- 1Department of Molecular Microbiology and Immunology (MMI), University of Texas at San Antonio, San Antonio, TX, United States
- 2South Texas Center for Emerging Infectious Diseases (STCEID), San Antonio, TX, United States
- 3United States (US) Department of Agriculture (USDA), Agricultural Research Service (ARS), Eastern Regional Research Center, Wyndmoor, PA, United States
A laboratory-acquired E. coli O157:H7 infection with associated severe sequelae including hemolytic uremic syndrome occurred in an individual working in the laboratory with a mixture of nalidixic acid-resistant (NalR) O157:H7 mutant strains in a soil-biochar blend. The patient was hospitalized and treated with an intravenous combination of metronidazole and levofloxacin. The present study investigated the source of this severe laboratory acquired infection and further examined the influence of the antibiotics used during treatment on the expression and production of Shiga toxin. Genomes of two Stx2a-and eae-positive O157:H7 strains isolated from the patient’s stool were sequenced along with two pairs of the wt strains and their derived NalR mutants used in the laboratory experiments. High-resolution SNP typing determined the strains’ individual genetic relatedness and unambiguously identified the two laboratory-derived NalR mutant strains as the source of the researcher’s life-threatening disease, rather than a conceivable ingestion of unrelated O157:H7 isolates circulating at the same time. It was further confirmed that in sublethal doses, the antibiotics increased toxin expression and production. Our results support a simultaneous co-infection with clinical strains in the laboratory, which were the causative agents of previous O157:H7 outbreaks, and further that the administration of antibiotics may have impacted the outcome of the infection.
Introduction
Shiga toxin-producing Escherichia coli (STEC) are important food-borne pathogens. Illness usually begins as watery diarrhea, advancing to bloody diarrhea and hemorrhagic colitis (HC). Infection may progress to a serious sequela known as hemolytic uremic syndrome (HUS), which can lead to end-stage renal disease and death (Feng et al., 2022). STEC O157:H7, often referred to as enterohemorrhagic E. coli (EHEC), is the serotype that has most often been associated with outbreaks and severe disease; however, in recent years an increased prevalence of infections caused by non-O157 STEC serogroups has been recognized (Gould et al., 2013; NACMCF, 2019). STEC refers to those strains of E. coli that produce at least one member of a class of phage-encoded potent cytotoxins called Shiga toxin (Stx) or verotoxin (VT), based on cytotoxicity on Vero cells (Bergan et al., 2012). The production of Stx, as either Shiga toxin 1 or 2, or subtypes and variants thereof is a virulence hallmark of STEC. There are several subtypes within the Stx1 and Stx2 types; three are recognized for Stx1 (Stx1a, Stx1c, and Stx1d), while multiple are currently recognized for Stx2 (Stx2a through m) (Scheutz et al., 2012; Bai et al., 2018; EFSA BIOHAZ Panel et al., 2020; Yang et al., 2020a; Bai et al., 2021). Stx1a and Stx2a are the prototypes of the Stx1 and Stx2 types and are considered ‘‘wild type’’ Stx1 and Stx2 (Skinner et al., 2014). Although Stx1a has been linked to human illness, STEC that produce subtypes Stx2a, Stx2c, and Stx2d are more often associated with the development of HC and HUS (Friedrich et al., 2002; Bielaszewska et al., 2006; Melton-Celsa, 2014).
Currently, no effective prophylaxis exists for HUS (Serna and Boedeker, 2008; Goldwater and Bettelheim, 2012), and data suggest that exposure to a variety of antibiotics may increase the risk of HUS in patients infected with STEC (Mody et al., 2021; Wong et al., 2000; Tarr et al., 2005; McGannon et al., 2010; Krüger et al., 2011; Bielaszewska et al., 2012; Wong et al., 2012). The bacterial SOS-response triggered by DNA damage is linked to phage induction and consequently, an increase in Stx production (Kimmitt et al., 2000; Fadlallah et al., 2015; Krüger and Lucchesi, 2015). Several studies have shown that Stx-production is induced by the chemotherapeutic agent mitomycin C due to activation of the SOS response following DNA damage (Hull et al., 1993; Shimizu et al., 2009; McGannon et al., 2010). It has been reported that antibiotic treatment of E. coli O157:H7 infections is contraindicated as it has been associated with severe sequelae such as HUS (Wong et al., 2000; Serna and Boedeker, 2008). Nevertheless, some clinical studies have revealed conflicting results (Tarr et al., 2005; Smith et al., 2012), and this issue remains a controversial one (Mohsin et al., 2015). Informed by the metadata analyses of several studies the use of antibiotics in individuals with STEC infections is not recommended (Freedman et al., 2016). Variables that contribute to the development of HUS include host factors, such as age (Tserenpuntsag et al., 2005) and the characteristics of the strain involved (Grif et al., 1998; Ogura et al., 2015; Yin et al., 2015). Furthermore, STEC strains that carry the stx2a variant are more often associated with severe infection, and there are several subtypes of stx2a-phages that are associated with different toxin production levels (Ogura et al., 2015).
Shiga toxin presence and activity can be detected by Vero cell cytotoxicity, immunologic, and PCR-based assays (Gerritzen et al., 2011; Parma et al., 2012; Scheutz et al., 2012; Qin et al., 2015; He Y et al., 2016; Armstrong et al., 2018). Different immunologic Stx detection assays have shown good specificity for the different Stx types and subtypes (Parma et al., 2012; He Z. et al., 2016; Armstrong et al., 2018). PCR-based assays targeting the stx genes have also been used to detect specific stx types and subtypes (Gerritzen et al., 2011; Scheutz et al., 2012).
To investigate the source of this E. coli O157:H7 infection, strains that were recovered from the patient in the hospital along with strains used by the researcher in laboratory experiments were sequenced and subjected to high-resolution SNP typing. Our second major objective was to determine whether the hospital administered antibiotics may have had an impact on the Stx-expression and -production levels, and ultimately on disease severity.
Materials and Methods
Clinical History and Strains Used in the Study
A laboratory-acquired E. coli O157:H7 infection occurred in 2013 in an individual who was working in the laboratory with a mixture of six E. coli O157:H7 strains in a soil-biochar blend. Symptoms included bloody diarrhea, hemolytic uremic syndrome (HUS), complete kidney failure, encephalopathy, respiratory failure, and retinal hemorrhages. After symptoms developed, the researcher was hospitalized. Pulsed-field gel electrophoresis (PFGE) analysis of the two isolates recovered from the patient’s stool in the hospital and the six nalidixic acid-resistant (NalR) mutant strains the researcher used in the laboratory experiment showed that the two patient isolates had indistinguishable PFGE patterns from laboratory strains E. coli O157:H7 strains RM7386 (7386) and RM6535 (6535). Strains used in this study, their characteristics, and sources are shown in Table 1.
Genome Sequencing, Assembly and Annotation
Strains were cultured in LB broth o/n at 37°C with shaking at 180 rpm. Total genomic DNA was extracted from the o/n culture using the QIAamp DNA Mini Kit (Qiagen, Inc., Valencia, CA, USA) for Illumina MiSeq and Nanopore MinION sequencing. For PacBio RS II sequencing, genomic DNA was extracted using the Genomic-tip 500/G kit (Qiagen). To close the genomes, we pursued a hybrid approach combining short-read Illumina MiSeq, long-read PacBio RS II, and Minion (Oxford Nanopore Technologies) technologies. For Illumina sequencing, a paired-end library was prepared for all strains using the NxSeq AmpFREE Low DNA Library Kit (Lucigen) and sequenced with 250-bp read length using the MiSeq Reagent Kit v2 500-cycle (Illumina) following the manufacturer’s guidelines. For MinION sequencing, genomic DNA of E. coli O157:H7 strain 7386 wt was diluted to a concentration of 1.5 µg genomic DNA in 46 µl nuclease-free water. The library was prepared using the Ligation Sequencing Kit 1D (SQK-LSK108) in combination with the Native Barcoding Kit (EXP-NBD103) according to the manufacturer’s instructions, and sequencing was performed on a MinION Mk1B. MinION reads were assembled with Canu version 1.1 (Koren et al., 2017). In addition, PacBio long-reads were generated at the University of Delaware DNA Sequencing and Genotyping Center, Delaware Biotechnology Institute in Newark, DE and at the Drexel University Genomics Core Facility in Philadelphia, PA. Genomic DNA was sheared into approximately 10-kb fragments using g-TUBE (Covaris, Inc., Woburn, MA, USA). The library was prepared based on the 10-kb PacBio sample preparation protocol and sequenced using P6/C4 chemistry on four single-molecule real-time (SMRT) cells with a 180-min collection time. The continuous long-read data were assembled de novo using the PacBio hierarchical genome assembly process (HGAP version 2.3.0) (Chin et al., 2013). Contigs were merged and circularized using Circlator (v 1.0.2). Assemblies were polished, and motifs were detected using RS_Modification_and_Motif_Analysis (v 2.3.0). The integrity of the resulting PacBio assemblies was evaluated by Canu (v 1.1) assembly of the MinION (Koren et al., 2017) reads for strain 7386WT and further by using Illumina short-reads in combination with the available Nanopore and/or PacBio long-reads with the hybrid assembler Unicycler (Wick et al., 2017) with Pilon error correction (Walker et al., 2014). Finally, molecules were rotated to the oriC (Luo and Gao, 2019) or repA genes for the chromosome and pO157 plasmid and annotated using the NCBI Prokaryotic Genome Annotation Pipeline (PGAP) (Tatusova et al., 2016).
Identification of Virulence/Resistance Genes and Shiga-Toxin and Intimin Subtypes
Virulence and resistance genes were identified in silico with VirulenceFinder (Joensen et al., 2014; Kleinheinz et al., 2014; Joensen et al., 2015), VFDB (Chen et al., 2016) and Card (Alcock et al., 2020). To determine the Shiga toxin subtype, a single colony from each strain was selected from tryptic soy agar plates and grown in Luria Bertani medium overnight (o/n). DNA template was prepared by incubating 100 μl of the bacterial culture in 900 μl of sterile H2O at 100°C for 10 min. PCR assays to identify stx subtypes were performed according to Scheutz et al. (2012) using a ProFlex PCR system (Thermo Fisher, Waltham, MA, USA) with slight modifications as indicated in Baranzoni et al. (2016). The assays targeted stx2a, 2b, 2c, 2d, 2e, 2f, and 2g. Gel electrophoresis using 1 µl of amplified DNA was performed using 2.0% UltraPure Agarose (Invitrogen, Carlsbad, CA, USA) with 0.5X GelRed (Phenix Research Products, Candler, NC, USA) in 1X Tris-acetate-EDTA buffer at 100 V for 1 h, and products were visualized using an AlphaImager gel documentation system (Alpha Innotech, San Leandro, CA, USA). The allelic subtype of the intimin (eae) was determined in silico according to Lacher et al. (2006) and Yang et al. (2020b).
Core Genome SNP Discovery
The core genome SNP discovery pipeline is implemented on Galaxy (Goecks et al., 2010; Afgan et al., 2018), an open-source web-based bioinformatics platform. The SNP discovery strategy, detailed in Rusconi et al. (2016), allows to determine strain-to-strain variation and to establish phylogenetic relationships within the genomes of various microbial pathogens (Eppinger et al., 2010; Eppinger et al., 2011; Eppinger et al., 2014; Nicholson et al., 2020; Petro et al., 2020). The core genome is defined as the set of genic and intragenic regions that are not repeated, do not contain phages, IS elements, plasmid regions, genomic islands, or other mobile genetic elements, which evolve at different rates and are not indicative of evolutionary relationships. These regions were determined for the complete annotated reference E. coli O157:H7 strain EC4115 chromosome (CP001164.1) as follows: repeats with NUCmer (Delcher et al., 2003) by running the reference against itself to find repeated regions, prophages with PHASTER (Zhou et al., 2011; Arndt et al., 2016; Arndt et al., 2017), IS elements using ISEScan (Xie and Tang, 2017) in Galaxy (Afgan et al., 2018), and plasmids using PlasmidFinder (Carattoli et al., 2014). The SNP discovery and verification pipeline contains the following modules:
SNP Discovery and Typing
Illumina reads for all six strains were uploaded in Galaxy for read-based SNP discovery. First, reads were aligned with BWA-MEM (Li and Durbin, 2009) to the selected reference genome EC4115. Resulting alignments were processed with Freebayes (Garrison and Marth, 2012) with the following threshold settings: mapping quality 30, base quality 30, coverage 10, and allelic frequency 0.75. Assemblies were analyzed using the contig-based workflow. Genomes were aligned with NUCmer against the reference strain EC4115 and SNPs were called with delta-filter and show-snps distributed with the MUMmer package (Delcher et al., 2003). The resulting SNP panel for each of the query genomes was used for further processing.
SNP Curation
To account for false positive calls, we used several SNP curation strategies detailed in our previous works (Eppinger et al., 2011; Eppinger et al., 2014; Rusconi et al., 2016; Nyong et al., 2020): SNPs located within repetitive or mobile regions in the reference (repeats, bacteriophages, plasmids and/or IS elements) were excluded as previously described (Eppinger et al., 2011). SNPs were further curated by extracting the surrounding nucleotides (40 nt) for each predicted SNP in the reference genome and BLASTn of this fragment against the query genomes (Altschul et al., 1990). Finally, resulting alignments were parsed to remove SNP locations with missing information (“no hits”), SNPs derived from ambiguous hits (>=2), low alignment quality or misalignments, non-uniformly distributed regions, and InDels, as previously described (Eppinger et al., 2011; Rusconi et al., 2016). Multinucleotide insertions and deletions of polymorphic bases were not considered SNPs and were excluded
SNP Annotation and Distribution
The curated catalogued SNPs from each query genome were merged into a single SNP panel, hereby reporting the SNP position, allelic and genic/intergenic status, and annotation.
SNP Validation of In Silico Predicted SNPs
To confirm the in silico predicted SNPs linked to nalidixic acid resistance (Saenz et al., 2003; Fabrega et al., 2009), we performed Sanger amplicon sequencing of the DNA gyrase and topoisomerase IV genes in clinical strain pairs 7386Nal/M1300706001A and 6535Nal/M1300706002 (Genewiz). Primers were designed with Primer Express (Applied Biosystems) and are listed along with PCR cycling conditions in Supplementary Table 1. Each reaction was performed in a volume of 20 μl using the Phusion High-Fidelity PCR Master Mix (Thermo Scientific) followed by PCR product purification with GeneJET PCR Purification Kit (Thermo Scientific), prior to Sanger sequencing (Genewiz). To confirm SNP alleles, sequencing results were compared to the corresponding SNP positions in the reference strain EC4115.
Phylogenomics
SNP Based Phylogeny
The identified curated SNP panel was used for phylogenetic reconstruction by maximum parsimony with PAUP v4.0a136 (Wilgenbusch and Swofford, 2003) with a 100 bootstrap replicates. The SNP tree was visualized in Geneious (vR9) (Kearse et al., 2012) and the majority consensus tree was built in Mesquite (Maddison and Maddison, 2021) and decorated with Evolview (Zhang et al., 2012; He Z. et al., 2016). Calculation of the consistency index for each SNP allowed us to identify parsimony informative SNPs and flag homoplastic SNPs as described in our previous works (Rusconi et al., 2016; Nyong et al., 2020).
Whole Genome Alignment-Based Phylogeny
To establish a phylogenetic framework and position the laboratory- and patient strains in the broader context of the O157:H7 step-wise evolutionary model (Wick et al., 2005; Feng et al., 2007), we constructed a whole genome phylogeny, including representative isolates for each of the nine clades (Manning et al., 2008) (Supplementary Table 2). The phylogeny was inferred by whole genome alignment with Mugsy (Angiuoli and Salzberg, 2011) and RAxML with 100 bootstrap replicates (Stamatakis, 2014). The tree topology was visualized in Geneious (Kearse et al., 2012) and decorated with respective strain-associated metadata in Evolview (Zhang et al., 2012; He Z. et al., 2016).
Minimum Inhibitory Concentration (MIC) and Antibiotics-Induced Stx2 Production
Determination of the Concentration of Antibiotics to Assess Their Effect on Toxin Production
The antibiotics tested on the strains were the same as those used in the treatment of the patient. Patient treatment consisted of a combination of 500 mg of metronidazole (Flagyl, MET) and 250 mg of levofloxacin (Levaquin, LEV) each day for a total of 16 days. MET was administered more than once on a number of days, and LEV was administered 3 times on one of the days. The expected peak plasma concentrations (Cmax) for MET at the treatment dose are expected to be ~25 µg/ml [Mandell et al., 2005; National Center for Biotechnology Information, PubChem Compound Database: http://pubchem.ncbi.nlm.nih.gov/compound/metronidazole (metronidazole C6H9N3O3)], and this concentration was used for testing the bacterial cultures since this was just below the assessed MIC (Andrews, 2001). For LEV, the expected Cmax indicated in the literature is approximately 5.0 µg/ml (range 4.1 to 11.3 µg/ml) at the dosage used in the patient (Sowinski et al., 2003; National Center for Biotechnology Information, PubChem Compound Database: http://pubchem.ncbi.nlm.nih.gov/compound/levofloxacin [C18H20FN3O4]). However, a concentration of 5.0 µg/ml killed the bacteria, and thus, 50 ng/ml (just below the MIC) was used. MET (RPI Research Products International, Mount Prospect, IL) and LEV (Chem-Impex International, Inc., Wood Dale, IL) were tested alone and in combination. As a positive control, mitomycin C (MMC), (Millipore Sigma, St. Louis, MO) which induces production of Stx2 was used at a concentration of 50 ng/ml, and tryptic soy broth (TSB) (Becton Dickinson, Franklin Lakes, NJ) without antibiotics was used as the negative control. The experiments were performed in duplicate and repeated twice.
Determination of Stx2 Levels in Strains Exposed to Antibiotics
Single colonies of each strain were inoculated into 10 ml of TSB and grown overnight at 37°C at 150 rpm, and then diluted 1:50 into fresh TSB and TSB with different antibiotics (2.0 x 107 CFU/ml in 10 ml TSB) (Skinner et al., 2014) and incubated at 150 rpm for 18 h at 37°C. The TSB was supplemented with either 50 ng/ml MMC, 25 μg/ml MET, or 50 ng/ml LEV, and with both 25 μg/ml MET and 50 ng/ml LEV. After incubation of the strains with the antibiotics for 18 h, the cultures were diluted 10-fold in TSB and plated onto tryptic soy agar (TSA), and colonies were counted to determine the CFU/ml after exposure to the antibiotics. The cultures were then centrifuged at 5,000 × g for 15 min at 4°C, and the supernatants were sterilized using 0.2-μm filters. Subsequently, the sterile supernatants were used for the quantification of Stx2 amount using a commercial indirect ELISA kit (Eurofins-Abraxis, Warminster, PA, USA; (PN 542010; https://abraxis.eurofins-technologies.com/home/products/rapid-test-kits/bacterial-toxins/shiga-toxin-elisa-plates/shiga-toxin-2-stx-2-elisa-96-test/) as recommended by the manufacturer. The sensitivity of this method is 30 pg/ml. Positive and negative controls were those included in the kit. For quantification of Stx2 production, a standard curve was simultaneously generated with each assay using serial dilutions of purified lyophilized Stx2a toxin from E. coli (List Biological Laboratories, Campbell, CA, USA; https://www.listlabs.com/products/shiga-toxins). A linear production was observed when serial dilutions included concentrations from 2.5 ng/ml to 78 pg/ml. Samples were diluted until their ODs were included in those shown in the standard curve to be in the linear range; most samples required a 1:500 dilution. The results were analyzed spectrophotometrically at 450 nm using a TECAN Safire II plate reader (Tecan, Morrisville, NC). The experiments were performed in duplicate and repeated at least twice.
Reverse Transcription (RT)-qPCR to Determine Expression of Stx2a
Expression of the stx2a gene in the laboratory and clinical strains was quantified using a RT-qPCR assay under non-induced, MMC, and antibiotic-induced conditions. A total of six strains were grown from an overnight culture in Luria-Bertani broth (LB, Thermo Fisher Scientific) at 37°C and 180 rpm to an OD600 of 0.3-0.5. Cultures were induced either with MMC at 0.5 µg/ml (Allué-Guardia et al., 2014) or a combination of the two hospital administered antibiotics, MET and LEV, at concentrations of 25 µg/ml and 50 ng/ml. After cultures were incubated for 18 h at 37°C at 180 rpm, total RNA was extracted using the PureLink RNA Mini Kit (Thermo Fisher Scientific) and treated with DNAse I (DNAse I, amplification grade, Invitrogen) following the manufacturer’s instructions. The RNA quantity and integrity were assessed at an absorbance of 260 nm using a UV-spectrophotometer and by agarose gel electrophoresis. RNA was then converted into cDNA (RevertAid H minus first strand cDNA Synthesis kit (Thermo Scientific), and stx2a was quantified using the Go-Taq® qPCR Master Mix (Promega, Madison, WI) and MicroAmp Fast optical real-time 96-well PCR plates (Applied Biosystems, Grand Island, NY) on an ABI StepOne PLUS Real-Time PCR system (Applied Biosystems). The primers and the cycling program used are described in Supplementary Table 1 (Wang et al., 2002; Gobert et al., 2007). Specificity was checked by analyzing the melting curves. Expression levels of stx2a were normalized against the endogenous gene tufA using the ABI StepOne PLUS System SDS software (Applied Biosystems) and are shown in relative quantity (RQ). Two biological replicates were conducted for each strain tested.
Statistical Analyses
Statistical significance was determined by two-way analysis of variance ANOVA using Prism (version 9.0.1) (GraphPad Software, San Diego, CA) for comparison of Shiga toxin production and expression among the different culture conditions within each strain and among the different strains for each culture condition. Differences among groups were performed by Tukey’s multiple comparison test. Kruskal Wallis-non-parametric ANOVA and Dunn’s multiple comparison test were used for analysis of log CFU/ml results. Statistical significance was considered when p < 0.05. A confidence level of 95% was applied.
Results and Discussion
Laboratory-Associated Infection With E. coli O157:H7
A researcher working with strains of E. coli O157:H7 developed sharp elbow pain with flu-like myalgias, followed by pain in multiple joints and eye pain two days after conducting an experiment with a six-strain cocktail of NalR E. coli O157:H7. His illness progressed to bloody diarrhea and severe abdominal pain that was alleviated with hydromorphone. Assessment following hospital admission was to refrain from antibiotic use until it was determined that infection was not due to STEC. Nonetheless, on the same day of admission, serologic testing for Campylobacter using the ImmunoCard STAT!®CAMPY assay returned a positive result. The patient was then started on two cycles of LEV and MET on days 1 and 2, before laboratory tests indicated the presence of STEC. The antibiotics were stopped on day 3 and then recommenced on day 4 for a total treatment time of 16 days, and the patient was diagnosed with pancolitis.
The E. coli O157:H7 infection was reported to the PA State Health Department where stool samples were sent for confirmation. The patient’s clinical course continued to deteriorate, and one week after hospitalization, the patient developed HUS, followed by respiratory failure, encephalopathy with seizures, and retinal hemorrhages. After treatment with hemodialysis and plasmapheresis the condition gradually improved and the individual was discharged 24 days after admission. The researcher was working with a mixture of a total of six STEC strains in the laboratory. The two strains isolated from the patient had indistinguishable PFGE patterns to two laboratory strains 6535 and 7386 that were part of the STEC strain mixture, pointing to a likely source of the patient’s severe O157:H7 co-infection. These clinical isolates are linked to an outbreak caused by contaminated iceberg lettuce and bagged lettuce, respectively (Table 1). Both strains carried the loci encoding the stx2a subtype and the eae-γ1 gene. The virulence profiles are shown in Supplementary Table 3, neither strain encodes antibiotic resistance genes. Cases of laboratory-associated infections caused by E. coli O157:H7 have been reported (Booth and Rowe, 1993; Burnens et al., 1993; Rao et al., 1996; Coia, 1998; Salerno et al., 2004; Spina et al., 2005). The latter article describes four laboratory-associated cases that occurred in New York State from 1999 to 2004. The authors stated that standard laboratory biosafety practices had not been strictly followed, and in the four cases, the low infectious dose and ability of the pathogen to survive on surfaces for prolonged periods contributed to transmission. Regular assessment of laboratory safety protocols, adequate training, and ensuring compliance are important to avert exposure to potential hazards and for prevention of laboratory-acquired infections.
It has been reported that stressing E. coli O157:H7 with quinolone antibiotics such as LEV can induce a bacterial stress response due to perturbation of the DNA gyrase (topoisomerase IV) cascading to a Gram-negative “SOS response” (Nassar et al., 2013), leading to increased production and release of Shiga toxin 2 (Stx2) and escalating the likelihood of hemolytic uremic syndrome (Wong et al., 2000; Panos et al., 2006; McGannon et al., 2010; Smith et al., 2012; Wong et al., 2012). However, treatment with certain classes of antibiotics does not result in an increase in Stx production (McGannon et al., 2010; Bielaszewska et al., 2012). McGannon et al. (2010) reported that antibiotics that interfere with DNA synthesis, including ciprofloxacin and trimethoprim-sulfamethoxazole, increased Stx production, but other classes of antibiotics that target the cell wall, ribosome, or RNA polymerase did not. Antibiotics (LEV and MET) were administered to the patient described in this report due to a positive result with the stool antigen test for Campylobacter. LEV is a quinolone that interferes with DNA replication, and MET belongs to the nitroimidazole class and inhibits nucleic acid synthesis. The stool antigen test for Campylobacter used on the patient has been reported to have a positive predictive value (PPV) of only 36.6% (Fitzgerald et al., 2016). Later it was however determined that the patient was not infected with Campylobacter. This conclusion was based on a negative PCR result testing the patient’s stool for Campylobacter, thus the initial Campylobacter antigen test gave likely a false positive result. A similar incident occurred in June of 2013 in Virginia when a 69-year-old woman infected with STEC O111 was misdiagnosed with Campylobacter by the same immunoassay used on the patient in the current report (Operario et al., 2014). The woman was treated with azithromycin, ciprofloxacin, and metronidazole, and she subsequently developed hemolytic uremic syndrome. The authors stated that “Campylobacter was not confirmed by culture or PCR, suggesting that the initial Campylobacter EIA [enzyme immunoassay] was likely a false-positive result.”
Whole Genome Sequence Typing (WGST) and Phylogenomic Relationship of Patient Recovered Isolates
Our phylogenomic analyses revealed how the analyzed laboratory- and patient strains fit into the phylogenomic context of the O157:H7 lineage. Numerous genomic epidemiology studies of STEC have embraced whole genome sequence typing (Franz et al., 2014; Sadiq et al., 2014; Eppinger and Cebula, 2015; Pightling et al., 2018), which proved critical for strain attribution and source identification (Bono, 2009; Eppinger et al., 2011; Holmes et al., 2015; Strachan et al., 2015; Yin et al., 2015; Cowley et al., 2016; Lupolova et al., 2016; Lee et al., 2017). To further investigate the source of infection, we sequenced a total of six genomes associated with this clinical case using NGS short- and long-read technologies on the Illumina, Oxford Nanopore, and PacBio platforms. The two isolate sets were comprised of wt outbreak strains 7386 (Washington lettuce, clade 8) and 6535 (Taco John, clade 2), the two derived NalR mutant strains the patient was exposed to in the laboratory, and further the two isolates recovered from the patient. To investigate the genetic relatedness of the wt and respective derived NalR mutants and clinical strains recovered from the patient, we reconstructed a phylogenomic hypotheses inferred from whole genome alignment (Figure 1). We included representative isolates from the stepwise evolutionary model of O157:H7, which has been refined by many groups (Whittam et al., 1988; Wick et al., 2005; Feng et al., 2007; Leopold et al., 2009; Zhou et al., 2010; Jung et al., 2013). The tree topology partitions the isolates into nine distinct clusters, as previously established by Manning (Manning et al., 2008). As evident from the tree topology, the Taco John (6535) and Washington lettuce outbreak (7386) strains, along with their laboratory-derived NalR mutants and isolates recovered from the patient, cluster with the representative clades 2 and 8 strains PA11 (Hartzell et al., 2011) and EC4115 (Eppinger et al., 2011), respectively. It is in the nature of isolates linked to single outbreaks to form tight clonal clusters and consequently outbreak investigations require the application of high-resolution subtyping strategies (Eppinger et al., 2011; Rusconi et al., 2016). To increase resolution and resolve the intimate relationship of the strains, we applied high resolution SNP typing (Figure 2). Core genome SNPs are highly informative in the context of outbreak investigation to differentiate “near clonal” outbreak isolates in support of strain attribution and outbreak ex- and inclusion (Eppinger et al., 2014; Jenkins et al., 2015; Rusconi et al., 2016). Whole genome SNP discovery and typing provided the necessary resolution to resolve the genetically homogenous population structure and allowed to differentiate near clonal laboratory wt and NalR mutant and clinical strains. The SNP analysis yielded a total of 588 SNPs, of which 572 were parsimony informative. Phylogenetic reconstruction based on the curated high-quality SNP panel with PAUP (Wilgenbusch and Swofford, 2003) identified two genetically distinct clusters separated by 551 SNPs, each comprised of the respective laboratory wt strains, 7386 and 6535, derived NalR mutants, and clinical isolates recovered from the patient. Strains within clusters 7386 and 6535 are near clonal and are either indistinguishable on the SNP-level or separated by one or two SNPs (Figure 2 and Supplementary Table 4). The NalR mutants feature non-synonymous SNPs in both the DNA gyrase and topoisomerase genes, which are known mutations conferring this particular resistance phenotype (Saenz et al., 2003; Fabrega et al., 2009). The in silico predicted SNPs for these two genes were further confirmed by Sanger amplicon sequencing. In STEC O157:H7 we previously demonstrated that such numbers of SNPs can arise during a single passage in the laboratory (Eppinger et al., 2011). The recorded SNP numbers in the two clusters are further in line with the numbers reported for serial patient-derived O157:H7 isolates that underwent short-term microevolutionary changes (Rusconi et al., 2016). Taken together, the phylogenomics data identified the laboratory derived NalR mutant strains as the source of the researcher’s life-threatening disease rather than a conceivable ingestion of unrelated STEC O157:H7 isolates circulating at the same time. The results also clearly support a simultaneous co-infection with laboratory-housed strains, which were the causative agents of previous O157:H7 outbreaks. Co-infections have been only observed rarely in STEC (Rivas et al., 1993; Gilmour et al., 2007; Cheung et al., 2020), and the potential impact on disease manifestation by such mixed infections of strains featuring distinct genome and virulence traits has not been evaluated.
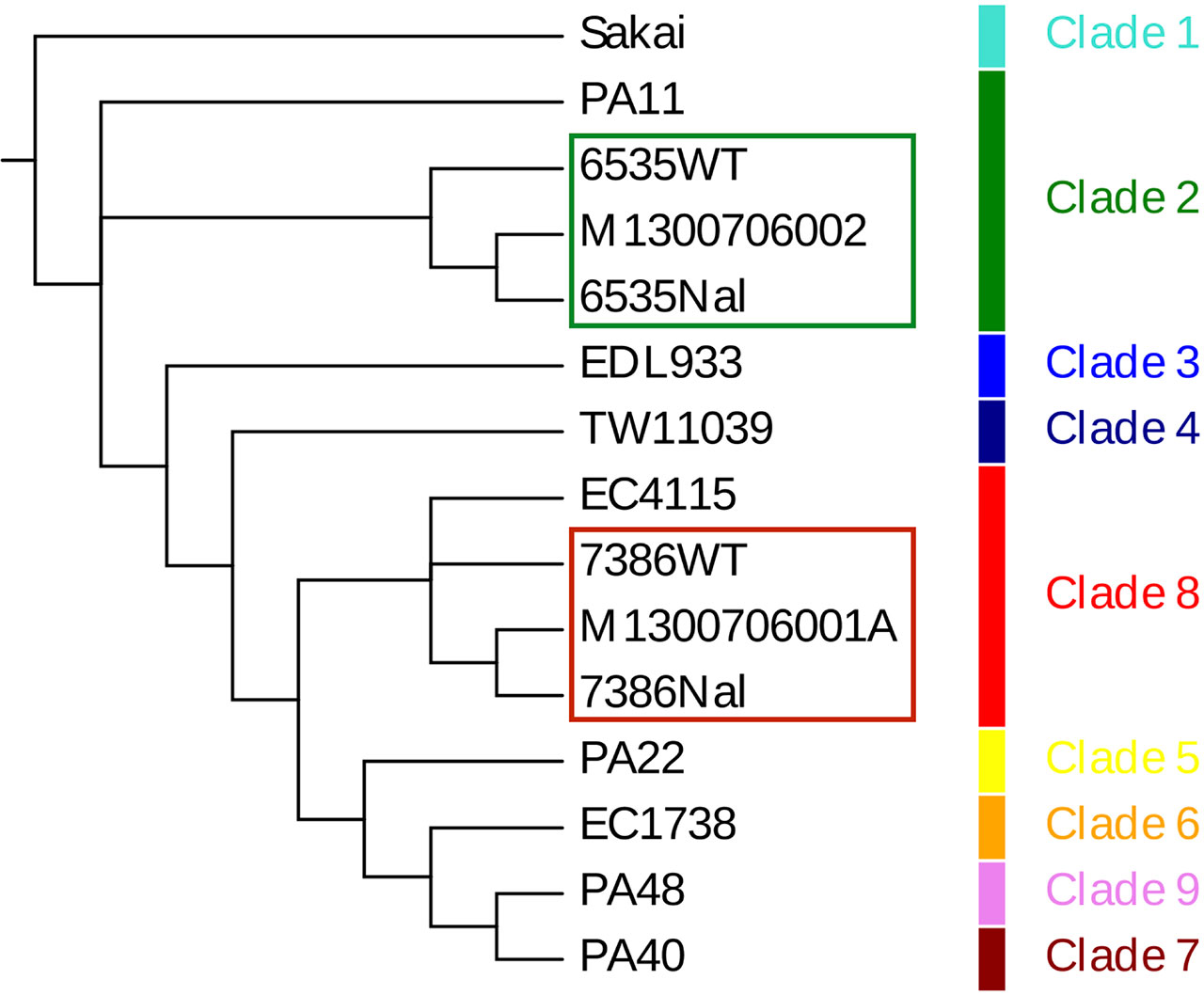
Figure 1 Whole genome phylogeny of representative EHEC O157:H7 strains Genomes of a total of 15 E. coli strains were aligned using Mugsy (Angiuoli and Salzberg, 2011) and included wt/NalR laboratory strains 7386 and 6535 and two clinical strains recovered from the patient and were complemented by representative isolates in the stepwise evolutionary model of EHEC O157:H7 (Feng et al., 2007; Manning et al., 2008).The phylogenetic tree was constructed using RAxML (Stamatakis, 2014) with 100 bootstrap replicates and decorated with the strain-associated metadata in Evolview (He Z. et al., 2016). Bootstrap values below 100 are shown in the tree.
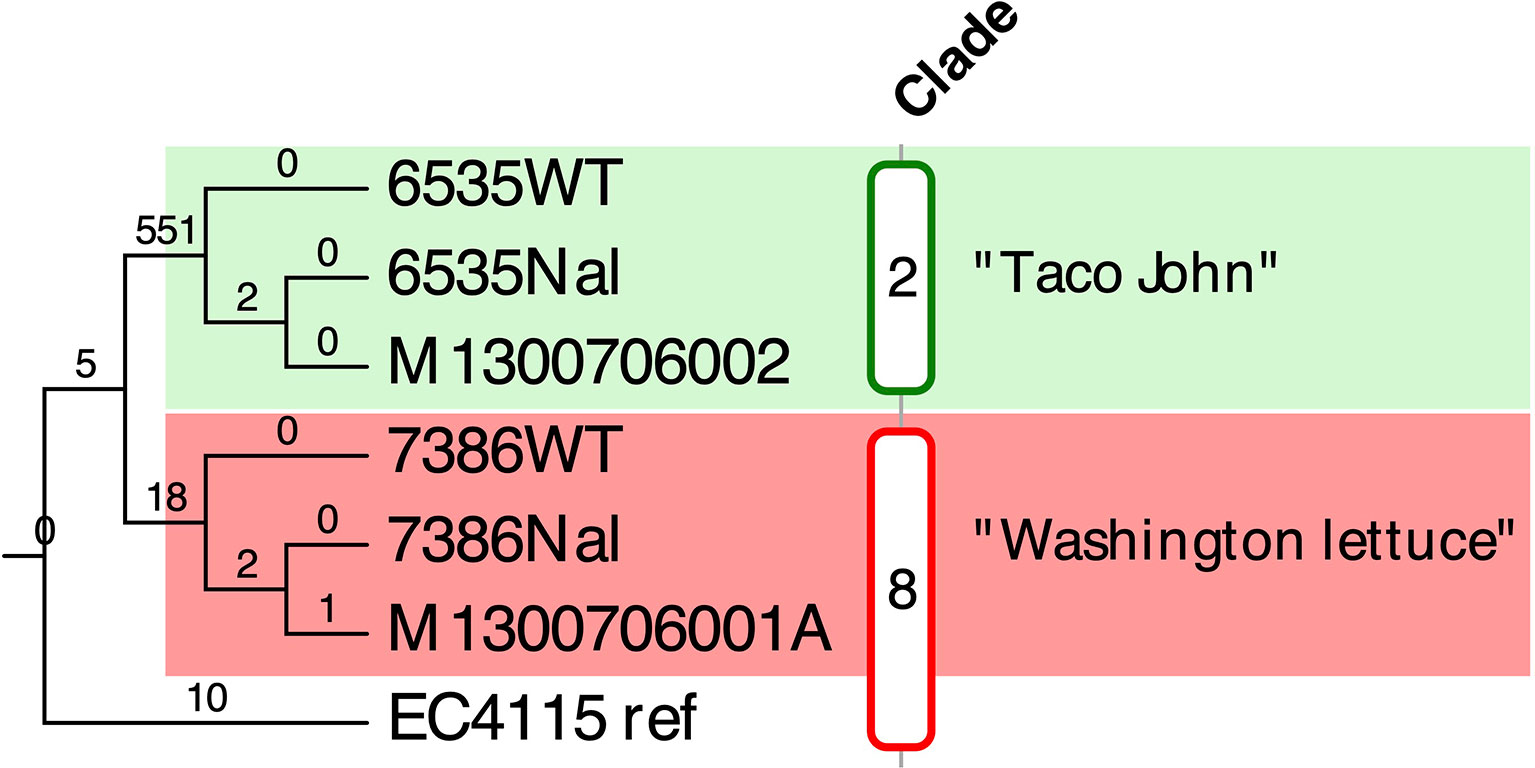
Figure 2 Maximum parsimony (MP) SNP-based tree of analyzed laboratory and clinical EHEC O157:H7 strains Comparison of the six genomes yielded a total of 588 SNPs of which 572 were parsimony informative. The tree shown is a majority-consensus tree of 100 equally parsimonious trees with a consistency index of 0.998 and was recovered using a heuristic search in Paup 4.0a163 (Wilgenbusch and Swofford, 2003). The phylogeny revealed two distinct clusters comprised of laboratory strains 7386 and 6535 and respective derived NalR mutants and clinical isolates recovered from the patient. The SNP tree unambiguously identified the two laboratory strains as source of this life threatening O157:H7 co-infection. As evidenced both patient recovered strains form tight clonal clusters with their respective laboratory progenitor strains and the tree topology unambiguously identified the laboratory strains as progenitor and thus source of the researchers’ infection. Only nodes with bootstrap values below 100 are listed.
Determination of Stx2a Production by ELISA and Stx2a Expression by qRT-PCR Following Exposure to the Antibiotics
We recorded production traits of Stx2a, the most potent allelic subtype associated with human disease (Tesh et al., 1993; Orth et al., 2007; Fuller et al., 2011), in response to MET and LEV in wt strains, laboratory-derived NalR mutants, and clinical isolates. For a comprehensive readout, we recorded both toxin transcript and protein levels under spontaneous (non-induced) and MMC-inducing conditions (Figure 3). Expression and production levels of the stx2a gene and Stx2 were measured by RT-qPCR (Figures 3A, B) and ELISA (Figures 3C, D), respectively. MMC is a potent Stx2 prophage-inducing agent that triggers toxin production via the SOS response mechanism (Raya and H’bert, 2009; Pacheco and Sperandio, 2012; Allué-Guardia et al., 2014). Bacterial counts (log CFU/ml) after 18 h of growth at 37°C in TSB medium alone were very similar among the six strains analyzed (Table 2). Statistically significant reductions in CFU/ml, compared to TSB, were observed in the presence of MET in 7386 wt, 7386 NalR, 6535 wt, and patient isolates M1300706001A and M1300706002 (Table 2). The addition of both MET and LEV to the cultures resulted in statistically significant reductions in patient-derived strain M1300706001A and in 7386 NalR compared to counts observed in TSB alone (Table 2). LEV had no significant effect on the reduction of CFU/ml in any strain compared to TSB (Table 2). Therefore, the reduction in bacterial growth observed in cultures with the combination of MET and LEV were primarily due to MET.
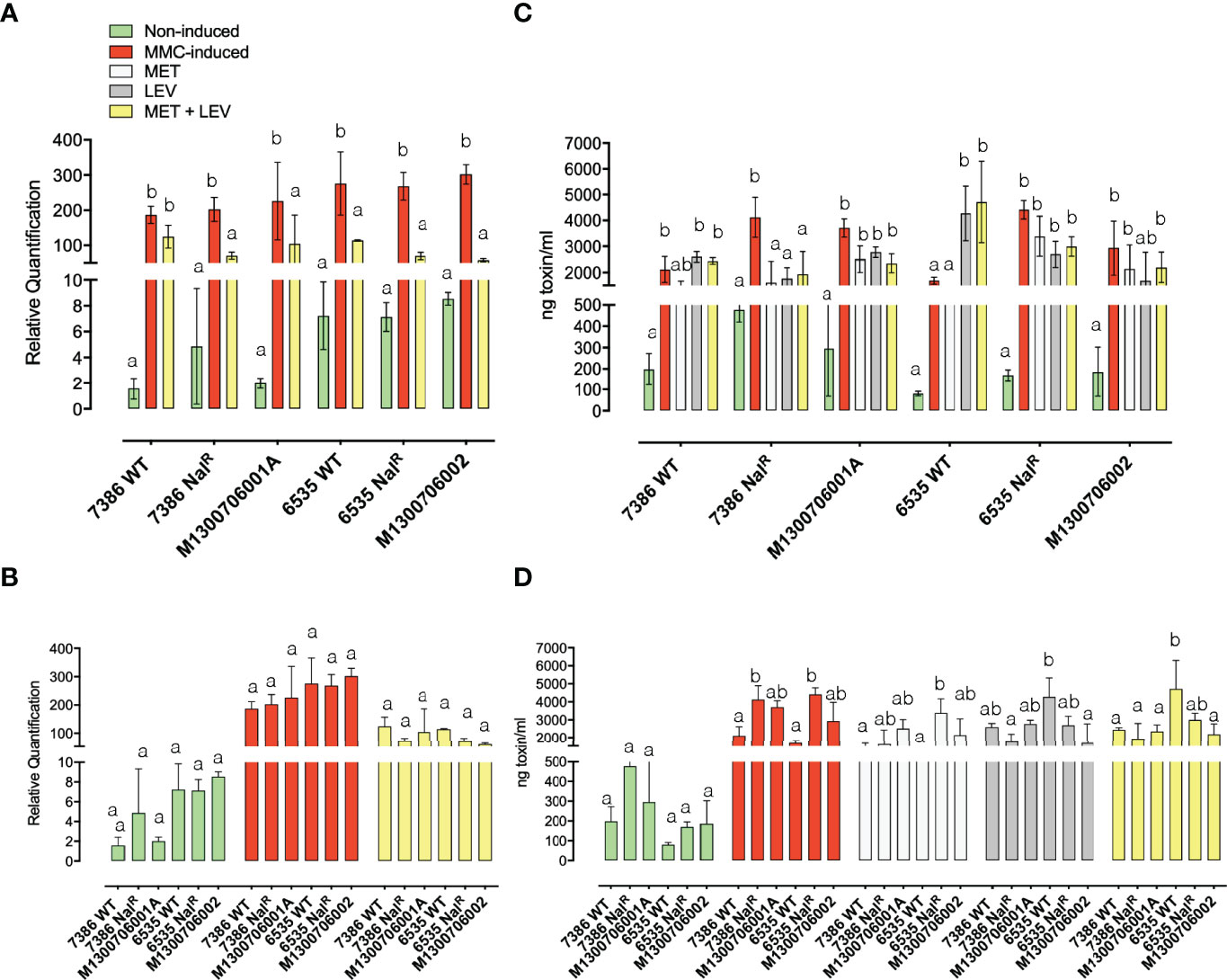
Figure 3 Stx-virulence phenotypes in response to hospital administered antibiotics Stx-production of the infection-associated laboratory and clinical isolates were recorded under non-induced, MMC-induced conditions and hospital administered antibiotics. Transcripts of stx2a (A, B) were measured by RT-qPCR using two biological and technical replicates and normalized against the endogenous gene tufA. Values are shown in relative quantitation (RQ), after using the comparative CT (ΔΔCT) method (Livak and Schmittgen, 2001). Stx2-production (C, D) was quantified by ELISA. Error bars depict standard deviation of two biological replicates. Statistical significance of the RT-qPCR and ELISA for each individual strain when comparing conditions (A, C), and for each condition when comparing strains is shown (B, D). Different letters among conditions in each strain (A, C) and among strains in each condition (B, D) indicate statistically significant differences.
Several biotic and abiotic cues can induce the Stx converting prophage complement in STEC hosts, including antibiotics (Pacheco and Sperandio, 2012). Therapeutic use of antibiotics for STEC infections is thus controversial, as certain antibiotics are known to induce Stx2 phages and consequently toxin production through SOS-dependent activation of the phage lytic cycle (Kimmitt et al., 2000; Wong et al., 2000; Zhang et al., 2000; McGannon et al., 2010). Elevated toxin levels and stx2a transcripts were observed when compared to spontaneous conditions after exposure to MET and LEV (Tables 3, 4). Significantly increased production of Stx2 was observed in each of the six strains analyzed with combined MET and LEV compared to TSB (p<0.05), except for 7386 NalR, which showed high levels of toxin production in the presence of TSB alone. The highest Stx2 production with combined MET and LEV was observed in 6535 wt (average 4717 ng toxin/ml) with significantly higher Stx2 production compared to 7386 wt, 7386 NalR, and both patient isolates (p<0.05) (Figure 3D). Significantly higher production of Stx2 was observed in 6535 wt compared to the 6335 NalR patient isolate M1300706002 (Table 3). The 6535 wt strain produced significantly less Stx2 under MET-induction when compared to the combination of the two antibiotics.
Stx2 production levels with exposure to MMC were higher than those induced by combined MET and LEV in the two NalR mutant strains and in the two patient isolates (Table 3), but statistically significantly increased Stx2 production was only observed in 7386 NalR. Lower Stx2 production by MMC was observed in both wt strains, with significantly lower production in 6535 wt compared to exposure to LEV and the combination of MET and LEV.
Stx2 transcript levels were examined only in the presence of LEV and MET combined, and not with the antibiotics individually (Figures 3A, B and Table 4). Results indicated that the induction effect of these antibiotics was not as pronounced as in samples treated with MMC. Results with MET+LEV treatments were not significantly different than treatment with TSB alone with the exception of strain 7386 wt. Another notable exception was that results with strain 7386 wt did not show a statistical difference between MMC and MET+LEV treatments.
When comparing stx2 expression versus Stx2 production results, a similar trend was observed in most strains. 7386 wt showed no significant differences in both expression and production of toxins in MMC versus MET+LEV, while the other strains showed significant differences in both toxin expression and production in MMC versus MET+LEV (Tables 3, 4). Host derived strains M1300706001A/7386-NalR and M1300706002/6535-NalR showed significant differences in stx2a gene expression under MMC versus MET+LEV conditions, but not in toxin production. This may indicate a delayed production of toxins in this strain; however, this would require further study. We note here a disconnection between transcript and Stx2 protein levels has been previously reported in O157:H7 strains, though the reason for this apparent lack of correlation remains to be elucidated (Leenanon et al., 2003; Neupane et al., 2011).
Conclusions
High-resolution genomic epidemiology techniques have been extensively used in outbreak investigations to identify the contaminated source and emergence of hypervirulent STEC lineages (Underwood et al., 2013; Amigo et al., 2015; Dallman et al., 2015a; Dallman et al., 2015b; Eppinger and Cebula, 2015). Assuring a timely and informed response in the control of microbial outbreaks is challenging, and techniques with high discriminatory power become of particular importance to distinguish outbreak isolates that form tight clonal complexes with only few genetic polymorphisms. Particularly, de novo SNP typing complements often surpass other more labor-intensive molecular typing schemes that have been developed for STEC pathogen populations over the last decades (Sadiq et al., 2014). Our investigation of this clinical STEC case identified a laboratory contamination with an Stx2a-positive O157:H7 strain mixture as the source of a severe human infection. PFGE in combination with SNP profiling allowed us to establish a “close” clonal relationship of the compared laboratory wt/NalR-mutant and patient strains reflecting the short-term microevolutionary changes in their genomes. The detected SNP numbers are in line with reports by us and other groups for serial outbreak isolates of O157:H7 (Dallman et al., 2015b; Rusconi et al., 2016; Dallman et al., 2021). Unfortunately, to date no effective treatment or prophylaxis for HUS is known (Goldwater and Bettelheim, 2012). Certain antibiotics are known to mobilize Stx2a-phages and ultimately cause toxin production, thus the therapeutic use of antibiotics remains highly controversial (Mead and Griffin, 1998; Rahal et al., 2015; Freedman et al., 2016). The treatment regimen of the patient provided us with the unique opportunity to assess the impact of the individual administered antibiotics or cocktails on the strains’ Stx production capabilities. In vitro assays showed that exposure to MET and LEV likely increased the pathogenic potential of the infective strains. In sublethal doses, these antibiotics elevated both toxin-transcript and -production levels in this clinical case, and in consequence may have exacerbated the symptoms and the severity of the disease. As demonstrated in this study, the integration of genome and virulence information is critical for outbreak investigations and improved risk assessment of STEC (Sadiq et al., 2014; Eppinger and Cebula, 2015), and our findings call for awareness of increased Stx production capabilities following the therapeutic treatment with antibiotics.
Accession Numbers
The sequencing datasets for all isolates analyzed in this study have been deposited in the Sequence Read Archive (SRA) and the Whole Genome Shotgun Repository at National Center for Biotechnology Information (NCBI) under BioProjects PRJNA407949 and PRJNA750123. Accessions for reads, assembled, and annotated draft genomes, along with strain-associated metadata are provided in Supplementary Table 2.
Data Availability Statement
The datasets presented in this study can be found in online repositories. The names of the repository/repositories and accession number(s) can be found in the article/supplementary material.
Author Contributions
Conceived and designed the experiments, ME and PF. Analyzed the data, ME, SA, AA-G, LB, JG, and PF. Sequence assemblies and submissions to the NCBI repositories, AK and ME. Wrote the paper, ME, SA, and PF. All authors contributed to the article and approved the submitted version.
Funding
Research reported in this publication was supported by the National Institute of General Medical Sciences of the National Institutes of Health under Award Numbers [SC1GM135110] and the US Department of Homeland Security [2014-ST-062-000058] to ME. The content is solely the responsibility of the authors and does not necessarily represent the official views of the National Institutes of Health or the US Department of Homeland Security. The mention of a trade name, proprietary product, or specific equipment does not constitute a guarantee or warranty by the USDA and does not imply approval to the exclusion of other products that might be suitable. The USDA and FDA are an equal opportunity employer and provider. SA co-prepared this article in her personal capacity before joining FDA. The opinions expressed in this article are the author’s own and do not reflect the view of the FDA, the Department of Health and Human Services or the United States government.
Author Disclaimer
The portion of this manuscript prepared by SA was done in her personal capacity before joining FDA. The opinions expressed in this article are the author’s own and do not reflect the view of the FDA, the Department of Health and Human Services or the United States government.
Conflict of Interest
The authors declare that the research was conducted in the absence of any commercial or financial relationships that could be construed as a potential conflict of interest.
Publisher’s Note
All claims expressed in this article are solely those of the authors and do not necessarily represent those of their affiliated organizations, or those of the publisher, the editors and the reviewers. Any product that may be evaluated in this article, or claim that may be made by its manufacturer, is not guaranteed or endorsed by the publisher.
Acknowledgments
This work received support by the South Texas Center of Emerging Infectious Diseases (STCEID), the Department of Molecular Microbiology and Immunology (MMI) and the High-Performance Computing Cluster operated by University Technology Solutions at the University of Texas at San Antonio. We thank Sara SK Koenig for critically reading the manuscript.
Supplementary Material
The Supplementary Material for this article can be found online at: https://www.frontiersin.org/articles/10.3389/fcimb.2022.888568/full#supplementary-material
Supplementary Table 1 | PCR-primers and -cycling conditions.
Supplementary Table 2 | Genomes and associated metadata of strains used in this study.
Supplementary Table 3 | Virulence profiles of strains 7386 and 6535.
Supplementary Table 4 | Catalogued core genome SNPs.
References
Afgan, E., Baker, D., Batut, B., Van Den Beek, M., Bouvier, D., Cech, M., et al. (2018). The Galaxy Platform for Accessible, Reproducible and Collaborative Biomedical Analyses: 2018 Update. Nucleic Acids Res. 46, W537–W544. doi: 10.1093/nar/gky379
Alcock, B. P., Raphenya, A. R., Lau, T. T. Y., Tsang, K. K., Bouchard, M., Edalatmand, A., et al. (2020). CARD 2020: Antibiotic Resistome Surveillance With the Comprehensive Antibiotic Resistance Database. Nucleic Acids Res. 48, D517–D525. doi: 10.1093/nar/gkz935
Allué-Guardia, A., Martinez-Castillo, A., Muniesa, M. (2014). Persistence of Infectious Shiga Toxin-Encoding Bacteriophages After Disinfection Treatments. Appl. Environ. Microbiol. 80, 2142–2149. doi: 10.1128/AEM.04006-13
Altschul, S. F., Gish, W., Miller, W., Myers, E. W., Lipman, D. J. (1990). Basic Local Alignment Search Tool. J. Mol. Biol. 215, 403–410. doi: 10.1016/S0022-2836(05)80360-2
Amigo, N., Mercado, E., Bentancor, A., Singh, P., Vilte, D., Gerhardt, E., et al. (2015). Clade 8 and Clade 6 Strains of Escherichia Coli O157:H7 From Cattle in Argentina Have Hypervirulent-Like Phenotypes. PloS One 10, e0127710. doi: 10.1371/journal.pone.0127710
Andrews, J. M. (2001). Determination of Minimum Inhibitory Concentrations. J. Antimicrob. Chemother. 48, Suppl. doi: 10.1093/jac/48.suppl_1.5
Angiuoli, S. V., Salzberg, S. L. (2011). Mugsy: Fast Multiple Alignment of Closely Related Whole Genomes. Bioinformatics 27, 334–342. doi: 10.1093/bioinformatics/btq665
Armstrong, C. M., Ruth, L. E., Capobianco, J. S., Strobaugh, T. P., Jr, Rubio, F. M., Gehring, A. G. (2018). Detection of Shiga Toxin 2 Produced by Escherichia Coli in Foods Using a Novel AlphaLISA. Toxins 10 (11), E422. doi: 10.3390/toxins10110422
Arndt, D., Grant, J. R., Marcu, A., Sajed, T., Pon, A., Liang, Y., et al. (2016). PHASTER: A Better, Faster Version of the PHAST Phage Search Tool. Nucleic Acids Res. 44, W16–W21. doi: 10.1093/nar/gkw387
Arndt, D., Marcu, A., Liang, Y., Wishart, D. S. (2017). PHAST, PHASTER and PHASTEST: Tools for Finding Prophage in Bacterial Genomes. Brief Bioinform. 20, 1560–1567. doi: 10.1093/bib/bbx121
Bai, X., Fu, S., Zhang, J., Fan, R., Xu, Y., Sun, H., et al. (2018). Identification and Pathogenomic Analysis of an Escherichia Coli Strain Producing a Novel Shiga Toxin 2 Subtype. Sci. Rep. 8, 6756. doi: 10.1038/s41598-018-25233-x
Bai, X., Scheutz, F., Dahlgren, H. M., Hedenström, I., Jernberg, C. (2021). Characterization of Clinical Escherichia Coli Strains Producing a Novel Shiga Toxin 2 Subtype in Sweden and Denmark. Microorganisms 9 (11), 2374. doi: 10.3390/microorganisms9112374
Baranzoni, G. M., Fratamico, P. M., Gangiredla, J., Patel, I., Bagi, L. K., Delannoy, S., et al. (2016). Characterization of Shiga Toxin Subtypes and Virulence Genes in Porcine Shiga Toxin-Producing Escherichia Coli. Front. Microbiol. 7. doi: 10.3389/fmicb.2016.00574
Bergan, J., Dyve Lingelem, A. B., Simm, R., Skotland, T., Sandvig, K. (2012). Shiga Toxins. Toxicon 60, 1085–1107. doi: 10.1016/j.toxicon.2012.07.016
Bielaszewska, M., Friedrich, A. W., Aldick, T., Schurk-Bulgrin, R., Karch, H. (2006). Shiga Toxin Activatable by Intestinal Mucus in Escherichia Coli Isolated From Humans: Predictor for a Severe Clinical Outcome. Clin. Infect. Dis. 43, 1160–1167. doi: 10.1086/508195
Bielaszewska, M., Idelevich, E. A., Zhang, W., Bauwens, A., Schaumburg, F., Mellmann, A., et al. (2012). Effects of Antibiotics on Shiga Toxin 2 Production and Bacteriophage Induction by Epidemic Escherichia Coli O104:H4 Strain. Antimicrob. Agents Chemother. 56, 3277–3282. doi: 10.1128/AAC.06315-11
Bono, J. L. (2009). Genotyping Escherichia Coli O157:H7 for its Ability to Cause Disease in Humans. Curr. Protoc. Microbiol. 5, 5A.3. doi: 10.1002/9780471729259.mc05a03s14
Booth, L., Rowe, B. (1993). Possible Occupational Acquisition of Escherichia Coli O157 Infection. Lancet 342, 1298–1299. doi: 10.1016/0140-6736(93)92388-A
Burnens, A. P., Zbinden, R., Kaempf, L., Heinzer, I., Nicolet, J. (1993). A Case of Laboratory-Acquired Infection With Escherichia Coli O157:H7. Zentbl Bakteriol 279, 512–517. doi: 10.1016/S0934-8840(11)80423-8
Carattoli, A., Zankari, E., Garcia-Fernandez, A., Voldby Larsen, M., Lund, O., Villa, L., et al. (2014). In Silico Detection and Typing of Plasmids Using PlasmidFinder and Plasmid Multilocus Sequence Typing. Antimicrob. Agents Chemother. 58, 3895–3903. doi: 10.1128/AAC.02412-14
Chen, L., Zheng, D., Liu, B., Yang, J., Jin, Q. (2016). VFDB 2016: Hierarchical and Refined Dataset for Big Data Analysis–10 Years on. Nucleic Acids Res. 44, D694–D697. doi: 10.1093/nar/gkv1239
Cheung, D. A., Nicholson, A., Butterfield, T. R., Dacosta, M. (2020). Prevalence, Co-Infection and Antibiotic Resistance of Escherichia Coli From Blood and Urine Samples at a Hospital in Jamaica. J. Infect. Dev. Ctries 14, 146–152. doi: 10.3855/jidc.11361
Chin, C. S., Alexander, D. H., Marks, P., Klammer, A. A., Drake, J., Heiner, C., et al. (2013). Nonhybrid, Finished Microbial Genome Assemblies From Long-Read SMRT Sequencing Data. Nat. Methods 10, 563–569. doi: 10.1038/nmeth.2474
Coia, J. E. (1998). Nosocomial and Laboratory-Acquired Infection With Escherichia Coli O157. J. Hosp Infect. 40, 107–113. doi: 10.1016/S0195-6701(98)90089-5
Cowley, L. A., Dallman, T. J., Fitzgerald, S., Irvine, N., Rooney, P. J., Mcateer, S. P., et al. (2016). Short-Term Evolution of Shiga Toxin-Producing Escherichia Coli O157:H7 Between Two Food-Borne Outbreaks. Microbial Genomics 2 (9), e000084. doi: 10.1099/mgen.0.000084
Dallman, T. J., Ashton, P. M., Byrne, L., Perry, N. T., Petrovska, L., Ellis, R. J., et al. (2015a). Applying Phylogenomics to Understand the Emergence of Shiga-Toxin-Producing Escherichia Coli O157:H7 Strains Causing Severe Human Disease in the UK. Microbial Genomics 1(3), e000029. doi: 10.1099/mgen.0.000029
Dallman, T. J., Byrne, L., Ashton, P. M., Cowley, L. A., Perry, N. T., Adak, G., et al. (2015b). Whole-Genome Sequencing for National Surveillance of Shiga Toxin-Producing Escherichia Coli O157. Clin. Infect. Dis. 61, 305–312. doi: 10.1093/cid/civ318
Dallman, T. J., Greig, D. R., Gharbia, S. E., Jenkins, C. (2021). Phylogenetic Structure of Shiga Toxin-Producing Escherichia Coli O157:H7 From Sub-Lineage to SNPs. Microb. Genom 7. doi: 10.1099/mgen.0.000544
Delcher, A. L., Salzberg, S. L., Phillippy, A. M. (2003). Using MUMmer to Identify Similar Regions in Large Sequence Sets. Curr. Protoc. Bioinf. 10 (10.13). doi: 10.1002/0471250953.bi1003s00
EFSA BIOHAZ Panel, Koutsoumanis, K., Allende, A., Alvarez-Ordóñez, A., Bover-Cid, S., Chemaly, M., et al. (2020). Scientific Opinion on the Pathogenicity Assessment of Shiga Toxin-Producing Escherichia Coli (STEC) and the Public Health Risk Posed by Contamination of Food With STEC. EFSA J. 18 (1), 5967, 105. doi: 10.2903/j.efsa.2020.5967
Eppinger, M., Cebula, T. A. (2015). Future Perspectives, Applications and Challenges of Genomic Epidemiology Studies for Food-Borne Pathogens: A Case Study of Enterohemorrhagic Escherichia Coli (EHEC) of the O157:H7 Serotype. Gut Microbes 6, 194–201. doi: 10.4161/19490976.2014.969979
Eppinger, M., Mammel, M. K., Leclerc, J. E., Ravel, J., Cebula, T. A. (2011). Genomic Anatomy of Escherichia Coli O157:H7 Outbreaks. Proc. Natl. Acad. Sci. USA 108, 20142–20147. doi: 10.1073/pnas.1107176108
Eppinger, M., Pearson, T., Koenig, S. S., Pearson, O., Hicks, N., Agrawal, S., et al. (2014). Genomic Epidemiology of the Haitian Cholera Outbreak: A Single Introduction Followed by Rapid, Extensive, and Continued Spread Characterized the Onset of the Epidemic. MBio 5, e01721. doi: 10.1128/mBio.01721-14
Eppinger, M., Worsham, P. L., Nikolich, M. P., Riley, D. R., Sebastian, Y., Mou, S., et al. (2010). Genome Sequence of the Deep-Rooted Yersinia Pestis Strain Angola Reveals New Insights Into the Evolution and Pangenome of the Plague Bacterium. J. Bacteriol. 192, 1685–1699. doi: 10.1128/JB.01518-09
Fabrega, A., Madurga, S., Giralt, E., Vila, J. (2009). Mechanism of Action of and Resistance to Quinolones. Microb. Biotechnol. 2, 40–61. doi: 10.1111/j.1751-7915.2008.00063.x
Fadlallah, S. M., Rahal, E. A., Sabra, A., Kissoyan, K. A., Matar, G. M. (2015). Effect of Rifampicin and Gentamicin on Shiga Toxin 2 Expression Level and the SOS Response in Escherichia Coli O104:H4. Foodborne Pathog. Dis. 12, 47–55. doi: 10.1089/fpd.2014.1824
Feng, P. C., Monday, S. R., Lacher, D. W., Allison, L., Siitonen, A., Keys, C., et al. (2007). Genetic Diversity Among Clonal Lineages Within Escherichia Coli O157:H7 Stepwise Evolutionary Model. Emerg. Infect. Dis. 13, 1701–1706. doi: 10.3201/eid1311.070381
Feng, J., Xu, K., Shi, K., Xu, L., Liu, L., Wang, F., et al. (2022). Incidence and Cost of Haemolytic Uraemic Syndrome in Urban China: A National Population-Based Analysis. BMC Nephrol 23 (1), 122. doi: 10.1186/s12882-022-02746-2
Fitzgerald, C., Patrick, M., Gonzalez, A., Akin, J., Polage, C. R., Wymore, K., et al. (2016). Multicenter Evaluation of Clinical Diagnostic Methods for Detection and Isolation of Campylobacter Spp. From Stool. J. Clin. Microbiol. 54, 1209–1215. doi: 10.1128/JCM.01925-15
Franz, E., Delaquis, P., Morabito, S., Beutin, L., Gobius, K., Rasko, D. A., et al. (2014). Exploiting the Explosion of Information Associated With Whole Genome Sequencing to Tackle Shiga Toxin-Producing Escherichia Coli (STEC) in Global Food Production Systems. Int. J. Food Microbiol 187, 57–72. doi: 10.1016/j.ijfoodmicro.2014.07.002
Freedman, S. B., Xie, J., Neufeld, M. S., Hamilton, W. L., Hartling, L., Tarr, P. I., et al. (2016). Shiga Toxin-Producing Escherichia Coli Infection, Antibiotics, and Risk of Developing Hemolytic Uremic Syndrome: A Meta-Analysis. Clin. Infect. Dis. 62, 1251–1258. doi: 10.1093/cid/ciw099
Friedrich, A. W., Bielaszewska, M., Zhang, W. L., Pulz, M., Kuczius, T., Ammon, A., et al. (2002). Escherichia Coli Harboring Shiga Toxin 2 Gene Variants: Frequency and Association With Clinical Symptoms. J. Infect. Dis. 185, 74–84. doi: 10.1086/338115
Fuller, C. A., Pellino, C. A., Flagler, M. J., Strasser, J. E., Weiss, A. A. (2011). Shiga Toxin Subtypes Display Dramatic Differences in Potency. Infect. Immun. 79, 1329–1337. doi: 10.1128/IAI.01182-10
Garrison, E., Marth, G. (2012) Haplotype-Based Variant Detection From Short-Read Sequencing. Available at: http://adsabs.harvard.edu/abs/2012arXiv1207.3907G (Accessed July 1, 2012).
Gerritzen, A., Wittke, J.-W., Wolff, D. (2011). Rapid and Sensitive Detection of Shiga Toxin-Producing Escherichia Coli Directly From Stool Samples by Real-Time PCR in Comparison to Culture, Enzyme Immunoassay and Vero Cell Cytotoxicity Assay. Clin. Lab. 57, 993–998.
Gilmour, M. W., Tabor, H., Wang, G., Clark, C. G., Tracz, D. M., Olson, A. B., et al. (2007). Isolation and Genetic Characterization of a Coinfection of non-O157 Shiga Toxin-Producing Escherichia Coli. J. Clin. Microbiol. 45, 3771–3773. doi: 10.1128/JCM.01125-07
Gobert, A. P., Vareille, M., Glasser, A. L., Hindre, T., De Sablet, T., Martin, C. (2007). Shiga Toxin Produced by Enterohemorrhagic Escherichia Coli Inhibits PI3K/NF-kappaB Signaling Pathway in Globotriaosylceramide-3-Negative Human Intestinal Epithelial Cells. J. Immunol. 178, 8168–8174. doi: 10.4049/jimmunol.178.12.8168
Goecks, J., Nekrutenko, A., Taylor, J., Galaxy, T. (2010). Galaxy: A Comprehensive Approach for Supporting Accessible, Reproducible, and Transparent Computational Research in the Life Sciences. Genome Biol. 11, R86. doi: 10.1186/gb-2010-11-8-r86
Goldwater, P. N., Bettelheim, K. A. (2012). Treatment of Enterohemorrhagic Escherichia Coli (EHEC) Infection and Hemolytic Uremic Syndrome (HUS). BMC Med. 10, 12. doi: 10.1186/1741-7015-10-12
Gould, L. H., Mody, R. K., Ong, K. L., Clogher, P., Cronquist, A. B., Garman, K. N., et al. (2013). Increased Recognition of non-O157 Shiga Toxin-Producing Escherichia Coli Infections in the United States During 2000-2010: Epidemiologic Features and Comparison With E. Coli O157 Infections. Foodborne Pathog. Dis. 10, 453–460. doi: 10.1089/fpd.2012.1401
Grif, K., Dierich, M. P., Karch, H., Allerberger, F. (1998). ). Strain-Specific Differences in the Amount of Shiga Toxin Released From Enterohemorrhagic Escherichia Coli O157 Following Exposure to Subinhibitory Concentrations of Antimicrobial Agents. Eur. J. Clin. Microbiol. Infect. Dis. 17, 761–766. doi: 10.1007/s100960050181
Hartzell, A., Chen, C., Lewis, C., Liu, K., Reynolds, S., Dudley, E. G. (2011). Escherichia Coli O157:H7 of Genotype Lineage-Specific Polymorphism Assay 211111 and Clade 8 are Common Clinical Isolates Within Pennsylvania. Foodborne Pathog. Dis. 8, 763–768. doi: 10.1089/fpd.2010.0762
He, Y., Kong, Q., Patfield, S., Skinner, C., Rasooly, R. (2016). A New Immunoassay for Detecting All Subtyped of Shiga Toxins Produced by Shiga Toxin-Producing E. Coli in Ground Beef. PloS One 11 (1), e0148092. doi: 10.1371/journal.pone.0148092
He, Z., Zhang, H., Gao, S., Lercher, M. J., Chen, W. H., Hu, S. (2016). Evolview V2: An Online Visualization and Management Tool for Customized and Annotated Phylogenetic Trees. Nucleic Acids Res. 44, W236–W241. doi: 10.1093/nar/gkw370
Holmes, A., Allison, L., Ward, M., Dallman, T. J., Clark, R., Fawkes, A., et al. (2015). Utility of Whole-Genome Sequencing of Escherichia Coli O157 for Outbreak Detection and Epidemiological Surveillance. J. Clin. Microbiol. 53, 3565–3573. doi: 10.1128/JCM.01066-15
Hull, A. E., Acheson, D. W., Echeverria, P., Donohue-Rolfe, A., Keusch, G. T. (1993). Mitomycin Immunoblot Colony Assay for Detection of Shiga-Like Toxin-Producing Escherichia Coli in Fecal Samples: Comparison With DNA Probes. J. Clin. Microbiol. 31, 1167–1172. doi: 10.1128/jcm.31.5.1167-1172.1993
Jenkins, C., Dallman, T. J., Launders, N., Willis, C., Byrne, L., Jorgensen, F., et al. (2015). Public Health Investigation of Two Outbreaks of Shiga Toxin-Producing Escherichia Coli O157 Associated With Consumption of Watercress. Appl. Environ. Microbiol. 81, 3946–3952. doi: 10.1128/AEM.04188-14
Joensen, K. G., Scheutz, F., Lund, O., Hasman, H., Kaas, R. S., Nielsen, E. M., et al. (2014). Real-Time Whole-Genome Sequencing for Routine Typing, Surveillance, and Outbreak Detection of Verotoxigenic Escherichia Coli. J. Clin. Microbiol. 52, 1501–1510. doi: 10.1128/JCM.03617-13
Joensen, K. G., Tetzschner, A. M., Iguchi, A., Aarestrup, F. M., Scheutz, F. (2015). Rapid and Easy in Silico Serotyping of Escherichia Coli Isolates by Use of Whole-Genome Sequencing Data. J. Clin. Microbiol. 53, 2410–2426. doi: 10.1128/JCM.00008-15
Jung, W. K., Bono, J. L., Clawson, M. L., Leopold, S. R., Shringi, S., Besser, T. E. (2013). Lineage and Genogroup-Defining Single Nucleotide Polymorphisms of Escherichia Coli O157:H7. Appl. Environ. Microbiol. 79, 7036–7041. doi: 10.1128/AEM.02173-13
Kearse, M., Moir, R., Wilson, A., Stones-Havas, S., Cheung, M., Sturrock, S., et al. (2012). Geneious Basic: An Integrated and Extendable Desktop Software Platform for the Organization and Analysis of Sequence Data. Bioinformatics 28, 1647–1649. doi: 10.1093/bioinformatics/bts199
Kimmitt, P. T., Harwood, C. R., Barer, M. R. (2000). Toxin Gene Expression by Shiga Toxin-Producing Escherichia Coli: The Role of Antibiotics and the Bacterial SOS Response. Emerg. Infect. Dis. 6, 458–465. doi: 10.3201/eid0605.000503
Kleinheinz, K. A., Joensen, K. G., Larsen, M. V. (2014). Applying the ResFinder and VirulenceFinder Web-Services for Easy Identification of Acquired Antibiotic Resistance and E. Coli Virulence Genes in Bacteriophage and Prophage Nucleotide Sequences. Bacteriophage 4, e27943. doi: 10.4161/bact.27943
Koren, S., Walenz, B. P., Berlin, K., Miller, J. R., Bergman, N. H., Phillippy, A. M. (2017). Canu: Scalable and Accurate Long-Read Assembly via Adaptive K-Mer Weighting and Repeat Separation. Genome Res. 27, 722–736. doi: 10.1101/gr.215087.116
Krüger, A., Lucchesi, P. M. (2015). Shiga Toxins and Stx Phages: Highly Diverse Entities. Microbiology 161, 451–462. doi: 10.1099/mic.0.000003
Krüger, A., Lucchesi, P. M., Parma, A. E. (2011). Verotoxins in Bovine and Meat Verotoxin-Producing Escherichia Coli Isolates: Type, Number of Variants, and Relationship to Cytotoxicity. Appl. Environ. Microbiol. 77, 73–79. doi: 10.1128/AEM.01445-10
Lacher, D. W., Steinsland, H., Whittam, T. S. (2006). Allelic Subtyping of the Intimin Locus (Eae) of Pathogenic Escherichia Coli by Fluorescent RFLP. FEMS Microbiol. Lett. 261, 80–87. doi: 10.1111/j.1574-6968.2006.00328.x
Lee, K. I., Morita-Ishihara, T., Iyoda, S., Ogura, Y., Hayashi, T., Sekizuka, T., et al. (2017). A Geographically Widespread Outbreak Investigation and Development of a Rapid Screening Method Using Whole Genome Sequences of Enterohemorrhagic Escherichia Coli O121. Front. Microbiol. 8, 701. doi: 10.3389/fmicb.2017.00701
Leenanon, B., Elhanafi, D., Drake, M. A. (2003). Acid Adaptation and Starvation Effects on Shiga Toxin Production by Escherichia Coli O157:H7. J. Food Prot 66, 970–977. doi: 10.4315/0362-028X-66.6.970
Leopold, S. R., Magrini, V., Holt, N. J., Shaikh, N., Mardis, E. R., Cagno, J., et al. (2009). A Precise Reconstruction of the Emergence and Constrained Radiations of Escherichia Coli O157 Portrayed by Backbone Concatenomic Analysis. Proc. Natl. Acad. Sci. U. S. A. 106, 8713–8718. doi: 10.1073/pnas.0812949106
Li, H., Durbin, R. (2009). Fast and Accurate Short Read Alignment With Burrows-Wheeler Transform. Bioinformatics 25, 1754–1760. doi: 10.1093/bioinformatics/btp324
Livak, K. J., Schmittgen, T. D. (2001). Analysis of Relative Gene Expression Data Using Real-Time Quantitative PCR and the 2(-Delta Delta C(T)) Method. Methods 25, 402–408. doi: 10.1006/meth.2001.1262
Luo, H, Gao, F. (2019). DoriC 10.0: An Updated Database of Replication Origins in Prokaryotic Genomes Including Chromosomes and Plasmids. Nucleic Acids Res. 47 (D1), D74–D77. doi: 10.1093/nar/gky1014
Lupolova, N., Dallman, T. J., Matthews, L., Bono, J. L., Gally, D. L. (2016). Support Vector Machine Applied to Predict the Zoonotic Potential of E. Coli O157 Cattle Isolates. Proc. Natl. Acad. Sci. U.S.A. 113, 11312–11317. doi: 10.1073/pnas.1606567113
Maddison, W. P., Maddison, D. R. (2021) Mesquite: A Modular System for Evolutionary Analysis. Version 3.70. Available at: http://www.mesquiteproject.org.
Mandell, G. L., Bennett, J. E., Dolin, R. (2005). Principles and Practice of Infectious Diseases. 6th Ed (Churchill Livingston, New York: Elsevier).
Manning, S. D., Motiwala, A. S., Springman, A. C., Qi, W., Lacher, D. W., Ouellette, L. M., et al. (2008). Variation in Virulence Among Clades of Escherichia Coli O157:H7 Associated With Disease Outbreaks. Proc. Natl. Acad. Sci. U.S.A. 105, 4868–4873. doi: 10.1073/pnas.0710834105
McGannon, C. M., Fuller, C. A., Weiss, A. A. (2010). Different Classes of Antibiotics Differentially Influence Shiga Toxin Production. Antimicrob. Agents Chemother. 54, 3790–3798. doi: 10.1128/AAC.01783-09
Mead, P. S., Griffin, P. M. (1998). Escherichia Coli O157:H7. Lancet 352, 1207–1212. doi: 10.1016/S0140-6736(98)01267-7
Melton-Celsa, A. R. (2014). Shiga Toxin (Stx) Classification, Structure, and Function. Microbiol. Spectr. 2 (4), EHEC–0024-2013. doi: 10.1128/microbiolspec.EHEC-0024-2013
Mody, RK, Hoekstra, RM, Scott, MK, Dunn, J, Smith, K, Tobin-D'Angelo, M, et al (2021). Risk of Hemolytic Uremic Syndrome Related to Treatment of Escherichia coli O157 Infection with Different Antimicrobial Classes. Microorganisms 9 (9), 1997. doi: 10.3390/microorganisms9091997
Mohsin, M., Guenther, S., Schierack, P., Tedin, K., Wieler, L. H. (2015). Probiotic Escherichia Coli Nissle 1917 Reduces Growth, Shiga Toxin Expression, Release and Thus Cytotoxicity of Enterohemorrhagic Escherichia Coli. Int. J. Med. Microbiol. 305, 20–26. doi: 10.1016/j.ijmm.2014.10.003
Nassar, F. J., Rahal, E. A., Sabra, A., Matar, G. M. (2013). ). Effects of Subinhibitory Concentrations of Antimicrobial Agents on Escherichia Coli O157:H7 Shiga Toxin Release and Role of the SOS Response. Foodborne Pathog. Dis. 10 (9), 805–812. doi: 10.1089/fpd.2013.1510
National Advisory Committee on Microbiological Criteria for Foods (NACMCF) (2019). Response to Questions Posed by the Food and Drug Administration Regarding Virulence Factors and Attributes That Define Foodborne Shiga Toxin-Producing Escherichia Coli (STEC) as Severe Human Pathogens. J. Food Prot 82, 724–767. doi: 10.4315/0362-028X.JFP-18-479
Neupane, M., Abu-Ali, G. S., Mitra, A., Lacher, D. W., Manning, S. D., Riordan, J. T. (2011). Shiga Toxin 2 Overexpression in Escherichia Coli O157:H7 Strains Associated With Severe Human Disease. Microb. Pathog. 51, 466–470. doi: 10.1016/j.micpath.2011.07.009
Nicholson, T. L., Waack, U., Anderson, T. K., Bayles, D. O., Zaia, S. R., Goertz, I., et al. (2020). Comparative Virulence and Genomic Analysis of Streptococcus Suis Isolates. Front. Microbiol. 11, 620843. doi: 10.3389/fmicb.2020.620843
Nyong, E. C., Zaia, S. R., Allué-Guardia, A., Rodriguez, A. L., Irion-Byrd, Z., Koenig, S. S. K., et al. (2020). Pathogenomes of Atypical non-Shigatoxigenic Escherichia Coli NSF/SF O157:H7/NM: Comprehensive Phylogenomic Analysis Using Closed Genomes. Front. Microbiol. 11, 619. doi: 10.3389/fmicb.2020.00619
Ogura, Y., Mondal, S. I., Islam, M. R., Mako, T., Arisawa, K., Katsura, K., et al. (2015). The Shiga Toxin 2 Production Level in Enterohemorrhagic Escherichia Coli O157:H7 is Correlated With the Subtypes of Toxin-Encoding Phage. Sci. Rep. 5, 16663. doi: 10.1038/srep16663
Operario, D. J., Moonah, S., Houpt, E. (2014). Hemolytic Uremic Syndrome Following Infection With O111 Shiga Toxin-Producing Escherichia Coli Revealed Through Molecular Diagnostics. J. Clin. Microbiol. 52, 1003–1005. doi: 10.1128/JCM.02855-13
Orth, D., Grif, K., Khan, A. B., Naim, A., Dierich, M. P., Wurzner, R. (2007). The Shiga Toxin Genotype Rather Than the Amount of Shiga Toxin or the Cytotoxicity of Shiga Toxin In Vitro Correlates With the Appearance of the Hemolytic Uremic Syndrome. Diagn. Microbiol. Infect. Dis. 59, 235–242. doi: 10.1016/j.diagmicrobio.2007.04.013
Pacheco, A. R., Sperandio, V. (2012). Shiga Toxin in Enterohemorrhagic E. Coli: Regulation and Novel Anti-Virulence Strategies. Front. Cell Infect. Microbiol. 2, 81. doi: 10.3389/fcimb.2012.00081
Panos, G. Z., Betsi, G. I., Falagas, M. E. (2006). Systematic Review: Are Antibiotics Detrimental or Beneficial for the Treatment of Patients With Escherichia Coli O157:H7 Infection? Aliment Pharmacol. Ther. 24, 731–742. doi: 10.1111/j.1365-2036.2006.03036.x
Parma, Y. R., Chacana, P. A., Lucchesi, P. M. S., Rogé, A., Granobles Velandia, C. V., Krüger, A., et al. (2012). Detection of Shiga Toxin-Producing Escherichia Coli by Sandwich Enzyme-Linked Immunosorbent Assay Using Chicken Egg Yolk IgY Antibodies. Front. Cell Infect. Microbiol. 2. doi: 10.3389/fcimb.2012.00084
Petro, C. D., Duncan, J. K., Seldina, Y. I., Allué-Guardia, A., Eppinger, M., Riddle, M. S., et al. (2020). Genetic and Virulence Profiles of Enteroaggregative Escherichia Coli (EAEC) Isolated From Deployed Military Personnel (DMP) With Travelers' Diarrhea. Front. Cell Infect. Microbiol. 10, 200. doi: 10.3389/fcimb.2020.00200
Pightling, A. W., Pettengill, J. B., Luo, Y., Baugher, J. D., Rand, H., Strain, E. (2018). Interpreting Whole-Genome Sequence Analyses of Foodborne Bacteria for Regulatory Applications and Outbreak Investigations. Front. Microbiol. 9, 1482. doi: 10.3389/fmicb.2018.01482
Qin, X., Klein, E. J., Galanakis, E., Thomas, A. A., Stapp, J. R., Rich, S., et al. (2015). Real-Time PCR Assay for Detection and Differentiation of Shiga Toxin-Producing Escherichia Coli From Clinical Samples. J. Clin. Microbiol. 53, 2148–2153. doi: 10.1128/JCM.00115-15
Rahal, E. A., Fadlallah, S. M., Nassar, F. J., Kazzi, N., Matar, G. M. (2015). Approaches to Treatment of Emerging Shiga Toxin-Producing Escherichia Coli Infections Highlighting the O104:H4 Serotype. Front. Cell Infect. Microbiol. 5, 24. doi: 10.3389/fcimb.2015.00024
Rao, G. G., Saunders, V. P., Masterson, R. G. (1996). Laboratory Acquired Verotoxin Producing Escherichia Coli (VTEC) Infection. J. Hosp Infect. 33, 228–230. doi: 10.1016/S0195-6701(96)90008-0
Raya, R. R., H’bert, E. ,. M. (2009). Isolation of Phage via Induction of Lysogens. Methods Mol. Biol. 501, 23–32. doi: 10.1007/978-1-60327-164-6_3
Rivas, M., Voyer, L., Tous, M., Leardini, N., De Mena, M. F., Wainsztein, R., et al. (1993). Hemolytic Uremic Syndrome: Co-Infection With Two Different Serotypes of Shiga-Like Toxin Producing Escherichia Coli. Medicina (B Aires) 53, 487–490.
Rusconi, B., Sanjar, F., Koenig, S. S., Mammel, M. K., Tarr, P. I., Eppinger, M. (2016). Whole Genome Sequencing for Genomics-Guided Investigations of Escherichia Coli O157:H7 Outbreaks. Front. Microbiol. 7, 985. doi: 10.3389/fmicb.2016.00985
Sadiq, S. M., Hazen, T. H., Rasko, D. A., Eppinger, M. (2014). EHEC Genomics: Past, Present, and Future. Microbiol. Spectr. 2 (4), EHEC–0020-2013. doi: 10.1128/microbiolspec.EHEC-0020-2013
Saenz, Y., Zarazaga, M., Brinas, L., Ruiz-Larrea, F., Torres, C. (2003). Mutations in gyrA and parC Genes in Nalidixic Acid-Resistant Escherichia Coli Strains From Food Products, Humans and Animals. J. Antimicrob. Chemother. 51, 1001–1005. doi: 10.1093/jac/dkg168
Salerno, A., Meyers, K., McGowan, K., Kaplan, B. (2004). ). Hemolytic Uremic Syndrome in a Child With Laboratory-Acquired Escherichia Coli O157:H7. J. Pediatr. 145, 412–414. doi: 10.1016/j.jpeds.2004.05.040
Scheutz, F., Teel, L. D., Beutin, L., Pierard, D., Buvens, G., Karch, H., et al. (2012). Multicenter Evaluation of a Sequence-Based Protocol for Subtyping Shiga Toxins and Standardizing Stx Nomenclature. J. Clin. Microbiol. 50, 2951–2963. doi: 10.1128/JCM.00860-12
Serna, A.4., Boedeker, E. C. (2008). Pathogenesis and Treatment of Shiga Toxin-Producing Escherichia Coli Infections. Curr. Opin. Gastroenterol. 24, 38–47. doi: 10.1097/MOG.0b013e3282f2dfb8
Shimizu, T., Ohta, Y., Noda, M. (2009). Shiga Toxin 2 is Specifically Released From Bacterial Cells by Two Different Mechanisms. Infect. Immun. 77, 2813–2823. doi: 10.1128/IAI.00060-09
Skinner, C., Patfield, S., Stanker, L. H., Fratamico, P. (2014). New High-Affinity Monoclonal Antibodies Against Shiga Toxin 1 Facilitate the Detection of Hybrid Stx1/Stx2. In vivo PloS One 9 (6), e99854. doi: 10.1371/journal.pone.0099854
Smith, K. E., Wilker, P. R., Reiter, P. L., Hedican, E. B., Bender, J. B., Hedberg, C. W. (2012). Antibiotic Treatment of Escherichia Coli O157 Infection and the Risk of Hemolytic Uremic Syndrome, Minnesota. Pediatr. Infect. Dis. J. 31, 37–41. doi: 10.1097/INF.0b013e31823096a8
Sowinski, K. M., Lucksiri, A., Kays, M. B., Scott, M. K., Mueller, B. A., Hamburger, R. J. (2003). Levofloxacin Pharmacokinetics in ES.D and Removal by the Cellulose Acetate High Performance-210 Hemodialyzer. Am. J. Kidney Dis. 42, 342–349. doi: 10.1016/S0272-6386(03)00660-7
Spina, N., Zansky, S., Dumas, N., Kondracki, S. (2005). Four Laboratory-Associated Cases of Infection With Escherichia Coli O157:H7. J. Clin. Microbiol. 43, 2938–2939. doi: 10.1128/JCM.43.6.2938-2939.2005
Stamatakis, A. (2014). RAxML Version 8: A Tool for Phylogenetic Analysis and Post-Analysis of Large Phylogenies. Bioinformatics 30, 1312–1313. doi: 10.1093/bioinformatics/btu033
Strachan, N. J., Rotariu, O., Lopes, B., Macrae, M., Fairley, S., Laing, C., et al. (2015). Whole Genome Sequencing Demonstrates That Geographic Variation of Escherichia Coli O157 Genotypes Dominates Host Association. Sci. Rep. 5, 14145. doi: 10.1038/srep14145
Tarr, P. I., Gordon, A., Chandler, W. L. (2005). Shiga-Toxin-Producing Escherichia Coli and Haemolytic Uraemic Syndrome. Lancet 365 (9464), 1073–1086. doi: 10.1016/S0140-6736(05)71144-2
Tatusova, T., Dicuccio, M., Badretdin, A., Chetvernin, V., Nawrocki, E. P., Zaslavsky, L., et al. (2016). NCBI Prokaryotic Genome Annotation Pipeline. Nucleic Acids Res. 44, 6614–6624. doi: 10.1093/nar/gkw569
Tesh, V. L., Burris, J. A., Owens, J. W., Gordon, V. M., Wadolkowski, E. A., O'brien, A. D., et al. (1993). Comparison of the Relative Toxicities of Shiga-Like Toxins Type I and Type II for Mice. Infect. Immun. 61, 3392–3402. doi: 10.1128/iai.61.8.3392-3402.1993
Tserenpuntsag, B., Chang, H. G., Smith, P. F., Morse, D. L. (2005). Hemolytic Uremic Syndrome Risk and Escherichia Coli O157:H7. Emerg. Infect. Dis. 11, 1955–1957. doi: 10.3201/eid1112.050607
Underwood, A. P., Dallman, T., Thomson, N. R., Williams, M., Harker, K., Perry, N., et al. (2013). Public Health Value of Next-Generation DNA Sequencing of Enterohemorrhagic Escherichia Coli Isolates From an Outbreak. J. Clin. Microbiol. 51, 232–237. doi: 10.1128/JCM.01696-12
Walker, B. J., Abeel, T., Shea, T., Priest, M., Abouelliel, A., Sakthikumar, S., et al. (2014). Pilon: An Integrated Tool for Comprehensive Microbial Variant Detection and Genome Assembly Improvement. PloS One 9, e112963. doi: 10.1371/journal.pone.0112963
Wang, G., Clark, C. G., Rodgers, F. G. (2002). Detection in Escherichia Coli of the Genes Encoding the Major Virulence Factors, the Genes Defining the O157:H7 Serotype, and Components of the Type 2 Shiga Toxin Family by Multiplex PCR. J. Clin. Microbiol. 40, 3613–3619. doi: 10.1128/JCM.40.10.3613-3619.2002
Whittam, T. S., Wachsmuth, I. K., Wilson, R. A. (1988). Genetic Evidence of Clonal Descent of Escherichia Coli O157:H7 Associated With Hemorrhagic Colitis and Hemolytic Uremic Syndrome. J. Infect. Dis. 157, 1124–1133. doi: 10.1093/infdis/157.6.1124
Wick, R. R., Judd, L. M., Gorrie, C. L., Holt, K. E. (2017). Unicycler: Resolving Bacterial Genome Assemblies From Short and Long Sequencing Reads. PloS Comput. Biol. 13, e1005595. doi: 10.1371/journal.pcbi.1005595
Wick, L. M., Qi, W., Lacher, D. W., Whittam, T. S. (2005). Evolution of Genomic Content in the Stepwise Emergence of Escherichia Coli O157:H7. J. Bacteriol. 187, 1783–1791. doi: 10.1128/JB.187.5.1783-1791.2005
Wilgenbusch, J. C., Swofford, D. (2003). Inferring Evolutionary Trees With PAUP*. Curr. Protoc. Bioinf. 6, 6.4. doi: 10.1002/0471250953.bi0604s00
Wong, C. S., Jelacic, S., Habeeb, R. L., Watkins, S. L., Tarr, P. I. (2000). The Risk of the Hemolytic-Uremic Syndrome After Antibiotic Treatment of Escherichia Coli O157:H7 Infections. N. Engl. J. Med. 342, 1930–1936. doi: 10.1056/NEJM200006293422601
Wong, C. S., Mooney, J. C., Brandt, J. R., Staples, A. O., Jelacic, S., Boster, D. R., et al. (2012). ). Risk Factors for the Hemolytic Uremic Syndrome in Children Infected With Escherichia Coli O157:H7: A Multivariable Analysis. Clin. Infect. Dis. 55, 33–41. doi: 10.1093/cid/cis299
Xie, Z., Tang, H. (2017). ISEScan: Automated Identification of Insertion Sequence Elements in Prokaryotic Genomes. Bioinformatics 33, 3340–3347. doi: 10.1093/bioinformatics/btx433
Yang, X., Bai, X., Zhang, J., Sun, H., Fu, S., Fan, R., et al. (2020a). Escherichia Coli Strains Producing a Novel Shiga Toxin 2 Subtype Circulate in China. Int. J. Med. Microbiol. 310 (1), 151377. doi: 10.1016/j.ijmm.2019.151377
Yang, X., Sun, H., Fan, R., Fu, S., Zhang, J., Matussek, A., et al. (2020b). Genetic Diversity of the Intimin Gene (Eae) in non-O157 Shiga Toxin-Producing Escherichia Coli Strains in China. Sci. Rep. 10, 3275. doi: 10.1038/s41598-020-60225-w
Yin, S., Rusconi, B., Sanjar, F., Goswami, K., Xiaoli, L., Eppinger, M., et al. (2015). Escherichia Coli O157:H7 Strains Harbor at Least Three Distinct Sequence Types of Shiga Toxin 2a-Converting Phages. BMC Genomics 16, 733. doi: 10.1186/s12864-015-1934-1
Zhang, H., Gao, S., Lercher, M. J., Hu, S., Chen, W. H. (2012). EvolView, an Online Tool for Visualizing, Annotating and Managing Phylogenetic Trees. Nucleic Acids Res. 40, W569–W572. doi: 10.1093/nar/gks576
Zhang, X., Mcdaniel, A. D., Wolf, L. E., Keusch, G. T., Waldor, M. K., Acheson, D. W. (2000). Quinolone Antibiotics Induce Shiga Toxin-Encoding Bacteriophages, Toxin Production, and Death in Mice. J. Infect. Dis. 181, 664–670. doi: 10.1086/315239
Zhou, Y., Liang, Y., Lynch, K. H., Dennis, J. J., Wishart, D. S. (2011). PHAST: A Fast Phage Search Tool. Nucleic Acids Res. 39, W347–W352. doi: 10.1093/nar/gkr485
Keywords: Shiga toxin (Stx) producing Escherichia coli (STEC), O157:H7, laboratory infection, genome sequencing, single nucleotide polymorphisms (SNP) typing
Citation: Eppinger M, Almería S, Allué-Guardia A, Bagi LK, Kalalah AA, Gurtler JB and Fratamico PM (2022) Genome Sequence Analysis and Characterization of Shiga Toxin 2 Production by Escherichia coli O157:H7 Strains Associated With a Laboratory Infection. Front. Cell. Infect. Microbiol. 12:888568. doi: 10.3389/fcimb.2022.888568
Received: 03 March 2022; Accepted: 03 May 2022;
Published: 13 June 2022.
Edited by:
Leticia Veronica Bentancor, National University of José C. Paz, ArgentinaReviewed by:
Fernando Navarro-Garcia, Instituto Politécnico Nacional de México (CINVESTAV), MexicoPaula María Alejandra Lucchesi, National University of Central Buenos Aires, Argentina
Copyright © 2022 Eppinger, Almería, Allué-Guardia, Bagi, Kalalah, Gurtler and Fratamico. This is an open-access article distributed under the terms of the Creative Commons Attribution License (CC BY). The use, distribution or reproduction in other forums is permitted, provided the original author(s) and the copyright owner(s) are credited and that the original publication in this journal is cited, in accordance with accepted academic practice. No use, distribution or reproduction is permitted which does not comply with these terms.
*Correspondence: Mark Eppinger, bWFyay5lcHBpbmdlckB1dHNhLmVkdQ==; Pina M. Fratamico, cGZyYXRhbWljby5hcnNAZ21haWwuY29t; cGluYS5mcmF0YW1pY29AdXNkYS5nb3Y=
†Present address: Sonia Almeria, ;Food and Drug Administration (FDA), Center for Food Safety and Applied Nutrition (CFSAN), Office of Applied Research and Safety Assessment (OARSA), Laurel, MD, United States
Anna Allué-Guardia, ;Texas Biomedical Research Institute (TBRI), San Antonio, TX, United States