R., Takeda, H., Sawasaki, T., et al. (2021). AGIA Tag System for Ultrastructural Protein Localization Analysis in Blood-Stage Plasmodium falciparum. Front. Cell. Infect. Microbiol. 11. doi: 10.3389/fcimb.2021.777291
Nishi, T., Shinzawa, N., Yuda, M., Iwanaga, S. (2021). Highly Efficient CRISPR/Cas9 System in Plasmodium falciparum Using Cas9-Expressing Parasites and a Linear Donor Template. Sci. Rep. 11 (1), 18501. doi: 10.1038/s41598-021-97984-z
Nsanzabana, C. (2019). Resistance to Artemisinin Combination Therapies (ACTs): Do Not Forget the Partner Drug! Trop. Med. Infect. Dis. 4 (1), 26. doi: 10.3390/tropicalmed4010026
Okell, L. C., Reiter, L. M., Ebbe, L. S., Baraka, V., Bisanzio, D., Watson, O. J., et al. (2018). Emerging Implications of Policies on Malaria Treatment: Genetic Changes in the Pfmdr-1 Gene Affecting Susceptibility to Artemether-Lumefantrine and Artesunate-Amodiaquine in Africa. BMJ Glob. Health 3 (5), e000999. doi: 10.1136/bmjgh-2018-000999
Phompradit, P., Muhamad, P., Wisedpanichkij, R., Chaijaroenkul, W., Na-Bangchang, K. (2014). Four Years' Monitoring of In Vitro Sensitivity and Candidate Molecular Markers of Resistance of Plasmodium falciparum to Artesunate-Mefloquine Combination in the Thai-Myanmar Border. Malar. J. 13, 23. doi: 10.1186/1475-2875-13-23
Phyo, A. P., Ashley, E. A., Anderson, T. J. C., Bozdech, Z., Carrara, V. I., Sriprawat, K., et al. (2016). Declining Efficacy of Artemisinin Combination Therapy Against P. falciparum Malaria on the Thai-Myanmar Border, (2003-2013): The Role of Parasite Genetic Factors. Clin. Infect. Dis. 63 (6), 784–791. doi: 10.1093/cid/ciw388
Price, R. N., Uhlemann, A. C., Brockman, A., McGready, R., Ashley, E., Phaipun, L., et al. (2004). Mefloquine Resistance in Plasmodium falciparum and Increased Pfmdr1 Gene Copy Number. Lancet 364 (9432), 438–447. doi: 10.1016/S0140-6736(04)16767-6
Price, R. N., Uhlemann, A. C., van Vugt, M., Brockman, A., Hutagalung, R., Nair, S., et al. (2006). Molecular and Pharmacological Determinants of the Therapeutic Response to Artemether-Lumefantrine in Multidrug-Resistant Plasmodium falciparum Malaria. Clin. Infect. Dis. 42 (11), 1570–1577. doi: 10.1086/503423
Ross, L. S., Dhingra, S. K., Mok, S., Yeo, T., Wicht, K. J., Kümpornsin, K., et al. (2018). Emerging Southeast Asian PfCRT Mutations Confer Plasmodium falciparum Resistance to the First-Line Antimalarial Piperaquine. Nat. Commun. 9 (1), 3314. doi: 10.1038/s41467-018-05652-0
Shinzawa, N., Nishi, T., Hiyoshi, F., Motooka, D., Yuda, M., Iwanaga, S. (2020). Improvement of CRISPR/Cas9 System by Transfecting Cas9-Expressing Plasmodium berghei With Linear Donor Template. Commun. Biol. 3 (1), 426. doi: 10.1038/s42003-020-01138-2
Sidhu, A. B., Uhlemann, A. C., Valderramos, S. G., Valderramos, J. C., Krishna, S., Fidock, D. A. (2006). Decreasing Pfmdr1 Copy Number in Plasmodium falciparum Malaria Heightens Susceptibility to Mefloquine, Lumefantrine, Halofantrine, Quinine, and Artemisinin. J. Infect. Dis. 194 (4), 528–535. doi: 10.1086/507115
Sidhu, A. B., Valderramos, S. G., Fidock, D. A. (2005). Pfmdr1 Mutations Contribute to Quinine Resistance and Enhance Mefloquine and Artemisinin Sensitivity in Plasmodium falciparum. Mol. Microbiol. 57 (4), 913–926. doi: 10.1111/j.1365-2958.2005.04729.x
Si, Y., Zeng, W., Li, N., Wang, C., Siddiqui, F., Zhang, J., et al. (2021). In Vitro Susceptibility of Plasmodium falciparum Isolates From the China-Myanmar Border Area to Piperaquine and Association With Candidate Markers. Antimicrob. Agents Chemother 65 (5), e02305-20. doi: 10.1128/AAC.02305-20
Toenhake, C. G., Fraschka, S. A., Vijayabaskar, M. S., Westhead, D. R., van Heeringen, S. J., Bártfai, R. (2018). Chromatin Accessibility-Based Characterization of the Gene Regulatory Network Underlying Plasmodium falciparum Blood-Stage Development. Cell Host Microbe 23 (4), 557–69.e9. doi: 10.1016/j.chom.2018.03.007
Triglia, T., Wang, P., Sims, P. F., Hyde, J. E., Cowman, A. F. (1998). Allelic Exchange at the Endogenous Genomic Locus in Plasmodium falciparum Proves the Role of Dihydropteroate Synthase in Sulfadoxine-Resistant Malaria. EMBO J. 17 (14), 3807–3815. doi: 10.1093/emboj/17.14.3807
van der Pluijm, R. W., Imwong, M., Chau, N. H., Hoa, N. T., Thuy-Nhien, N. T., Thanh, N. V., et al. (2019). Determinants of Dihydroartemisinin-Piperaquine Treatment Failure in Plasmodium falciparum Malaria in Cambodia, Thailand, and Vietnam: A Prospective Clinical, Pharmacological, and Genetic Study. Lancet Infect. Dis. 19 (9), 952–961. doi: 10.1016/S1473-3099(19)30391-3
van der Pluijm, R. W., Tripura, R., Hoglund, R. M., Pyae Phyo, A., Lek, D., Ul Islam, A., et al. (2020). Triple Artemisinin-Based Combination Therapies Versus Artemisinin-Based Combination Therapies for Uncomplicated Plasmodium falciparum Malaria: A Multicentre, Open-Label, Randomised Clinical Trial. Lancet 395 (10233), 1345–1360. doi: 10.1016/S0140-6736(20)30552-3
Veiga, M. I., Dhingra, S. K., Henrich, P. P., Straimer, J., Gnädig, N., Uhlemann, A. C., et al. (2016). Globally Prevalent PfMDR1 Mutations Modulate Plasmodium falciparum Susceptibility to Artemisinin-Based Combination Therapies. Nat. Commun. 7, 11553. doi: 10.1038/ncomms11553
Veiga, M. I., Ferreira, P. E., Malmberg, M., Jörnhagen, L., Björkman, A., Nosten, F., et al. (2012). Pfmdr1 Amplification Is Related to Increased Plasmodium falciparum In Vitro Sensitivity to the Bisquinoline Piperaquine. Antimicrob. Agents Chemother. 56 (7), 3615–3619. doi: 10.1128/AAC.06350-11
WHO. World Malaria Report (2021). Available at: https://www.who.int/publications/i/item/9789240040496.
Wichers, J. S., Scholz, J. A. M., Strauss, J., Witt, S., Lill, A., Ehnold, L. I., et al. (2019). Dissecting the Gene Expression, Localization, Membrane Topology, and Function of the Plasmodium falciparum STEVOR Protein Family. mBio 10 (4). doi: 10.1128/mBio.01500-19
Keywords: Plasmodium falciparum, CRISPR/Cas9, mdr1, plasmepsin, drug resistance
Citation: Kubota R, Ishino T, Iwanaga S and Shinzawa N (2022) Evaluation of the Effect of Gene Duplication by Genome Editing on Drug Resistance in Plasmodium falciparum. Front. Cell. Infect. Microbiol. 12:915656. doi: 10.3389/fcimb.2022.915656
Received: 08 April 2022; Accepted: 01 June 2022;
Published: 05 July 2022.
Edited by:
Paul R. Gilson, Burnet Institute, AustraliaReviewed by:
Moaz Ahmad, National Institutes of Health (NIH), United StatesSlavica Pavlovic Djuranovic, Washington University in St. Louis, United States
Copyright © 2022 Kubota, Ishino, Iwanaga and Shinzawa. This is an open-access article distributed under the terms of the Creative Commons Attribution License (CC BY). The use, distribution or reproduction in other forums is permitted, provided the original author(s) and the copyright owner(s) are credited and that the original publication in this journal is cited, in accordance with accepted academic practice. No use, distribution or reproduction is permitted which does not comply with these terms.
*Correspondence: Naoaki Shinzawa, c2hpbnphd2EudmlwQHRtZC5hYy5qcA==