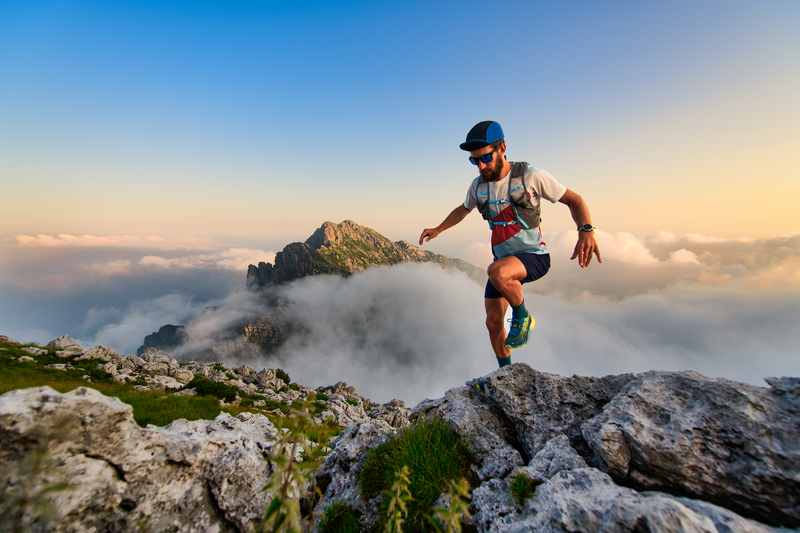
95% of researchers rate our articles as excellent or good
Learn more about the work of our research integrity team to safeguard the quality of each article we publish.
Find out more
REVIEW article
Front. Cell. Infect. Microbiol. , 31 May 2022
Sec. Parasite and Host
Volume 12 - 2022 | https://doi.org/10.3389/fcimb.2022.908241
This article is part of the Research Topic Disruptive Technologies for the Study of Host-Pathogen Interactions View all 8 articles
Forces and mechanical properties of cells and tissues set constraints on biological functions, and are key determinants of human physiology. Changes in cell mechanics may arise from disease, or directly contribute to pathogenesis. Malaria gives many striking examples. Plasmodium parasites, the causative agents of malaria, are single-celled organisms that cannot survive outside their hosts; thus, thost-pathogen interactions are fundamental for parasite’s biological success and to the host response to infection. These interactions are often combinations of biochemical and mechanical factors, but most research focuses on the molecular side. However, Plasmodium infection of human red blood cells leads to changes in their mechanical properties, which has a crucial impact on disease pathogenesis because of the interaction of infected red blood cells with other human tissues through various adhesion mechanisms, which can be probed and modelled with biophysical techniques. Recently, natural polymorphisms affecting red blood cell biomechanics have also been shown to protect human populations, highlighting the potential of understanding biomechanical factors to inform future vaccines and drug development. Here we review biophysical techniques that have revealed new aspects of Plasmodium falciparum invasion of red blood cells and cytoadhesion of infected cells to the host vasculature. These mechanisms occur differently across Plasmodium species and are linked to malaria pathogenesis. We highlight promising techniques from the fields of bioengineering, immunomechanics, and soft matter physics that could be beneficial for studying malaria. Some approaches might also be applied to other phases of the malaria lifecycle and to apicomplexan infections with complex host-pathogen interactions.
Malaria is an infectious disease caused by multiple species of Plasmodium protozoan parasites of the phylum Apicomplexa. Malaria killed more than half a million people in 2020 (World Malaria Report, 2021), with Plasmodium falciparum causing the overwhelming majority of deaths. Although Plasmodium research has typically focussed on the genetic and biochemical determinants of disease, recent advances in imaging and mechanobiology offer the opportunity to explore how mechanics and the environment contribute to various aspects of the Plasmodium life cycle and pathogenesis, in particular the blood stage reported in Figure 1. Multiple biophysical tools are now being applied to understanding parasite-host interactions in malaria, particularly the mechanisms of highly dynamic processes such as parasite-red blood cell (RBC) invasion, and cytoadhesion, where the infected red blood cell (iRBC) interacts with the microvasculature and other human cells; both invasion and cytoadhesion play a critical role in severe malaria pathogenesis.
Figure 1 The blood stage of Plasmodium falciparum. Life cycles of Apicomplexa involve asexual and sexual reproduction stages in different hosts. Plasmodium’s asexual replication in humans occurs in the liver and bloodstream while the sexual stages occur in the mosquito. Parasites are injected into the human skin through the bite of an infected female Anopheles mosquito (Frevert et al., 2005; Amino et al., 2006); they first travel to the liver (Menard et al., 2013), where they proliferate into merozoites, before being released into the blood stream. Merozoites invade circulating RBCs initiating the blood stage (Cowman et al., 2017). While invasion typically occurs in less than a minute, within the RBC the parasite undergoes maturation in approximately 48 hours from a ring-stage to a trophozoite by digesting haemoglobin before starting nuclear divisions into daughter merozoites as schizont. At this point, the iRBCs rupture releasing up to 30 daughter merozoites (Rudlaff et al., 2020) producing an exponential growth of parasites inside the host that causes all the symptoms of the disease. To avoid clearance by the spleen, trophozoites and schizonts of P. falciparum adhere to the endothelium and to other uninfected RBCs (rosettes) and sequester in the vasculature, leading to severe complications associated with this malaria parasite such as cerebral and placental malaria. In each cycle, 5-10% of parasites develop into the sexual forms and are transmitted to the mosquito vector to complete the life cycle (Frischknecht and Matuschewski, 2017). Image made using ©BioRender (https://biorender.com).
Progress in live imaging has been at the heart of recent discoveries: improvements on real-time and time-lapse microscopy speed and resolution have been crucial in imaging Plasmodium parasites in in vitro and in vivo experimental settings. Likewise, many biophysical methods have been applied to quantitatively characterise the mechanical properties and the adhesion force of healthy RBCs and Plasmodium-infected RBCs in bulk and at single-cell level [refer to (Depond et al., 2019)]: all terms and the relative technologies presented in this work are summarised in the Glossary Table 1. Techniques to probe and measure forces at the cell scale include micropipette aspiration (Mohandas et al., 1984), atomic force microscopy (Sinha et al., 2015), and optical tweezers (Crick et al., 2014). Recently, membrane flickering spectroscopy has been used as a powerful tool to elucidate the role of RBC mechanics in disease protection (Kariuki et al., 2020). To address the intricacies of parasite and human biology, sophisticated microfluidic devices have been engineered to tune physical and biological parameters in well-controlled reproducible assays while maintaining aspects of physiological relevance, and create in vitro models that more accurately reproduce in vivo tissue microenvironments.
These concepts fit within the broader efforts in “mechanobiology”, i.e. the effort to generally understand the relationship between a cell and its surroundings. Here we use this term to specifically refer to how Plasmodium parasites respond to or cause changes in host cell mechanics, physical forces, and substrate structure, and if mechanical pathways influence the infected cells or host tissues.
In this review, we focus on disruptive technologies that have been used to investigate the physical traits of P. falciparum-human interactions during invasion (Section 1) and cytoadhesion (Section 2), where such technologies have generated paradigm shifts in our knowledge of malaria and important insights into potential new intervention strategies. Section 3 will describe the biomechanical determinants of invasion and cytoadhesion in other species of Plasmodium. This review takes a problem-oriented approach, to show how specific research questions in malaria have been addressed using biophysical techniques, hoping to inform the reader in the importance of biophysics in infection, and inspire to adopt similar approaches to tackle their own research questions.
Malaria clinical symptoms arise during the Plasmodium blood stage, which starts when merozoites recognise and invade human RBCs. The invasion process is essential for parasite survival and replication, and consists of a sequence of mechanical and biochemical events that are precisely timed and tightly regulated. During invasion parasites are extracellular and hence exposed to the antibody mediated immune system, representing a potential target for vaccine and drug development. Advanced microscopy techniques such as cryo-electron tomography (Hanssen et al., 2013), cryo-X-ray tomography (Hanssen et al., 2011), electron microscopy (Kumar et al., 2017), and super-resolution structured illumination microscopy (Riglar et al., 2011) have been crucial to unveil the structure of merozoite organelles involved in invasion, and the molecular mechanisms that determine the irreversible attachment between merozoites and RBCs (Mauritz et al., 2010; Cho et al., 2012). However, these techniques operate with fixed samples providing static snapshots, which is not ideal for a highly dynamic and rapid process such as invasion, which is usually complete in less than a minute. Therefore, a main challenge in the field has been to follow the egress-invasion sequence in real time, and this is typically achieved with live imaging in brightfield, phase contrast, differential interference contrast (DIC), and epifluorescence (Glushakova et al., 2005; Gilson and Crabb, 2009; Crick et al., 2013; Introini et al., 2018a).
Over the last two decades, progress on the video temporal and spatial resolution permitted the recording of the kinetics of merozoite egress and subsequent invasion of RBCs up to hundreds of frames per second with sub-μm resolution, and has been used to assess the effect of antibodies, enzyme inhibitors, and ion signalling on the invasion process (Glushakova et al., 2005; Gilson and Crabb, 2009; Crick et al., 2013; Weiss et al., 2015; Bustamante et al., 2017). The egress was initially described in the literature as an “explosive” phenomenon (Lew, 2005; Mauritz et al., 2010), but Abkarian et al. (Abkarian et al., 2011) revealed a detailed dynamic morphology of the host cell membrane rupture using high-speed video microscopy, as represented in Figure 2. At the instant of schizont rupture, a pore opening in the red cell membrane allows only 1 or 2 merozoites to emerge. Once the pore reaches a critical radius, the host cell membrane rapidly curls outwards to form a circular toroid around the initial opening, and then buckles, undergoing eversion to push out and disperse the rest of the merozoites. Finally, the everted membrane spontaneously forms vesicles (Lew, 2011). This curling-buckling-eversion-vesiculation sequence is completed in about 400-800 ms (Callan-Jones et al., 2012; Braun-Breton and Abkarian, 2016) and resembles, on a shorter time scale, the spontaneous vesiculation of uninfected RBCs found previously by Lew et al. (Lew et al., 1988; Tiffert and Lew, 2014). This similarity may be evidence of the parasite exploiting an intrinsic host cell property and remodelling the cortical cytoskeleton for a rapid response during egress (Kabaso et al., 2010).
Figure 2 Biophysical techniques and methods to probe the mechanics of P. falciparum invasion. The sequence egress, pre-invasion, recoil phase, internalisation and finally echinocytosis is represented with a focus on the biophysical approaches used to measure the mechanical properties of the RBC and the parasite, and their interactions. The technique is underlined, the quantity measured with the relative technique is in italics, and the dashed sentences report the main findings. Image made using ©BioRender (https://biorender.com). LLSM image was taken from Geoghegan et al. (Geoghegan et al., 2021).
The three main phases in which invasion is usually divided i) pre-invasion, ii) recoil phase, and iii) internalisation (Gilson and Crabb, 2009; Groomes et al., 2022) are described in Figure 2, highlighting the biophysical techniques developed to measure both the parasite and the RBC physical quantities at each phase. During pre-invasion, which lasts between 2-50 s, merozoites make reversible contacts with RBCs triggering transient deformations of the cell membrane from the contact site. The merozoite re-positions itself on the surface of the RBC until it is apically aligned, and binds to the RBC using adhesins on the merozoite coat (Tham et al., 2015; Weiss et al., 2016). After a recoil phase where RBC deformations cease and the biconcave shape is restored, the secretory organelles located in the merozoite apex release ligands into the RBC and the merozoite is irreversibly attached (Treeck et al., 2009; Crosnier et al., 2011). The tight junction is then formed, and penetration begins driven by the merozoite actomyosin motor. Merozoite internalisation is frequently, but not always, accompanied by echinocytosis (taking approximately 5-15 minutes (Gilson and Crabb, 2009) where the RBC outer surface curls and forms spikes, before restoring their original biconcave shape with the parasite-ring inside. Transition of RBCs from discocytes to echinocytes results from the expansion of the outer leaflet of the plasma membrane with respect to the inner one (Lim et al., 2002), producing convex structures on the cell surface (echinocytic spicules) to accommodate the extra area, and resulting in increased bending modulus, shear modulus, and surface area (Park et al., 2010) (Table 1). Computational modelling of this invasion processes is a huge and still open challenge since invasion ligand expression is controlled in time and space, and ligand-receptor interaction binding rates are modulated by membrane properties (Mognetti et al., 2019; Hillringhaus et al., 2020).
The latest imaging upgrade to investigate Plasmodium invasion is given by the 4D lattice light-sheet microscopy (LLSM), described by the pioneering work of Chen and colleagues (Chen et al., 2014). LLSM provides volumetric information at hundreds of images per second, and a distinct advantage of this method over the conventional 2D microscopy consists of looking at parasites and RBCs from multiple orientations given by the near isotropic resolution (Geoghegan et al., 2021). Compared to confocal laser scanning microscopy, the thinness of the sheet produces a high axial resolution (the ability to discern between two points at different depth along the optical axis of the imaging objective) and larger field of view, a better signal-to-noise ratio when using multiple fluorescent probes, and less photobleaching. LLSM has succeeded in characterising all stages of invasion quantitatively, including parasite internalisation and parasitophorous vacuole membrane (PVM) formation (Geoghegan et al., 2021) (Figure 2). Despite many shape changes throughout the invasion process, the membrane area and volume of host RBCs were previously thought to remain essentially unchanged (Mauritz et al., 2009; Waldecker et al., 2017). Here the authors showed a stepwise decrease in RBC surface area which coincides with the total sum of surface areas lost for the PVM of each parasite invading the same RBC.
Recent imaging of CRISPR Cas9 engineered parasite lines with fluorescent tags (Lee et al., 2019), has boosted the study of specific ligand-receptor interactions at the molecular level. This has permitted the localisation of apical ligands that take part in the invasion (Treeck et al., 2009), and showed that the invasion proceeds with the wider end of the merozoite leading, rather than the narrower pointed end, highlighting a remarkable motion of the merozoite that until now was mostly overlooked (Yahata et al., 2021). Moreover, machine learning algorithms for image recognition have been employed to automatically detect infected cells and their stage (Davidson et al., 2021).
One challenge for live-cell imaging is the long, repeated exposure to light which affects both the fragile parasites and the RBCs. Phototoxicity induces lysis of the acidic food vacuole of the parasites (Wissing et al., 2002) and damages RBC haemoglobin inducing autofluorescence (Shrirao et al., 2021), making it very difficult to follow the parasites during the entire intraerythrocytic cycle. Using DIC and confocal microscopy, Gruring et al. (Grüring et al., 2011) were able to visualise the 48-hour development of P. falciparum, elucidating the trafficking mechanism of exported proteins to the host membrane and revealing a fascinating morphology of the parasite at the ring stage. Contrary to the familiar features visible with Giemsa staining, rings are not always circular but assume transient amoeboid forms during the first 5-10 hours post invasion. This phenomenon would require further investigations in real time to explain this initial amoeboid cytoplasm.
The initial merozoite-red cell adherence can occur at any point on the merozoite surface due to low-affinity and reversible interactions between Merozoite Surface Proteins (MSPs) and receptors on the red cell membrane - with coat filaments of 20-150 nm length joining the two surfaces (Bannister et al., 1986). Subsequent reorientation and final attachment require Erythrocyte-Binding-Like (EBL), Reticulocyte binding homologous (Rh), and AMA-1 ligands that span the red cell membrane and link to the merozoite cytoskeleton. These adhesive ligands are secreted from the organelles located at the apex of the 1 μm ovoidal merozoite, which was for a long time presented and modelled as being the narrow end, but recently this depiction has been questioned (Yahata et al., 2021). In hindsight, if the parasite is pear-shaped it will rotate to have the less curved side attached to the membrane. This will reduce bending energy, and allow a larger adhesive area per merozoite. The merozoite shape and orientation therefore play a key role in the mechanics of invasion, which needs further investigation.
Beyond mediating attachment, such ligand families can also transduce signals and modify the physical properties of the host cell membrane (described in Table 1). For example, recombinant proteins Rh5 binding to Basigin mediate Ca2+ signal in the RBC triggering phosphorylation of specific proteins and the subsequent rearrangements of the RBC cytoskeleton that facilitate the insertion and anchoring of rhoptry proteins and junction formation (Aniweh et al., 2017), and increases deformability, i.e. decreases the Young’s modulus of RBCs, albeit to a lesser extent than binding of MSPs, Rh4, or EBA175, hence favouring invasion (Sisquella et al., 2017) (Figure 2). EBA175 RII binding to the RBC receptor Glycophorin A (GYPA) induces a reduction in the host cell bending modulus that increases invasion efficiency, by lowering the energy necessary to bend the RBC surface and facilitate merozoite attachment. The possible caveat is that the local concentration of EBA175 (or any other ligand) on the merozoite surface is unknown, so it is hard to predict how well the experimental set up mimics the actual host RBC-merozoite interaction during invasion. These measurements were performed with AFM, and confirmed using flickering spectroscopy (Koch et al., 2017), as described in Figure 2. Flickering spectroscopy is an optical technique that extracts the cell bending modulus (the energy required to bend the membrane) and tension (the resistance of the membrane to stretch) by analysing the plasma membrane thermal fluctuations. By extending this approach into the dynamical domain and measuring the relaxation rates, it is possible to extract the cytosolic viscosity (Helfrich and Servuss, 1984; Monzel and Sengupta, 2016) (example in Figure 2) Flickering experiments served to investigate the biophysical properties of RBCs depending on oxygenation level, cell morphology, hydration state (Yoon et al., 2009), glucose concentration (Tapia et al., 2021) and parasite infection (Davies et al., 2020); as well as exploring the biophysical impact of malaria-protective polymorphisms affecting RBCs (such as hemoglobinopathies S and C (Froühlich et al., 2019), Dantu blood group (Kariuki et al., 2020) and beta-thalassaemia Introini et al., 2022). The advantages of this method to measure deformability over the more traditional tools, as AFM and optical tweezers, are i) the easy set-up where only a good quality camera is required, ii) the non-invasiveness since samples are not directly manoeuvred, and finally iii) the ability to decouple bending modulus and tension, while other techniques yield the effective shear modulus of the composite system (Kariuki et al., 2020) (see Table 1). It is notable that the theoretical assumptions behind this method are valid only for circular-like closed objects like vesicles and RBCs.
But how strong is the attachment between the RBC and the invading merozoite? Crick et al. measured for the first time the detachment force between a merozoite and an RBC using optical tweezers (40 ± 8 pN) (Crick et al., 2014). Within few seconds from egress, a merozoite is brought in contact with nearby RBCs forming a chain of RBC-merozoite-RBC, and the two trapped RBCs are pulled away simultaneously using optical tweezers (sketch in Figure 2). For a given rate of extension, RBC have been shown to behave like linear springs, i.e. they have a well -stiffness. Thus, the detachment force can be calculated knowing this RBC stiffness and observing the maximum elongation of the cells just before detachment. Generally used for mechanical measurements, optical tweezers are a powerful method for positioning μm-size colloids and cells, and therefore optimal for manipulating individual merozoites and RBCs during invasion (Mauritz et al., 2010). The main difficulty of this technique comes from the low yield due to the short viability of free P. falciparum merozoites after egress (< 2 minutes). Only treatments like heparin that block invasion at the early stage show a reduction in the detachment force attesting that the force measured comes from ligands and receptors participating in the pre-invasion phase (Crick et al., 2014). The electrostatic attraction of the positively charged apex of the merozoite towards the negative surface of the RBC measured using AFM with surface potential microscopy (Akaki et al., 2002) adds to the force generated by EBL and Rh ligands, although the exact ligand responsible for the measured force has not been identified yet, as most EBL and Rh ligands mediating attachment have a redundant function (Menard et al., 2013; Weiss et al., 2015) (Figure 2).
A hallmark of invasion videos is the dynamic deformations of the RBC membrane elicited by merozoite adhesion during pre-invasion. These RBC deformations wrap the merozoites with different strength which correlate with successful invasion (Weiss et al., 2015; Kariuki et al., 2020; Geoghegan et al., 2021) and have been categorised visually from weak to strong by using a scale from 0 to 3 (Weiss et al., 2015). Calcium influx from the merozoite to the RBC was hypothesised to trigger deformations to assist parasite reorientation (Lew and Tiffert, 2007), but this has been disproved by using simultaneously bright-field and fluorescence live microscopy, suggesting instead that local membrane deformations might simply be a passive byproduct of parasite adhesion to the RBC (Introini et al., 2018a; Hillringhaus et al., 2020). This view was supported by Dasgupta et al.’s numerical simulations (Dasgupta et al., 2014) showing that merozoite shape and apical adhesion gradient contributed extensively to wrapping, with an indentation force during the early stage of the invagination estimated of 13 pN (Abdalrahman and Franz, 2017). This is in fact what happens when placing spent merozoites close to RBCs using optical tweezers, they in fact had lost the ability to invade 2-3 minutes post-egress but nonetheless adhere to RBCs and still induce transient local membrane deformations (Crick et al., 2013; Crick et al., 2014).
Altogether, these approaches show that biophysical measurements are necessary to understand how the strength of interaction between specific ligands and RBC receptors affects the host cell mechanics.
Not only do merozoite interactions modify the biomechanical properties of the RBC to facilitate parasite entry, but naturally occurring mutations in proteins of RBC membrane can impact the host cell biophysics and confer protection against malaria. Malaria has provided a strong selective force on the human genome, leading to the selection of many polymorphisms that provide some level of protection against severe disease. Given that all the symptoms and pathology of malaria occur during the intraerythrocytic stage, unsurprisingly most of these polymorphisms relate to the structure and function of red blood cells (Kariuki and Williams, 2020). Plasmodium merozoites are less able to invade RBCs with higher membrane rigidity (Mohandas et al., 1984; Chasis et al., 1985), as documented in a plethora of mutations in the RBC membrane associated with protection against malaria (Mohandas et al., 1984; Froühlich et al., 2019). A recent example is the rare Dantu variant, present at appreciable frequency almost only in East Kenya, which is caused by a rearrangement of Glycophorin A and B genes that results in the expression of a hybrid Dantu protein with a GYPA extracellular domain/GYPB tail fusion that does not interact with the RBC cytoskeleton (Malaria Genomic Epidemiology Network., 2015; Leffler et al., 2017; Ndila et al., 2018). By combining real-time imaging with flickering spectroscopy, Kariuki et al. (Kariuki et al., 2020) were able to quantitate for the first time both the biophysical properties of the host cell and the invasion outcome by P. falciparum merozoites, and compare these properties across Dantu genotypes. These data suggested that there is a threshold for RBC membrane tension, above which the chance of successful invasion is significantly reduced. Knowing the membrane tension and bending modulus of RBCs it is possible to calculate the relative wrapping energy, which is higher for both Dantu and heterozygous beta thalassaemia individuals (Introini et al., 2022). Using a mechanically realistic model of a deformable RBC and an attractive potential to mimic the adhesion gradients at the surface of the merozoite, Hillringhaus et al. (Hillringhaus et al., 2019) found that an increased membrane rigidity results in poor merozoite alignment which could explain why several other RBC polymorphisms associated with membrane tension such as Southeast Asian hereditary ovalocytosis (Mohandas et al., 1984) protect people from malaria infection.
Merozoites were often observed laterally attached to Dantu RBCs but seldom poised for invasion, a recurrent feature observed also in invasion attempts of denser RBCs (Tiffert et al., 2005). Invasion is indeed reduced in dehydrated RBCs (hence higher haemoglobin concentration and cytoplasmatic viscosity), and mutations related to RBC hydration also have effects on RBC deformability (e.g., PIEZO1 (Ma et al., 2018) and the ATP2B4 (Timmann et al., 2012; Lessard et al., 2017; Villegas-Mendez et al., 2021) gene that encodes the plasma membrane calcium ATPase 4 responsible for the intracellular calcium level). PIEZO 1 is a mechanosensitive channel that can be activated when RBCs are stretched in the circulation, causing small-dehydration events via brief surges in RBC calcium (Lew and Tiffert, 2017; Rogers and Lew, 2021). An analogous phenomenon may happen during the deformation phase if merozoite contact is sufficient to mechanically activate the PIEZO 1 ion channel. However, studies on the mechanics and susceptibility to invasion of the human gain-of-function PIEZO1 allele variant, E756del, show discordant results (Thye et al., 2022): some did not indicate RBC dehydration or hinder P. falciparum invasion and growth in vitro (Nguetse et al., 2020), others found that cell density and shear stress contribute to lower parasite replication in E765del PIEZO1 blood in vitro (Glushakova et al., 2022). Since the mechanical activation of PIEZO 1 happens when RBCs squeeze within capillaries, future studies under flow might shed light on the role of PIEZO 1 in malaria invasion (see Section 2).
In general, invasion can be explained as a combined effect of passive processes such as merozoite adhesion-driven wrapping (Section 1.2), and active parasite-induced processes such as RBC cytoskeleton remodelling by merozoite ligand injection (Dasgupta et al., 2014) (Section 1.2) and merozoite motor contribution (deformations are inhibited when impairing the parasite motor with cytochalasin D) (Weiss et al., 2015; Geoghegan et al., 2021). Merozoites do not have flagella or cilia but instead use a substrate-dependent locomotion called gliding motility to invade target host cells. The motor complex (glideosome) that powers invasion in Plasmodium merozoites (Baum et al., 2006) is the same that allows Plasmodium sporozoites to traverse tissues, and is also present in tachyzoites of Toxoplasma gondii and conserved across other Apicomplexan parasites including Cryptosporidium (Wetzel et al., 2005) and Babesia (Asada et al., 2012). As a result, this motility mechanism plays a key role in determining the variety of hosts and tissues targeted by these pathogens. Merozoite penetration is driven by the actomyosin motor that drags the tight junction adhesins rearward, thereby creating a traction force that propels the parasite forward into the RBC. The last act is the PVM and host membrane sealing which seems also common among Apicomplexa: Toxoplasma gondii shows a twisting (corkscrew-like) motion (Pavlou et al., 2018) and this is probably alike to the helical motility of Plasmodium merozoites (Blake et al., 2020; Geoghegan et al., 2021).
P. falciparum merozoites were for a long time thought to not display the gliding motility of other stages, but Yahata et al. (Yahata et al., 2021) recently demonstrated that they glide on specific polymer-coated substrates in vitro. Gliding behaviour depends on the substrate coating: the percentage of motile parasites on glass coverslip is very low and merozoites follow a Brownian motion when released, this is possibly the reason why this phenomenon has not been observed before with the same microscopy techniques. Tracking merozoite directions after egress with time-lapse imaging shows that P. falciparum merozoite average gliding speed is 0.6 μm/s and the longest gliding time is 43 s, significantly lower than Plasmodium sporozoites, and Babesia bovis merozoites (Yahata et al., 2021). The short duration of P. falciparum merozoite motility corresponds to the 2-minute viability of merozoites post egress already shown using optical tweezers (Crick et al., 2014), and indicates declining of motor function over time. It is not clear if this motility is actively used during invasion to discriminate between RBCs, for example based on their mechanical properties, and thus direct invasion in selected cells.
It remains experimentally challenging to investigate how physical traits impact P. falciparum invasion due to the short duration of merozoite invasion and the heterogeneity of RBC mechanical properties. Nonetheless, ground-breaking technological improvements are already helping to answer some of the urgent outstanding questions in malaria formulated by Groomes and co-authors (Groomes et al., 2022). Invasion is a balance between parasite and host cell contributions, evolved toward maximal efficient use of parasite force and modulation of the host cell biophysical properties. Merozoite attachment alters the biomechanical properties of the RBC membrane and triggers dramatic surface deformations that are thought to accommodate the parasite for invasion. The mechanism of these large deformation responses remains an open question for future research. A first step forward would be the quantification of the deformation strength based on more standardised physical parameters such as curvature variation and duration, and not only by subjective observation. This can be achieved by continuous advances in imaging technologies (e.g., LLSM) that help reveal novel features of RBC remodelling and clarify concealed mechanisms through previously unachieved resolutions. High-speed video microscopy combined with flickering spectroscopy has played an important role to establish a membrane tension threshold for Plasmodium invasion, and in principle it could be optimised to measure the changes in the biophysical properties of the invaded RBC at every step of merozoite reorientation and internalisation. However, flickering spectroscopy relies on the detection of the entire cell contour, and the drastic changes in the RBC membrane during the pre-invasion phase and echinocytosis limit the use of this technique. Finally, if flickering experiments, from video recording to complete analysis, became fully automated and higher throughput, it could be employed to screen compounds that inhibit P. falciparum invasion by modifying the host cell membrane biomechanics. If feasible, it should be more difficult for Plasmodium parasites to develop resistance to such a host-targeted approach.
An emerging area of research is the development of fluorophores that allow the measurement of forces (Valanciunaite et al., 2020), and they could be in future applied to the study of RBC membrane biophysics or even food vacuole and endoplasmatic reticulum in living parasites. Probes that are sensitive to lipid order (Niko et al., 2016) and “Flippers” (Goujon et al., 2019) that respond to the change of plasma membrane tension by changing their fluorescence lifetime could be applied to measure lipid organisation and tension of RBCs before and during invasion. However, these techniques rely on Fluorescence Lifetime Imaging Microscopy (FLIM) (Deo, 2020) to measure the probe fluorescence decay time that might be slower than invasion, and therefore their use might be limited by the short duration of malaria invasion.
In malaria, optical tweezers were used directly on RBCs attached to a merozoite to obtain the system detachment force, but optical tweezers have been typically used to quantify the stretchability of cells by trapping and pulling beads conjugated to a target membrane. Future lines of research at the interface between soft matter and cell biology could involve biomimetic approaches to investigate host-pathogen interactions, such as the use of DNA-functionalised colloids of micrometre dimension, vesicles or beads coated with proteins (Mognetti et al., 2019). These synthetic systems provide a better control of ligand adhesion rate and diffusion, overcoming challenges of real biological systems like the wide variability of biophysical properties within a single population of RBCs, even from a single donor. The use of engineered parasites in optical tweezer experiments could help dissecting the orientation of each EBL and Rh families and their contribution to the strength measured.
Finally, future studies should explore how host-pathogen interactions change when the invasion occurs in flow. All studies presented so far have been performed in static cultures, but looking at invasion under flow is important because shear forces can alter ligand-receptor interactions and activate mechanosensitive channels in RBCs. It is known that shaking malaria cultures increases the efficiency of invasion, reduces asynchronous development and multiple invasions (multiple merozoites invading the same RBC) (Allen and Kirk, 2010). This is due to a more uniform distribution of metabolites and nutrients among parasites, as well as a more efficient dispersal of the merozoites after egress (Collins et al., 2017). Shaking can even have a profound effect on the invasion phenotype: P. falciparum strains cultured in moving suspension as opposed to static conditions switch from sialic acid-dependent to independent invasion pathways accompanied by upregulation of the corresponding key invasion genes (Awandare et al., 2018; Nyarko et al., 2020). Moving suspension culture may possibly select for parasites with stronger interactions to fasten onto the RBC surface prior to entry, and therefore a thorough study of the hydrodynamic forces at play and how they regulate this switching mechanism will provide insight into the nature of invasion, as occurs in vivo. In this view, microfluidic devices and deformation cytometry (Xavier et al., 2016), a technique that relates cell shape to its stiffness based on the chamber flow rate, would be ideal to systematically control the rheology and physical environment while assessing if the familiar features of static invasion, such as RBC deformations, merozoite reorientation and invasion rate, remain the same in flow settings.
A remarkable feature of human RBCs is their high deformability when traversing small diameter capillaries, thus exposing maximal surface area and minimal distance to tissue cells for optimal gas exchange across capillary walls. The principal techniques employed to investigate the biophysical properties of iRBCs described in Sections 2.1, 2.2 are summarised in Figure 3A. The use of microfluidics has vastly improved our understanding on the mechanical properties of uninfected and iRBC, as well as their interactions with the microvasculature. Microfluidic devices are fabricated by lithography patterning of optically clear flexible polymers, such as poly-dimethylsiloxane (PDMS), and provide control of the size and geometry of microfluidic networks at micrometre resolution (Antia et al., 2008). For example, early studies with microfluidic devices consisting of 2-8 μm microchannel constrictions revealed that P. falciparum-infection prevented iRBC passage through small diameter constrictions. Later, wedged PDMS capillary constrictions were used to determine the smallest diameter a cell can pass through without increasing its surface area or lysing. Overall, this study found that schizonts lose the property of flowing through the narrowest constrictions (Herricks et al., 2009) (Figure 3A). Moreover, Namvar and colleagues demonstrated using similar chips that surface area-to-volume ratio, not cellular viscoelasticity, determines RBC traversal through small capillaries, the opposite is true for obstruction caused by P. falciparum-iRBC (Pf-iRBC) trophozoites, which are a result of changes in viscosity and not surface area-to-volume ratio (Namvar et al., 2021).
Figure 3 Biophysical methods give insights into the mechanics of P. falciparum iRBCs and their cytoadhering features. (A) Optical and mechanical techniques have been employed to investigate the effect of the parasite infection onto the host cell membrane biophysics. (B) Adhesion between the iRBC and the endothelium is studied at the molecular level and using microfluidic devices, the latter give information about the iRBC dynamics and pathogenesis of malaria by reproducing the physiological in vivo situation. The technique is underlined, the quantity measured with the relative technique is in italics, and the dashed sentences report the main results achieved. Image made using ©BioRender (https://biorender.com).
Late-stage Pf-iRBCs-iRBCs become less deformable and get trapped in the splenic endothelial slits, a narrow 2 μm passage between cell junctions in splenic sinusoids. Microsphiltration assesses the ability of iRBCs to squeeze through narrow fissures between metallic microspheres of 5-25 μm in diameter arranged in a matrix, by computing the retention rate from the upstream and downstream proportions of iRBCs (Deplaine et al., 2011; Lavazec, 2017; Ndour, 2017). It has been applied as high-throughput screening of drug-induced parasite stiffening (Duez et al., 2018). Additionally, microfluidic devices have been used to mimic spleen filtration and the process of pitting with an array of rectangular pillars of PDMS with tuneable slit sizes down to few micrometres (Figure 3A) (Rigat-Brugarolas, 2014). Elizalde-Torrent and colleagues showed that by regulating the slit size and the blood flow it is possible to remove the solid parasite from the cytoplasm of an iRBC without destructing the cell (Elizalde-Torrent et al., 2021). Taken together, Pf-iRBCs become more rigid as the parasite progresses through the blood developmental cycle, increasing their vulnerability to spleen clearance as shown by the use of microfluidic devices.
The increase in parasitised RBC rigidity is in part due to the presence of a growing nondeformable intracellular parasite (Fedosov et al., 2011a), and to a number of parasite-encoded proteins, that are exported into the infected cell where they associate with the RBC membrane and cytoskeleton. Plasma membrane deformability of iRBCs has been measured by optical tweezers, as reported in Figure 3A, following the thermal motion of single RBCs held in a laser trap (Paul et al., 2019) or by stretching cells attached to beads (Suresh et al., 2005; Mills et al., 2007). The shear modulus of RBCs is found to increase by up to 10-fold during parasite development (Suresh et al., 2005), in the range of 20-60 µN/m at the trophozoite stage and 25-90 µN/m at the schizont stage (Suresh, 2006) (Table 1). Another non-invasive optical technique, tomographic phase microscopy, has been used to obtain information about the morphology of parasite vacuoles and decreased haemoglobin content by mapping the refractive index of iRBCs (Park et al., 2008).
A unique feature of P. falciparum is sequestration of iRBC in the microvasculature, a survival mechanism to prevent clearance by the spleen and prolong the time in the vasculature to allow the parasite to complete its intra-erythrocytic life cycle. Although sequestration has evolved as a protective mechanism for the parasite, it can lead to severe disease when large numbers of iRBC accumulate in specific microvascular beds. This phenomenon is linked to severe disease complications such as cerebral malaria or placental malaria. Parasite binding to endothelial cells (ECs) is mediated by the variant adhesion protein family P. falciparum erythrocyte membrane protein 1 (PfEMP1), which is encoded by a repertoire of approximately 60 var genes, and expressed at the surface of the iRBC (Smith et al., 2013). P. falciparum has evolved a complex protein machinery and subcellular structures to support trafficking of PfEMP1 to the membrane of iRBC. For example, P. falciparum has expanded a family of FIKK kinases implicated in this function. Flickering spectroscopy (Figure 3A) seems to suggest that a member of this family, FIKK 4.1, plays also a role in rigidification of the iRBC cytoskeleton (Davies et al., 2020). Other P. falciparum erythrocyte-binding proteins such as STEVOR and the ring-infected erythrocyte surface antigen (RESA) have been proved to concur to iRBC membrane rigidity as assessed by microsphere filtrations and ektacytometry (Sanyal et al., 2012). Other parasite features responsible for the rigidification of RBCs are the knobs: 50-150 nm structures formed by the self-assembly of the parasite knob-associated histidine-rich protein (KAHRP). Knobs act as cytoadhesive platforms responsible for the correct display of PfEMP1, while interacting with the host cytoskeleton proteins (Aikawa et al., 1996). KAHRP interactions with the RBC cytoskeleton are highly dynamic; super resolution approaches have recently revealed that it interacts with both β-spectrin and actin in ring stages, while being exclusively associated with actin in trophozoites (Sanchez, 2022). The presence of positively charged knobs partially contributes to iRBC binding to the negative membrane of ECs (Aikawa et al., 1996) (Figure 3A), and their increased membrane density in the last 24 hours of parasite maturation has been identified as one of the primary stiffening factors of the host cell (Herricks et al., 2009; Zhang et al., 2015). The role of KAHRP in the increased rigidity of parasitised RBCs has been determined by micropipette aspiration (Figure 3A), by measuring the membrane elastic shear modulus of iRBCs. Transgenic kahrp knockout parasites presented significant lower membrane shear elastic modulus than wild type lines, and this effect was attributed to the high transcriptional expression of KAHRP and to its clustering at the membrane (Glenister et al., 2002). Recently, the use of flickering spectroscopy by Fröhlich et al. (Froühlich et al., 2019) has confirmed iRBC stiffening during the parasite intra-erythrocytic cycle. In particular, trophozoites present a fourfold increase in surface tension increased, and a sevenfold rise in cytosol viscosity compared to uninfected RBCs. Mathematical simulations have revealed that knob formation strongly affects the tension by suppressing membrane fluctuations and increasing membrane-cytoskeleton coupling. In this study, flickering spectroscopy has been also used to better understand how changes in RBCs mechanical properties in hemoglobinopathies might confer protection to severe malaria. Uninfected RBCs containing haemoglobin S (HbAS) and C (HbAC) display surface tensions significantly higher than wild type RBC (HbAA), in line with the idea that higher membrane rigidity hampers parasite infection, and HbAS and HbAC trophozoites show an increased bending modulus and fewer but enlarged knobs (Froühlich et al., 2019).
Pf-iRBCs bind to a broad array of endothelial receptors. Among these, PfEMP1 binding to ICAM-1 (Ockenhouse et al., 1992) and EPCR (Turner et al., 2013) has been associated to cerebral malaria (Lennartz et al., 2017), binding to CD36 (Barnwell et al., 1989) to uncomplicated malaria (Storm et al., 2019) and binding to the glycan CSA has been linked to placental malaria. Figure 3B zooms on iRBCs interactions to endothelial receptors. Early studies of iRBC sequestration under fluid shear stress were performed in bulky flow chambers, usually made of rigid materials impermeable to gas exchange. In these devices, iRBC binding was perfused with a flow pump over a channel seeded with ECs or coated with endothelial receptors. Parasite binding was usually quantified under flow or after fixation of the experiment.
In order to avoid splenic clearance iRBC-EC bonds must withstand considerable forces (2-100 dyne/cm2) due to fluid shear stress caused by blood flow (Koutsiaris et al., 2013). There are three types of bonds that can be formed between a receptor-ligand pair: slip bonds, catch bonds, and ideal bonds. Slip bonds break faster when an external force is applied; catch bonds, on the other hand, take longer to dissociate when subjected to an increasing force, and finally the lifetime of an ideal bond is independent on the force acting on it. Lim et al. studied the single molecule interaction between iRBCs and ICAM-1 and CD36 by functionalising an AFM cantilever with these human recombinant proteins (Lim et al., 2017) (Figure 3B). They found that indeed ICAM-1 forms catch bond interactions with iRBCs that persist even under flow (single and multiple bonds), while iRBCs formed slip bonds with CD36. Surprisingly the rate of association of iRBC-ICAM-1 bonds is ten times lower than iRBC-CD36 (Lim et al., 2017). This result suggests that ICAM-1 is not the sole mediator for malaria cytoadhesion in the brain, and highlights the importance of other brain receptors such as EPCR.
Over the years, experimenting with the impact of flow has become much more accessible due to the commercialisation of easy to use small single-channel flow chambers. These commercial devices are being used to identify endothelial receptors that mediate binding of P. falciparum-tissue culture adapted parasite lines (Lennartz et al., 2017) or field isolates (Storm et al., 2019). These experiments have been crucial to reveal static and rolling interactions with the aforementioned receptors (Figure 3B). Flow experiments revealed that the rolling of schizont-stage infected cells under physiological flow conditions resembles the behaviour of leukocytes during acute inflammation (Helms et al., 2016; Dasanna et al., 2017). Mathematical simulations are in agreement with the experimental results (Dasanna et al., 2019), and indicate that the peculiar flip of trophozoites is due to the inertia of the solid parasite mass localised on one side of the host cell (Fedosov et al., 2011b). The rolling behaviour of late stage iRBCs can be linked to knob distribution and the stiffer plasma membrane, which is less prone to elastic deformation in flow (Lansche et al., 2018). Conversely, deformable uninfected RBCs undergo different motions near the vessel wall like tumbling, tank-treading, and rolling depending on the flow rate.
Additionally, microfluidic platforms have proven to be a reliable and physiologically relevant in vitro alternative to study the role of endothelial glycocalyx (Figure 3B) (Haymet et al., 2021). Glycocalyx breakdown has been recently associated with severe malaria (Hempel et al., 2016). For instance, brain swelling associated to cerebral malaria in children (Seydel et al., 2015), and fatal outcomes in Indonesian adults with falciparum malaria (Yeo et al., 2019; Georgiadou and Cunnington, 2019) can be traced to glycocalyx degradation. Nevertheless, the study of glycocalyx on malaria cytoadherence has been hindered by the experimental difficulty to obtain a mature glycocalyx in vitro (Barker et al., 2004; Tsvirkun et al., 2017). The glycocalyx participates in sensing mechanotransduction and shear stress influences its structure and thickness (Moore et al., 2021). Glycocalyx thickness varies from hundreds of nm to 1 μm depending on the techniques used to measure it - including electron and confocal microscopy (Kolářová et al., 2014), atomic force microscopy (Marsh and Waugh, 2013), and lately super resolution stochastic optical reconstruction microscopy (STORM) (Fan et al., 2019); and in vivo intravital microscopy (Liu et al., 2014; Kataoka et al., 2015). Initial studies on glass microcapillaries coated with a polymer brush to experimentally mimic the glycocalyx layer, found that RBCs travel with a velocity significantly lower than in bare capillaries (Lanotte et al., 2014), leading to the conclusion that the excessive flow resistance found in vivo can be attributed to the glycocalyx, as hemodynamic simulations previously suggested (Pries and Secomb, 2005). Perfusion systems lined with a human umbilical vein endothelial cell (HUVEC) monolayer have been instrumental to produce cell alignment (Sinha et al., 2016) and a glycocalyx layer similar to the one seen in vivo (Gouverneur et al., 2006). The loss of the sialic acids of the EC glycocalyx by enzymatic treatment increases adhesion of iRBCs without affecting their motion in proximity to the endothelium but decreasing their velocity by 10-14% (Introini et al., 2018b).
A main limitation of flow chambers is that they generally consist of a 1 mm-wide single channel in a linear display, and are therefore far away from recapitulating the complex hierarchical microvascular geometry or small vascular constrictions such as 5-10 μm capillaries or 2 μm endothelial slits. Recently, brain organoids have emerged as important 3D multicellular models (Velasco et al., 2019; Adams, 2021), but they have very heterogeneous non-controllable architecture and until today these systems lack perfusable vasculature.
Bioengineered 3D microvessel models are physiologically relevant alternatives to understand vascular dysfunctions and infections, as they reproduce the microvasculature architecture, geometry, and fluidic properties (Tan et al., 2020). These devices are built using microfabrication techniques, in hydrogels such as collagen or fibrin enclosed in PDMS or acrylic housing jigs. The dimensions of the endothelial microvessel networks can be controlled with micrometre precision by soft lithography patterning (Long et al., 2021). Bernabeu et al. examined the binding affinity of Pf-iRBC to 3D brain microvessels along a shear gradient (Bernabeu et al., 2019). This revealed that infected cells expressing PfEMP1 that bind to ICAM-1 were more adherent at shear forces greater than 1 dyne/cm2, further demonstrating iRBC-ICAM-1 catch bond behaviour. Binding of iRBC to EPCR was enhanced under low shear stress, suggesting that this bond might display catch-bond behaviour at lower shear forces (Bernabeu et al., 2019). However, future AFM approaches are needed to confirm this finding.
Further advances in microfabrication have generated endothelialised capillary-size vessels down to 5 μm in diameter by using multiphoton ablation (Rayner et al., 2021). This cutting-edge approach was used to study malaria microvascular obstruction and revealed that the rigidity of Pf-iRBC alone is not responsible for capillary blockade (Arakawa et al., 2020). Knobless parasites expressing PfEMP1 accumulated in the boundary between capillaries and venules, and the presence of knobs increased the affinity to capillary regions exposed to high shear flow. This system revealed highly distinctive spatial and temporal kinetics of iRBC accumulation and reproduced sequestration patterns observed in fatal cerebral malaria patients (Milner et al., 2015).
The study of malaria pathogenesis in more physiological systems is important, as endothelial transcription is modulated by both flow and stiffness of the extracellular matrix substrate. ECs possess mechanosensitive molecules such as caveolins, PIEZO1 or the glycocalyx that activate transcriptional pathways that modulate receptor expression as well as junctional proteins in response to culture under flow (Raasch et al., 2015; Reinitz et al., 2015). Furthermore, ECs can sense the mechanics of the surrounding tissue via the formation of focal adhesions (Wang et al., 2019), and when grown on stiff substrates become more permeable and sensitive to barrier disruptive molecules (Gordon et al., 2020). For example, ECs cultured on stiffer substrates have reduced glycocalyx (Mahmoud et al., 2021) and ICAM-1 (Scott et al., 2016) expression. Collectively this implies that the mechanics of the extracellular matrix may impact endothelial function and malaria pathology. While no studies to date have directly compared the effect of substrate stiffness to iRBC binding, future research needs to take these variables into account, as Pf-iRBC sequestration in the microvasculature of humans occurs at different stiffnesses (Zhang and Reinhart-King, 2020) and under a wide range of flow properties. As highlighted in Figure 3B, the study of malaria pathogenesis in bioengineered 3D microvessels with tunable flow and substrate biomechanical properties offers the opportunity to answer these questions.
Microfluidics is essential for the study of cytoadhesion because it allows systematic tuning of biological (haematocrit, parasitaemia) and physical (flow rate, geometry, substrate stiffness, temperature) parameters (Krishnan et al., 2011), however many aspects need to be explored further. For example, malaria cytoadherence increases at an elevated temperature (Udomsangpetch et al., 2002; Zhang et al., 2018), while deformability of trophozoites and schizonts decreases irreversibly (Mills et al., 2007; Park et al., 2008), and this plastic behaviour of iRBCs in response to fever could have implications when treatments are used to suppress fever episodes. The possibility of adopting customised designs and materials that reflect a range of vascular organisations, architectures, and cellular compositions across different organs (Budday et al., 2015) could shed light on the heterogeneity of malaria pathology. With the support of computational modelling (Lykov et al., 2015; Ishida et al., 2017), it could deliver a comprehensive picture of malaria disease to direct drug intervention. Another important bottleneck of current imaging experiments, both static and in flow, is image analysis. This is currently done almost exclusively manually, selecting individual cells, and following their behaviour over time. There is an urgent need for automated image analysis methods that can handle large image datasets in different conditions and extract key cell properties. This advancement would improve experiment reproducibility and provide more quantitative information.
An important host response to malaria infection is the activation of multiple immune pathways to facilitate parasite clearance. However, the subsequent release of cytokines can activate the endothelium, promote iRBC sequestration and severity (Pais and Penha-Gonçalves, 2018). While these immunological interactions play crucial roles in influencing malaria pathogenesis, the biomechanical processes that underpin them remain unknown. Instead, the biomechanics of phagocytosis and immune synapse formation have been well studied in the context of other diseases, as reviewed in (Chua et al., 2021). Monocytes, macrophages and neutrophils have all demonstrated various abilities to phagocytose Pf-iRBC and merozoites. Phagocytes recognise target cells, particles, or debris which stimulates the cell to form a cup-like protrusion that spreads over the target and resulting in its eventual engulfment. The spatiotemporal forces that are exerted on a particle during phagocytosis have recently been determined by microparticle traction force microscopy and LLSM, revealing that macrophages generate F-actin and myosin-I rich “teeth”-like protrusions (Vorselen et al., 2021). Interestingly, macrophages were three times more efficient at phagocytosing stiffened microparticles (Jaumouillé et al., 2019), and need longer period of time to internalise ellipsoid particles over spherical ones (Paul et al., 2013). It is therefore likely that significantly stiffer late stage iRBCs are more easily phagocytosed during infection. Likewise, CD8+ T cells and natural killer cells show greater activation (Liu et al., 2021), form larger synapses (Friedman et al., 2021), and demonstrate greater cytotoxicity against stiffer targets (Tello-Lafoz et al., 2021). Critically, cytotoxic T cells and macrophages accumulate in the cerebral vasculature during cerebral malaria, and are thought to be at least partially responsible for disease severity (Riggle et al., 2020). Understanding the biomechanics and the functional consequences of iRBC interactions with the human immune system in the microvascular context will shed light on malaria pathogenesis.
Only five out of over 200 known Plasmodium species are clinically relevant to humans: P. falciparum, P. vivax, P. malariae, P. ovale, and P. knowlesi. Of these, P. falciparum and P. vivax contribute the majority of cases. P. falciparum remains the only species for which all stages have been cultured in vitro, and most research efforts and antimalarial therapies/vaccine candidates are focused on it. The lack of research in other species, particularly P. vivax and the zoonotic species P. knowlesi, will be the limiting factor that will hold malaria elimination back. These species are quite distantly related to each other, and rely on both unique and conserved molecular interactions to invade human cells and cause a broad spectrum of clinical presentations, posing quite different challenges for disease control and antimalarial development (Keeling and Rayner, 2015). For example, P. vivax can remain in dormant forms in the liver causing a relapse of the disease at a later time (Prudencio et al., 2006).
Plasmodium species have different durations of the intraerythrocytic cycle, ranging from 27 (P. knowlesi), 48 (P. falciparum, vivax, and ovale), and 72 hours (P. malariae), and number of daughter merozoites in a schizont (up to 16 for P. knowlesi and to 24 for P. vivax). P. knowlesi merozoites are 2-3 μm, twice the size of their falciparum counterparts, and therefore easier to follow by video microscopy. The first images of malaria merozoites were recorded in 1975 by Dvorak et al. (Dvorak et al., 1975) were in fact of P. knowlesi infecting monkey RBCs. Thanks to their larger dimensions and by using P. knowlesi merozoites expressing fluorescent-tagged apical membrane antigen-1 (AMA-1), it was demonstrated for the first time that the apical complex is located at the wider end of the zoite (Yahata et al., 2021), contrary to what previously thought from early images of invading parasites by transmission electron microscopy (Miller et al., 1979). P. knowlesi have longer-lived (316 s) merozoites that glide onto RBC surfaces at 1.1 μm/s, faster than P. falciparum. Moreover, they can glide across multiple human RBC, up to 7, before selecting a cell to invade, and this behaviour resembles the travel of sporozoites along several hepatocytes before invading one (Mota et al., 2001; Tavares et al., 2013). Gliding could be advantageous for the parasites to sense suitable RBCs for invasion, facilitating the identification of preferred cells like young RBCs called reticulocytes which make up for less than 2% of circulating RBCs.
Mechanical and rheological differences among Plasmodium species are of pathological relevance. P. vivax has a distinct preference for immature reticulocytes, identified by the transferrin receptor (CD71) on their surface that is progressively lost during reticulocyte maturation into erythrocyte. Invasion accelerates the ejection of host material, including CD71, completing the maturation by 3 hours post invasion (Malleret, 2015). Similarly to P. falciparum (Narla and Mohandas, 2017), P. knowlesi-infected human RBCs (Barber et al., 2018) become more rigid progressing throughout the blood stage. Instead, both bulk and single-cell techniques (micropipette aspiration, laminar shear flow system, microfluidics) show that P. vivax-rings are more deformable than uninfected cells (Suwanarusk et al., 2004; Handayani et al., 2009; Malleret, 2015). This is possibly related to a two-fold increase in surface area of Pv-iRBCs, and hence a marked increase in their surface area to volume ratio (Suwanarusk et al., 2004). Methods to measure the RBC mechanical properties can assist in establishing whether higher RBC tension confers protection across red cell ages and genetic backgrounds, and explore whether Plasmodium species and strains are differently affected by this mechanism. This will be critical to understanding whether tension is a potential cross-species intervention target.
Cytoadherence phenomenon was long believed to be restricted to P. falciparum infection, however some evidence now supports that also other Plasmodium species undergo cytoadhesion events (Totino and Lopes, 2017). The occurrence of severe forms of P. vivax infections, such as cerebral and placental malaria, which were previously reported to be exclusively associated with P. falciparum, suggests that P. vivax can, to some extent, present adhesive phenotypes, even if it does not present any protein homologous to PfEMP1 (Totino and Lopes, 2017). Rosette formation, when uninfected RBCs adhere to iRBCs, appears in P. falciparum, P. vivax (Lee et al., 2014), and P. knowlesi, even though in the latter with less frequency and in the presence of human serum (Lee et al., 2021). Knob formation and sequestration are distinct characteristics of Pf-iRBCs. Pk-iRBCs (Russell and Cooke, 2017) do not develop knobs, but still can bind to lung and kidney endothelial cells primed with P. knowlesi culture supernatant via a functional ortholog of PfEMP1, although not to the cerebral endothelium (Lee et al., 2021). Such novel findings on the capability of other Plasmodium species to undergo cytoadhesion beyond P. falciparum will require further investigations that can be addressed by the use of biophysical approaches and concepts.
In this review we have emphasised the state-of-the-art technologies developed to probe the mechanics of malaria infection, specifically during Plasmodium invasion, maturation, and cytoadhesion, and how these would shape the future directions of malaria research. The contribution of RBC mechanics to invasion and vascular sequestration has recently become more evident thanks to the numerous biophysical techniques developed and applied to malaria. Some methods from soft matter, surface physics, and biomimetic nanotechnology (Wei et al., 2022) have been implemented to develop alternative therapeutic strategies, and together with novel ideas from the physics area that can be optimised for malaria research (Mognetti et al., 2019; Saint-Sardos et al., 2020) they should become part of our future toolbox to tackle new research questions.
VI, MG, and MB wrote the manuscript. JR, PC, and MB provided feedback and corrected the manuscript. All authors contributed to the article and approved the submitted version.
VI is funded by the Wellcome Trust Junior Interdisciplinary Fellowship (Wellcome 20485/Z/16/Z). MG is funded by the EIPOD4 fellowship programme (Grant agreement number 847543). PC and his lab are funded by the EPSRC (EP/R011443/1). JR and his lab are funded by the Wellcome Trust (220266/Z/20/Z). MB and her lab receive core program funding of the European Molecular Biology Laboratory (EMBL).
The authors declare that the research was conducted in the absence of any commercial or financial relationships that could be construed as a potential conflict of interest.
All claims expressed in this article are solely those of the authors and do not necessarily represent those of their affiliated organizations, or those of the publisher, the editors and the reviewers. Any product that may be evaluated in this article, or claim that may be made by its manufacturer, is not guaranteed or endorsed by the publisher.
The authors thank Virgilio Lew and Teresa Tiffert for many discussions over the years, and Dean Kos for providing insightful comments and proof-reading the manuscript.
Abdalrahman, T., Franz, T. (2017). Analytical Modeling of the Mechanics of Early Invasion of a Merozoite Into a Human Erythrocyte. J. Biol. Phys. 43 (4), 471–479. doi: 10.1007/s10867-017-9463-6
Abkarian, M., Massiera, G., Berry, L., Roques, M., Braun-Breton, C. (2011). A Novel Mechanism for Egress of Malarial Parasites From Red Blood Cells. Blood 117 (15), 4118–4124. doi: 10.1182/blood-2010-08-299883
Adams, Y., Olsen, R. W., Bengtsson, A., Dalgaard, N., Zdioruk, M., Satpathi, S., Behera, P. K., et al. (2021). Plasmodium Falciparum Erythrocyte Membrane Protein 1 Variants Induce Cell Swelling and Disrupt the Blood-Brain Barrier in Cerebral Malaria. J. Exp. Med. 218 (3), e20201266. doi: 10.1084/jem.20201266
Ademiloye, A. S., Zhang, L. W., Liew, K. M. (2017). A Three-Dimensional Quasicontinuum Approach for Predicting Biomechanical Properties of Malaria-Infected Red Blood Cell Membrane. Appl. Math. Modelling 49, 35–47. doi: 10.1016/j.apm.2017.04.030
Aikawa, M. (1997). Studies on Falciparum Malaria With Atomic-Force and Surface-Potential Microscopes. Ann. Trop. Med. Parasitol. 91 (7), 689–692. doi: 10.1080/00034989760419
Aikawa, M., Kamanura, K., Shiraishi, S., Matsumoto, Y., Arwati, H., Torii, M., et al (1996). Membrane Knobs of Unfixed Plasmodium Falciparum Infected Erythrocytes: New Findings as Revealed by Atomic Force Microscopy and Surface Potential Spectroscopy. Exp. Parasitol. 84 (3), 339–343. doi: 10.1006/expr.1996.0122
Akaki, M., Nagayasu, E., Nakano, Y., Aikawa, M. (2002). Surface Charge of Plasmodium Falciparum Merozoites as Revealed by Atomic Force Microscopy With Surface Potential Spectroscopy. Parasitol. Res. 88 (1), 16–20. doi: 10.1007/s004360100485
Allen, R. J. W., Kirk, K. (2010). Plasmodium Falciparum Culture: The Benefits of Shaking. Mol. Biochem. Parasitol. 169 (1), 63–65. doi: 10.1016/j.molbiopara.2009.09.005
Amino, R., Thiberge, S., Martin, B., Celli, S., Shorte, S., Frischknecht, F., et al (2006). Quantitative Imaging of Plasmodium Transmission From Mosquito to Mammal. Nat. Med. 12 (2), 220–224. doi: 10.1038/nm1350
Aniweh, Y., Gao, X., Hao, P., Meng, W., Lai, S. K., Gunalan, K., et al (2017). P. Falciparum RH5-Basigin Interaction Induces Changes in the Cytoskeleton of the Host RBC. Cell. Microbiol. 19 (9), e12747. doi: 10.1111/cmi.12747
Antia, M., Gao, X., Hao, P., Meng, W., Lai, S. K., Gunalan, K., et al (2008). Microfluidic Approaches to Malaria Pathogenesis. Cell. Microbiol. 10 (10), 1968–1974. doi: 10.1111/j.1462-5822.2008.01216.x
Arakawa, C., Gunnarsson, C., Howard, C., Bernabeu, M., Phong, K., Yang, E., et al (2020). Biophysical and Biomolecular Interactions of Malaria-Infected Erythrocytes in Engineered Human Capillaries. Sci. Adv. 6 (3), eaay7243. doi: 10.1126/sciadv.aay7243
Asada, M., Goto, Y., Yahata, K., Yokoyama, N., Kawai, S., Inoue, N., et al (2012). Gliding Motility of Babesia Bovis Merozoites Visualized by Time-Lapse Video Microscopy. PloS One 7 (4), e35227. doi: 10.1371/journal.pone.0035227
Awandare, G. A., Nyarko, P. B., Aniweh, Y., Ayivor-Djanie, R., Stoute, J. A., et al (2018). Plasmodium Falciparum Strains Spontaneously Switch Invasion Phenotype in Suspension Culture. Sci. Rep. 8 (1), 5782. doi: 10.1038/s41598-018-24218-0
Bannister, L. H., Mitchell, G. H., Butcher, G. A., Dennis, E. D., Cohen, S., et al (1986). Structure and Development of the Surface Coat of Erythrocytic Merozoites of Plasmodium Knowlesi. Cell Tissue Res. 245 (2), 281–290. doi: 10.1007/bf00213933
Barber, B. E., Russell, B., Grigg, M. J., Zhang, R., William, T., Amir, A., et al (2018). Reduced Red Blood Cell Deformability in Plasmodium Knowlesi Malaria. Blood Adv. 2 (4), 433–443. doi: 10.1182/bloodadvances.2017013730
Barker, A. L., Konopatskaya, O., Neal, C. R., Macpherson, J. V., Whatmore, J. L., Winlove, C. P., et al (2004). Observation and Characterisation of the Glycocalyx of Viable Human Endothelial Cells Using Confocal Laser Scanning Microscopy. Phys. Chem. Chem. Phys. 6, 1006–1011. doi: 10.1039/B312189E
Barnwell, J. W., Asch, A. S., Nachman, R. L., Yamaya, M., Aikawa, M., Ingravallo, P., et al (1989). A Human 88-kD Membrane Glycoprotein (CD36) Functions In Vitro as a Receptor for a Cytoadherence Ligand on Plasmodium Falciparum-Infected Erythrocytes. J. Clin. Invest. 84 (3), 765–772. doi: 10.1172/JCI114234
Baum, J., Papenfuss, A. T., Baum, B., Speed, T. P., Cowman, A. F., et al (2006). Regulation of Apicomplexan Actin-Based Motility. Nat. Rev. Microbiol. 4 (8), 621–628. doi: 10.1038/nrmicro1465
Bernabeu, M., Gunnarsson, C., Vishnyakova, M., Howard, C. C., Nagao, R. J., Avril, M., et al (2019). Binding Heterogeneity of Plasmodium Falciparum to Engineered 3D Brain Microvessels Is Mediated by EPCR and ICAM-1. MBio 10 (3), e00420–e00419. doi: 10.1128/mBio.00420-19
Bessis, M., Mohandas, N., Feo, C. (1980). Automated Ektacytometry: A New Method of Measuring Red Cell Deformability and Red Cell Indices. Blood Cells 6 (3), 315–327. doi: 10.1007/978-3-642-67756-4_13
Betz, T., Lenz, M., Joanny, J. F., Sykes, C. (2009). ATP-Dependent Mechanics of Red Blood Cells. PNAS 106 (36), 15320–15325. doi: 10.1073/pnas.0904614106
Blake, T. C. A., Haase, S., Baum, J. (2020). Actomyosin Forces and the Energetics of Red Blood Cell Invasion by the Malaria Parasite Plasmodium Falciparum. PloS Pathog. 16 (10), e1009007. doi: 10.1371/journal.ppat.1009007
Braun-Breton, C., Abkarian, M. (2016). Red Blood Cell Spectrin Skeleton in the Spotlight. Trends Parasitol. 32 (2), 90–92. doi: 10.1016/j.pt.2015.11.011
Budday, S., Nay, R., de Rooij, R., Steinmann, P., Wyrobek, T., Ovaert, T. C., et al (2015). Mechanical Properties of Gray and White Matter Brain Tissue by Indentation. J. Mechanical Behav. Biomed. Mater. 46, 318–330. doi: 10.1016/j.jmbbm.2015.02.024
Bustamante, L. Y., Powell, G. T., Lin, Y. C., Macklin, M. D., Cross, N., Kemp, A., et al (2017). Synergistic Malaria Vaccine Combinations Identified by Systematic Antigen Screening. PNAS 114 (45), 12045–12050. doi: 10.1073/pnas.1702944114
Byeon, H., Ha, Y. R., Lee, S. J. (2015). Holographic Analysis on Deformation and Restoration of Malaria-Infected Red Blood Cells by Antimalarial Drug. J. Biomed. Optics 20 (11), 115003. doi: 10.1117/1.JBO.20.11.115003
Callan-Jones, A., Albarran Arriagada, O. E., Massiera, G., Lorman, V., Abkarian, M. (2012). Red Blood Cell Membrane Dynamics During Malaria Parasite Egress. Biophys. J. 103 (12), 2475–2483. doi: 10.1016/j.bpj.2012.11.008
Chasis, J. A., Mohandas, N., Shohet, S. B. (1985). Erythrocyte Membrane Rigidity Induced by Glycophorin A-Ligand Interaction. Evidence for a Ligand-Induced Association Between Glycophorin A and Skeletal Proteins. J. Clin. Invest. 75 (6), 1919–1926. doi: 10.1172/JCI111907
Chen, B. C., Legant, W. R., Wang, K., Shao, L., Milkie, D. E., Davidson, M. W., et al (2014). Lattice Light-Sheet Microscopy: Imaging Molecules to Embryos at High Spatiotemporal Resolution. Science 346 (6208), 1257998. doi: 10.1126/science.1257998
Cho, S., Kim, S., Kim, Y., Park, Y. (2012). Optical Imaging Techniques for the Study of Malaria. Trends Biotechnol. 30 (2), 71–79. doi: 10.1016/j.tibtech.2011.08.004
Chua, C. L. L., Ng, I. M. J., Yap, B. J. M., Teo, A. (2021). Factors Influencing Phagocytosis of Malaria Parasites: The Story So Far. Malaria J. 20 (1), 319. doi: 10.1186/s12936-021-03849-1
Collins, C. R., Hackett, F., Atid, J., Tan, M. S. Y., Blackman, M. J. (2017). The Plasmodium Falciparum Pseudoprotease SERA5 Regulates the Kinetics and Efficiency of Malaria Parasite Egress From Host Erythrocytes. PloS Pathog. 13 (7), e1006453. doi: 10.1371/journal.ppat.1006453
Cowman, A. F., Tonkin, C. J., Tham, W. H., Duraisingh, M. T. (2017). The Molecular Basis of Erythrocyte Invasion by Malaria Parasites. Cell Host Microbe 22 (2), 232–245. doi: 10.1016/j.chom.2017.07.003
Crick, A. J., Tiffert, T., Shah, S. M., Kotar, J., Lew, V. L., Cicuta, P. (2013). An Automated Live Imaging Platform for Studying Merozoite Egress-Invasion in Malaria Cultures. Biophys. J. 104 (5), 997–1005. doi: 10.1016/j.bpj.2013.01.018
Crick, A. J., Theron, M., Tiffert, T., Lew, V. L., Cicuta, P., Rayner, J. C., et al (2014). Quantitation of Malaria Parasite-Erythrocyte Cell-Cell Interactions Using Optical Tweezers. Biophys. J. 107 (4), 846–853. doi: 10.1016/j.bpj.2014.07.010
Crosnier, C., Bustamante, L. Y., Bartholdson, S. J., Bei, A. K., Theron, M., Uchikawa, M., et al (2011). Basigin Is a Receptor Essential for Erythrocyte Invasion by Plasmodium Falciparum. Nature 480, 534–537. doi: 10.1038/nature10606
Dasanna, A. K., Lansche, C., Lanzer, M., Schwarz, U. S., et al (2017). Rolling Adhesion of Schizont Stage Malaria-Infected Red Blood Cells in Shear Flow. Biophys. J. 112 (9), 1908–1919. doi: 10.1016/j.bpj.2017.04.001
Dasanna, A. K., Fedosov, D. A., Gompper, G., Schwarz, U.S. (2019). State Diagram for Wall Adhesion of Red Blood Cells in Shear Flow: From Crawling to Flipping. Soft. Matter 15 (27), 5511–5520. doi: 10.1039/c9sm00677j
Dasgupta, S., Auth, T., Gov, N. S., Satchwell, T. J., Hanssen, E., Zuccala, E. S., et al (2014). Membrane-Wrapping Contributions to Malaria Parasite Invasion of the Human Erythrocyte. Biophys. J. 107 (1), 43–54. doi: 10.1016/j.bpj.2014.05.024
Davidson, M. S., Andradi-Brown, C., Yahiya, S., Chmielewski, J., O’Donnell, A. J., Gurung, P., et al (2021). Automated Detection and Staging of Malaria Parasites From Cytological Smears Using Convolutional Neural Networks. Biol. Imaging 1, e2. doi: 10.1017/S2633903X21000015
Davies, H., Belda, H., Broncel, M., Ye, X., Bisson, C., Introini, V., et al (2020). An Exported Kinase Family Mediates Species-Specific Erythrocyte Remodelling and Virulence in Human Malaria. Nat. Microbiol. 5 (6), 848–863. doi: 10.1038/s41564-020-0702-4
Deo, C. (2020). Hybrid Fluorescent Probes for Imaging Membrane Tension Inside Living Cells. ACS Cent. Sci. 6 (8), 1285–1287. doi: 10.1021/acscentsci.0c00977
Deplaine, G., Safeukui, I., Jeddi, F., Lacoste, F., Brousse, V., Perrot, S., et al (2011). The Sensing of Poorly Deformable Red Blood Cells by the Human Spleen Can Be Mimicked. Vitro Blood 117 (8), e88–e95. doi: 10.1182/blood-2010-10-312801
Depond, M., Henry, B., Buffet, P., Ndour, P. A. (2019). Methods to Investigate the Deformability of RBC During Malaria. Front. Physiol. 10. doi: 10.3389/fphys.2019.01613
Duez, J., Carucci, M., Garcia-Barbazan, I., Corral, M., Perez, O., Presa, J. L., et al (2018). High-Throughput Microsphiltration to Assess Red Blood Cell Deformability and Screen for Malaria Transmission–Blocking Drugs. Nat. Protoc. 13 (6), 1362–1376. doi: 10.1038/nprot.2018.035
Dulinska, I., Targosz, M., Strojny, W., Lekka, M., Czuba, P., Balwierz, W., et al (2006). Stiffness of Normal and Pathological Erythrocytes Studied by Means of Atomic Force Microscopy. J. Biochem. Biophys. Methods 66 (1-3), 1–11. doi: 10.1016/j.jbbm.2005.11.003
Dvorak, J. A., Miller, L. H., Whitehouse, W. C., Shiroishi, T. (1975). Invasion of Erythrocytes by Malaria Merozoites. Science 187 (4178), 748–750. doi: 10.1126/science.803712
Ebel, E. R., Kuypers, F. A., Lin, C., Petrov, D. A., Egan, E. S., et al (2021). Common Host Variation Drives Malaria Parasite Fitness in Healthy Human Red Cells. ELife 10, e69808. doi: 10.7554/eLife.69808
Elizalde-Torrent, A., Trejo-Soto, C., Méndez-Mora, L., Nicolau, M., Ezama, O., Gualdrón-López, M., et al (2021). Pitting of Malaria Parasites in Microfluidic Devices Mimicking Spleen Interendothelial Slits. Sci. Rep. 11 (1), 22099. doi: 10.1038/s41598-021-01568-w
Engelhardt, H., Gaub, H., Sackmann, E. (1984). Viscoelastic Properties of Erythrocyte Membranes in High-Frequency Electric Fields. Nature 30, 7378. doi: 10.1038/307378a0
Esposito, A., Choimet, J. B., Skepper, J. N., Mauritz, J. M.A., Lew, V. L., Kaminski, C. F., et al (2010). Quantitative Imaging of Human Red Blood Cells Infected With Plasmodium Falciparum. Biophys. J. 99 (3), 953–960. doi: 10.1016/j.bpj.2010.04.065
Evans, E., Mohandas, N., Leung, A. (1984). Static and Dynamic Rigidities of Normal and Sickle Erythrocytes. Major Influence of Cell Hemoglobin Concentration. J. Clin. Invest. 73 (2), 477–488. doi: 10.1172/jci111234
Evans, J., Gratzer, W., Mohandas, N., Parker Sleep, K. J. (2008). Fluctuations of the Red Blood Cell Membrane: Relation to Mechanical Properties and Lack of ATP Dependence. Biophys. J. 94 (10), 4134–4144. doi: 10.1529/biophysj.107.117952
Fan, J., Sun, Y., Xia, Y., Tarbell, J. M., Fu, B. M. (2019). Endothelial Surface Glycocalyx (ESG) Components and Ultra-Structure Revealed by Stochastic Optical Reconstruction Microscopy (STORM). Biorheology 56 (2-3), 77–88. doi: 10.3233/BIR-180204
Fedosov, D. A., Caswell, B., Suresh, S., Karniadakis, G. E. (2011a). Quantifying the Biophysical Characteristics of Plasmodium-Falciparum-Parasitized Red Blood Cells in Microcirculation. PNAS 108 (1), 35–39. doi: 10.1073/pnas.1009492108
Fedosov, D. A., Caswell, B., Karniadakis, G. E. (2011b). Wall Shear Stress-Based Model for Adhesive Dynamics of Red Blood Cells in Malaria. Biophys. J. 100 (9), 2084–2093. doi: 10.1016/j.bpj.2011.03.027
Fontes, A., Fernandes, H. P., de Thomaz, A. A., Barbosa, L. C., Barjas-Castro, M. L., Cesar, C. L. (2008). Measuring Electrical and Mechanical Properties of Red Blood Cells With Double Optical Tweezers. J. Biomed. Optics 13 (1), 014001. doi: 10.1117/1.2870108
Frevert, U., Engelmann, S., Zougbédé, S., Stange, J., Ng, B., Matuschewski, K., Liebes, L., et al (2005). Intravital Observation of Plasmodium Berghei Sporozoite Infection of the Liver. PloS Biol. 3, e192. doi: 10.1371/journal.pbio.0030192
Friedman, D., Simmonds, P., Hale, A., Bere, L., Hodson, N. W., White, M. R. H., et al (2021). Natural Killer Cell Immune Synapse Formation and Cytotoxicity are Controlled by Tension of the Target Interface. J. Cell Sci. 134 (7), jcs.258570. doi: 10.1242/jcs.258570
Frischknecht, F., Matuschewski, K. (2017). Plasmodium Sporozoite Biology. Cold Spring Harbor Perspect. Med. 7, a025478. doi: 10.1101/cshperspect.a025478
Froühlich, B., Jäger, J., Lansche, C., Sanchez, C. P., Cyrklaff, M., Buchholz, B., et al (2019). Hemoglobin S and C Affect Biomechanical Membrane Properties of P. Falciparum -Infected Erythrocytes. Commun. Biol. 2 (1), 1–11. doi: 10.1038/s42003-019-0556-6
Geoghegan, N. D., Evelyn, C., Whitehead, L. W., Pasternak, M., McDonald, P., Triglia, T., et al (2021). 4D Analysis of Malaria Parasite Invasion Offers Insights Into Erythrocyte Membrane Remodeling and Parasitophorous Vacuole Formation. Nat. Commun. 12, 3620. doi: 10.1038/s41467-021-23626-7
Georgiadou, A., Cunnington, A. J. (2019). Shedding of the Vascular Endothelial Glycocalyx: A Common Pathway to Severe Malaria? Clin. Infect. Dis.: Off. Publ. Infect. Dis. Soc. America 69 (10), 1721–1723. doi: 10.1093/cid/ciz043
Gilson, P. R., Crabb, B. S. (2009). Morphology and Kinetics of the Three Distinct Phases of Red Blood Cell Invasion by Plasmodium Falciparum Merozoites. Int. J. Parasitol. 39 (1), 91–96. doi: 10.1016/j.ijpara.2008.09.007
Glenister, F. K., Coppel, R. L., Cowman, A. F., Mohandas, N., Cooke, B. M., et al (2002). Contribution of Parasite Proteins to Altered Mechanical Properties of Malaria-Infected Red Blood Cells. Blood 1 99 (3), 1060–1063. doi: 10.1182/blood.v99.3.1060
Glodek, A. M., Mirchev, R., Golan, D. E., Khoory, J. A., Burns, J. M., Shevkoplyas, S. S., et al (2010). Ligation of Complement Receptor 1 Increases Erythrocyte Membrane Deformability. Blood 116 (26), 6063–6071. doi: 10.1182/blood-2010-04-273904
Glushakova, S., Yin, D., Li, T., Zimmerberg, J. (2005). Membrane Transformation During Malaria Parasite Release From Human Red Blood Cells. Curr. Biol. 15 (18), 1645–1650. doi: 10.1016/j.cub.2005.07.067
Glushakova, S., Kegawa, Y., Bezrukov, L., Waters, H., Blank, P. S., Zimmerberg, J., et al (2022). PIEZO1 E756del Gain of Function Mutation Makes Human Erythrocytes Less Habitable for Malaria Parasites. Biophys. J. 121 (3), 495a. doi: 10.1016/j.bpj.2021.11.332
Gordon, E., Schimmel, L., Frye, M. (2020). The Importance of Mechanical Forces for In Vitro Endothelial Cell Biology. Front. Physiol. 11. doi: 10.3389/fphys.2020.00684
Goujon, A., Colom, A., Straková, K., Mercier, V., Mahecic, D., Manley, S., et al (2019). Mechanosensitive Fluorescent Probes to Image Membrane Tension in Mitochondria, Endoplasmic Reticulum, and Lysosomes. J. Am. Chem. Soc. 141 (8), 3380–3384. doi: 10.1021/jacs.8b13189
Gouverneur, M., Spaan, J. A.E., Pannekoek, H., Fontijn, R. D., Vink, H. (2006). Fluid Shear Stress Stimulates Incorporation of Hyaluronan Into Endothelial Cell Glycocalyx. Am. J. Physiol. Heart Circulatory Physiol. 290 (1), H458–H452. doi: 10.1152/ajpheart.00592.2005
Groomes, P. V., Kanjee, U., Duraisingh, M. T. (2022). RBC Membrane Biomechanics and Plasmodium Falciparum Invasion: Probing Beyond Ligand-Receptor Interactions. Trends Parasitol. 38 (4), 301–315. doi: 10.1016/j.pt.2021.12.005
Grüring, C., Heiber, A., Kruse, F., Ungefehr, J., Gilberger, T.-W., Spielmann, T. (2011). Development and Host Cell Modifications of Plasmodium Falciparum Blood Stages in Four Dimensions. Nat. Commun. 2 (1), 165. doi: 10.1038/ncomms1169
Handayani, S., Chiu, D. T., Tjitra, E., Kuo, J. S., Lampah, D., Kenangalem, E., et al (2009). High Deformability of Plasmodium Vivax-Infected Red Blood Cells Under Microfluidic Conditions. J. Infect. Dis. 199 (3), 445–450. doi: 10.1086/596048
Hanssen, E., Knoechel, C., Klonis, N., Abu-Bakar, N., Deed, S., LeGros, M., et al (2011). Cryo Transmission X-Ray Imaging of the Malaria Parasite, P. Falciparum. J. Struct. Biol. 173 (1), 161–168. doi: 10.1016/j.jsb.2010.08.013
Hanssen, E., Dekiwadia, C., Riglar, D. T., Rug, M., Lemgruber, L., Cowman, A. F., et al (2013). Electron Tomography of Plasmodium Falciparum Merozoites Reveals Core Cellular Events That Underpin Erythrocyte Invasion: Ultrastructure of the Invading Malaria Parasite. Cell. Microbiol. 15 (9), 1457–1472. doi: 10.1111/cmi.12132
Haymet, A. B., Bartnikowski, N., Wood, E. S., Vallely, M. P., McBride, A., Yacoub, S., et al (2021). Studying the Endothelial Glycocalyx In Vitro: What Is Missing? Front. Cardiovasc. Med. 8. doi: 10.3389/fcvm.2021.647086
Helfrich, W., Servuss, R. M. (1984). Undulations, Steric Interaction and Cohesion of Fluid Membranes. Il. Nuovo Cimento D. 3 (1), 137–151. doi: 10.1007/bf02452208
Helms, G., Dasanna, A. K., Schwarz, U. S., Lanzer, M. (2016). Modeling Cytoadhesion of Plasmodium Falciparum-Infected Erythrocytes and Leukocytes-Common Principles and Distinctive Features. FEBS Lett. 590 (13), 1955–1971. doi: 10.1002/1873-3468.12142
Hempel, C., Pasini, E. M., Kurtzhals, J. A. L. (2016). Endothelial Glycocalyx: Shedding Light on Malaria Pathogenesis. Trends Mol. Med. 22 (6), 453–457. doi: 10.1016/j.molmed.2016.04.004
Herricks, T., Antia, M., Rathod, P. K. (2019). Deformability Limits of Plasmodium Falciparum-Infected Red Blood Cells. Cell. Microbiol. 11 (9), 1340–1353. doi: 10.1111/j.1462-5822.2009.01334.x
Hillringhaus, S., Dasanna, A. K., Gompper, G., Fedosov, D. A. (2009). Importance of Erythrocyte Deformability for the Alignment of Malaria Parasite Upon Invasion. Biophys. J. 117 (7), 1202–1214. doi: 10.1016/j.bpj.2019.08.027
Hillringhaus, S., Dasanna, A. K., Gompper, G., Fedosov, D. A. (2020). Stochastic Bond Dynamics Facilitates Alignment of Malaria Parasite at Erythrocyte Membrane Upon Invasion. ELife 9, e56500. doi: 10.7554/eLife.56500
Hosseini, S. M., Feng, J. J. (2012). How Malaria Parasites Reduce the Deformability of Infected Red Blood Cells. Biophys. J. 103 (1), 1–10. doi: 10.1016/j.bpj.2012.05.026
Introini, V., Crick, A., Tiffert, T., Kotar, J., Lin, Y. C., Cicuta, P. (2018a). Evidence Against a Role of Elevated Intracellular Ca2+ During Plasmodium Falciparum Preinvasion. Biophys. J. 114 (7), 1695–1706. doi: 10.1016/j.bpj.2018.02.023
Introini, V., Carciati, A., Tomaiuolo, G., Cicuta, P., abd Guido, S. (2018b). Endothelial Glycocalyx Regulates Cytoadherence in Plasmodium Falciparum Malaria. J. R. Soc. Interface 15 (149), 20180773. doi: 10.1098/rsif.2018.0773
Introini, V., Marin-Menendez, A., Nettesheim, G., Lin, Y.-C., Kariuki, S. N., Smith, A. L., et al (2022). The Erythrocyte Membrane Properties of Beta Thalassaemia Heterozygotes and Their Consequences for Plasmodium Falciparum Invasion. Cold Spring Harbor Laboratory doi: 10.1101/2022.01.13.476158
Ishida, S., Ami, A., Imai, Y. (2017). Factors Diminishing Cytoadhesion of Red Blood Cells Infected by Plasmodium Falciparum in Arterioles. Biophys. J. 113 (5), 1163–1172. doi: 10.1016/j.bpj.2017.07.020
Jaumouillé, V., Cartagena-Rivera, A. X., Waterman, C. M. (2019). Coupling of β2 Integrins to Actin by a Mechanosensitive Molecular Clutch Drives Complement Receptor-Mediated Phagocytosis. Nat. Cell Biol. 21 (11), 1357–1369. doi: 10.1038/s41556-019-0414-2
Kabaso, D., Shlomovitz, R., Auth, T., Lew, V. L., Gov, N. S. (2010). Curling and Local Shape Changes of Red Blood Cell Membranes Driven by Cytoskeletal Reorganization. Biophys. J. 99 (3), 808–816. doi: 10.1016/j.bpj.2010.04.067
Kariuki, S. N., Marin-Menendez, A., Introini, V., Ravenhill, B. J., Lin, Y. C., Macharia, A., et al (2020). Red Blood Cell Tension Protects Against Severe Malaria in the Dantu Blood Group. Nature 585, 579–583. doi: 10.1038/s41586-020-2726-6
Kariuki, S. N., Williams, T. N. (2020). Human Genetics and Malaria Resistance. Hum. Genet. 139 (6-7), 801–811. doi: 10.1007/s00439-020-02142-6
Kataoka, H., Ushiyama, A., Kawakami, H., Akimoto, Y., Matsubara, S., Iijima, T., et al (2015). Fluorescent Imaging of Endothelial Glycocalyx Layer With Wheat Germ Agglutinin Using Intravital Microscopy. Microscopy Res. Tech. 79 (1), 31–37. doi: 10.1002/jemt.22602
Keeling, P. J., Rayner, J. C. (2015). The Origins of Malaria: There Are More Things in Heaven and Earth. Parasitology 142, S16–S25. doi: 10.1017/S0031182014000766
Koch, M., Wright, K. E., Otto, O., Herbig, M., Salinas, N. D., Tolia, N. H., et al (2017). Plasmodium Falciparum Erythrocyte-Binding Antigen 175 Triggers a Biophysical Change in the Red Blood Cell That Facilitates Invasion. PNAS 114 (16), 4225–4230. doi: 10.1073/pnas.1620843114
Kolářová, H., Ambrůzová, B., Švihálková Šindlerová, L., Klinke, A., Kubala, L. (2014). Modulation of Endothelial Glycocalyx Structure Under Inflammatory Conditions. Mediators Inflammation 2014, 694312. doi: 10.1155/2014/694312
Koutsiaris, A. G., Tachmitzi, S. V., Batis, N. (2013). Wall Shear Stress Quantification in the Human Conjunctival Pre-Capillary Arterioles In Vivo. Microvascular Res. 85, 34–39. doi: 10.1016/j.mvr.2012.11.003
Krishnan, R., Klumpers, D. D., Park, C. Y., Rajendran, K., Trepat, X., van Bezu, J. (2011). Substrate Stiffening Promotes Endothelial Monolayer Disruption Through Enhanced Physical Forces. Am. J. Physiol. Cell Physiol. 300 (1), C146–C154. doi: 10.1152/ajpcell.00195.2010
Kumar, K., Srinivasan, P., Nold, M. J., Moch, J. K., Reiter, K., Sturdevant, D., et al (2017). Profiling Invasive Plasmodium Falciparum Merozoites Using an Integrated Omics Approach. Sci. Rep. 7 (1), 17146. doi: 10.1038/s41598-017-17505-9
Lanotte, L., Tomaiuolo, G., Misbah, C., Bureau, L., Guido, S., et al (2014). Red Blood Cell Dynamics in Polymer Brush-Coated Microcapillaries: A Model of Endothelial Glycocalyx. Vitro Biomicrofluidics 8 (1), 014104. doi: 10.1063/1.4863723
Lansche, C., Dasanna, A. K., Quadt, K., Fröhlich, B., Missirlis, D., Tétard, M., et al (2018). The Sickle Cell Trait Affects Contact Dynamics and Endothelial Cell Activation in Plasmodium Falciparum-Infected Erythrocytes. Commun. Biol. 1 (1), 211. doi: 10.1038/s42003-018-0223-3
Lavazec, C. (2017). Molecular Mechanisms of Deformability of Plasmodium-Infected Erythrocytes. Curr. Opin. Microbiol. 40, 138–144. doi: 10.1016/j.mib.2017.11.011
Lee, M. C. S., Lindner, S. E., Lopez-Rubio, J. J., Llinás, M. (2019). Cutting Back Malaria: CRISPR/Cas9 Genome Editing of Plasmodium. Briefings in Functional. Genomics 18 (5), 281–289. doi: 10.1093/bfgp/elz012
Lee, W.-C., Shahari, S., Nguee, S. Y.T., Lau, Y. L., Rénia, L., et al (2021). Cytoadherence Properties of Plasmodium knowlesi-infected erythrocytes. Front. Microbiol. 12. doi: 10.3389/fmicb.2021.804417
Lee, W.-C., Malleret, B., Lau, Y. L., Mauduit, M., Fong, M. Y., Cho, J. S., et al. (2014). Glycophorin C (CD236R) Mediates Vivax Malaria Parasite Rosetting to Normocytes. Blood 123 (18), e100–e109. doi: 10.1182/blood-2013-12-541698
Leffler, E. M., Band, G., Busby, G. B.J., Kivinen, K., Le, Q. S., Clarke, G. M., et al (2017). Resistance to Malaria Through Structural Variation of Red Blood Cell Invasion Receptors. Science 356 (6343). doi: 10.1126/science.aam6393
Lennartz, F., Adams, Y., Bengtsson, A., Olsen, R. W., Turner, L., Ndam, N. T., et al (2017). Structure-Guided Identification of a Family of Dual Receptor-Binding PfEMP1 That is Associated With Cerebral Malaria. Cell Host Microbe 21 (3), 403–414. doi: 10.1016/j.chom.2017.02.009
Lessard, S., Gatof, E. S., Beaudoin, M., Schupp, P. G., Sher, F., Ali, A., et al (2017). An Erythroid-Specific ATP2B4 Enhancer Mediates Red Blood Cell Hydration and Malaria Susceptibility. J. Clin. Invest. 127 (8), 3065–3074. doi: 10.1172/JCI94378
Lew, V. L. (2005). Malaria: Endless Fascination With Merozoite Release. Curr. Biol. 15 (18), R760–R761. doi: 10.1016/j.cub.2005.08.059
Lew, V. L. (2011). Malaria: Surprising Mechanism of Merozoite Egress Revealed. Curr. Biol. 21 (9), R314–R316. doi: 10.1016/j.cub.2011.03.066
Lew, V. L., Hockaday, A., Freeman, C. J., Bookchin, R. M., et al (1988). Mechanism of Spontaneous Inside-Out Vesiculation of Red Cell Membranes. J. Cell Biol. 106 (6), 1893–1901. doi: 10.1083/jcb.106.6.1893
Lew, V. L., Tiffert, T. (2007). Is Invasion Efficiency in Malaria Controlled by Pre-Invasion Events? Trends Parasitol. 23 (10), 481–484. doi: 10.1016/j.pt.2007.08.001
Lew, V. L., Tiffert, T. (2017). On the Mechanism of Human Red Blood Cell Longevity: Roles of Calcium, the Sodium Pump, PIEZO1, and Gardos Channels. Front. Physiol. 8. doi: 10.3389/fphys.2017.00977
Lim, H. W. G., Wortis, M., Mukhopadhyay, R. (2002). Stomatocyte-Discocyte-Echinocyte Sequence of the Human Red Blood Cell: Evidence for the Bilayer- Couple Hypothesis From Membrane Mechanics. PNAS 99 (26), 16766–16769. doi: 10.1073/pnas.202617299
Lim, Y. B., Thingna, J., Cao, J., Lim, C. T. (2017). Single Molecule and Multiple Bond Characterization of Catch Bond Associated Cytoadhesion in Malaria. Sci. Rep. 7 (1), 4208. doi: 10.1038/s41598-017-04352-x
Liu, W., Wang, X., Bai, K., Lin, M., Sukhorukov, G., Wang, W. (2014). Microcapsules Functionalized With Neuraminidase can Enter Vascular Endothelial Cells In Vitro. J. R. Soc. Interface 11 (101), 20141027. doi: 10.1098/rsif.2014.1027
Liu, Y., Zhang, T., Zhang, H., Li, J., Zhou, N., Fiskesund, R., et al (2021). Cell Softness Prevents Cytolytic T-Cell Killing of Tumor-Repopulating Cells. Cancer Res. 81 (2), 476–488. doi: 10.1158/0008-5472.CAN-20-2569
Long, R. K. M., Piatti, L., Korbmacher, F., Bernabeu, M. (2021). Understanding Parasite-Brain Microvascular Interactions With Engineered 3D Blood-Brain Barrier Models. Mol. Microbiol. 117 (3), 693–704. doi: 10.1111/mmi.14852
Lykov, K., Li, X., Lei, H., Pivkin, I. V., Karniadakis, G. E. (2015). Inflow/Outflow Boundary Conditions for Particle-Based Blood Flow Simulations: Application to Arterial Bifurcations and Trees. PloS Comput. Biol. 11 (8), e1004410. doi: 10.1371/journal.pcbi.1004410
Ma, S., Cahalan, S., LaMonte, G., Grubaugh, N. D., Zeng, W., Murthy, S. E., et al (2018). Common PIEZO1 Allele in African Populations Causes RBC Dehydration and Attenuates Plasmodium Infection. Cell 173 (2), 443–455.e12. doi: 10.1016/j.cell.2018.02.047
Mahmoud, M., Cancel, L., Tarbell, J. M. (2021). Matrix Stiffness Affects Glycocalyx Expression in Cultured Endothelial Cells. Front. Cell Dev. Biol. 9. doi: 10.3389/fcell.2021.731666
Malaria Genomic Epidemiology Network (2015). A Novel Locus of Resistance to Severe Malaria in a Region of Ancient Balancing Selection. Nature 526 (7572), 253–257. doi: 10.1038/nature15390
Malleret, B., Li, A., Zhang, R., Tan, K. S. W., Suwanarusk, R., Claser, C. (2015). Plasmodium Vivax: Restricted Tropism and Rapid Remodeling of CD71-Positive Reticulocytes. Blood 125 (8), 1314–1324. doi: 10.1182/blood-2014-08-596015
Marsh, G., Waugh, R. E. (2013). Quantifying the Mechanical Properties of the Endothelial Glycocalyx With Atomic Force Microscopy. J. Visualized Experiments 72, e50163. doi: 10.3791/50163
Mauritz, J. M. A., Esposito, A., Ginsburg, H., Kaminski, C. F., Tiffert, T., Lew, V. L. (2009). The Homeostasis of Plasmodium Falciparum-Infected Red Blood Cells. PloS Comput. Biol. 5 (4), e1000339. doi: 10.1371/journal.pcbi.1000339
Mauritz, J. M. A., Esposito, A., Tiffert, T., Skepper, J. N., Warley, A., Yoon, Y. Z. (2010). Biophotonic Techniques for the Study of Malaria-Infected Red Blood Cells. Med. Biol. Eng. Computing 48 (10), 1055–1063. doi: 10.1007/s11517-010-0668-0
Menard, R., Tavares, J., Cockburn, I., Markus, M., Zavala, F., Amino, R. (2013). Looking Under the Skin: The First Steps in Malarial Infection and Immunity. Nat. Rev. Microbiol. 11, 701–712. doi: 10.1038/nrmicro3111
Mikkelsen, R. B., Tanabe, K., Wallach, D. F. (1982). Membrane Potential of Plasmodium-Infected Erythrocytes. J. Cell Biol. 93 (3), 685–689. doi: 10.1083/jcb.93.3.685
Mikkelsen, R. B., Wallach, D. F. H., Van Doren, E., Nillni, E. A. (1986). Membrane Potential of Erythrocytic Stages of Plasmodium Chabaudi Free of the Host Cell Membrane. Mol. Biochem. Parasitol. 21 (1), 83–92. doi: 10.1016/0166-6851(86)90082-4
Miller, L. H., Aikawa, M., Johnson, J. G., Shiroishi, T. (1979). Interaction Between Cytochalasin B-Treated Malarial Parasites and Erythrocytes. Attachment and Junction Formation. J. Exp. Med. 149 (1), 172–184. doi: 10.1084/jem.149.1.172
Mills, J. P., Diez-Silva, M., Quinn, D. J., Dao, M., Lang, M. J., Tan, K. S. W., et al (2007). Effect of Plasmodial RESA Protein on Deformability of Human Red Blood Cells Harboring. PNAS 104 (22), 9213–9217. doi: 10.1073/pnas.0703433104
Milner, D. A., et al, Lee, J. J., Frantzreb, C., Whitten, R. O., Kamiza, S., Carr, R. A., et al (2015). Quantitative Assessment of Multiorgan Sequestration of Parasites in Fatal Pediatric Cerebral Malaria. J. Infect. Dis. 212 (8), 1317–1321. doi: 10.1093/infdis/jiv205
Mognetti, B. M., Cicuta, P., Di Michele, L. (2019). Programmable Interactions With Biomimetic DNA Linkers at Fluid Membranes and Interfaces. Rep. Prog. Phys. 82 (11), 116601. doi: 10.1088/1361-6633/ab37ca
Mohandas, N., Lie-Injo, L., Friedman, M., Mak, J. (1984). Rigid Membranes of Malayan Ovalocytes: A Likely Genetic Barrier Against Malaria. Blood 63 (6), 1385–1392. doi: 10.1182/blood.V63.6.1385.1385
Monzel, C., Sengupta, K. (2016). Measuring Shape Fluctuations in Biological Membranes. J. Phys. D.: Appl. Phys. 49 (24), 243002. doi: 10.1088/0022-3727/49/24/243002
Moore, K. H., Murphy, H. A., George, E. M. (2021). The Glycocalyx: A Central Regulator of Vascular Function. Am. J. Physiol. Regulatory Integr. Comp. Physiol. 320 (4), R508–R518. doi: 10.1152/ajpregu.00340.2020
Mota, M. M., Pradel, G., Vanderberg, J. P., Hafalla, J. C. R., Frevert, U., Nussenzweig, R. S., et al (2001). Migration of Plasmodium Sporozoites Through Cells Before Infection. Science 291, 141–144. doi: 10.1126/science.291.5501.141
Namvar, A., Blanch, A. J., Dixon, M. W., Carmo, O. M. S., Liu, B., Tiash, S., et al (2021). Surface Area-to-Volume Ratio, Not Cellular Viscoelasticity, Is the Major Determinant of Red Blood Cell Traversal Through Small Channels. Cell. Microbiol. 23 (1), e13270. doi: 10.1111/cmi.13270
Narla, J., Mohandas, N. (2017). Red Cell Membrane Disorders. Int. J. Lab. Hematol. 39 (S1), 47–52. doi: 10.1111/ijlh.12657
Nash, G. B., O’Brien, E., Gordon-Smith, E., Dormandy, J. (1989). Abnormalities in the Mechanical Properties of Red Blood Cells Caused by Plasmodium falciparum. Blood 1 74 (2), 855–861. doi: 10.1182/blood.V74.2.855.bloodjournal742855
Ndila, C. M., Uyoga, S., Macharia, A. W., Nyutu, G., Peshu, N., Ojal, J., et al (2018). Human Candidate Gene Polymorphisms and Risk of Severe Malaria in Children in Kilifi, Kenya: A Case-Control Association Study. Lancet Haematol. 5 (8), e333–e345. doi: 10.1016/S2352-3026(18)30107-8
Ndour, P. A., Larréché, S., Mouri, O., Argy, N., Gay, F., Roussel, C., et al (2017). Measuring the Plasmodium Falciparum HRP2 Protein in Blood From Artesunate-Treated Malaria Patients Predicts Post-Artesunate Delayed Hemolysis. Sci. Trans. Med. 9 (397), eaaf9377. doi: 10.1126/scitranslmed.aaf9377
Nguetse, C. N., Purington, N., Ebel, E. R., Shakya, B., Tetard, M., Kremsner, P. G., et al (2020). A Common Polymorphism in the Mechanosensitive Ion Channel PIEZO1 Is Associated With Protection From Severe Malaria in Humans. PNAS 117 (16), 9074–9081. doi: 10.1073/pnas.1919843117
Niko, Y., Didier, P., Mely, Y., Konishi, G., Klymchenko, A. S. (2016). Bright and Photostable Push-Pull Pyrene Dye Visualizes Lipid Order Variation Between Plasma and Intracellular Membranes. Sci. Rep. 6 (1), 18870. doi: 10.1038/srep18870
Nyarko, P. B., Tarr, S. J., Aniweh, Y., Stewart, L. B., Conway, D. J., Awandare, G. A. (2020). Investigating a Plasmodium Falciparum Erythrocyte Invasion Phenotype Switch at the Whole Transcriptome Level. Sci. Rep. 10, 245. doi: 10.1038/s41598-019-56386-y
Ockenhouse, C. F., Betageri, R., Springer, T. A., Staunton, D. E. (1992). Plasmodium Falciparum-Infected Erythrocytes Bind ICAM-1 at a Site Distinct From LFA-1, Mac-1, and Human Rhinovirus. Cell 68 (1), 63–69. doi: 10.1016/0092-8674(92)90206-r
Pais, T. F., Penha-Gonçalves, C. (2018). Brain Endothelium: The “Innate Immunity Response Hypothesis” in Cerebral Malaria Pathogenesis. Front. Immunol. 9. doi: 10.3389/fimmu.2018.03100
Park, Y., Diez-Silva, M., Popescu, G., Lykotrafitis, G., Choi, W., Feld, M. S., et al (2008). Refractive Index Maps and Membrane Dynamics of Human Red Blood Cells Parasitized by Plasmodium Falciparum. PNAS 105 (37), 13730–13735. doi: 10.1073/pnas.0806100105
Park, Y., Best, C. A., Badizadegan, K., Dasari, R. R., Feld, M. S., Kuriabova, T., et al (2010). Measurement of Red Blood Cell Mechanics During Morphological Changes. PNAS 107 (15), 6731–6736. doi: 10.1073/pnas.0909533107
Paul, D., Achouri, S., Yoon, Y. Z., Herre, J., Bryant, C. E., Cicuta, P. (2013). Phagocytosis Dynamics Depends on Target Shape. Biophys. J. 105 (5), 1143–1150. doi: 10.1016/j.bpj.2013.07.036
Paul, A., Ramdani, G., Tatu, U., Langsley, G., Natarajan, V. (2019). Studying the Rigidity of Red Blood Cells Induced by Plasmodium Falciparum Infection. Sci. Rep. 9 (1), 6336. doi: 10.1038/s41598-019-42721-w
Pavlou, G., Biesaga, M., Touquet, B., Lagal, V., Balland, M., Dufour, A., et al (2018). Toxoplasma Parasite Twisting Motion Mechanically Induces Host Cell Membrane Fission to Complete Invasion Within a Protective Vacuole. Cell Host Microbe 24 (1), 81–96.e5. doi: 10.1016/j.chom.2018.06.003
Popescu, G., Ikeda, T., Goda, K., Best-Popescu, C. A., Laposata, M., Manley, S., et al (2006). Optical Measurement of Cell Membrane Tension. Phys. Rev. Lett. 97 (21), 218101. doi: 10.1103/PhysRevLett.97.218101
Pries, A. R., Secomb, T. W. (2005). Microvascular Blood Viscosity In Vivo and the Endothelial Surface Layer. Am. J. Physiol. Heart Circulatory Physiol. 289 (6), H2657–H2664. doi: 10.1152/ajpheart.00297.2005
Prudencio, M., Rodriguez, A., Mota, M. M. (2006).The Silent Path to Thousands of Merozoites: The Plasmodium Liver Stage. Nat. Rev. Microbiol. 4, 849–856, 2006. doi: 10.1038/nrmicro1529
Raasch, M., Rennert, K., Jahn, T., Peters, S., Henkel, T., Huber, O. (2015). Microfluidically Supported Biochip Design for Culture of Endothelial Cell Layers With Improved Perfusion Conditions. Biofabrication 7 (1), 15013. doi: 10.1088/1758-5090/7/1/015013
Rayner, S. G., Howard, C. C., Mandrycky, C. J., Stamenkovic, S., Himmelfarb, J., et al (2021). Multiphoton-Guided Creation of Complex Organ-Specific Microvasculature. Adv. Healthc. Mater. 10 (10), e2100031. doi: 10.1002/adhm.202100031
Reid, H. L., Barnes, A. J., Lock, P. J., Dormandy, J. A., Dormandy, T. L. (1976). A Simple Method for Measuring Erythrocyte Deformability. J. Clin. Pathol. 29 (9), 855–858. doi: 10.1136/jcp.29.9.855
Reinitz, A., DeStefano, J., Ye, M., Wong, A. D., Searson, P. C. (2015). Human Brain Microvascular Endothelial Cells Resist Elongation Due to Shear Stress. Microvascular Res. 99, 8–18. doi: 10.1016/j.mvr.2015.02.008
Rigat-Brugarolas, L. G., Elizalde-Torrent, A., Bernabeu, M., De Niz, M., Martin-Jaular, L., Fernandez-Becerra, C., et al. (2014). A Functional Microengineered Model of the Human Spleenon-on-a-Chip. Lab. Chip. 14 (10), 1715–1724. doi: 10.1039/c3lc51449h
Riggle, B. A., Manglani, M., Maric, D., Johnson, K. R., Lee, M.-H., Neto, O. L.A., et al (2020). CD8+ T Cells Target Cerebrovasculature in Children With Cerebral Malaria. J. Clin. Invest. 130 (3), 1128–1138. doi: 10.1172/JCI133474
Riglar, D. T., Richard, D., Wilson, D. W., Boyle, M. J., Dekiwadia, C., Turnbull, L., et al (2011). Super-Resolution Dissection of Coordinated Events During Malaria Parasite Invasion of the Human Erythrocyte. Cell Host Microbe 9 (1), 9–20. doi: 10.1016/j.chom.2010.12.003
Rogers, S., Lew, V. L. (2021). Up-Down Biphasic Volume Response of Human Red Blood Cells to PIEZO1 Activation During Capillary Transits. PloS Comput. Biol. 17 (3), e1008706. doi: 10.1371/journal.pcbi.1008706
Rudlaff, R. M., Kraemer, S., Marshman, J., Dvorin, J. D. (2020). Three-Dimensional Ultrastructure of Plasmodium Falciparum Throughout Cytokinesis. PloS Pathog. 16 (6), e1008587. doi: 10.1371/journal.ppat.1008587
Rug, M., Prescott, S. W., Fernandez, K. M., Cooke, B. M., Cowman, A. F. (2006). The Role of KAHRP Domains in Knob Formation and Cytoadherence of P. Falciparum-Infected Human Erythrocytes. Blood 108 (1), 370–378. doi: 10.1182/blood-2005-11-4624
Russell, B. M., Cooke, B. M. (2017). The Rheopathobiology of Plasmodium vivax and Other Important Primate Malaria Parasites. Trends Parasitol. 33 (4), 321–334. doi: 10.1016/j.pt.2016.11.009
Safeukui, I., Buffet, P. A., Perrot, S., Sauvanet, A., Aussilhou, B., Dokmak, S., et al (2013). Surface Area Loss and Increased Sphericity Account for the Splenic Entrapment of Subpopulations of Plasmodium Falciparum Ring-Infected Erythrocytes. PloS One 8, e60150. doi: 10.1371/journal.pone.0060150
Saint-Sardos, A., Sart, S., Lippera, K., Brient‐Litzler, E., Michelin, S., Amselem, G., et al (2020). High-Throughput Measurements of Intra-Cellular and Secreted Cytokine From Single Spheroids Using Anchored Microfluidic Droplets. Small 16 (49), e2002303. doi: 10.1002/smll.202002303
Sanchez, C. P., Patra, P., Chang, S. S., Karathanasis, C., Hanebutte, L., Kilian, N., et al. (2022). KAHRP Dynamically Relocalizes to Remodeled Actin Junctions and Associates With Knob Spirals in Plasmodium Falciparum-Infected Erythrocytes. Mol. Microbiol. 117 (2), 274–292. doi: 10.1111/mmi.14811
Sanyal, S., Egée, S., Bouyer, G., Perrot, S., Safeukui, I., Bischoff, E., et al (2012). Plasmodium Falciparum STEVOR Proteins Impact Erythrocyte Mechanical Properties. Blood 119 (2), e1–e8. doi: 10.1182/blood-2011-08-370734
Schrier, S. L., Rachmilewitz, E., Mohandas, N. (1989). Cellular and Membrane Properties of Alpha and Beta Thalassemic Erythrocytes are Different: Implication for Differences in Clinical Manifestations. Blood 74 (6), 2194–2202. doi: 10.1182/blood.v74.6.2194.2194
Scott, H. A., Quach, B., Yang, X., Ardekani, S., Cabrera, A. P., Wilson, R., et al (2016). Matrix Stiffness Exerts Biphasic Control Over Monocyte–Endothelial Adhesion via Rho-Mediated ICAM-1 Clustering. Integr. Biol.: Quantitative Biosci. Nano Macro 8 (8), 869–878. doi: 10.1039/c6ib00084c
Seydel, K. B., Kampondeni, S. D., Valim, C., Potchen, M. J., Milner, D. A., Muwalo, F. W., et al (2015). Brain Swelling and Death in Children With Cerebral Malaria. New Engl. J. Med. 372 (12), 1126–1137. doi: 10.1056/NEJMoa1400116
Shrirao, A. B., Schloss, R. S., Fritz, Z., Shrirao, M. V., Rosen, R., Yarmush, M. L. (2021). Autofluorescence of Blood and Its Application in Biomedical and Clinical Research. Biotechnol. Bioengineer. 118 (12), 4550–4576. doi: 10.1002/bit.27933
Sinha, A., Chu, T. T.T., Dao, M., Chandramohanadas, R. (2015). Single-Cell Evaluation of Red Blood Cell Bio-Mechanical and Nano-Structural Alterations Upon Chemically Induced Oxidative Stress. Sci. Rep. 5, 9768. doi: 10.1038/srep09768
Sinha, R., Le Gac, S., Verdonschot, N., van denBerg, A., Koopman, B., Rouwkema, J. (2016). Endothelial Cell Alignment as a Result of Anisotropic Strain and Flow Induced Shear Stress Combinations. Sci. Rep. 6 (1), 29510. doi: 10.1038/srep29510
Sisquella, X., Nebl, T., Thompson, J. K., Whitehead, L., Malpede, B. M., Salinas, N. D., et al (2017). Plasmodium Falciparum Ligand Binding to Erythrocytes Induce Alterations in Deformability Essential for Invasion. ELife 6, 1–20. doi: 10.7554/eLife.21083
Smith, J. D., Rowe, J. A., Higgins, M. K., Lavstsen, T. (2013). Malaria’s Deadly Grip: Cytoadhesion of Plasmodium Falciparum-Infected Erythrocytes. Cell. Microbiol. 15 (12), 1976–1983. doi: 10.1111/cmi.12183
Storm, J., Jespersen, J. S., Seydel, K. B., Szestak, T., Mbewe, M., Chisala, N. V., et al (2019). Cerebral Malaria Is Associated With Differential Cytoadherence to Brain Endothelial Cells. EMBO Mol. Med. 11 (2), e9164. doi: 10.15252/emmm.201809164
Suresh, S. (2006). Mechanical Response of Human Red Blood Cells in Health and Disease: Some Structure-Property-Function Relationships. J. Mater. Res. 21, 1871–1877. doi: 10.1557/jmr.2006.0260
Suresh, S., Spatz, J., Mills, J. P., Micoulet, A., Dao, M., Lim, C. T., et al (2005). Connections Between Single-Cell Biomechanics and Human Disease States: Gastrointestinal Cancer and Malaria. Acta Biomater. 1, 15–30. doi: 10.1016/j.actbio.2004.09.001
Suwanarusk, R., Cooke, B. M., Dondorp, A. M., Silamut, K., Sattabongkot, J., White, N. J., et al (2004). The Deformability of Red Blood Cells Parasitized by Plasmodium falciparum and P. vivax. J. Infect. Dis. 189, 2, 190–194. doi: 10.1086/380468
Tan, S. Y., Leung, Z., Wu, A. R. (2020). Recreating Physiological Environments In Vitro: Design Rules for Microfluidic-Based Vascularized Tissue Constructs. Small 16 (9), e1905055. doi: 10.1002/smll.201905055
Tapia, J., Vera, N., Aguilar, J., González, M., Sánchez, S. A., Coelho, P., et al (2021). Correlated Flickering of Erythrocytes Membrane Observed With Dual Time Resolved Membrane Fluctuation Spectroscopy Under Different D-Glucose Concentrations. Sci. Rep. 11 (1), 2429. doi: 10.1038/s41598-021-82018-5
Tavares, J., Formaglio, P., Thiberge, S., Mordelet, E., Van Rooijen, N., Medvinsky, A., et al (2013). Role of Host Cell Traversal by the Malaria Sporozoite During Liver Infection. J. Exp. Med. 210, 905–991. doi: 10.1084/jem.20121130
Tello-Lafoz, M., Srpan, K., Sanchez, E. E., Hu, J., Remsik, J., Romin, Y., et al (2021). Cytotoxic Lymphocytes Target Characteristic Biophysical Vulnerabilities in Cancer. Immunity 54 (5), 1037–1054.e7. doi: 10.1016/j.immuni.2021.02.020
Tham, W.-H., Lim, N. T.Y., Weiss, G. E., Lopaticki, S., Ansell, B. R.E., Bird, M., et al (2015). Plasmodium Falciparum Adhesins Play an Essential Role in Signalling and Activation of Invasion Into Human Erythrocytes. PloS Pathog. 11 (12), e1005343. doi: 10.1371/journal.ppat.1005343
Thye, T., Evans, J. A., Ruge, G., Loag, W., Ansong , D., Agbenyega , T., et al (2022). Human Genetic Variant E756del in the Ion Channel PIEZO1 Not Associated With Protection From Severe Malaria in a Large Ghanaian Study. J. Hum. Genet. 67 (1), 65–67. doi: 10.1038/s10038-021-00958-2
Tiffert, T., Lew, V. L., Ginsburg, H., Krugliak, M., Croisille, L., Mohandas, N., et al (2005). The Hydration State of Human Red Blood Cells and Their Susceptibility to Invasion by Plasmodium Falciparum. Blood 105 (12), 4853–4860. doi: 10.1182/blood-2004-12-4948
Tiffert, T., Lew, V. L. (2014). Dynamic Morphology and Cytoskeletal Protein Changes During Spontaneous Inside-Out Vesiculation of Red Blood Cell Membranes. Pflugers Archiv.: Eur. J. Physiol. 466 (12), 2279–2288. doi: 10.1007/s00424-014-1483-5
Timmann, C., Thye, T., Vens, M., Evans, J., May, J., Ehmen, C., et al (2012). Genome-Wide Association Study Indicates Two Novel Resistance Loci for Severe Malaria. Nature 489 (7416), 443–446. doi: 10.1038/nature11334
Totino, P. R., Lopes, S. C. (2017). Insights Into the Cytoadherence Phenomenon of Plasmodium Vivax: The Putative Role of Phosphatidylserine. Front. Immunol. 8. doi: 10.3389/fimmu.2017.01148
Treeck, M., Zacherl, S., Herrmann, S., Cabrera, A., Kono, M., Struck, N. S., et al (2009). Functional Analysis of the Leading Malaria Vaccine Candidate AMA-1 Reveals an Essential Role for the Cytoplasmic Domain in the Invasion Process. PloS Pathog. 5 (3), e1000322. doi: 10.1371/journal.ppat.1000322
Tsvirkun, D., Grichine, A., Duperray, A., Misbah, C., Bureau, L. (2017). Microvasculature on a Chip: Study of the Endothelial Surface Layer and the Flow Structure of Red Blood Cells. Sci. Rep. 7, 45036. doi: 10.1038/srep45036
Turner, L., Lavstsen, T., Berger, S. S., Wang, C. W., Petersen, J. E.V., Avril, M., et al (2013). Severe Malaria Is Associated With Parasite Binding to Endothelial Protein C Receptor. Nature 498 (7455), 502–505. doi: 10.1038/nature12216
Udomsangpetch, R., Pipitaporn, B., Silamut, K., Pinches, R., Kyes, S., Looareesuwan, S., et al (2002). Febrile Temperatures Induce Cytoadherence of Ring-Stage Plasmodium Falciparum-Infected Erythrocytes. PNAS 99, 11825–11829. doi: 10.1073/pnas.172398999
Valanciunaite, J., Kempf, E., Seki, H., Danylchuk, D. I., Peyriéras, N., Niko, Y., Klymchenko, A., et al. (2020). Polarity Mapping of Cells and Embryos by Improved Fluorescent Solvatochromic Pyrene Probe. Analytical Chem. 92 (9), 6512–6520. doi: 10.1021/acs.analchem.0c00023
Velasco, S., Kedaigle, A. J., Simmons, S. K., Nash, A., Rocha, M., Quadrato, G., et al (2019). Individual Brain Organoids Reproducibly Form Cell Diversity of the Human Cerebral Cortex. Nature 570 (7762), 523–527. doi: 10.1038/s41586-019-1289-x
Villegas-Mendez, A., Stafford, N., Haley, M. J., Pravitasari, N. E., Baudoin, F., Ali, A., et al (2021). The Plasma Membrane Calcium ATPase 4 Does Not Influence Parasite Levels But Partially Promotes Experimental Cerebral Malaria During Murine Blood Stage Malaria. Malaria J. 20 (1), 297. doi: 10.1186/s12936-021-03832-w
Vorselen, D., Barger, S. R., Wang, Y., Cai, W., Theriot, J. A., Gauthier, N. C., et al (2021). Phagocytic “Teeth” and Myosin-II “Jaw” Power Target Constriction During Phagocytosis. ELife 10, e68627. doi: 10.7554/eLife.68627
Waldecker, M., Dasanna, A. K., Lansche, C., Linke, M., Srismith, S., Cyrklaff, M., et al (2017). Differential Time-Dependent Volumetric and Surface Area Changes and Delayed Induction of New Permeation Pathways in P. Falciparum-Infected Hemoglobinopathic Erythrocytes. Cell. Microbiol. 19 (2), e12650. doi: 10.1111/cmi.12650
Wang, W., Lollis, E. M., Bordeleau, F., Reinhart-King, C. A. (2019). Matrix Stiffness Regulates Vascular Integrity Through Focal Adhesion Kinase Activity. FASEB 33 (1), 1199–1208. doi: 10.1096/fj.201800841R
Wei, W., Cheng, W., Dai, W., Lu, F., Cheng, Y., Jiang, T., et al (2022). A Nanodrug Coated With Membrane From Brain Microvascular Endothelial Cells Protects Against Experimental Cerebral Malaria. Nano Lett. 22 (1), 211–219. doi: 10.1021/acs.nanolett.1c03514
Weiss, G. E., Gilson, P. R., Taechalertpaisarn, T., Tham, W.-H., de Jong, N. W.M., Harvey, K. L. (2015). Revealing the Sequence and Resulting Cellular Morphology of Receptor-Ligand Interactions During Plasmodium Falciparum Invasion of Erythrocytes. PloS Pathog. 11 (2), e1004670. doi: 10.1371/journal.ppat.1004670
Weiss, G. E., Crabb, B. S., Gilson, P. R., et al. (2016). Overlaying Molecular and Temporal Aspects of Malaria Parasite Invasion. Trends Parasitol. 32 (4), 284–295. doi: 10.1016/j.pt.2015.12.007
Wetzel, D. M., Schmidt, J., Kuhlenschmidt, M. S., Dubey, J. P., Sibley, L. D. (2005). Gliding Motility Leads to Active Cellular Invasion by Cryptosporidium Parvum Sporozoites. Infect. Immun. 73 (9), 5379–5387. doi: 10.1128/IAI.73.9.5379-5387.2005
Wissing, F., Sanchez, C. P., Rohrbach, P., Ricken, S., Lanzer, M. (2002). Illumination of the Malaria Parasite Plasmodium Falciparum Alters Intracellular Ph: Implications for Live Cell Imaging. J. Biol. Chem. 277 (40), 37747–37755. doi: 10.1074/jbc.m204845200
World Malaria Report (2021). Available at: https://www.who.int/teams/globalmalaria-programme/reports/world-malaria-report-2021
Xavier, M., Rosendahl, P., Herbig, M., Kräter, M., Spencer, D., Bornhäuser, M., et al (2016). Mechanical Phenotyping of Primary Human Skeletal Stem Cells in Heterogeneous Populations by Real-Time Deformability Cytometry. Integr. Biol.: Quantitative Biosci. Nano Macro 8 (5), 616–623. doi: 10.1039/c5ib00304k
Yahata, K., Hart, M. N., Davies, H., Asada, M., Wassmer, S. C., Templeton, T. J., et al (2021). Gliding Motility of Plasmodium Merozoites. PNAS 118 (48), e2114442118. doi: 10.1073/pnas.2114442118
Yeo, T. W., Weinberg, J. B., Lampah, D. A., Kenangalem, E., Bush, P., Chen, Y., et al (2019). Glycocalyx Breakdown is Associated With Severe Disease and Fatal Outcome in Plasmodium Falciparum Malaria. Clin. Infect. Dis.: Off. Publ. Infect. Dis. Soc. America 69 (10), 1712–1720. doi: 10.1093/cid/ciz038
Yoon, Y.-Z., Kotar, J., Yoon, G., Cicuta, P. (2008). The Nonlinear Mechanical Response of the Red Blood Cell. Phys. Biol. 5 (3), 36007. doi: 10.1088/1478-3975/5/3/036007
Yoon, Y.-Z., Hong, H., Brown, A., Kim, D. C., Kang, D. J., Lew, V. L., et al (2009). Flickering Analysis of Erythrocyte Mechanical Properties: Dependence on Oxygenation Level, Cell Shape, and Hydration Level. Biophys. J. 97 (6), 1606–1615. doi: 10.1016/j.bpj.2009.06.028
Zhang, Y., Huang, C., Kim, S., Golkaram, M., Dixon, M. W.A., Tilley, L., et al (2015). Multiple Stiffening Effects of Nanoscale Knobs on Human Red Blood Cells Infected With Plasmodium Falciparum Malaria Parasite. PNAS 112 (19), 6068–6073. doi: 10.1073/pnas.1505584112
Zhang, R., Chandramohanadas, R., Lim, C. T., Dao, M. (2018). Febrile Temperature Elevates the Expression of Phosphatidylserine on Plasmodium Falciparum (FCR3CSA) Infected Red Blood Cell Surface Leading to Increased Cytoadhesion. Sci. Rep. 8, 15022. doi: 10.1038/s41598-018-33358-2
Keywords: malaria, biophysics, imaging, Plasmodium, cytoadhesion, microfluidics, mechanobiology
Citation: Introini V, Govendir MA, Rayner JC, Cicuta P and Bernabeu M (2022) Biophysical Tools and Concepts Enable Understanding of Asexual Blood Stage Malaria. Front. Cell. Infect. Microbiol. 12:908241. doi: 10.3389/fcimb.2022.908241
Received: 30 March 2022; Accepted: 27 April 2022;
Published: 31 May 2022.
Edited by:
Paul R. Gilson, Burnet Institute, AustraliaReviewed by:
Catherine Lavazec, U1016 Institut Cochin (INSERM), FranceCopyright © 2022 Introini, Govendir, Rayner, Cicuta and Bernabeu. This is an open-access article distributed under the terms of the Creative Commons Attribution License (CC BY). The use, distribution or reproduction in other forums is permitted, provided the original author(s) and the copyright owner(s) are credited and that the original publication in this journal is cited, in accordance with accepted academic practice. No use, distribution or reproduction is permitted which does not comply with these terms.
*Correspondence: Viola Introini, dmkyMTFAY2FtLmFjLnVr
†ORCID: Viola Introini, orcid.org/0000-0001-9012-4696
Matt A. Govendir, orcid.org/0000-0002-2222-9143
Pietro Cicuta, orcid.org/0000-0002-9193-8496
Julian C. Rayner, orcid.org/0000-0002-9835-1014
Maria Bernabeu, orcid.org/0000-0001-7212-6209
Disclaimer: All claims expressed in this article are solely those of the authors and do not necessarily represent those of their affiliated organizations, or those of the publisher, the editors and the reviewers. Any product that may be evaluated in this article or claim that may be made by its manufacturer is not guaranteed or endorsed by the publisher.
Research integrity at Frontiers
Learn more about the work of our research integrity team to safeguard the quality of each article we publish.