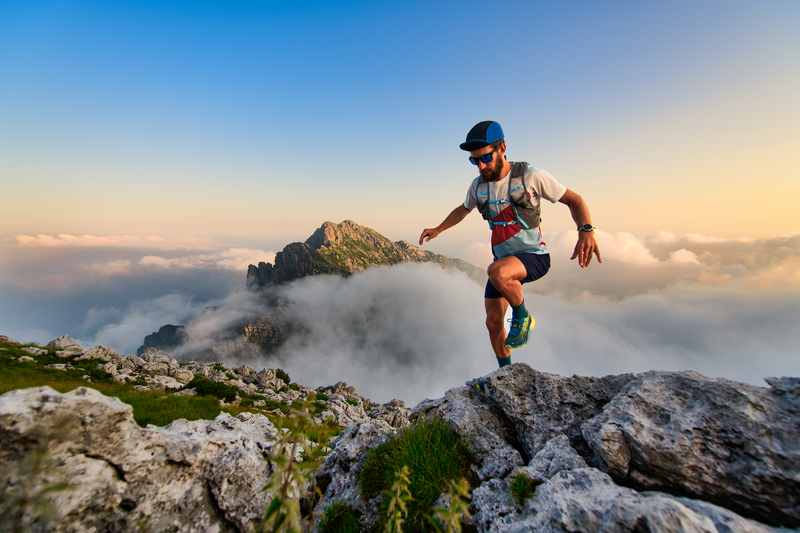
94% of researchers rate our articles as excellent or good
Learn more about the work of our research integrity team to safeguard the quality of each article we publish.
Find out more
REVIEW article
Front. Cell. Infect. Microbiol. , 24 June 2022
Sec. Molecular Bacterial Pathogenesis
Volume 12 - 2022 | https://doi.org/10.3389/fcimb.2022.913292
This article is part of the Research Topic Pathogenic Neisseria: Pathogenicity, Vaccines, and Antibiotic Resistance View all 6 articles
Neisseria meningitidis and Neisseria gonorrhoeae are two obligate human pathogens that have evolved to be uniquely adapted to their host. The meningococcus is frequently carried asymptomatically in the nasopharynx, while gonococcal infection of the urogenital tract usually elicits a marked local inflammatory response. Other members of the Neisseria genus are abundant in the upper airway where they could engage in co-operative or competitive interactions with both these pathogens. Here, we briefly outline the potential sites of contact between Neisseria spp. in the body, with emphasis on the upper airway, and describe the growing yet circumstantial evidence for antagonism from carriage studies and human volunteer challenge models with Neisseria lactamica. Recent laboratory studies have characterized antagonistic mechanisms that enable competition between Neisseria species. Several of these mechanisms, including Multiple Adhesin family (Mafs), Two Partner Secretion Systems, and Type VI secretion system, involve direct contact between bacteria; the genetic organisation of these systems, and the domain structure of their effector molecules have striking similarities. Additionally, DNA from one species of Neisseria can be toxic to another species, following uptake. More research is needed to define the full repertoire of antagonistic mechanisms in Neisseria spp., their distribution in strains, their range of activity, and contribution to survival in vivo. Understanding the targets of effectors could reveal how antagonistic relationships between close relatives shape subsequent interactions between pathogens and their hosts.
Neisseria meningitidis and Neisseria gonorrhoeae are closely related bacteria which are well-known human pathogens. N. meningitidis is a leading cause of community acquired sepsis and meningitis, particularly in children and young adults (Nadel and Ninis, 2018). Despite being a feared pathogen, N. meningitidis colonises the upper respiratory tract of healthy individuals without causing symptoms; only a small fraction of people who become colonised with the meningococcus subsequently go on to develop invasive disease. The bacterium can interact with epithelial cells via Type four pili, which allow initial attachment, mediate signaling and promote the formation of bacterial aggregates referred to as microcolonies (Pujol et al., 1997).
In contrast to the meningococcus, acquisition of N. gonorrhoeae, which typically colonises the urogenital tract, often results in local symptoms indicative of an inflammatory response (Hobbs et al., 2011). The development of a purulent discharge and/or dysuria usually prompts treatment with antibiotics, and eradication of infection after a few days (Unemo et al., 2019). In addition, asymptomatic infection with N. gonorrhoeae can also occur in both males and females (Chang et al., 2020).
Both species are exquisitely adapted to humans and are not found in any other host or in the external environment. Therefore, the evolution and virulence of both pathogens will be shaped by the environments they encounter in infected individuals. The microbiome is an aspect of the host environment which is receiving increasing attention because of the emerging evidence that it has a major impact on health and illness (Lynch and Pedersen, 2016). Within the gastrointestinal tract, there is clear evidence that the microbiome can exclude pathogens by competing for common host resources including nutrients and receptors. In addition, bacteria can gain an advantage over competitors occupying the same site by direct antagonism (Sassone-Corsi and Raffatellu, 2015). Far less is known about the interaction between bacteria at other body sites.
Aside from N. meningitidis and N. gonorrhoeae, several other members of the genus Neisseria are carried by humans (Liu et al., 2015) so have the potential to interact with the meningococcus and gonococcus. As discussed in this review, little is known about the co-existence and community behaviour of these different species, or whether competition has any role in shaping colonization and pathogenesis. However, it is now apparent that members of this genus possess tools with potential to antagonize their close neighbors. Here we review current knowledge about the sites colonised by both pathogenic and commensal species of Neisseria, highlight relevant epidemiological studies that point to potential antagonism between members of this genus in the upper airways, and detail evidence of antagonistic factors produced by Neisseria spp. We also discuss future avenues for research and discuss roles that competitive interactions between these related bacteria might play in shaping pathogen biology.
The duration of N. meningitidis carriage has been examined in a few longitudinal studies, which indicate that any given strain can persist in a host for a period of over several months (Glitza et al., 2008). The rate of carriage is markedly affected by the host’s age, housing and other social behaviors. The proportion of carriers rises in teenagers from low levels in childhood (around 1-2%) to peak levels (around 20-40%) between the ages of 20-24 years (Cartwright et al., 1987; Caugant et al., 1994). Overcrowding and living in cramped conditions are associated with increased meningococcal carriage, as seen in students and military recruits (Glover, 1918; Neal et al., 2000). Other factors consistently associated with increased carriage include male sex, active/passive smoking, and recent kissing (Maclennan et al., 2006).
The non-pathogenic Neisseria spp. are also residents of the upper airway (Liu et al., 2015). Overall, the most prevalent genera found in the upper respiratory tract are Streptococcus, Fusobacterium, and Prevotella (Kumpitsch et al., 2019). However, microbiome studies using 16S rRNA amplification and sequencing have highlighted the presence of Neisseria spp. at multiple sites in the nasopharynx and oral cavity, as the fourth most abundant genus (Ramos-Sevillano et al., 2018) suggesting there is opportunity for Neisseria spp., to interact with each other in this niche. However, a significant limitation of this is that 16S sequencing lacks the discriminatory power to distinguish between Neisseria species. As a consequence, the overwhelming majority of studies that have examined human colonization with Neisseria spp. have relied on culture and characterization of bacteria. Although this allows definitive species identification, the initial recovery of Neisseria from samples usually relies on plating to ‘selective’ media (i.e., containing agents such as colistin) to allow growth of N. meningitidis (the subject of most colonization studies) but suppress growth of other members of the flora. This reduces sensitivity and can introduce bias in results; for example, species including Neisseria cinerea, Neisseria sicca, Neisseria perflava, and Neisseria mucosa, are sensitive to agents such as colistin, so might not be recovered (Sáez Nieto et al., 1998; Clark et al., 2021). Specific methods have been developed to detect species such as N. cinerea, which was found in the nasopharynx of over 28% of healthy individuals attending a sexual health clinic in San Francisco (Knapp and Hook, 1988). In addition, N. mucosa, N. sicca, N. subflava, Neisseria lactamica, Neisseria polysaccharea, Neisseria bergheri and possibly novel Neisseria species have been isolated from the pharynx/oropharynx using culture-based approaches (Sheikhi et al., 2015; Diallo et al., 2016; Diallo et al., 2019; Calder et al., 2020).
A study of 40 healthy adults examined the co-colonization of different Neisseria species during nasopharyngeal carriage (Sáez Nieto et al., 1998). Virtually all participants carried N. perflava-sicca with 45% of people carrying at least one additional member of Neisseria spp. Of those carrying Neisseria spp., pulsed-field gel electrophoresis of isolates revealed that just over half were colonised by multiple strains of the same species. This is an important finding given that even members of the same species can have different antagonistic potential (e.g., Type VI secretion systems, see below). This work provides evidence of the co-existence of multiple species within the same person, so there is scope for interaction between species and strains.
Evidence of co-colonization was confirmed in an important study that analyzed data from the Human Metagenomic Project (HMP) for the presence of Neisseria in the upper airway from 520 healthy individuals aged 18-40 years (Donati et al., 2016). The use of sequence data from the HMP circumvents limitations of culture-based methods, and also has the advantage that samples were taken from multiple sites in the oropharynx, providing some spatial resolution of the distribution of Neisseria. The authors assembled core genomes for each species from the metagenomic data to determine their presence in samples. Interestingly, there is clear evidence of tissue tropism for different Neisseria spp. in the oropharynx. For example, samples from the surface of the tongue were enriched for Neisseria flavescens and N. subflava, while gingival plaque harbored a combination of N. mucosa, N. sicca, and Neisseria macacae. These sites contained the most abundant and diverse populations of Neisseria, while longitudinal samples revealed stable colonization with the same strain on the dorsum of the tongue, similar to the longer-term carriage observed for N. meningitidis. Of note, N. meningitidis was not prevalent in this population and was predominantly found in the pharynx. Unfortunately, there were fewer samples from this site compared with the oral cavity so further work is needed to define the community of Neisseria spp. that co-exist with the meningococcus in the throat.
Work in the early 1970s showed that the upper respiratory tract is also an occasional home for N. gonorrhoeae (Bro-jorgensen and Jensen, 1973; Wiesner et al., 1973). This bacterium is the causative agent of gonorrhoea and provokes an acute inflammatory response in the urogenital tract, its usual residence in humans. However, the gonococcus can also be isolated from the oropharynx, with carriage often associated with infection at other body sites, such as the genitourinary tract (Peters et al., 2011). Usually, the gonococcus does not cause symptoms when found in the upper respiratory tract, but it still poses a threat due to the ability of obtaining resistance mutations from the commensal Neisseria community (Spratt et al., 1992; Wadsworth et al., 2018; Fiore et al., 2020).
In contrast to the upper airway, Neisseria spp. are not a significant component of the microbiome of the genitourinary tract, with most information available for the vaginal flora. Based on early studies, which relied on limited culture-based recovery and identification, some commensal Neisseria spp. such as Neisseria catarrhalis, N. sicca, N. flava, and N. lactamica have been occasionally recovered from the genital tract, where they generally cause no local symptoms (Wilkinson, 1952; Johnson, 1983). For example, at one sexual health clinic, N. lactamica was only isolated once from over 20,000 patients (Telfer Brunton et al., 1980). Instead, Lactobacillus predominates in the vaginal microbiota, and shares this niche with other genera including Gardnerella, Atopobium, Prevotella, and Streptococcus (Ravel et al., 2011). The low pH of the female vagina is generated by acid-producing strains of Lactobacillus and is likely detrimental for the survival of N. gonorrhoeae and other Neisseria spp. (Graver and Wade, 2011). Therefore, an important factor for genitourinary infection with the gonococcus might be disruption of the healthy microbiome, as seen in conditions such as bacterial vaginosis (Fredricks et al., 2005). Far less is known about the flora of the urethra in males, even though it might influence whether exposure to the gonococcus leads to acquisition and the development of disease. Of note, N. meningitidis sometimes causes a clinical syndrome which is indistinguishable from gonorrhoea, with purulent urethritis and/or cervicitis; outbreaks of meningococcal urethritis have been documented in high-risk populations (for review see (Humbert and Christodoulides, 2019). Interestingly, the meningococcal strains that cause symptomatic urogenital disease share certain features, such as loss of capsule expression and changes in respiratory pathways that might enable them to adapt to this niche (Tzeng et al., 2017).
In summary, current scientific knowledge suggests that the upper airway is the predominant niche where Neisseria species may encounter one another. However, although carriage surveys have indicated the prevalence of different Neisseria species among populations and age groups, further unbiased sequence-based studies of co-colonization of the nasopharynx and urogenital tract by Neisseria species and strains are required for a clearer picture of the relevance and frequency of multi-species and multi-strain colonization which can guide studies on bacterial antagonism.
Current insights into relationships between Neisseria species in vivo have been gained from studies showing the age-related, inverse association of carriage of N. lactamica and N. meningitidis, and observations from human challenge experiments (Gold et al., 1978; Evans et al., 2011; Deasy et al., 2015). N. lactamica is perhaps the best characterized species among the non-pathogenic Neisseria species and has been investigated largely for its potential to elicit protective immune responses against meningococci, both through colonization (Gold et al., 1978) and by its use as a potential vaccine, either as N. lactamica derived outer membrane vesicles (Gorringe et al., 2009) or more recently as a live, recombinant strain for delivery of meningococcal antigens (Laver et al., 2021). N. lactamica is frequently carried asymptomatically in the nasopharynx of infants and children (Gold et al., 1978; Kristiansen et al., 2012; Diallo et al., 2016). Gold et al., observed that in the first four years of life, 59% of children have carried N. lactamica at least once (Gold et al., 1978). On the other hand, carriage of meningococcus is less frequent in these younger age groups (Gold et al., 1978; Holten et al., 1978; Gelosa, 1981; Saez-Nieto et al., 1985; Cartwright et al., 1987; Diallo et al., 2016). Interestingly, in older age groups the inverse pattern is observed i.e., in young adults, meningococcal carriage is prevalent, but N. lactamica is less frequent (Gold et al., 1978; Kristiansen et al., 2012; Diallo et al., 2016). This epidemiological relationship has been proposed to be due to N. lactamica inducing cross protective humoral immunity against meningococcus (Gold et al., 1978; Evans et al., 2011). The ability of the meningococcus but not N. lactamica to utilize propionate produced by anaerobes that are enriched in the adult upper airway microbiome has also been proposed as a possible explanation (Catenazzi et al., 2014). Experimental human infection experiments have provided further insight into carriage of N. lactamica and the relationship with carriage of N. meningitidis. In a study by Evans et al, in which adult volunteers aged 18-45 years were experimentally inoculated with N. lactamica, over 60% became colonised. Some individuals sustained colonization for 24 weeks, demonstrating that this organism can establish colonization of adults (Evans et al., 2011). Notably however, none of 26 individuals who were colonised after inoculation with N. lactamica acquired N. meningitidis throughout the duration of the study, while three out of 20 control subjects did. Although an increase in cross reactive anti-meningococcal opsonophagocytic responses (which significantly increased in colonised individuals) could account for this, immunization with N. lactamica OMVs did not prevent N. meningitidis carriage, leading the authors to propose that the presence of N. lactamica in the nasopharynx, rather than any subsequent immune responses, may inhibit acquisition of the meningococcus. In a subsequent study, 149 18–25-year-old university students were inoculated with N. lactamica (Deasy et al., 2015); by two weeks after inoculation 33% were colonised by the commensal species and carriage rates remained around 20-30% over 26 weeks. Importantly, carriage of N. meningitidis increased over time in the control group, but there was a significant reduction in meningococcal carriage in the participants who were colonised with N. lactamica. Furthermore, in individuals who were carriers of meningococcus at the time of inoculation, there was a greater reduction of N. meningitidis carriage after two weeks in those who became colonised by N. lactamica compared to those who were inoculated but not colonised. This indicates that colonization with N. lactamica could both prevent acquisition of the pathogen and displace existing N. meningitidis (Deasy et al., 2015). Similarly, colonization by N. lactamica following challenge was lower in individuals carrying N. meningitidis at the time of challenge compared with those who were not colonised by meningococcus, suggesting that carriage of meningococcus could inhibit colonization by N. lactamica (Deasy et al., 2015). These findings suggest that presence of either species can impact successful establishment of colonization of the human nasopharynx by a closely related organism, a concept that is consistent with the low frequency of co-colonization by both organisms reported by others (Simmons et al., 2000; Cleary et al., 2016).
Currently only a few studies have explored competitive interactions between Neisseria species in vitro. The commensal N. cinerea has been shown to impact association of the meningococcus with human epithelial cells; both pre-infection of cells with N. cinerea followed by N. meningitidis, or simultaneous infection with both species in an equal ratio leads to a relative reduction in the association of the pathogen with cells, compared to single species infections (Custodio et al., 2020). It is not clear what underlies this observation. In other studies, intra- or inter-species competition in Neisseria has been examined in the context of specific factors predicted to function as inhibitory molecules, such as polymorphic toxins, bacteriocins and more recently methylated DNA as discussed below.
Inhibitory interactions between bacteria can be mediated by secreted or translocated proteinaceous polymorphic toxins. These mediators of bacterial antagonism follow some general design principles; they consist of components necessary for secretion, a toxin, and an immunity protein. The toxin is generally a multidomain protein with the N-terminal domain implicated in secretion and the C-terminal domain mediating the toxic activity (Ruhe et al., 2020). Usually, the gene immediately downstream of the toxin-encoding gene codes for the immunity protein. These immunity proteins prevent self-intoxication, but also confer immunity against related toxins secreted by kin (Zhang et al., 2012).
The MafABI system, which constitutes a novel group of polymorphic toxins in Neisseria spp., contains the outer membrane-associated protein MafA, the two-domain toxin MafB, and the immunity protein MafI (Arenas et al., 2015; Jamet et al., 2015). maf genes are prevalent in pathogenic Neisseria and are estimated to comprise about 2% of the genome (Jamet et al., 2015). In N. meningitidis and N. gonorrhoeae, maf genes are organized into five maf genomic islands (MGI-1 to MGI-5) which are classified based on conserved locations in the genomes. MGI-1, -2 and -3 are found in meningococci and gonococci, while MGI-4 and -5 are only present in gonococcal genomes. A scheme of a maf island (MGI-1) is shown in Figure 1. All MGIs contain a mafA gene but differ in the number of full-length mafB and mafI genes. MGIs also harbor a variable number of truncated mafB genes with cognate mafI genes. This feature, of having multiple copies of genes encoding C-terminal toxin domains with a cognate immunity protein, is common to loci for several polymorphic toxins (Figure 1). maf genes also exist in commensal Neisseria. MGI-1 is found in N. cinerea (ATCC 14685) and N. polysaccharea (ATCC 43768), N. lactamica 020-06 has MGI-1, MGI-3 and frameshifted MGI-2 and MGI-5, and N. flavescens (NRL30031/H210) have a fragmented MGI-5. Based on the presence of both intact mafA and mafB in some of the MGIs they could potentially still be functional. In contrast, N. elongata (ATCC29315), N. mucosa (ATCC 25996 and C102) and N. subflava (NJ9703) do not appear to have mafA or mafB homologues (Jamet et al., 2015), although this does not exclude the presence of maf genes in other strains.
Figure 1 Schematic of genomic organization of polymorphic toxin system loci found in Neisseria species. Examples of a Maf genomic island, a TPS island and T6SS associated effectors are shown. MGI-1 is the consensus organization of this island (Arenas et al., 2015; Jamet et al., 2015), whereas the TPS island and T6SS locus indicated are from N. meningitidis (Nm) FAM18 (Arenas et al., 2013) and N. cinerea CCUG346T (Custodio et al., 2021), respectively. The secretion elements of the Maf and Tps toxins are different between systems but are generally located in the 5’-end of the genomic islands. mafB includes a region encoding a domain of unknown function named DUF1020, tpsA includes a region encoding a filamentous hemagglutinin adhesin (FHA) domain, whereas T6SS effector genes like those described in N. cinerea (Nc) include regions encoding Rearrangement Hot Spot (RHS) domains. The repertoire of toxin genes is diverse, but each is followed by a gene encoding a cognate immunity protein.
MafB toxins themselves comprise a conserved N-terminus which includes a domain of unknown function (DUF1020 domain) that is unique to Neisseria MafB toxins (Figure 1). They can be divided into three classes, denoted as MafB1-3, based on variation in specific motifs located at the end of the conserved N-terminal domain. The C-terminal toxin domains are highly variable, meaning that different MafB toxins likely have different modes of toxicity. MafB from MGI-1 in N. meningitidis 8013 (MafBMGI-1NEM8013) was shown to be an RNase with EndoU ribonuclease activity; this toxin can degrade RNA in vitro, an activity that is blocked by the immunity protein (Jamet et al., 2015). Bioinformatic analysis has indicated that MafB2MGI-2Nm053442 also possesses an EndoU nuclease fold domain (Zhang et al., 2011). However, little is known about the specific activities of other MafB toxins.
The secretion pathway of MafB has been investigated. Due to the presence of a conserved N-terminal signal sequence, the toxin is proposed to cross the inner membrane via the Sec system (Jamet et al., 2015). Original observations that MafB could be detected in culture supernatants in the absence of a MafA led to speculation that the toxins may be secreted by association with outer membrane vesicles (Jamet et al., 2015). A later study revealed that MafA mediates MafB translocation across the outer membrane, and after secretion MafB can undergo further maturation via auto-proteolysis of a HINT domain (Arenas et al., 2020). In contrast, the path of entry of secreted toxins into target cells is poorly understood and merits further study. There is also evidence showing that there is some degree of MafA selectivity during MafB secretion. Whereas MafB1 is only secreted by MafA from the same MGI, MafB3 can be secreted by MafA from other MGIs (Arenas et al., 2020). This selectivity could be important in interpreting the potential secretion of MafB toxins in Neisseria, since certain isolates have multiple MGIs, some of which lack MafA.
The MafABI system has been shown to contribute to bacterial competition, but not all MafB toxins mediate these effects; so far only MafB toxins from MGI-2 and -3 have been associated with growth inhibitory activity (Arenas et al., 2015; Jamet et al., 2015). Overexpression of the MafB toxin (MafB1MGI-2NEM8013) and its cognate immunity protein in strain NEM8013 enabled this strain to inhibit growth of an unencapsulated N. meningitidis NEM8013 devoid of the cognate mafI gene (Jamet et al., 2015). Interestingly, expression of this same MafB toxin from a plasmid in E. coli BL21(DE3) did not impact E. coli growth (Jamet et al., 2015); whether this is due to this MafB toxin having a Neisseria specific target, or the mode of delivery of MafB is unclear. It is also of note that growth inhibition was seen when the Neisseria target strain was unencapsulated, given that many carried meningococci lack the genes required for capsule synthesis and transport (Claus et al., 2002), and the down regulation of capsule is a key step in meningococcal pathogenesis (Tzeng et al., 2016). The presence of a capsule offers protection from polymorphic toxin-mediated growth inhibition in other important human pathogens such as Acinetobacter baumannii (Krasauskas et al., 2020).
Little is known about the roles of other MafB toxins. Therefore, elucidation of toxin activity, and further competition studies with other meningococcal lineages or Neisseria species is needed to provide insight into Maf system functions. Interestingly, MGI-4 and -5 are only present in gonococcal genomes (Jamet et al., 2015) and the bacterial transcriptional regulator NadR represses expression of mafA in the presence of 4-HPA, a molecule that is present in human saliva (Liguori et al., 2016), suggesting that MafB toxins may be species specific or adapted to particular niches. Currently all studies of the MafABI system have been conducted in N. meningitidis, meaning that almost nothing is known of this system’s involvement in antagonism in N. gonorrhoeae or commensal species.
In addition to MafB toxins, meningococci can express other polymorphic toxins, which belong to the family of two-partner secretion (TPS) systems. These are widespread in Gram-negative bacteria and encode distinct functions (Guérin et al., 2017), including toxins that can mediate inter-bacterial antagonism, also known as contact dependent inhibition (CDI) systems (Aoki et al., 2005). In general, CDI toxins are large, filamentous proteins that interact with, and are secreted by, a partner transporter via sequences in their N-terminus. The C-terminal (CT) domain is required for toxicity and is delivered to the target cell after cleavage from the secreted toxin (Ruhe et al., 2018). In different bacteria these domains are highly variable and have been shown to have different toxic capabilities, such as DNase or tRNAse activities. These systems often form loci that contain the transporter, toxin and a cognate immunity protein (Ruhe et al., 2020).
In N. meningitidis, TPS systems comprise the secreted toxin, generically known as TpsA, and the partner outer membrane transporter, TpsB (Van Ulsen et al., 2008; Arenas et al., 2013). Based on genome analysis meningococci can encode up to five TpsA toxins and two TpsB transporters (Van Ulsen et al., 2008). Similar to Mafs, the genes encoding the transporter and the toxins are located in islands. Also similar to maf islands, downstream of tpsA genes are multiple shorter tpsA-like genes known as tpsC cassettes as they encode for toxic domains without the regions necessary for secretion. Adjacent to them are genes referred to as IORFS (Interspacing Open Reading Frames) which confer immunity (Arenas et al., 2013) and Figure 1).
TpsA1 from N. meningitidis (also called HrpA) has been shown to have roles in adherence (Schmitt et al., 2007), intracellular replication (Talà et al., 2008), biofilm formation (Neil and Apicella, 2009), and can also act as a CDI toxin (Arenas et al., 2013). Experimental analysis has demonstrated that a wild-type N. meningitidis strain (FAM18) which expresses only a single TpsA toxin (TpsA1, Figure 1) can inhibit growth of an un-encapsulated isogenic mutant lacking the tps island when grown together on solid media (Arenas et al., 2013). Complementation of the mutant with IORF1 abolished the growth inhibition by the wild type, providing evidence that IORFS confer immunity. The contribution of the tpsC cassettes in the tps islands to growth inhibition is not clear. Given their shared sequence homology it was proposed that they act as donors for recombination with tpsA, allowing shuffling of the toxin domain and thereby altering the activity of the system (Arenas et al., 2013). However, although there is some evidence of recombination between tpsC and tpsA loci from genome analysis and in vitro experiments, the frequency of such events is low and may even be unfavourable, possibly by affecting the repertoire of immunity genes and rendering the bacterium susceptible to growth inhibition by kin (Arenas et al., 2013). Thus, the current hypothesis for the presence of multiple tpsC-IORFs in tps islands is to provide immunity against different TpsA toxins, rather than serve as sequences for generating new toxins (Arenas et al., 2013).
Another way to deliver polymorphic toxins is via a Type VI Secretion System (T6SS). In this system toxins are delivered after they are loaded onto a multiprotein “needle” that is assembled in the cytosol and fired into target cells (Basler et al., 2013). To eject this poison-tipped needle, a machinery is built that spans both membranes of the attacking cell (Pukatzki et al., 2007; Leiman et al., 2009). This injection machinery is evolutionary linked to bacteriophages that deliver their genomic payload into prey cells (Cianfanelli et al., 2016b). T6SS toxins (usually referred to as effectors) are multidomain proteins and are related to Rearrangement Hot Spot (RHS) proteins (Hill et al., 1994). The N-terminal regions of many T6SS effectors also comprise domains with homology to one of the three main parts of the needle, the tip (PAAR), head (VgrG), or shaft (Hcp) (Zhang et al., 2012; Whitney et al., 2014; Unterweger et al., 2015; Flaugnatti et al., 2016; Ma et al., 2017). However, some toxins do not obviously contain PAAR, VgrG or Hcp homologous regions, indicating that there might be other ways they associate with the needle. Like the Maf and TPS systems, the C-terminal domain of T6SS effectors carries the toxic component (Figure 1). Examples of toxic activities include nuclease (Pissaridou et al., 2018), peptidoglycan glycoside hydrolase (Whitney et al., 2013), ion-selective pore formation (Mariano et al., 2019), phospholipase (Flaugnatti et al., 2016) and NADase (Tang et al., 2018). The RHS domain between the N- and C-terminus contains several repeating motifs, which when folded form a β-sheet cage around the toxic domain (Günther et al., 2022). At the border between these three domains are dual autoproteolytic DPxGL motifs (Jurėnas et al., 2021). It is not clear when this cleavage occurs, but since the RHS domain forms a protective shell around the C-terminal toxin, and since the effector needs to be attached to the needle, which happens via the N-terminus, proteolysis is thought to occur after secretion (Jurėnas et al., 2021). Secretion of the toxin-loaded needle happens first by assembly of the basal plate spanning the inner membrane. This basal plate contains VgrG and PAAR and therefore also in some systems toxins which share homologous regions (Cianfanelli et al., 2016a). The construction of the needle is then formed by the progressive addition of Hcp sheathed by TssBC complexes (Cherrak et al., 2019). To fire the needle, ClpV, an ATPase, induces a conformational change in TssBC, pushing the needle through the outer membrane and into a nearby target cell, delivering effectors to the cytoplasm or periplasm (Cherrak et al., 2019). So, similar to the Maf and TPS systems, the mechanisms of secretion of toxins are partly understood, but questions remain about how they are delivered into target cells.
Among Neisseria, so far T6SS genes have only been identified in some genomes of commensal species but not all members of those species have equal potential for antagonistic effects (Marri et al., 2010; Calder et al., 2020; Custodio et al., 2021). To date only a single Neisseria T6SS has been experimentally characterized, found in an isolate of N. cinerea, CCUG346T (Custodio et al., 2021). In this strain, the genes for the secretion apparatus, putative effectors and cognate immunity proteins are all located on a large plasmid. The putative effectors have predicted DNAse, RNAase or phospholipase activities and, except for one, are located along with their cognate immunity gene in proximity to the T6SS structural components. Although toxicity of the effectors has been shown by expression in E. coli (Custodio et al., 2021) their targets, and individual impact upon growth of competing Neisseria has not been determined. Nevertheless, the presence of the T6SS confers an advantage, as demonstrated by the significant T6SS-dependent reduction in recovery of prey strains of either N. cinerea that lack the T6SS, and also N. meningitidis or N. gonorrhoeae in co-culture experiments (Custodio et al., 2021). These initial findings point to a role of T6SSs in intra- and inter- species antagonism. Elucidating how widespread these systems are and understanding the diversity of effectors and immunity elements within and between the species will be of interest for understanding the importance and potential roles of T6SSs in competition between Neisseria species.
Bacteriocins are antimicrobial peptides and proteins produced by bacteria. They are generally defined as ribosomally synthesised peptides, to differentiate them from non-ribosomally produced antimicrobial products. Bacteriocins comprise a diverse group of molecules including small peptides (less than 10 kDa) which can be broadly classified according to whether they are modified or not (reviewed in (Soltani et al., 2020)), as well as larger proteins such as colicins (Cascales et al., 2007; Kleanthous, 2010) or complexes such as phage tail-like bacteriocins (Scholl, 2017). Bacteriocins can target bacterial cell membranes resulting in lysis or can exploit specific receptors and outer membrane proteins for translocation into the periplasm or cytoplasm, where they can affect fundamental processes in target cells (Rebuffat, 2022). Bacteriocin production is widespread among bacteria and, due to their potent but relatively specific activity, these molecules have been proposed as alternatives to antibiotics (Cotter et al., 2013; Telhig et al., 2020). Producer strains have self-immunity via several possible mechanisms, for example by expression of cognate immunity proteins, similar to the polymorphic toxin-immunity pairs, or export pumps (for review see (Rebuffat, 2022)). While some bacteriocins display a broad target range, many exert their antimicrobial activity on organisms belonging to the same species or genus as the producer strain. Bacteriocins are diffusible molecules, hence their expression can provide the producing strain with a competitive advantage within bacterial communities, and bacteriocin production has been shown to impact niche competition in the gastrointestinal tract in animal models (reviewed in (García-Bayona and Comstock, 2018; Heilbronner et al., 2021). Consistent with a role in shaping complex bacterial communities, many of the species in the human microbiome have the capacity to produce bacteriocins (Drissi et al., 2015; Walsh et al., 2015; Zheng et al., 2015).
The potential production of bacteriocins by pathogenic Neisseria species has been explored in several studies. Early work on N. gonorrhoeae described the production of “gonocin”, following the observation that some strains inhibited growth of other gonococci, and that the growth inhibition phenotype had characteristics of bacteriocins defined at the time (Flynn and Mcentegart, 1972; Lawton et al., 1976). However, similar investigations identified gonococcal growth inhibitory substances that were proposed to be metabolites or fatty acids and lyso-phosphatidyl ethanolamine (Walstad et al., 1974); these had broad anti-gonococcal activity, including against producer strains, not consistent with an effect mediated by a bacteriocin. A similar but undefined inhibitory activity was also reported by Knapp et al., who suggested that a toxic metabolite rather than a bacteriocin was responsible and may have led to the conflicting reports of ‘gonocin’ production (Knapp et al., 1975). A meningococcal bacteriocin, ‘meningocin’ was first proposed in a study by Kingsbury which described an inducible (by mitomycin C or UV), heat stable, protease sensitive inhibitory activity against meningococci and some non-pathogenic Neisseria (i.e., N. flavescens, N. subflava and N. perflava) (Kingsbury, 1966). Meningococcal growth inhibition against N. gonorrhoeae has also been described (Allunans and Bøvre, 1996); interestingly anti-gonococcal activity was also reported in a strain of meningococcus isolated from the urethra (Volk and Kraus, 1973). The first partial purification of a putative meningococcal bacteriocin led to the identification of the active component as a 47-48 kDa polypeptide (Allunans et al., 1998), suggestive of a colicin-type bacteriocin. However, the product was not sufficiently active or not available in sufficient amounts to allow further characterisation. Finally, two genes (NMB0097 and NMB0098) have been implicated in the secretion of a potential meningocin; homologues were not detected in any sensitive strains and deletion of either gene in a producer meningococcal strain abolished growth inhibition (Allunans et al., 2008), however the genes encoding the proposed meningococcal bacteriocin(s) have not been identified.
Despite the lack of any genetically and biochemically characterized Neisseria bacteriocin, these studies suggest that both N. meningitidis and N. gonorrhoeae have the capacity to produce antimicrobial substances that impair growth of related strains and other Neisseria spp. The presence of bacteriocin genes in genomes of Neisseria species in the oral cavity has been reported (Zheng et al., 2015) and with available whole genome sequences and online tools for identification of bacteriocin gene clusters (Walsh et al., 2015), there is scope to define the capacity of different Neisseria isolates to synthesise and secrete antimicrobial peptides, providing a better understanding of their antagonistic potential. Furthermore, the observation that both gonococci and meningococci have selective growth inhibitory properties against related strains or species, whether mediated by bacteriocins or other molecules, provides the foundation for future investigations. Indeed, in a recent antibiotic discovery project, N. mucosa was shown to display activity against other Neisseria in delayed antagonism assays, possibly through production of an antimicrobial secondary metabolite (Aho et al., 2020), revealing potentially novel and exploitable antagonism strategies among Neisseria species.
Perhaps one of the most fascinating mechanisms of antagonism described among Neisseria species is the killing of N. gonorrhoeae by Neisseria elongata DNA. These species can interact intimately via Type four pili (Tfp) which permits bacteria: bacteria interactions and DNA exchange, including the transfer of resistance elements (Higashi et al., 2011). However, N. elongata can also inhibit gonococcal growth in vitro via a DNA-uptake dependent mechanism, as well as enhance clearance of gonococci in a mouse model of cervico-vaginal infection (Kim et al., 2019). The toxicity of DNA, released by N. elongata and taken up by the naturally competent gonococcus, was shown to be due to their different DNA methylation status which would result from their different repertoires of restriction modification systems (Kim et al., 2019). The proposed model by which subsequent cell death occurs postulates that due to the relatedness of the two species, the high degree of genomic DNA sequence homology allows for multiple foci of recombination to form following uptake; at these sites mismatched methylation leads to restriction enzyme cleavage and the eventual degradation of the chromosome (Kim et al., 2019; So and Rendón, 2019). These findings may have broader implications, as DNA from several commensals had the same effect, and N. elongata DNA was also shown to kill the meningococcus (Kim et al., 2019). Thus, this DNA based killing mechanism may be an important influence on the interactions among these related organisms.
Interestingly some gonococci and meningococci can also release DNA, via a Type IV secretion system (T4SS) (Dillard and Seifert, 2001; Hamilton et al., 2005) and by autolysis (Lappann et al., 2010), respectively. N. meningitidis extracellular DNA (eDNA) contributes to biofilm stability of certain lineages of N. meningitidis and this has been proposed to impact their colonization capacity. For example strains which are unable to use eDNA for biofilm formation (i.e., those from the ST8 and ST11 lineages) may engage in more transient interactions with the host (Lappann et al., 2010). Therefore, extracellular Neisseria DNA could have multiple, contrasting influences on the ability of these organisms to successfully colonise the human nasopharynx. Also of interest, based on the model of Kim et al., is the fact that restriction modification systems undergo phase variation in Neisseria gonorrhoeae (Sánchez-Busó et al., 2019). Whether this variation could impact DNA-dependent antagonism among kin for example, is an interesting avenue to explore.
As discussed in this review, several different growth inhibitory activities and mediators of bacterial antagonism have been identified among the members of the Neisseria genus. These include examples of well characterized contact-dependent bacterial antagonism mechanisms, such as the Tps systems and T6SS, less well-characterized activities for which the genetic basis or active components are as yet undefined (bacteriocins and antimicrobials) and a novel DNA-based killing mechanism. An overview of our current understanding of these growth inhibitory activities among Neisseria species is shown in Figure 2. Although this shows that both pathogenic and non-pathogenic Neisseria species are equipped with ways to inhibit potential competitors, in most cases these have been identified and analyzed in only a limited number of strains. Systematic genomic analysis and in vitro verification of these activities across and within species is lacking. In the case of polymorphic toxins and T6SSs for which the genetic components have been defined, interrogation of publicly available Neisseria species genomic sequence data [e.g., PubMLST (Jolley et al., 2018)] using homology searches could provide insights into the distribution and diversity of the systems. In addition, online tools for identification of bacteriocin synthetic loci (Van Heel et al., 2018) may reveal candidate genes responsible for the growth inhibitory substances described in early studies.
Figure 2 Schematic overview of antagonism systems in Neisseria. Experimentally validated growth inhibitory activities of different Neisseria species are shown. Commensal Neisseria species (N. mucosa, N. cinerea and N. elongata) are shown in purple, Neisseria gonorrhoeae (red), and Neisseria meningitidis (blue) in both capsulated (with grey outline) and unencapsulated form (without grey outline) are shown. The various mediators of growth inhibition/killing are indicated, with arrows pointing from the attacker to the prey cell based on current in vitro experimental evidence. Potential bacteriocins are indicated with a question mark.
Defining the distribution and conservation of the different systems would not only provide indication of the diversity and prevalence of these systems, but would also inform the judicious choice of strains for experiments to better understand the breadth of activity of antagonistic factors. For example, the effect of Mafs and TPS toxins have so far only been examined against isogenic strains that have been genetically engineered to lack immunity factors as well as other protective features such as capsule. Moreover, our understanding of these systems at both the molecular and functional level is limited. Key questions include how their expression and secretion is regulated in different strains, how toxins target and enter neighboring cells and the precise mechanism of toxicity. In particular, defining the role of the Neisseria-specific Maf toxins might shed light on novel modes of toxin secretion or function. Furthermore, given the sequence similarity in the C-terminal toxic domains of Maf proteins and Tps toxins (Arenas et al., 2015), the conserved modular architectures of the polymorphic toxins and the intriguing genetic locus arrangements (Figure 1), the possibility of interplay between these systems merits investigation.
Importantly, although this review has focused on antagonistic mechanisms that the Neisseria spp. use against each other, it is possible that these could be effective against other members of the microbiota, or host cells, as has been observed for some T6SS effectors (Monjarás Feria and Valvano, 2020). Defining toxin or antimicrobial targets may provide a clearer picture of their potential contribution to either Neisseria specific or more widespread bacterial competition. In addition, defining the nature and mode of action of toxins or secreted compounds could lead to development of novel antimicrobials, or help define novel targets, which is of particular interest for compounds with inhibitory or killing activity against the pathogenic Neisseria and in particular for N. gonorrhoeae which has developed high levels of antibiotic resistance (Unemo et al., 2019).
There remain challenges in extrapolating in vitro evidence of antagonism to its relevance for interactions between bacteria in the upper airway. Several of these antagonistic mechanisms are ‘contact-dependent’ systems which exert effects only on close neighbors. Neisseria species usually form aggregates known as microcolonies or biofilms mediated by Type four pili. In vitro, heterogeneity in Tfp can lead to spatial segregation within colonies, even of related strains (Oldewurtel et al., 2015; Zöllner et al., 2017; Pönisch et al., 2018) and this localized sorting can impact the efficacy of contact dependent systems (Custodio et al., 2021). The development of more complex in vitro (Łaniewski et al., 2017; Audry et al., 2019) and animal models (Yi et al., 2003; Weyand et al., 2013; Johswich et al., 2015; Ma et al., 2018) offers the promise of dissecting the specific contributions of potentially antagonistic factors in the establishment and stability of microbial communities. Clearly, the ground-breaking approach of challenging human volunteers with commensal species (Evans et al., 2011; Deasy et al., 2015) would provide the most physiologically relevant information, but is not suitable for high-throughput analysis and elucidation of molecular mechanisms.
Importantly, antagonistic interactions between these related bacteria might play a key role in shaping pathogen biology and behaviour. A key feature of the pathogens N. meningitidis and N. gonorrhoeae is their ability to adapt and undergo phase and antigenic variation. These features have resulted in them being highly adept at evading host immunity. Competitive interbacterial interactions may also be a driving force for variation with potential to impact factors implicated in virulence or colonization. Therefore, future work to understand the extent to which antagonistic relationships could drive variation or evolution of specific traits and alter pathogen physiology is warranted.
In summary, the upper respiratory tract can be regarded as a busy cross-roads for Neisseria spp., where pathogenic species may reside alongside a range of commensal Neisseria species. Both the meningococcus and some commensal species establish colonization lasting months, offering opportunity for co-operation and/or competition, which could impact the composition and shape of the community. In the future, it will be important to expand our emerging knowledge of antagonistic properties of these organisms and establish their underlying mechanisms. This will allow a better understanding of their roles in shaping interactions with each other and with the host.
RB, CT, and RE wrote and critically reviewed the manuscript. RB created figures with input from RE and CT. All authors approved the submitted version.
Work in CT’s lab is supported by a Wellcome Trust Investigator award (102908/Z/13/Z) and the lab has received funding from Meningitis Now.
The authors declare that the research was conducted in the absence of any commercial or financial relationships that could be construed as a potential conflict of interest.
All claims expressed in this article are solely those of the authors and do not necessarily represent those of their affiliated organizations, or those of the publisher, the editors and the reviewers. Any product that may be evaluated in this article, or claim that may be made by its manufacturer, is not guaranteed or endorsed by the publisher.
Aho, E. L., Ogle, J. M., Finck, A. M. (2020). The Human Microbiome as a Focus of Antibiotic Discovery: Neisseria Mucosa Displays Activity Against Neisseria Gonorrhoeae. Front. Microbiol. 11, 577762. doi: 10.3389/fmicb.2020.577762
Allunans, J., Bøvre, K. (1996). Bacteriocins in Neisseria Meningitidis. Screening of Systemic Patient Strains and Pharyngeal Isolates From Healthy Carriers. Apmis 104, 206–212. doi: 10.1111/j.1699-0463.1996.tb00709.x
Allunans, J., Bjørås, M., Seeberg, E., bøvre, K. (1998). Production, Isolation and Purification of Bacteriocins Expressed by Two Strains of Neisseria Meningitidis. Apmis 106, 1181–1187. doi: 10.1111/j.1699-0463.1998.tb00275.x
Allunans, J., Kristiansen, K. I., Assalkhou, R., Bjørås, M. (2008). Bacteriocins (Meningocins) in Norwegian Isolates of Neisseria Meningitidis: Possible Role in the Course of a Meningococcal Epidemic. Apmis 116, 333–344. doi: 10.1111/j.1600-0463.2008.00850.x
Aoki, S. K., Pamma, R., Hernday, A. D., Bickham, J. E., Braaten, B. A., Low, D. A. (2005). Contact-Dependent Inhibition of Growth in Escherichia Coli. Science 309, 1245–1248. doi: 10.1126/science.1115109
Arenas, J., Catón, L., Van den hoeven, T., De maat, V., Cruz Herrero, J., Tommassen, J. (2020). The Outer-Membrane Protein Mafa of Neisseria Meningitidis Constitutes a Novel Protein Secretion Pathway Specific for the Fratricide Protein Mafb. Virulence 11, 1701–1715. doi: 10.1080/21505594.2020.1851940
Arenas, J., De maat, V., Catón, L., Krekorian, M., Herrero, J. C., Ferrara, F., et al. (2015). Fratricide Activity of Mafb Protein of N. Meningitidis Strain B16b6. BMC Microbiol. 15, 156. doi: 10.1186/s12866-015-0493-6
Arenas, J., Schipper, K., Van ulsen, P., Van der ende, A., Tommassen, J. (2013). Domain Exchange at the 3' End of the Gene Encoding the Fratricide Meningococcal Two-Partner Secretion Protein a. BMC Genomics 14, 622. doi: 10.1186/1471-2164-14-622
Audry, M., Robbe-Masselot, C., Barnier, J. P., Gachet, B., Saubaméa, B., Schmitt, A., et al. (2019). Airway Mucus Restricts Neisseria Meningitidis Away From Nasopharyngeal Epithelial Cells and Protects the Mucosa From Inflammation. mSphere 4 (6), e00494-19. doi: 10.1128/mSphere.00494-19
Basler, M., Ho, B. T., Mekalanos, J. J. (2013). Tit-For-Tat: Type VI Secretion System Counterattack During Bacterial Cell-Cell Interactions. Cell 152, 884–894. doi: 10.1016/j.cell.2013.01.042
Bro-jorgensen, A., Jensen, T. (1973). Gonococcal Pharyngeal Infections. Report of 110 Cases. Br. J. Vener. Dis. 49, 491–499. doi: 10.1136/sti.49.6.491
Calder, A., Menkiti, C. J., Çağdaş, A., Lisboa Santos, J., Streich, R., Wong, A., et al. (2020). Virulence Genes and Previously Unexplored Gene Clusters in Four Commensal Neisseria Spp. Isolated From the Human Throat Expand the Neisserial Gene Repertoire. Microb. Genom. 6, (9). doi: 10.1099/mgen.0.000423
Cartwright, K. A., Stuart, J. M., Jones, D. M., Noah, N. D. (1987). The Stonehouse Survey: Nasopharyngeal Carriage of Meningococci and Neisseria Lactamica. Epidemiol. Infect. 99, 591–601. doi: 10.1017/S0950268800066449
Cascales, E., Buchanan, S. K., Duche, D., Kleanthous, C., Lloubes, R., Postle, K., et al. (2007). Colicin Biology. Microbiol. Mol. Biol. Rev. 71, 158–229. doi: 10.1128/MMBR.00036-06
Catenazzi, M. C., Jones, H., Wallace, I., Clifton, J., Chong, J. P., Jackson, M. A., et al. (2014). A Large Genomic Island Allows Neisseria Meningitidis to Utilize Propionic Acid, With Implications for Colonization of the Human Nasopharynx. Mol. Microbiol. 93, 346–355. doi: 10.1111/mmi.12664
Caugant, D. A., Høiby, E. A., Magnus, P., Scheel, O., Hoel, T., Bjune, G., et al. (1994). Asymptomatic Carriage of Neisseria Meningitidis in a Randomly Sampled Population. J. Clin. Microbiol. 32, 323–330. doi: 10.1128/jcm.32.2.323-330.1994
Chang, S.-X., Chen, K.-K., Liu, X.-T., Xia, N., Xiong, P.-S., Cai, Y.-M. (2020). Cross-Sectional Study of Asymptomatic Neisseria Gonorrhoeae and Chlamydia Trachomatis Infections in Sexually Transmitted Disease Related Clinics in Shenzhen, China. PLoS One 15, e0234261. doi: 10.1371/journal.pone.0234261
Cherrak, Y., Flaugnatti, N., Durand, E., Journet, L., Cascales, E. (2019). Structure and Activity of the Type VI Secretion System. Microbiol. Spectr. 7 (4), 7.4.11. doi: 10.1128/microbiolspec.PSIB-0031-2019
Cianfanelli, F. R., Alcoforado Diniz, J., Guo, M., De Cesare, V., TROST, M., Coulthurst, S. J. (2016a). VgrG and PAAR Proteins Define Distinct Versions of a Functional Type VI Secretion System. PLoS Pathog. 12, e1005735. doi: 10.1371/journal.ppat.1005735
Cianfanelli, F. R., Monlezun, L., Coulthurst, S. J. (2016b). Aim, Load, Fire: The Type VI Secretion System, a Bacterial Nanoweapon. Trends Microbiol. 24, 51–62. doi: 10.1016/j.tim.2015.10.005
Clark, S. A., Gray, S., Finn, A., Borrow, R. (2021). Colistin Sensitivity and Factor H-Binding Protein Expression Among Commensal Neisseria Species. mSphere 6, e0017521. doi: 10.1128/mSphere.00175-21
Claus, H., Maiden, M. C. J., Maag, R., Frosch, M., Vogel, U. (2002). Many Carried Meningococci Lack the Genes Required for Capsule Synthesis and Transport. Microbiol. (Reading). 148, 1813–1819. doi: 10.1099/00221287-148-6-1813
Cleary, P. R., Calvert, N., Gee, S., Graham, C., Gray, S., Kaczmarski, E., et al. (2016). Variations in Neisseria Meningitidis Carriage by Socioeconomic Status: A Cross-Sectional Study. J. Public Health (Oxf). 38, 61–70. doi: 10.1093/pubmed/fdv015
Cotter, P. D., Ross, R. P., Hill, C. (2013). Bacteriocins — a Viable Alternative to Antibiotics? Nat. Rev. Microbiol. 11 (2), 95–105. doi: 10.1038/nrmicro2937
Custodio, R., Ford, R. M., Ellison, C. J., Liu, G., Mickute, G., Tang, C. M., et al. (2021). Type VI Secretion System Killing by Commensal Neisseria is Influenced by Expression of Type Four Pili. Elife 10, e63755. doi: 10.7554/eLife.63755.sa2
Custodio, R., Johnson, E., Liu, G., Tang, C. M., Exley, R. M. (2020). Commensal Neisseria Cinerea Impairs Neisseria Meningitidis Microcolony Development and Reduces Pathogen Colonisation of Epithelial Cells. PLoS Pathog. 16, e1008372. doi: 10.1371/journal.ppat.1008372
Deasy, A. M., Guccione, E., Dale, A. P., Andrews, N., Evans, C. M., Bennett, J. S., et al. (2015). Nasal Inoculation of the Commensal Neisseria Lactamica Inhibits Carriage of Neisseria Meningitidis by Young Adults: A Controlled Human Infection Study. Clin. Infect. Dis. 60, 1512–1520. doi: 10.1093/cid/civ098
Diallo, K., Maclennan, J., Harrison, O. B., Msefula, C., Sow, S. O., Daugla, D. M., et al. (2019). Genomic Characterization of Novel Neisseria Species. Sci. Rep. 9, 13742. doi: 10.1038/s41598-019-50203-2
Diallo, K., Trotter, C., Timbine, Y., Tamboura, B., Sow, S. O., Issaka, B., et al. (2016). Pharyngeal Carriage of Neisseria Species in the African Meningitis Belt. J. Infect. 72, 667–677. doi: 10.1016/j.jinf.2016.03.010
Dillard, J. P., Seifert, H. S. (2001). A Variable Genetic Island Specific for Neisseria Gonorrhoeae is Involved in Providing DNA for Natural Transformation and is Found More Often in Disseminated Infection Isolates. Mol. Microbiol. 41, 263–277. doi: 10.1046/j.1365-2958.2001.02520.x
Donati, C., Zolfo, M., Albanese, D., Tin Truong, D., Asnicar, F., Iebba, V., et al. (2016). Uncovering Oral Neisseria Tropism and Persistence Using Metagenomic Sequencing. Nat. Microbiol. 1, 16070. doi: 10.1038/nmicrobiol.2016.70
Drissi, F., Buffet, S., Raoult, D., Merhej, V. (2015). Common Occurrence of Antibacterial Agents in Human Intestinal Microbiota. Front. Microbiol. 6. doi: 10.3389/fmicb.2015.00441
Evans, C. M., Pratt, C. B., Matheson, M., Vaughan, T. E., Findlow, J., Borrow, R., et al. (2011). Nasopharyngeal Colonization by Neisseria Lactamica and Induction of Protective Immunity Against Neisseria Meningitidis. Clin. Infect. Dis. 52, 70–77. doi: 10.1093/cid/ciq065
Fiore, M. A., Raisman, J. C., Wong, N. H., Hudson, A. O., Wadsworth, C. B. (2020). Exploration of the Neisseria Resistome Reveals Resistance Mechanisms in Commensals That May Be Acquired by N. Gonorrhoeae Through Horizontal Gene Transfer. Antibiotics (Basel). 9 (10), 656. doi: 10.3390/antibiotics9100656
Flaugnatti, N., Le, T. T., Canaan, S., Aschtgen, M. S., Nguyen, V. S., Blangy, S., et al. (2016). A Phospholipase A1 Antibacterial Type VI Secretion Effector Interacts Directly With the C-Terminal Domain of the VgrG Spike Protein for Delivery. Mol. Microbiol. 99, 1099–1118. doi: 10.1111/mmi.13292
Flynn, J., Mcentegart, M. G. (1972). Bacteriocins From Neisseria Gonorrhoeae and Their Possible Role in Epidemiological Studies. J. Clin. Pathol. 25, 60–61. doi: 10.1136/jcp.25.1.60
Fredricks, D. N., Fiedler, T. L., Marrazzo, J. M. (2005). Molecular Identification of Bacteria Associated With Bacterial Vaginosis. N. Engl. J. Med. 353, 1899–1911. doi: 10.1056/NEJMoa043802
García-Bayona, L., Comstock, L. E. (2018). Bacterial Antagonism in Host-Associated Microbial Communities. Science 361 (6408), eaat2456. doi: 10.1126/science.aat2456
Gelosa, L. (1981). [Frequency and Characterization of "Neisseria Lactamica" Among the Population of Milan Italy (Author's Transl)]. Ann. Sclavo. 23, 275–281.
Glitza, I. C., Ehrhard, I., Müller-Pebody, B., Reintjes, R., Breuer, T., Ammon, A., et al. (2008). Longitudinal Study of Meningococcal Carrier Rates in Teenagers. Int. J. Hyg. Environ. Health 211, 263–272. doi: 10.1016/j.ijheh.2007.05.006
Glover, J. A. (1918). The Cerebro-Spinal Fever Epidemic of 1917 at X Depôt. J. Hyg. (Lond). 17 (2-3), 350–365. doi: 10.1017/s002217240000718x
Gold, R., Goldschneider, I., Lepow, M. L., Draper, T. F., Randolph, M. (1978). Carriage of Neisseria Meningitidis and Neisseria Lactamica in Infants and Children. J. Infect. Dis. 137, 112–121. doi: 10.1093/infdis/137.2.112
Gorringe, A. R., Taylor, S., Brookes, C., Matheson, M., Finney, M., Kerr, M., et al. (2009). Phase I Safety and Immunogenicity Study of a Candidate Meningococcal Disease Vaccine Based on Neisseria Lactamica Outer Membrane Vesicles. Clin. Vaccine Immunol. 16, 1113–1120. doi: 10.1128/CVI.00118-09
Graver, M. A., Wade, J. J. (2011). The Role of Acidification in the Inhibition of Neisseria Gonorrhoeae by Vaginal Lactobacilli During Anaerobic Growth. Ann. Clin. Microbiol. Antimicrob. 10, 8. doi: 10.1186/1476-0711-10-8
Guérin, J., Bigot, S., Schneider, R., Buchanan, S. K., Jacob-Dubuisson, F. (2017). Two-Partner Secretion: Combining Efficiency and Simplicity in the Secretion of Large Proteins for Bacteria-Host and Bacteria-Bacteria Interactions. Front. Cell. Infect. Microbiol. 7, 148. doi: 10.3389/fcimb.2017.00148
Günther, P., Quentin, D., Ahmad, S., Sachar, K., Gatsogiannis, C., Whitney, J. C., et al. (2022). Structure of a Bacterial Rhs Effector Exported by the Type VI Secretion System. PLoS Pathog. 18, e1010182. doi: 10.1371/journal.ppat.1010182
Hamilton, H. L., Domínguez, N. M., Schwartz, K. J., Hackett, K. T., Dillard, J. P. (2005). Neisseria Gonorrhoeae Secretes Chromosomal DNA via a Novel Type IV Secretion System. Mol. Microbiol. 55, 1704–1721. doi: 10.1111/j.1365-2958.2005.04521.x
Heilbronner, S., Krismer, B., Brötz-Oesterhelt, H., Peschel, A. (2021). The Microbiome-Shaping Roles of Bacteriocins. Nat. Rev. Microbiol. 19, 726–739. doi: 10.1038/s41579-021-00569-w
Higashi, D. L., Biais, N., Weyand, N. J., Agellon, A., Sisko, J. L., Brown, L. M., et al. (2011). N. Elongata Produces Type IV Pili That Mediate Interspecies Gene Transfer With N. Gonorrhoeae. PLoS One 6 (6), e21373. doi: 10.1371/journal.pone.0021373
Hill, C. W., Sandt, C. H., Vlazny, D. A. (1994). Rhs Elements of Escherichia Coli: A Family of Genetic Composites Each Encoding a Large Mosaic Protein. Mol. Microbiol. 12, 865–871. doi: 10.1111/j.1365-2958.1994.tb01074.x
Hobbs, M. M., Sparling, P. F., Cohen, M. S., Shafer, W. M., Deal, C. D., Jerse, A. E. (2011). Experimental Gonococcal Infection in Male Volunteers: Cumulative Experience With Neisseria Gonorrhoeae Strains FA1090 and MS11mkC. Front. Microbiol. 2, 123–123. doi: 10.3389/fmicb.2011.00123
Holten, E., Bratlid, D., Bøvre, K. (1978). Carriage of Neisseria Meningitidis in a Semi-Isolated Arctic Community. Scand. J. Infect. Dis. 10, 36–40. doi: 10.3109/inf.1978.10.issue-1.08
Humbert, M. V., Christodoulides, M. (2019). Atypical, Yet Not Infrequent, Infections With Neisseria Species. Pathogens 9 (1), 10. doi: 10.3390/pathogens9010010
Jamet, A., Jousset, A. B., Euphrasie, D., Mukorako, P., Boucharlat, A., Ducousso, A., et al. (2015). A New Family of Secreted Toxins in Pathogenic Neisseria Species. PLoS Pathog. 11, e1004592. doi: 10.1371/journal.ppat.1004592
Johnson, A. P. (1983). The Pathogenic Potential of Commensal Species of Neisseria. J. Clin. Pathol. 36, 213–223. doi: 10.1136/jcp.36.2.213
Johswich, K. O., Mccaw, S. E., Strobel, L., Frosch, M., Gray-Owen, S. D. (2015). Sterilizing Immunity Elicited by Neisseria Meningitidis Carriage Shows Broader Protection Than Predicted by Serum Antibody Cross-Reactivity in CEACAM1-Humanized Mice. Infect. Immun. 83, 354–363. doi: 10.1128/IAI.02495-14
Jolley, K. A., Bray, J. E., Maiden, M. C. J. (2018). Open-Access Bacterial Population Genomics: BIGSdb Software, the PubMLST.org Website and Their Applications. Wellcome. Open Res. 3, 124. doi: 10.12688/wellcomeopenres.14826.1
Jurėnas, D., Rosa, L. T., Rey, M., Chamot-Rooke, J., Fronzes, R., Cascales, E. (2021). Mounting, Structure and Autocleavage of a Type VI Secretion-Associated Rhs Polymorphic Toxin. Nat. Commun. 12, 6998. doi: 10.1038/s41467-021-27388-0
Kim, W. J., Higashi, D., Goytia, M., Rendón, M. A., Pilligua-Lucas, M., Bronnimann, M., et al. (2019). Commensal Neisseria Kill Neisseria Gonorrhoeae Through a DNA-Dependent Mechanism. Cell Host Microbe 26, 228–239.e8. doi: 10.1016/j.chom.2019.07.003
Kingsbury, D. T. (1966). Bacteriocin Production by Strains of Neisseria Meningitidis. J. Bacteriol. 91, 1696–1699. doi: 10.1128/jb.91.5.1696-1699.1966
Kleanthous, C. (2010). Swimming Against the Tide: Progress and Challenges in Our Understanding of Colicin Translocation. Nat. Rev. Microbiol. 8, 843–848. doi: 10.1038/nrmicro2454
Knapp, J. S., Falkow, S., Holmes, K. K. (1975). Reevaluation of Bacteriocinogeny in Neisseria Gonorrhoeae. J. Clin. Pathol. 28, 274–278. doi: 10.1136/jcp.28.4.274
Knapp, J. S., Hook, E. W., 3rd (1988). Prevalence and Persistence of Neisseria Cinerea and Other Neisseria Spp. In Adults. J. Clin. Microbiol. 26, 896–900. doi: 10.1128/jcm.26.5.896-900.1988
Krasauskas, R., Skerniškytė, J., Martinkus, J., Armalytė, J., Sužiedėlienė, E. (2020). Capsule Protects Acinetobacter Baumannii From Inter-Bacterial Competition Mediated by CdiA Toxin. Front. Microbiol. 11, 1493. doi: 10.3389/fmicb.2020.01493
Kristiansen, P. A., Diomandé, F., Ouédraogo, R., Sanou, I., Sangaré, L., Ouédraogo, A. S., et al. (2012). Carriage of Neisseria Lactamica in 1- to 29-Year-Old People in Burkina Faso: Epidemiology and Molecular Characterization. J. Clin. Microbiol. 50, 4020–4027. doi: 10.1128/JCM.01717-12
Kumpitsch, C., Koskinen, K., Schöpf, V., Moissl-Eichinger, C. (2019). The Microbiome of the Upper Respiratory Tract in Health and Disease. BMC Biol. 17, 87. doi: 10.1186/s12915-019-0703-z
Łaniewski, P., Gomez, A., Hire, G., So, M., Herbst-Kralovetz, M. M., Bäumler, A. J. (2017). Human Three-Dimensional Endometrial Epithelial Cell Model To Study Host Interactions With Vaginal Bacteria and Neisseria Gonorrhoeae. Infect. Immunity. 85 (3), e01049–e01016. doi: 10.1128/IAI.01049-16
Lappann, M., Claus, H., Van Alen, T., Harmsen, M., Elias, J., Molin, S., et al. (2010). A Dual Role of Extracellular DNA During Biofilm Formation of Neisseria Meningitidis. Mol. Microbiol. 75, 1355–1371. doi: 10.1111/j.1365-2958.2010.07054.x
Laver, J. R., Gbesemete, D., Dale, A. P., Pounce, Z. C., Webb, C. N., Roche, E. F., et al. (2021). A Recombinant Commensal Bacteria Elicits Heterologous Antigen-Specific Immune Responses During Pharyngeal Carriage. Sci. Transl. Med. 13 (601), eabe8573. doi: 10.1126/scitranslmed.abe8573
Lawton, W. D., Bellinger, M. A., Schling, M., Gaafar, H. A. (1976). Bacteriocin Production by Neisseria Gonorrhoeae. Antimicrob. Agents Chemother. 10, 417–420. doi: 10.1128/AAC.10.3.417
Leiman, P. G., Basler, M., Ramagopal, U. A., Bonanno, J. B., Sauder, J. M., Pukatzki, S., et al. (2009). Type VI Secretion Apparatus and Phage Tail-Associated Protein Complexes Share a Common Evolutionary Origin. Proc. Natl. Acad. Sci. U.S.A. 106, 4154–4159. doi: 10.1073/pnas.0813360106
Liguori, A., Malito, E., Lo Surdo, P., Fagnocchi, L., Cantini, F., Haag, A. F., et al. (2016). Molecular Basis of Ligand-Dependent Regulation of NadR, the Transcriptional Repressor of Meningococcal Virulence Factor NadA. PLoS Pathog. 12, e1005557. doi: 10.1371/journal.ppat.1005557
Liu, G., Tang, C. M., Exley, R. M. (2015). Non-Pathogenic Neisseria: Members of an Abundant, Multi-Habitat, Diverse Genus. Microbiol. (Reading). 161, 1297–1312. doi: 10.1099/mic.0.000086
Lynch, S. V., Pedersen, O. (2016). The Human Intestinal Microbiome in Health and Disease. New Engl. J. Med. 375, 2369–2379. doi: 10.1056/NEJMra1600266
Maclennan, J., Kafatos, G., Neal, K., Andrews, N., Cameron, J. C., Roberts, R., et al. (2006). Social Behavior and Meningococcal Carriage in British Teenagers. Emerg. Infect. Dis. 12, 950–957. doi: 10.3201/eid1206.051297
Ma, J., Pan, Z., Huang, J., Sun, M., Lu, C., Yao, H. (2017). The Hcp Proteins Fused With Diverse Extended-Toxin Domains Represent a Novel Pattern of Antibacterial Effectors in Type VI Secretion Systems. Virulence 8, 1189–1202. doi: 10.1080/21505594.2017.1279374
Ma, M., Powell, D. A., Weyand, N. J., Rhodes, K. A., Rendón, M. A., Frelinger, J. A., et al. (2018). A Natural Mouse Model for Neisseria Colonization. Infect. Immun. 86, e00839–e00817. doi: 10.1128/IAI.00839-17
Mariano, G., Trunk, K., Williams, D. J., Monlezun, L., Strahl, H., Pitt, S. J., et al. (2019). A Family of Type VI Secretion System Effector Proteins That Form Ion-Selective Pores. Nat. Commun. 10, 5484. doi: 10.1038/s41467-019-13439-0
Marri, P. R., Paniscus, M., Weyand, N. J., Rendón, M. A., Calton, C. M., Hernández, D. R., et al. (2010). Genome Sequencing Reveals Widespread Virulence Gene Exchange Among Human Neisseria Species. PLoS One 5, e11835. doi: 10.1371/journal.pone.0011835
Monjarás Feria, J., Valvano, M. A. (2020). An Overview of Anti-Eukaryotic T6SS Effectors. Front. Cell. Infect. Microbiol. 10. doi: 10.3389/fcimb.2020.584751
Nadel, S., Ninis, N. (2018). Invasive Meningococcal Disease in the Vaccine Era. Front. Pediatr. 6. doi: 10.3389/fped.2018.00321
Neal, K. R., Nguyen-Van-Tam, J. S., Jeffrey, N., Slack, R. C., Madeley, R. J., Ait-Tahar, K., et al. (2000). Changing Carriage Rate of Neisseria Meningitidis Among University Students During the First Week of Term: Cross Sectional Study. Bmj 320, 846–849. doi: 10.1136/bmj.320.7238.846
Neil, R. B., Apicella, M. A. (2009). Role of HrpA in Biofilm Formation of Neisseria Meningitidis and Regulation of the hrpBAS Transcripts. Infect. Immun. 77, 2285–2293. doi: 10.1128/IAI.01502-08
Oldewurtel, E. R., Kouzel, N., Dewenter, L., Henseler, K., Maier, B. (2015). Differential Interaction Forces Govern Bacterial Sorting in Early Biofilms. Elife 4, e10811. doi: 10.7554/eLife.10811.025
Peters, R. P. H., Verweij, S. P., Nijsten, N., Ouburg, S., Mutsaers, J., Jansen, C. L., et al. (2011). Evaluation of Sexual History-Based Screening of Anatomic Sites for Chlamydia Trachomatis and Neisseria Gonorrhoeae Infection in Men Having Sex With Men in Routine Practice. BMC Infect. Dis. 11, 203. doi: 10.1186/1471-2334-11-203
Pissaridou, P., Allsopp, L. P., Wettstadt, S., Howard, S. A., Mavridou, D. A. I., Filloux, A. (2018). The Pseudomonas Aeruginosa T6SS-VgrG1b Spike is Topped by a PAAR Protein Eliciting DNA Damage to Bacterial Competitors. Proc. Natl. Acad. Sci. U.S.A. 115, 12519–12524. doi: 10.1073/pnas.1814181115
Pönisch, W., Eckenrode, K. B., Alzurqa, K., Nasrollahi, H., Weber, C., Zaburdaev, V., et al. (2018). Pili Mediated Intercellular Forces Shape Heterogeneous Bacterial Microcolonies Prior to Multicellular Differentiation. Sci. Rep. 8, 16567. doi: 10.1038/s41598-018-34754-4
Pujol, C., Eugène, E., De Saint Martin, L., Nassif, X. (1997). Interaction of Neisseria Meningitidis With a Polarized Monolayer of Epithelial Cells. Infect. Immun. 65, 4836–4842. doi: 10.1128/iai.65.11.4836-4842.1997
Pukatzki, S., Ma, A. T., Revel, A. T., Sturtevant, D., Mekalanos, J. J. (2007). Type VI Secretion System Translocates a Phage Tail Spike-Like Protein Into Target Cells Where it Cross-Links Actin. Proc. Natl. Acad. Sci. U.S.A. 104, 15508–15513. doi: 10.1073/pnas.0706532104
Ramos-Sevillano, E., Wade, W. G., Mann, A., Gilbert, A., Lambkin-Williams, R., Killingley, B., et al. (2018). The Effect of Influenza Virus on the Human Oropharyngeal Microbiome. Clin. Infect. Dis. 68, 1993–2002. doi: 10.1093/cid/ciy821
Ravel, J., Gajer, P., Abdo, Z., Schneider, G. M., Koenig, S. S., Mcculle, S. L., et al. (2011). Vaginal Microbiome of Reproductive-Age Women. Proc. Natl. Acad. Sci. U.S.A. 108 Suppl 1, 4680–4687. doi: 10.1073/pnas.1002611107
Rebuffat, S. (2022). Ribosomally Synthesized Peptides, Foreground Players in Microbial Interactions: Recent Developments and Unanswered Questions. Nat. Prod. Rep. 39, 273–310. doi: 10.1039/D1NP00052G
Ruhe, Z. C., Low, D. A., Hayes, C. S. (2020). Polymorphic Toxins and Their Immunity Proteins: Diversity, Evolution, and Mechanisms of Delivery. Annu. Rev. Microbiol. 74, 497–520. doi: 10.1146/annurev-micro-020518-115638
Ruhe, Z. C., Subramanian, P., Song, K., Nguyen, J. Y., Stevens, T. A., Low, D. A., et al. (2018). Programmed Secretion Arrest and Receptor-Triggered Toxin Export During Antibacterial Contact-Dependent Growth Inhibition. Cell 175, 921–933.e14. doi: 10.1016/j.cell.2018.10.033
Saez-Nieto, J. A., Dominguez, J. R., Monton, J. L., Cristobal, P., Fenoll, A., Vazquez, J., et al. (1985). Carriage of Neisseria Meningitidis and Neisseria Lactamica in a School Population During an Epidemic Period in Spain. J. Hyg. (Lond). 94, 279–288. doi: 10.1017/S0022172400061507
Sáez Nieto, J. A., Marcos, C., Vindel, A. (1998). Multicolonization of Human Nasopharynx Due to Neisseria Spp. Int. Microbiol. 1, 59–63.
Sánchez-Busó, L., Golparian, D., Parkhill, J., Unemo, M., Harris, S. R. (2019). Genetic Variation Regulates the Activation and Specificity of Restriction-Modification Systems in Neisseria Gonorrhoeae. Sci. Rep. 9, 14685. doi: 10.1038/s41598-019-51102-2
Sassone-Corsi, M., Raffatellu, M. (2015). No Vacancy: How Beneficial Microbes Cooperate With Immunity To Provide Colonization Resistance to Pathogens. J. Immunol. 194, 4081–4087. doi: 10.4049/jimmunol.1403169
Schmitt, C., Turner, D., Boesl, M., Abele, M., Frosch, M., Kurzai, O. (2007). A Functional Two-Partner Secretion System Contributes to Adhesion of Neisseria Meningitidis to Epithelial Cells. J. Bacteriol. 189, 7968–7976. doi: 10.1128/JB.00851-07
Scholl, D. (2017). Phage Tail–Like Bacteriocins. Annu. Rev. Virol. 4, 453–467. doi: 10.1146/annurev-virology-101416-041632
Sheikhi, R., Amin, M., Rostami, S., Shoja, S., Ebrahimi, N. (2015). Oropharyngeal Colonization With Neisseria Lactamica, Other Nonpathogenic Neisseria Species and Moraxella Catarrhalis Among Young Healthy Children in Ahvaz, Iran. Jundishapur. J. Microbiol. 8, e14813. doi: 10.5812/jjm.14813
Simmons, G., Martin, D., Stewart, J., Bremner, D. (2000). Carriage of N. Lactamica in a Population at High Risk of Meningococcal Disease. Epidemiol. Infect. 125, 99–104. doi: 10.1017/S095026889900415X
Soltani, S., Hammami, R., Cotter, P. D., Rebuffat, S., Said, L. B., Gaudreau, H., et al. (2020). Bacteriocins as a New Generation of Antimicrobials: Toxicity Aspects and Regulations. FEMS Microbiol. Rev. 45 (1), fuaa039. doi: 10.1093/femsre/fuaa039
So, M., Rendón, M. A. (2019). Tribal Warfare: Commensal Neisseria Kill Pathogen Neisseria Gonorrhoeae Using its DNA. Microb. Cell. 6, 544–546. doi: 10.15698/mic2019.12.701
Spratt, B. G., Bowler, L. D., Zhang, Q. Y., Zhou, J., Smith, J. M. (1992). Role of Interspecies Transfer of Chromosomal Genes in the Evolution of Penicillin Resistance in Pathogenic and Commensal Neisseria Species. J. Mol. Evol. 34, 115–125. doi: 10.1007/BF00182388
Talà, A., Progida, C., De Stefano, M., Cogli, L., Spinosa, M. R., Bucci, C., et al. (2008). The HrpB-HrpA Two-Partner Secretion System is Essential for Intracellular Survival of Neisseria Meningitidis. Cell Microbiol. 10, 2461–2482. doi: 10.1111/j.1462-5822.2008.01222.x
Tang, J. Y., Bullen, N. P., Ahmad, S., Whitney, J. C. (2018). Diverse NADase Effector Families Mediate Interbacterial Antagonism via the Type VI Secretion System. J. Biol. Chem. 293, 1504–1514. doi: 10.1074/jbc.RA117.000178
Telfer Brunton, W. A., Young, H., Fraser, D. R. (1980). Isolation of Neisseria Lactamica From the Female Genital Tract. A Case Report. Br. J. Vener. Dis. 56 (5), 325–326. doi: 10.1136/sti.56.5.325
Telhig, S., Ben Said, L., Zirah, S., Fliss, I., Rebuffat, S. (2020). Bacteriocins to Thwart Bacterial Resistance in Gram Negative Bacteria. Front. Microbiol. 11, 586433. doi: 10.3389/fmicb.2020.586433
Tzeng, Y. L., Bazan, J. A., Turner, A. N., Wang, X., Retchless, A. C., Read, T. D., et al. (2017). Emergence of a New Neisseria Meningitidis Clonal Complex 11 Lineage 11.2 Clade as an Effective Urogenital Pathogen. Proc. Natl. Acad. Sci. U.S.A. 114, 4237–4242. doi: 10.1073/pnas.1620971114
Tzeng, Y. L., Thomas, J., Stephens, D. S. (2016). Regulation of Capsule in Neisseria Meningitidis. Crit. Rev. Microbiol. 42 (5), 759–772. doi: 10.3109/1040841X.2015.1022507
Unemo, M., Seifert, H. S., Hook, E. W., Hawkes, S., Ndowa, F., Dillon, J.-A. R. (2019). Gonorrhoea. Nat. Rev. Dis. Primers 5, 79. doi: 10.1038/s41572-019-0128-6
Unterweger, D., Kostiuk, B., Ötjengerdes, R., Wilton, A., Diaz-Satizabal, L., Pukatzki, S. (2015). Chimeric Adaptor Proteins Translocate Diverse Type VI Secretion System Effectors in Vibrio Cholerae. EMBO J. 34, 2198–2210. doi: 10.15252/embj.201591163
Van Heel, A. J., De Jong, A., Song, C., Viel, J. H., Kok, J., Kuipers, O. P. (2018). BAGEL4: A User-Friendly Web Server to Thoroughly Mine RiPPs and Bacteriocins. Nucleic Acids Res. 46 (W1), W278–w281. doi: 10.1093/nar/gky383
Van Ulsen, P., Rutten, L., Feller, M., Tommassen, J., van der Ende, A. (2008). Two-Partner Secretion Systems of Neisseria Meningitidis Associated With Invasive Clonal Complexes. Infect. Immun. 76, 4649–4658. doi: 10.1128/IAI.00393-08
Volk, J., Kraus, S. J. (1973). Asymptomatic Meningococcal Urethritis. Possible Protective Value Against Gonococcal Infection by Bacteriocin Production. Br. J. Vener. Dis. 49, 511–512. doi: 10.1136/sti.49.6.511
Wadsworth, C. B., Arnold, B. J., Sater, M. R. A., Grad, Y. H. (2018). Azithromycin Resistance Through Interspecific Acquisition of an Epistasis-Dependent Efflux Pump Component and Transcriptional Regulator in Neisseria Gonorrhoeae. mBio 9 (4), e01419-18. doi: 10.1128/mBio.01419-18
Walsh, C. J., Guinane, C. M., Hill, C., Ross, R. P., O’toole, P. W., Cotter, P. D. (2015). In Silico Identification of Bacteriocin Gene Clusters in the Gastrointestinal Tract, Based on the Human Microbiome Project’s Reference Genome Database. BMC Microbiol. 15, 183. doi: 10.1186/s12866-015-0515-4
Walstad, D. L., Reitz, R. C., Sparling, P. F. (1974). Growth Inhibition Among Strains of Neisseria Gonorrhoeae Due to Production of Inhibitory Free Fatty Acids and Lysophosphatidylethanolamine: Absence of Bacteriocins. Infect. Immun. 10, 481–488. doi: 10.1128/iai.10.3.481-488.1974
Weyand, N. J., Wertheimer, A. M., Hobbs, T. R., Sisko, J. L., Taku, N. A., Gregston, L. D., et al. (2013). Neisseria Infection of Rhesus Macaques as a Model to Study Colonization, Transmission, Persistence, and Horizontal Gene Transfer. Proc. Natl. Acad. Sci. U.S.A. 110, 3059–3064. doi: 10.1073/pnas.1217420110
Whitney, J. C., Beck, C. M., Goo, Y. A., Russell, A. B., Harding, B. N., De Leon, J. A., et al. (2014). Genetically Distinct Pathways Guide Effector Export Through the Type VI Secretion System. Mol. Microbiol. 92, 529–542. doi: 10.1111/mmi.12571
Whitney, J. C., Chou, S., Russell, A. B., Biboy, J., Gardiner, T. E., Ferrin, M. A., et al. (2013). Identification, Structure, and Function of a Novel Type VI Secretion Peptidoglycan Glycoside Hydrolase Effector-Immunity Pair. J. Biol. Chem. 288, 26616–26624. doi: 10.1074/jbc.M113.488320
Wiesner, P. J., Tronca, E., Bonin, P., Pedersen, A. H. B., Holmes, K. K. (1973). Clinical Spectrum of Pharyngeal Gonococcal Infection. New Engl. J. Med. 288, 181–185. doi: 10.1056/NEJM197301252880404
Wilkinson, A. E. (1952). Occurrence of Neisseria Other Than the Gonococcus in the Genital Tract. Br. J. Venereal. Dis. 28, 24–27. doi: 10.1136/sti.28.1.24
Yi, K., Stephens, D. S., Stojiljkovic, I. (2003). Development and Evaluation of an Improved Mouse Model of Meningococcal Colonization. Infect. Immun. 71, 1849–1855. doi: 10.1128/IAI.71.4.1849-1855.2003
Zhang, D., De Souza, R. F., Anantharaman, V., Iyer, L. M., Aravind, L. (2012). Polymorphic Toxin Systems: Comprehensive Characterization of Trafficking Modes, Processing, Mechanisms of Action, Immunity and Ecology Using Comparative Genomics. Biol. Direct. 7, 18. doi: 10.1186/1745-6150-7-18
Zhang, D., iyer, L. M., Aravind, L. (2011). A Novel Immunity System for Bacterial Nucleic Acid Degrading Toxins and its Recruitment in Various Eukaryotic and DNA Viral Systems. Nucleic Acids Res. 39, 4532–4552. doi: 10.1093/nar/gkr036
Zheng, J., Gänzle, M. G., Lin, X. B., Ruan, L., Sun, M. (2015). Diversity and Dynamics of Bacteriocins From Human Microbiome. Environ. Microbiol. 17, 2133–2143. doi: 10.1111/1462-2920.12662
Keywords: Neisseria, pathogen, commensal, antagonism, growth inhibition
Citation: Baerentsen R, Tang CM and Exley RM (2022) Et tu, Neisseria? Conflicts of Interest Between Neisseria Species. Front. Cell. Infect. Microbiol. 12:913292. doi: 10.3389/fcimb.2022.913292
Received: 05 April 2022; Accepted: 27 May 2022;
Published: 24 June 2022.
Edited by:
Jesús A. Arenas, University of Zaragoza, SpainReviewed by:
Wenxia Song, University of Maryland, College Park, United StatesCopyright © 2022 Baerentsen, Tang and Exley. This is an open-access article distributed under the terms of the Creative Commons Attribution License (CC BY). The use, distribution or reproduction in other forums is permitted, provided the original author(s) and the copyright owner(s) are credited and that the original publication in this journal is cited, in accordance with accepted academic practice. No use, distribution or reproduction is permitted which does not comply with these terms.
*Correspondence: Rachel M. Exley, cmFjaGVsLmV4bGV5MDJAcGF0aC5veC5hYy51aw==
Disclaimer: All claims expressed in this article are solely those of the authors and do not necessarily represent those of their affiliated organizations, or those of the publisher, the editors and the reviewers. Any product that may be evaluated in this article or claim that may be made by its manufacturer is not guaranteed or endorsed by the publisher.
Research integrity at Frontiers
Learn more about the work of our research integrity team to safeguard the quality of each article we publish.