- 1Laboratorio de Biología Molecular y Bioquímica en Trypanosoma cruzi y otros agentes infecciosos, CONICET for Instituto de Ciencia y Tecnología (ICT) Milstein - Consejo Nacional de Investigaciones Científicas y Tecnológicas (CONICET), Buenos Aires, Argentina
- 2Área Parasitología, Microbiología, Facultad de Ciencias Bioquímicas y Farmacéuticas, Universidad Nacional de Rosario (UNR), Rosario, Argentina
- 3Centro de Estudios Parasitológicos y Vectores (CEPAVE), CONICET - Universidad Nacional de La Plata (UNLP), La Plata, Argentina
Chagas disease is an endemic American parasitosis, caused by Trypanosoma cruzi. The current therapies, benznidazole (BZN) and nifurtimox (NFX), show limited efficacy and multiple side effects. Thus, there is a need to develop new trypanocidal strategies. Ivermectin (IVM) is a broad-spectrum antiparasitic drug with low human and veterinary toxicity with effects against T. brucei and Leishmania spp. Considering this and its relatively low cost, we evaluate IVM as a potential repurposed trypanocidal drug on T. cruzi and other trypanosomatids. We found that IVM affected, in a dose-dependent manner, the proliferation of T. cruzi epimastigotes as well as the amastigotes and trypomastigotes survival. The Selectivity Index for the amastigote stage with respect to Vero cells was 12. The IVM effect was also observed in Phytomonas jma 066 and Leishmania mexicana proliferation but not in Crithidia fasciculata. On the epimastigote stage, the IVM effect was trypanostatic at 50 μM but trypanocidal at 100 μM. The assays of the drug combinations of IVM with BNZ or NFX showed mainly additive effects among combinations. In silico studies showed that classical structures belonging to glutamate-gated Cl channels, the most common IVM target, are absent in kinetoplastids. However, we found in the studied trypanosomatid genomes one copy for putative IMPα and IMPβ, potential targets for IVM. The putative IMPα genes (with 76% similarity) showed conserved Armadillo domains but lacked the canonical IMPβ binding sequence. These results allowed us to propose a novel molecular target in T. cruzi and suggest IVM as a good candidate for drug repurposing in the Chagas disease context.
Introduction
Chagas disease, caused by the protozoan parasite Trypanosoma cruzi, is considered a neglected tropical disease (NTD) (World Health Organization, 2015). This originally American endemic affects at least 6 million people and has been globally spread with the recent migrations (Pérez-Molina and Molina, 2018), estimating that 75 million people are at risk of contracting the disease. The social and economic impact of this disease is significant, with 14,000 annual deaths and 40,000 new cases every year (Nunes et al., 2013).
In addition to the complexity of social, economic, and biomedical issues about Chagas disease, there are only two drugs accepted for its treatment: nifurtimox (NFX) and benznidazole (BZN). Both drugs, developed 50 years ago, show very variable parasitological cure rates depending on the disease stage and present several adverse effects (Nunes et al., 2013). Thus, there is a clear need to find new therapies against Chagas disease to provide better assistance and life quality to patients and to reduce the social and economic burden associated with contagion and chronicity. A cost- and time-saving strategy to discover new trypanocidal treatments is drug repurposing, finding new therapeutic indications for drugs that are already approved for its use in humans (Bellera et al., 2013).
Ivermectin (IVM) is a broad-spectrum antiparasitic drug, initially developed to combat parasitic worms in veterinary fields (Campbell et al., 1983). It is a mixture of semisynthetic macrocyclic lactones (~80% 22,23-dihydro-avermectin B1a and ~20% 22,23-dihydro-avermectin B1b) (Crump, 2017; Deng et al., 2019). Nowadays, IVM is used mainly as an oral medication in the primary treatment of onchocerciasis, lymphatic filariasis, and strongyloidiasis infections, all caused by different nematode species (Turner and Schaeffer, 1989; Laing et al., 2017). IVM also affects arthropod ectoparasites, being used in the treatment of scabies (Thomas et al., 2015), ticks (Sheele et al., 2014), and head lice (Devore and Schutze, 2015).
In WHO massive antiparasitic-drug administration programs, it was observed that the incidence of malaria seemed to decrease in pediatric groups treated with IVM (Alout et al., 2014; Chaccour and Rabinovich, 2019). Further studies showed that IVM has presented in vitro and in vivo antimalarial effects, arresting the cell cycle and inhibiting parasite development and survival (Mendes et al., 2017; de Carvalho et al., 2019). In regard to trypanosomatids, IVM showed effects on Leishmania spp. in animal models and patients’ therapy with cutaneous leishmaniasis (Opara and I.G, 2005; Kadir et al., 2009). IVM also showed promising results in the models of mice infected with T. brucei (Udensi and Fagbenro-Beyioku, 2012). Related to Chagas disease and IVM, few studies have been made, mainly centered in triatomine vectors (Dias et al., 2005; Dadé et al., 2014; Dadé et al., 2017). To our knowledge, the unique report of IVM in Chagas disease in humans was a descriptive work based on a survey conducted in a self-medicated population group living in an endemic area of Bolivia, in which IVM caused some improvements in their self-perception of Chagas disease symptoms (Forsyth, 2018).
Different mechanisms of action have been proposed to explain the IVM effect on diverse organisms. IVM potentiates the glutamate-gated Cl channel (GluCl), found in invertebrates (nematodes and arthropods) (Cully et al., 1994), resulting in the hyperpolarization of parasite neurons and muscles (Chen and Kubo, 2018). IVM also inhibits importin α/β (or “karyopherins”) (Wehbe et al., 2021), a heterodimer that transports proteins to the nucleus across the nuclear complex pore; this effect has been described in the asexual blood stages of Plasmodium falciparum (Panchal et al., 2014).
This evidence, together with its relative low cost, make IVM an interesting drug candidate to study for Chagas disease treatment. The aim of this work was to analyze the effect of IVM on different T. cruzi stages and other trypanosomatids’ proliferation and viability.
Materials and methods
Parasites and cell cultures
T. cruzi epimastigotes (Y, Dm28c/pLacZ, CL Brener, RA and Tulahuen 0 strains), Phytomonas Jma 066 promastigotes, and Crithidia fasciculata choanomastigotes were cultured at 28°C in a Brain Heart Tryptose (BHT) medium supplemented with hemin (20 μg/ml) and Leishmania mexicana mexicana promastigotes (WHO strain), kindly provided by Dr. Cazzulo, were cultured at 28°C in SDM-79 (M199 media, Gibco, Grand Island, NY, USA). T. cruzi trypomastigotes and amastigotes were obtained from Dm28c/pLacZ epimastigotes expressing β-galactosidase, according to Alonso et al. (2021). Vero cells were maintained at 37°C and 5% CO2 atmosphere in Dulbecco's Modified Eagle Medium (DMEM) high glucose (Gibco) supplemented with 2 mM glutamine. All media were supplemented with streptomycin (100 μg/ml) and penicillin (100 IU/ml) and 2%–10% heat-inactivated fetal bovine serum (FBS; Natocor, Córdoba, Argentina) as indicated.
Compounds and drugs
The stock solutions of the drugs (50 mM) were prepared in dimethyl sulfoxide (DMSO) for IVM (MW: 875.1, kindly provided by Pablo Cassará Laboratory), NFX (MW: 287.3, Sigma) and BZN (MW: 260.25, Sigma, Saint Louis, MO, USA). The stock solutions were diluted in culture media to obtain the working concentrations at the moment of the assay.
Effect of ivermectin on T. cruzi and related trypanosomatids in culture
The effect of IVM was tested after 72 h of culture, by counting the number of mobile parasites in a hemocytometer chamber, by measuring optical density (OD) at 630 nm and by the Thiazolyl Blue Tetrazolium Bromide (MTT) assay (Frank and Pace, 1998), as indicated. For this last methodology, treated cells were incubated for 3:30 h at 28°C in the absence of light. The formed formazan was resuspended in DMSO. The absorbance was read at 570 nm using an ELISA plate reader (Cambridge Technology, Lexington, MA, USA) using wells with only media and reagents as reaction blank.
For Dm28c/pLacZ epimastigote, trypomastigote, and amastigote cultures, the proliferation was measured indirectly by the cytoplasmic β-galactosidase activity. Briefly, after the treatment with IVM, parasites were lysed and incubated with the enzyme substrate, chlorophenol red β-D-galactopyranoside. The colorimetric reaction was measured at 570 nm (Alonso et al., 2021).
Under same culture conditions, DMSO (at the highest concentration used) and NFX or BZN were used as negative and positive controls, respectively.
Mammalian cytotoxic activity
Cytotoxicity was in vitro tested on Vero cells treated with increasing concentrations of IVM diluted in DMEM (up to 15 µM). The plate was then incubated for 24 h at 37°C with 5% CO2, and the MTT assay was performed as previously explained (see Effect of ivermectin on T. cruzi and related trypanosomatids in culture).
Cell proliferation recovery assay
Recovery assays were performed as Kessler et al. (2013). Briefly, T. cruzi epimastigotes were preincubated with IVM at 50 and 100 μM (four and eight times the calculated IVM EC5072h, respectively), during different periods of time (from 30 min to 3 h). Then, parasites were washed with sterile PBS, transferred to a drug-free BHT 10% FBS medium, and incubated at 28°C. Growth recovery was monitored at days 4 and 8 by cell density in a hemocytometer chamber and OD at 630 nm.
Drug combination study
To evaluate the effect of interaction between IVM and BZN or NFX on T. cruzi epimastigotes, a drug combination matrix was designed to test both drugs simultaneously or in a sequential manner. For simultaneous assays, the serial dilutions of IVM (0–50 μM) were placed in the columns of a 96-well plate and increasing concentrations of the second drug (BNZ or NFX) were added in each row, and then, parasites were seeded. For sequential assays, epimastigotes were first preincubated with IVM, NFX, or BZN in the corresponding matrix concentration; after 1 h, parasites were washed and seeded in a 96-well plate and the second drug was added following the combination matrix. The final concentration of DMSO was at most 0.5%. Treated cultures, blank samples (only BHT and drugs in their maximal concentration), and negative control (parasites in 0.5% DMSO) were incubated at 28˚C for 72 h, and parasite proliferation was measured by OD at 630 nm. Data were analyzed using the free software Combenefit (v. 2.021, Cambridge University, Cambridge, UK) (Di Veroli et al., 2016). Relative epimastigote proliferation obtained experimentally was compared against the predicted values according to Bliss Independence or Loewe additivity models. The synergy score calculated by the software is a positive value for a synergic effect, 0 for an additive effect (when drugs have no interaction), and negative for an antagonic effect. The results obtained were depicted in a synergy distribution plot where effects are indicated by a scale of colors and numbers, and statistical differences are marked with an asterisk.
In silico analysis
In order to postulate a possible mechanism of action of IVM in T. cruzi, an in silico study was performed, supported with bibliographic data. To search for orthologous protein sequences of importin α (IMPα) and importin β (IMPβ) in the trypanosomatids of interest (T. cruzi, L. exicana, C. fasciculata, and Phytomonas spp.) and other close-related species, TritrypDB (Tritrypdb.org), Uniprot (www.uniprot.org/), and National Center for Biotechnology Information (NCBI) databases were used. Sequence alignments and analysis were performed with ClustalOmega (www.ebi.ac.uk/Tools/msa/clustalo/), GeneDoc 2.7 (www.psc.edu/biomed/genedoc), and Jalview 2.11.1.2 (www.jalview.org). Protein domains were identified using PFAM (Mistry et al., 2021) and SMART (Letunic et al., 2021) online tools, both developed by EMBL-EBI.
Statistical analysis
All assays were independently performed in triplicate and data expressed as mean ± SEM. The significance of differences was evaluated with one-way analysis of variance (ANOVA) with post-hoc analysis using Dunnet’s test. A p <0.05 was considered significant. To calculate the EC5024/72h of the drug, normalized proliferation or viability values were plotted against the Log of drug concentration (μM) and fitted to a sigmoidal curve determined by a non-linear regression. The selectivity index (SI) was calculated as CC50Vero cells/EC50amastigotes. Tests were performed using the GraphPad prism version 5.00 for Windows (GraphPad Software, La Jolla, CA, USA, www.graphpad.com).
Results
Effect and specificity of ivermectin on T. cruzi, other related trypanosomatids and host cells
The drug effect was evaluated, as a first approach, by following epimastigote cultures during 8 days with/without increasing concentrations of IVM, NFX, and BNZ. IVM affected T. cruzi epimastigote proliferation in a dose-dependent manner with an EC5072h of 12.5 μM for the Y strain (Supplementary Figure 1) and 5.3 uM for the Dm28c strain. Other strains of T. cruzi (CL Brener, RA and Tulahuen 0) showed EC5072h values ranging from 8.1 to 9.2 μM (data not shown). These are encouraging values as they are found between those obtained for us for the reference drugs (Table 1) and in bibliography (Luna et al., 2009; Alonso et al., 2021).
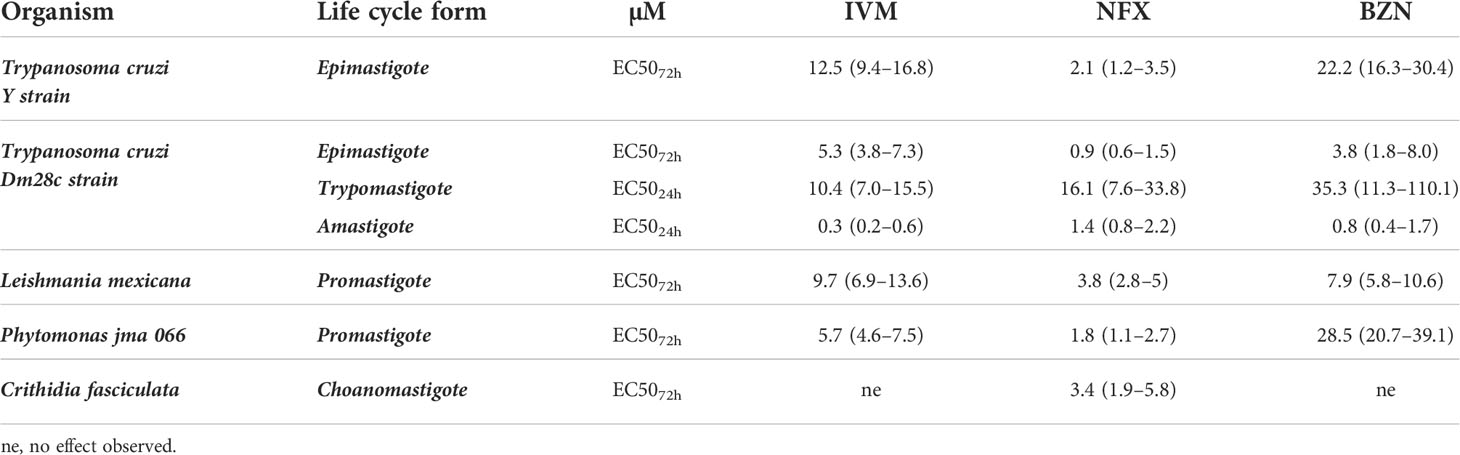
Table 1 EC50 values (μM) of ivermectin, nifurtimox, and benznidazole were determined in T. cruzi (Y strain-DTU II epimastigotes; Dm28c—Discreet Typing Units (DTU) I epimastigotes, trypomastigotes, and intracellular amastigotes), C. fasciculata choanomastigotes, and L. mexicana and Phytomonas jma 066 promastigotes.
The Dm28c strain expressing β-galactosidase, a quick and reproducible drug screening method, was used to evaluate the sensitivity of the trypomastigote and intracellular amastigote forms to IVM. Trypomastigotes showed an EC5024h of 10.4 μM, while intracellular amastigotes showed more sensitivity with an EC5024h of 0.3 μM (Table 1).
The IVM effect was also evaluated in trypanosomatid species related to T. cruzi; while it did not show effects on C. fasciculata (an insect trypanosomatid) under the evaluated concentrations (up to 200 μM), this drug affected the proliferation of Phytomonas jma 066 (a plant parasite) and L. mexicana (a human disease–causing parasite) with an EC5072h of 5.7 μM and 11.7 μM, respectively (Table 1). These results show that IVM has a specific broad range of effects on the different species of trypanosomatids as it has for other human pathogens.
The IVM cytotoxic effect at 24 h in Vero cells (CC5024h) was 3.6 µM (2.8–4.6) that, related to the EC5024h in amastigotes (both assays performed in similar conditions), results in a selectivity index (SI) of 12, being an SI value ≥10 commonly assumed as a promissory basal (Katsuno et al., 2015).
Effect of ivermectin in cell recovery assays in T. cruzi epimastigotes
To evaluate whether the effect of IVM was trypanostatic or trypanocidal, cell recovery assays were performed. The effect of a drug is considered trypanostatic when parasite proliferation recovers in a drug-free medium after a short exposure to high drug concentrations and trypanocidal when it has irreversible effects on parasite proliferation (Mosquillo et al., 2018). At 50 μM IVM (4× EC5072h), we observed that epimastigote proliferation was recovered by removing the drug after incubation for up to 1 h, indicating a trypanostatic effect, followed by a significant slight proliferation decrease effect after 3 h treatment (Figure 1A). The assays performed with 100 μM IVM (8× EC5072h) showed an irreversible effect of the drug on parasite proliferation, independently of the exposure time, indicating a trypanocidal effect at this concentration (Figure 1B). According to these results, IVM treatment during short periods of time can be trypanostatic or trypanocidal depending on the concentration used.
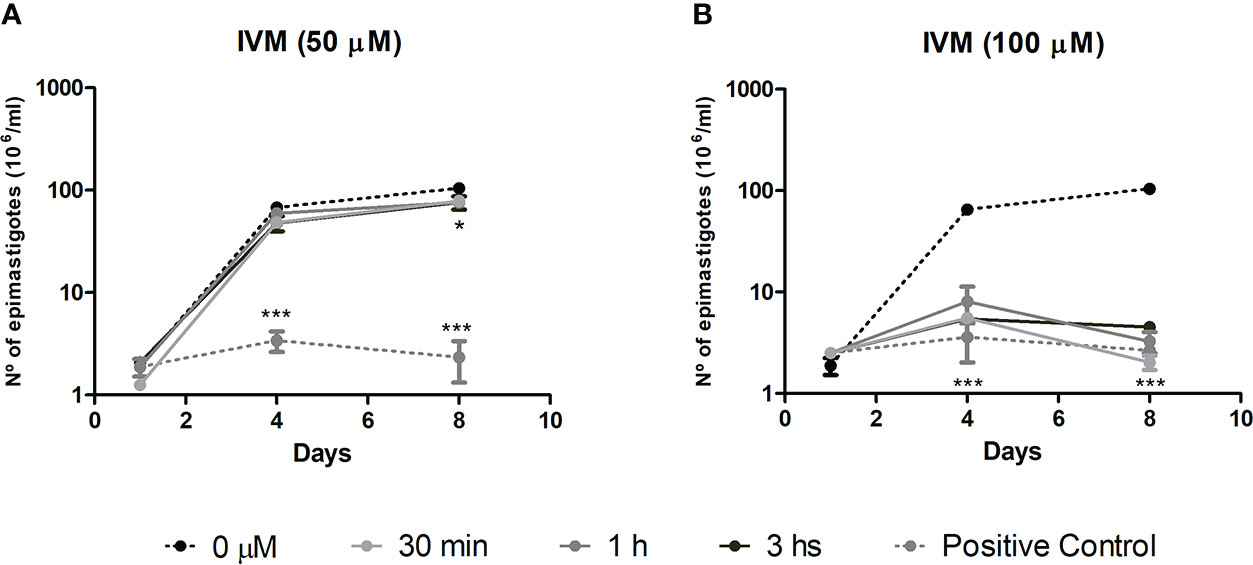
Figure 1 Cell proliferation recovery assay to test the effects of ivermectin (IVM) when exposed for short periods of time. T. cruzi epimastigotes were preincubated with (A) IVM 50 μM (4xEC5072h) or (B) IVM 100 μM (8xEC5072h) for 30 min, 1 or 3 h and then transferred to a drug-free medium for 8 days of culture. Untreated control: 0 μM IVM (0.5% dimethyl sulfoxide); positive control: 50 or 100 μM IVM during the 8 days of culture. Results are expressed as the number of epimastigotes (106)/ml, counted in a hemocytometer chamber. ***p<0.001, *p<0.05, ANOVA, Dunnett test.
Effect of ivermectin in combination with benznidazole and nifurtimox
To study the effect of the simultaneous or sequential combination of IVM with BZN or NFX, epimastigotes were cultured in combination matrices and the obtained data were analyzed with the free software Combenefit (Di Veroli et al., 2016), in which the null hypothesis of no synergy needs to be defined in order to assess the degree of the effect. In the case of IVM+BZN and IVM+NFX, the hypothesis was that these drugs have different mechanisms of action, taking the Bliss independence model as the best approach (Seguel et al., 2016; Tang, 2017). When the drugs were combined simultaneously, synergy distribution heatmaps-obtained from the comparison of experimental data with model-predicted values-showed an additive behavior in most of the concentrations. However, there were antagonistic effects at some combinations with middle and high concentrations of IVM (Figures 2A, B), and, on the other hand, some synergistic points at low concentrations of IVM-NFX were observed. In sequential assays, when IVM was preincubated for 1 h and then NFX or BZN was added, we only observed additive effects (Figures 2C, D), but when the preincubation was done with NFX or BZN, aside from additive effects, a slight antagonistic behavior at high concentrations of drugs was also observed (Figures 2E, F). Finally, we performed a control combination with NFX-BZN using the Loewe additivity model, based on the hypothesis that both drugs have common mechanisms of action (Tang, 2017), and the observed effects of combinations were mainly additive or antagonistic (Figure 2G).
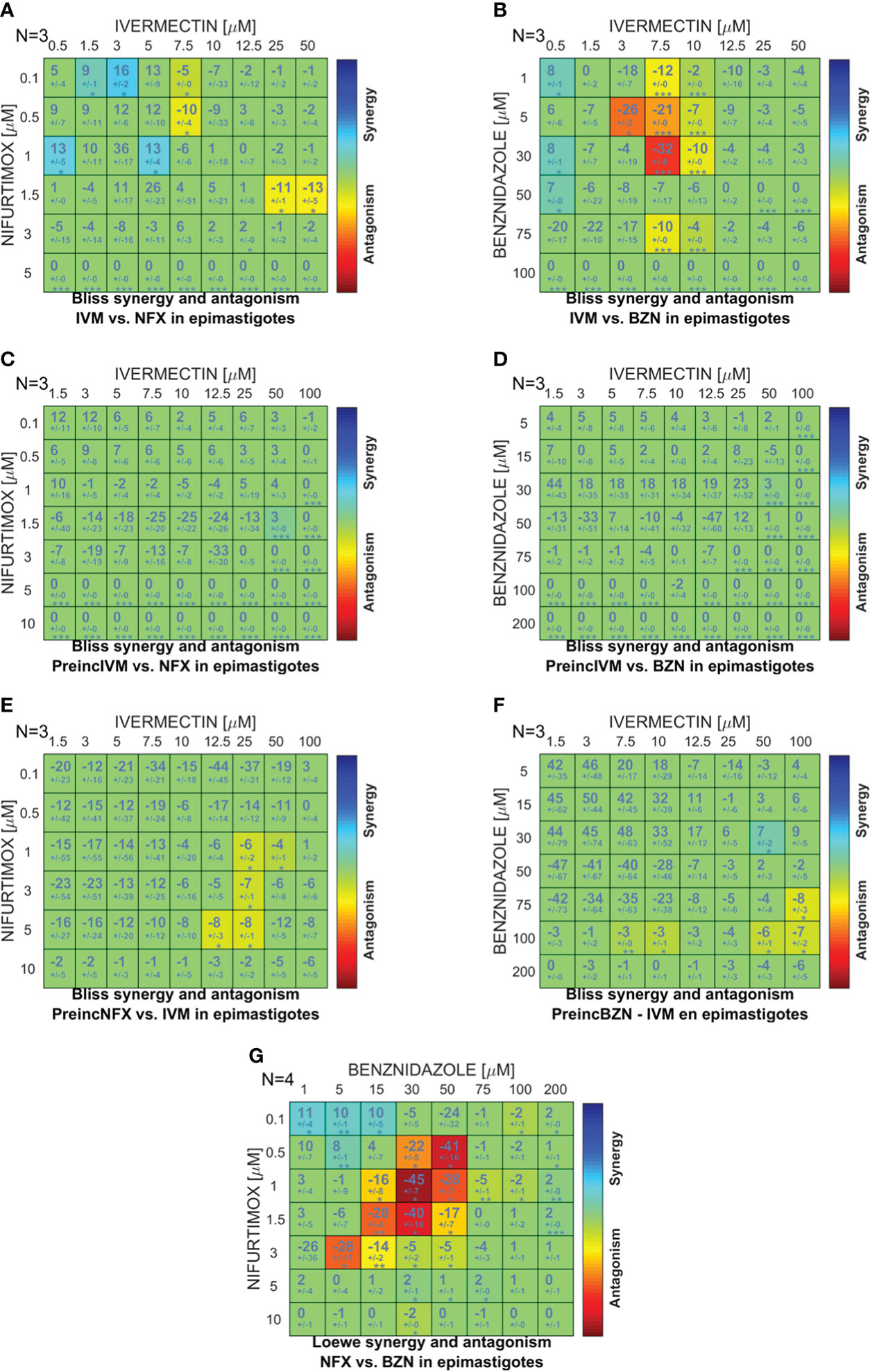
Figure 2 Comparison between the in vitro and predicted effects of drug combinations on T. cruzi epimastigote proliferation. A combination matrix was designed with IVM (0–50 µM) and benznidazole (BZN; 0–100 µM) or nifurtimox (NFX; 0–5 µM), and the drug combination effect was evaluated on the culture proliferation of epimastigotes at day 4. The results obtained in the in vitro culture assay were compared against the synergy scores predicted by applying the Bliss independence model for: (A) IVM-NFX and (B) IVM-BZN combined simultaneously; (C) IVM-NFX and (D) IVM-BZN with the preincubation of IVM for 1 h; (E) NFX-IVM and (F) BZN-IVM with preincubation of the trypanocidal drug for 1 h; and (G) by applying Loewe additivity model for the combination of NFX-BZN simultaneously. The scale of numbers and colors represents the type of effect obtained according to the comparison between in vitro and model-predicted results; the scale spans from statistically significant synergistic interaction (positive values—blue color, p<0.01) to statistically significant antagonism (negative values—red color, p<0.01).
Bioinformatic analysis of potential drug targets and mechanism of action of ivermectin in T. cruzi
In order to find a possible mechanism of action of IVM on T. cruzi, different known targets of the drug in other organisms were studied. A first search in TritrypsDB, performed for ligand-activated chloride channels, did not show any positive coincidence in the genome of T. cruzi. To verify these results, an extensive bibliographic search was performed, finding well-established phylogenetic relations between homologous genes encoding for a GluCl along the kingdoms of life (Rendon et al., 2011). Other authors, applying three independent approaches (Psi-BLAST, HMMer, and Interpro, all based on hidden Markov models), found genetic sequences that codify for pentameric ligand–gated ion channel structures from Cys-loop receptors in a wide array of protists (Jaiteh et al., 2016) that did not include kinetoplastid genomes. Then, we conclude that IVM would be acting through another mechanism in T. cruzi.
Another studied mechanism of IVM action is the inhibition of nuclear transport by dissociating the performed importin α/β1 (IMPα/β1) heterodimer as well as by preventing its formation (Yang et al., 2020). At the moment, there is a unique report about IMPα in T. cruzi (Canela-Pérez et al., 2020). In order to deepen this work, we found putative sequences of IMPα in T. cruzi (Gene DB: TcCLB.509965.110), L. mexicana (Gene DB: LmxM.29.1120), C. fasciculata (Gene DB: CFAC1_260031300), and Phytomonas spp. (Protein DB: W6KHG4). TcIMPα is present in the CL Brener strain, Esmeraldo-like and non-Esmeraldo-like haplotypes, in chromosome 32; its length is 1,602 bp, and the predicted protein has 533 aa. We also found one copy of IMPβ (Gene DB: TcCLB.504105.150). Only one variant of each protein is reported in trypanosomatids, unlike mammals in which there exists a family of IMPα and IMPβ (in humans, seven IMPα and more than 20 IMPβ), showing the relevance of the T. cruzi heterodimer as a potential druggable target (Wagstaff et al., 2012).
A structural characterization of the IMPα sequences was made by the alignment of these proteins with those already characterized in Mus musculus (UniProtKB DB: P52293), Homo sapiens (UniProtKB DB: P52292), and P. falciparum (UniProtKB DB: Q7KAV0) (Supplementary Figure 2). Each sequence was analyzed with Pfam and SMART (EMBL) in order to determine domains and relative positions. All proteins had seven-to-nine Armadillo domains (ARM), separated by a conserved glycine (Canela-Pérez et al., 2020), with each ARM being composed of three hydrophobic α-helices (Cingolani et al., 1999). These repeated ARM domains end with an atypical ARM repeat (ARM9), which could be the linker with CAS exportin, and the IBB domain (Importinβ-binding domain) is found in the first 100 amino acids (N-terminal) in mammals as H. sapiens and M. musculus. In trypanosomatids, ARM repeats appear after the first 100-110 aa but there is not a typical recognizable IBB, which could be related to the presence of an IBB domain with particular characteristics (Supplementary Figure 2).
Discussion
Drug repositioning is a widely used strategy to find new treatments for Chagas disease; several of the latest clinical trials were performed with repurposed drugs as posaconazole and fosravuconazole (Field et al., 2017). With IVM being a secure broad-spectrum antiparasitic drug used in human diseases, this drug would be an attractive repurposing candidate to study. Curiously, there are few reports that tangentially relate it with some Chagas disease aspects. Here, we present the first approach to study the effects of IVM on T. cruzi as a potential drug for Chagas disease.
In this report, we showed that IVM affected in a dose-dependent manner the proliferation of T. cruzi epimastigotes-a simple model to study trypanocidal drug effects-(EC5072h= 5.3–12.5 μM in the different strains evaluated) as well as amastigote (EC5024h=0.3 μM) and trypomastigote survival (EC5024h=10.4 μM). As BZN and NFX, IVM resulted to be more effective against amastigote stage, but with lower EC50 values (Luna et al., 2009), arousing interest as a potential trypanocidal drug. Moreover, the SI of IVM obtained for T. cruzi amastigotes was 12 in agreement with the drug selection criteria for infectious diseases that establish an SI value ≥10 as a promissory limit (Katsuno et al., 2015). On the other hand, the SI of NFX and BZN reported in bibliography are very variable, with values ranging from 1.5 to over 200 according to the strain and the cell line used (González et al., 2020). Reinforcing the idea of IVM as possible therapy against T. cruzi in humans, published pharmacokinetic data have shown a safety profile for the human use of the oral administration of IVM without adverse side effects, reaching plasmatic concentrations in the range of the EC50 values found in this work (Guzzo et al., 2002).
IVM also inhibited the in vitro proliferation of Phytomonas jma 066 and L. mexicana, as previously shown in L. tropica (Kadir et al., 2009), while C. fasciculata choanomastigotes resulted to be highly tolerant to IVM and BZN. Throughout our results, IVM has shown effects on a broad but specific range of trypanosomatids as it has for other human pathogens.
We found that the trypanocidal capacity of IVM depends on its concentration (8xEC5072h) but not on the exposure time. An irreversible effect was also observed on P. falciparum at 25-µM IVM during 24 h of exposure (Panchal et al., 2014). Different mechanisms of cellular death (as necrosis or autophagy) could be triggered in T. cruzi parasites in response to drugs (Kessler et al., 2013). Moreover, the same compound can trigger different-reversible or irreversible-mechanisms according to the concentration and duration of the stimuli. Additionally, when the underlying mechanism of the drug is trypanostatic, parasitemia could show a relapse after antitrypanosomal chemotherapy (Ercoli and Iudice, 1980). This variable behavior of a compound is an important issue for therapy design.
In the last few years, the investigation of drug combinations has become an important strategy to combat drug resistance and reduce treatment courses in NTDs. Here, different drug combination regimens were tested in vitro on the epimastigote culture, finding that a simultaneous combination of IVM-BZN and IVM-NFX showed mainly additive effects; however, some combinations showed significant antagonistic or synergic effects. These effects could be explained by different described mechanisms of IVM action, including an antagonism by increasing detoxification processes induced by this drug that avoid the effects of NFX or BZN (Lespine et al., 2007; Ménez et al., 2012; Nodari et al., 2020) or a synergism by inhibiting the activity of P-gp, a member of the superfamily of transporters ABC (ATP-binding cassette), that enhances the cytotoxicity of other drugs (Furusawa et al., 2000; Landfear, 2019). As mentioned by other authors, the in vitro assays about the interaction between two drugs are usually the first-step studies, although the observed results do not necessarily reflect the in vivo effects; pharmacokinetic and host factors (as the immune response) may lead to different outcomes due to the modification of the effective exposure of the parasites to the drugs (Vincent et al., 2012).
The IMPα and IMPβ heterodimer complex, one of the IVM molecular targets, was searched in trypanosomatid genomes. One copy of each gene was detected in T. cruzi, L. mexicana, Phytomonas spp., and C. fasciculata, in agreement with previous studies (Canela-Pérez et al., 2020). Through multiple sequence alignment and structural analysis, we identified ARM domains (between seven and nine, according to the organism) while the typical IBB domain was not recognizable in these trypanosomatids.
The IMPα ARM repeat domain is relevant for the interaction with cargo proteins via a nuclear localization sequence (NLS), a short amino acid motif, present in cargo proteins (Yang et al., 2020). The evidence of conserved ARM domains in trypanosomatids and the in vitro studies showing their capacity of recognizing and binding to the NLS of T. cruzi (Canela-Pérez et al., 2019) suggest that this conserved mechanism of intracellular trafficking is worthy to be studied (Canela-Pérez et al., 2020).
The IBB domain, in N-terminus of IMPα, has three clusters of basic amino acid residues, and it is essential for binding to IMPβ and for efficient nuclear entry (Cingolani et al., 1999); in addition, the IBB domain can bind to the NLS-binding site of IMPα, leading to the autoinhibition of intracellular transport (Dey and Patankar, 2018). However, most protozoans lack a typical IBB, showing an early divergence (Dey and Patankar, 2018; Mayol et al., 2020). For example, P. falciparum, T. gondii, and G. lamblia do not present the three conserved clusters of basic amino acids in this region, and particularly, P. falciparum has shown to have a reduced autoinhibition mechanism of nuclear transport (Dey and Patankar, 2018; Mayol et al., 2020).
IVM is nowadays considered a specific inhibitor of the classical transport pathway of nuclear proteins. Although little is known about nuclear transport in T. cruzi, IVM has been associated with the inhibition of nuclear transport by TcIMPα, showing that 25 μM IVM impaired the nuclear transport of an RNA polymerase of T. cruzi and 250 μM IVM has an inhibitory effect on the binding ability of TcIMPα to an NLS peptide (Canela-Pérez et al., 2018 and Canela-Pérez et al., 2020). The accumulating evidence about importins makes them an attractive potential druggable target to continue studying. Finally, the study of the mechanisms of action of IVM on T. cruzi and other trypanosomatids is relevant to understand the currently unknown aspects of the parasite biochemistry and to discover novel molecular targets with potential for trypanosomatid therapy.
Data availability statement
The raw data supporting the conclusions of this article will be made available by the authors, without undue reservation.
Author contributions
LF, MR, and CC have worked in the study design, writing, and result discussion; LF and MR have made most of the experimental work. VP has conducted the experiments in the infective stages of T. cruzi. AC and LL have contributed with proliferation assays and DEB with bioinformatic work and analysis. LF and CC coordinated the investigation. LF and MR contributed equally, sharing the first authorship. All authors have contributed and given approval to the final version of the manuscript.
Funding
The present work was supported by ICT Milstein - CONICET and The National Agency of Scientific and Technological Promotion (funds PICT 2015-0962 and PICT 2018-1124).
Acknowledgments
The authors would like to thank CONICET, the Argentine National Council responsible for RRHH, ANPCyT, the Argentine National Agency that funded this work, and Laboratorio Pablo Cassará for donating the ivermectin used in this work.
Conflict of interest
The authors declare that the research was conducted in the absence of any commercial or financial relationships that could be construed as a potential conflict of interest.
Publisher’s note
All claims expressed in this article are solely those of the authors and do not necessarily represent those of their affiliated organizations, or those of the publisher, the editors and the reviewers. Any product that may be evaluated in this article, or claim that may be made by its manufacturer, is not guaranteed or endorsed by the publisher.
Supplementary material
The Supplementary Material for this article can be found online at: https://www.frontiersin.org/articles/10.3389/fcimb.2022.885268/full#supplementary-material
References
Alonso, V. L., Manarin, R., Perdomo, V., Gulin, E., Serra, E., Cribb, P. (2021). In vitro drug screening against all life cycle stages of trypanosoma cruzi using parasites expressing β-galactosidase. J. Vis. Exp. 5 (177), e63210. doi: 10.3791/63210
Alout, H., Krajacich, B. J., Meyers, J. I., Grubaugh, N. D., Brackney, D. E., Kobylinski, K. C., et al. (2014). Evaluation of ivermectin mass drug administration for malaria transmission control across different West African environments. Malar. J. 13, 417. doi: 10.1186/1475-2875-13-417
Bellera, C. L., Balcazar, D. E., Alberca, L., Labriola, C. A., Talevi, A., Carrillo, C. (2013). Application of computer-aided drug repurposing in the search of new cruzipain inhibitors: Discovery of amiodarone and bromocriptine inhibitory effects. J. Chem. Inf. Model. 53, 2402–2408. doi: 10.1021/ci400284v
Campbell, W. C., Fisher, M. H., Stapley, E. O., Albers-Schonberg, G., Jacob, T. A. (1983). Ivermectin: a potent new antiparasitic agent. Science 221, 823–828. doi: 10.1126/science.6308762
Canela-Pérez, I., López-Villaseñor, I., Cevallos, A. M., Hernández, R. (2018). Nuclear distribution of the trypanosoma cruzi RNA pol I subunit RPA31 during growth and metacyclogenesis, and characterization of its nuclear localization signal. Parasitol. Res. 117, 911–918. doi: 10.1007/s00436-018-5747-4
Canela-Pérez, I., López-Villaseñor, I., Cevallos, A. M., Hernández, R. (2020). Trypanosoma cruzi importin α: ability to bind to a functional classical nuclear localization signal of the bipartite type. Parasitol. Res. 119, 3899–3907. doi: 10.1007/s00436-020-06885-z
Canela-Pérez, I., López-Villaseñor, I., Mendoza, L., Cevallos, A. M., Hernández, R. (2019). Nuclear localization signals in trypanosomal proteins. Mol. Biochem. Parasitol. 229, 15–23. doi: 10.1016/j.molbiopara.2019.02.003
Chaccour, C., Rabinovich, N. R. (2019). Advancing the repurposing of ivermectin for malaria. Lancet 393, 1480–1481. doi: 10.1016/S0140-6736(18)32613-8
Chen, I.-S., Kubo, Y. (2018). Ivermectin and its target molecules: shared and unique modulation mechanisms of ion channels and receptors by ivermectin. J. Physiol. 596, 1833–1845. doi: 10.1113/JP275236
Cingolani, G., Petosa, C., Weis, K., Müller, C. W. (1999). Structure of importin-beta bound to the IBB domain of importin-alpha. Nature 399, 221–229. doi: 10.1038/20367
Crump, A. (2017). Ivermectin: enigmatic multifaceted ‘wonder’ drug continues to surprise and exceed expectations. J. Antibiot. (Tokyo) 70, 495–505. doi: 10.1038/ja.2017.11
Cully, D. F., Vassilatis, D. K., Liu, K. K., Paress, P. S., van der Ploeg, L. H. T., Schaeffer, J. M., et al. (1994). Cloning of an avermectin-sensitive glutamate-gated chloride channel from caenorhabditis elegans. Nature 371, 707–711. doi: 10.1038/371707a0
Dadé, M., Daniele, M., Marin, G., Maria Pia, S., Mestorino, N. (2014). Ivermectin efficacy against triatoma infestans in vivo using hen model. J. Pharm. Pharmacol. 2, 353–358.
Dadé, M., Daniele, M., Mestorino, N., Dadé, M., Daniele, M., Mestorino, N. (2017). Evaluation of the toxic effects of doramectin, ivermectin and eprinomectin against triatoma infestans using a rat model. Biomédica 37, 324–332. doi: 10.7705/biomedica.v34i2.3316
de Carvalho, L. P., Sandri, T. L., José Tenório de Melo, E., Fendel, R., Kremsner, P. G., Mordmüller, B., et al. (2019). Ivermectin impairs the development of sexual and asexual stages of plasmodium falciparum In vitro. Antimicrob. Agents Chemother. 63, e00085–e00019. doi: 10.1128/AAC.00085-19
Deng, Q., Xiao, L., Liu, Y., Zhang, L., Deng, Z., Zhao, C. (2019). Streptomyces avermitilis industrial strain as cell factory for ivermectin B1a production. Synth. Syst. Biotechnol. 4, 34–39. doi: 10.1016/j.synbio.2018.12.003
Devore, C. D., Schutze, G. E. (2015). Head lice. Pediatrics 135, e1355–e1365. doi: 10.1542/peds.2015-0746
Dey, V., Patankar, S. (2018). Molecular basis for the lack of auto-inhibition of plasmodium falciparum importin α. Biochem. Biophys. Res. Commun. 503, 1792–1797. doi: 10.1016/j.bbrc.2018.07.115
Dias, J. C. P., Schofield, C. J., Machado, E. M. M., Fernandes, A. J. (2005). Ticks, ivermectin, and experimental chagas disease. Mem. Inst. Oswaldo Cruz 100, 829–832. doi: 10.1590/s0074-02762005000800002
Di Veroli, G. Y., Fornari, C., Wang, D., Mollard, S., Bramhall, J. L., Richards, F. M., et al. (2016). Combenefit: an interactive platform for the analysis and visualization of drug combinations. Bioinform. Oxf. Engl. 32, 2866–2868. doi: 10.1093/bioinformatics/btw230
Ercoli, N., Iudice, G. (1980). Trypanostatic drug action: its relation to relapse following chemotherapy. Chemotherapy 26, 218–223. doi: 10.1159/000237908
Field, M. C., Horn, D., Fairlamb, A. H., Ferguson, M. A. J., Gray, D. W., Read, K. D., et al. (2017). Anti-trypanosomatid drug discovery: an ongoing challenge and a continuing need. Nat. Rev. Microbiol. 15, 217–231. doi: 10.1038/nrmicro.2016.193
Forsyth, C. (2018). From lemongrass to ivermectin: Ethnomedical management of chagas disease in tropical Bolivia. Med. Anthropol. 37, 236–252. doi: 10.1080/01459740.2017.1360878
Frank, D. N., Pace, N. R. (1998). RIBONUCLEASE p: Unity and diversity in a tRNA processing ribozyme. Annu. Rev. Biochem. 67, 153–180. doi: 10.1146/annurev.biochem.67.1.153
Furusawa, S., Shibata, H., Nishimura, H., Nemoto, S., Takayanagi, M., Takayanagi, Y., et al. (2000). Potentiation of doxorubicin-induced apoptosis of resistant mouse leukaemia cells by ivermectin. Pharm. Pharmacol. Commun. 6, 129–134. doi: 10.1211/146080800128735764
González, A., Becerra, N., Kashif, M., González, M., Cerecetto, H., Aguilera, E., et al. (2020). In vitro and in silico evaluations of new aryloxy-1,4-naphthoquinones as anti-trypanosoma cruzi agents. Medicinal Chemistry Research 29, 665–674. doi: 10.1007/s00044-020-02512-9
Guzzo, C. A., Furtek, C. I., Porras, A. G., Chen, C., Tipping, R., Clineschmidt, C. M., et al. (2002). Safety, tolerability, and pharmacokinetics of escalating high doses of ivermectin in healthy adult subjects. J. Clin. Pharmacol. 42, 1122–1133. doi: 10.1177/009127002401382731
Jaiteh, M., Taly, A., Hénin, J. (2016). Evolution of pentameric ligand-gated ion channels: Pro-loop receptors. PloS One 11, e0151934–e0151934. doi: 10.1371/journal.pone.0151934
Kadir, M. A., Aswad, H. S., Al-Samarai, A. M., Al-Mula, G. A. (2009). Comparison between the efficacy of ivermectin and other drugs in treatment of cutaneous leishmaniasis. Iraqi J. Vet. Sci. 23, 175-180.
Katsuno, K., Burrows, J. N., Duncan, K., Hooft van Huijsduijnen, R., Kaneko, T., Kita, K., et al. (2015). Hit and lead criteria in drug discovery for infectious diseases of the developing world. Nat. Rev. Drug Discovery 14, 751–758. doi: 10.1038/nrd4683
Kessler, R. L., Soares, M. J., Probst, C. M., Krieger, M. A. (2013). Trypanosoma cruzi response to sterol biosynthesis inhibitors: Morphophysiological alterations leading to cell death. PloS One 8 (1), e5549. doi: 10.1371/journal.pone.0055497
Laing, R., Gillan, V., Devaney, E. (2017). Ivermectin - old drug, new tricks? Trends Parasitol. 33, 463–472. doi: 10.1016/j.pt.2017.02.004
Landfear, S. M. (2019). Protean permeases: Diverse roles for membrane transport proteins in kinetoplastid protozoa. Mol. Biochem. Parasitol. 227, 39–46. doi: 10.1016/j.molbiopara.2018.12.006
Lespine, A., Martin, S., Dupuy, J., Roulet, A., Pineau, T., Orlowski, S., et al. (2007). Interaction of macrocyclic lactones with p-glycoprotein: Structure–affinity relationship. Eur. J. Pharm. Sci. 30, 84–94. doi: 10.1016/j.ejps.2006.10.004
Letunic, I., Khedkar, S., Bork, P. (2021). SMART: recent updates, new developments and status in 2020. Nucleic Acids Res. 49, D458–D460. doi: 10.1093/nar/gkaa937
Luna, K. P., Hernández, I. P., Rueda, C. M., Zorro, M. M., Croft, S. L., Escobar, P. (2009). In vitro susceptibility of trypanosoma cruzi strains from santander, Colombia, to hexadecylphosphocholine (miltefosine), nifurtimox and benznidazole. Biomed. Rev. Inst. Nac. Salud 29, 448–455.
Mayol, G. F., Revuelta, M. V., Salusso, A., Touz, M. C., Rópolo, A. S. (2020). Evidence of nuclear transport mechanisms in the protozoan parasite giardia lamblia. Biochim. Biophys. Acta BBA Mol. Cell Res. 1867, 118566. doi: 10.1016/j.bbamcr.2019.118566
Mendes, A. M., Albuquerque, I. S., Machado, M., Pissarra, J., Meireles, P., Prudêncio, M. (2017). Inhibition of plasmodium liver infection by ivermectin. Antimicrob. Agents Chemother. 61, e02005–e02016. doi: 10.1128/AAC.02005-16
Ménez, C., Mselli-Lakhal, L., Foucaud-Vignault, M., Balaguer, P., Alvinerie, M., Lespine, A. (2012). Ivermectin induces p-glycoprotein expression and function through mRNA stabilization in murine hepatocyte cell line. Biochem. Pharmacol. 83, 269–278. doi: 10.1016/j.bcp.2011.10.010
Mistry, J., Chuguransky, S., Williams, L., Qureshi, M., Salazar, G. A., Sonnhammer, E. L. L., et al. (2021). Pfam: The protein families database in 2021. Nucleic Acids Res. 49, D412–D419. doi: 10.1093/nar/gkaa913
Mosquillo, M. F., Bilbao, L., Hernández, F., Tissot, F., Gambino, D., Garat, B., et al. (2018). Trypanosoma cruzi biochemical changes and cell death induced by an organometallic platinum-based compound. Chem. Biol. Drug Des. 92, 1657–1669. doi: 10.1111/cbdd.13332
Nodari, R., Corbett, Y., Varotto-Boccazzi, I., Porretta, D., Taramelli, D., Epis, S., et al. (2020). Effects of combined drug treatments on plasmodium falciparum: In vitro assays with doxycycline, ivermectin and efflux pump inhibitors. PloS One 15(4), e0232171. doi: 10.1371/journal.pone.0232171
Nunes, M. C. P., Dones, W., Morillo, C. A., Encina, J. J., Ribeiro, A. L. (2013). Council on chagas disease of the interamerican society of cardiology 2013. chagas disease: an overview of clinical and epidemiological aspects. J. Am. Coll. Cardiol. 62, 767–776. doi: 10.1016/j.jacc.2013.05.046
Opara, W. E. K., I.G, A. (2005). Cutaneous leishmaniasis: A report of its treatment with mectizan in sokoto, Nigeria. J. Med. Sci 5, 186–188. doi: 10.3923/jms.2005.186.188
Panchal, M., Rawat, K., Kumar, G., Kibria, K. M., Singh, S., Kalamuddin, M., et al. (2014). Plasmodium falciparum signal recognition particle components and anti-parasitic effect of ivermectin in blocking nucleo-cytoplasmic shuttling of SRP. Cell Death Dis. 5, e994. doi: 10.1038/cddis.2013.521
Pérez-Molina, J. A., Molina, I. (2018). Chagas disease. Lancet 391, 82–94. doi: 10.1016/S0140-6736(17)31612-4
Rendon, G., Kantorovitz, M. R., Tilson, J. L., Jakobsson, E. (2011). Identifying bacterial and archaeal homologs of pentameric ligand-gated ion channel (pLGIC) family using domain-based and alignment-based approaches. Channels Austin Tex 5, 325–343. doi: 10.4161/chan.5.4.16822
Seguel, V., Castro, L., Reigada, C., Cortes, L., Díaz, M. V., Miranda, M. R., et al. (2016). Pentamidine antagonizes the benznidazole’s effect in vitro, and lacks of synergy in vivo: Implications about the polyamine transport as an anti-trypanosoma cruzi target. Experiment. Parasitol 171, 23–32. doi: 10.1016/j.exppara.2016.10.007
Sheele, J. M., Ford, L. R., Tse, A., Chidester, B., Byers, P. A., Sonenshine, D. E. (2014). The use of ivermectin to kill ixodes scapularis ticks feeding on humans. Wilderness Environ. Med. 25, 29–34. doi: 10.1016/j.wem.2013.09.008
Tang, J. (2017). Informatics approaches for predicting, understanding, and testing cancer drug combinations. Methods Mol. Biol. Clifton NJ 1636, 485–506. doi: 10.1007/978-1-4939-7154-1_30
Thomas, J., Peterson, G. M., Walton, S. F., Carson, C. F., Naunton, M., Baby, K. E. (2015). Scabies: an ancient global disease with a need for new therapies. BMC Infect. Dis. 15, 250. doi: 10.1186/s12879-015-0983-z
Turner, M. J., Schaeffer, J. M. (1989). Mode of action of ivermectin BT - ivermectin and abamectin. Ed. Campbell, W. C. (New York, NY: Springer New York), 73–88. doi: 10.1007/978-1-4612-3626-9_5
Udensi, U. K., Fagbenro-Beyioku, A. F. (2012). Effect of ivermectin on trypanosoma brucei brucei in experimentally infected mice. J. Vector Borne Dis. 49, 143.
Vincent, I. M., Creek, D. J., Burgess, K., Woods, D. J., Burchmore, R. J. S., Barrett, M. P. (2012). Untargeted metabolomics reveals a lack of synergy between nifurtimox and eflornithine against trypanosoma brucei. PloS Negl. Trop. Dis. 6, e1618. doi: 10.1371/journal.pntd.0001618
Wagstaff, K. M., Sivakumaran, H., Heaton, S. M., Harrich, D., Jans, D. A. (2012). Ivermectin is a specific inhibitor of importin α/β-mediated nuclear import able to inhibit replication of HIV-1 and dengue virus. Biochem. J. 443, 851–856. doi: 10.1042/BJ20120150
Wehbe, Z., Wehbe, M., Iratni, R., Pintus, G., Zaraket, H., Yassine, H. M., et al. (2021). Repurposing ivermectin for COVID-19: Molecular aspects and therapeutic possibilities. Front. Immunol. 12. doi: 10.3389/fimmu.2021.663586
World Health Organization (2015). Chagas disease in Latin America : an epidemiological update based on 2010 estimates. Wkly. Epidemiol. Rec. 90, 33–44.
Keywords: Chagas disease, ivermectin, drug repurposing, trypanocidal drug, drug combination, Trypanosoma cruzi
Citation: Fraccaroli L, Ruiz MD, Perdomo VG, Clausi AN, Balcazar DE, Larocca L and Carrillo C (2022) Broadening the spectrum of ivermectin: Its effect on Trypanosoma cruzi and related trypanosomatids. Front. Cell. Infect. Microbiol. 12:885268. doi: 10.3389/fcimb.2022.885268
Received: 27 February 2022; Accepted: 05 July 2022;
Published: 28 July 2022.
Edited by:
Vilma G. Duschak, Consejo Nacional de Investigaciones Científicas y Técnicas (CONICET), ArgentinaReviewed by:
Esteban Serra, National University of Rosario, ArgentinaAna Lia Mazzeti, Minas Gerais State University, Brazil
Copyright © 2022 Fraccaroli, Ruiz, Perdomo, Clausi, Balcazar, Larocca and Carrillo. This is an open-access article distributed under the terms of the Creative Commons Attribution License (CC BY). The use, distribution or reproduction in other forums is permitted, provided the original author(s) and the copyright owner(s) are credited and that the original publication in this journal is cited, in accordance with accepted academic practice. No use, distribution or reproduction is permitted which does not comply with these terms.
*Correspondence: Laura Fraccaroli, bGZyYWNjYXJvbGlAZnVuZGFjaW9uY2Fzc2FyYS5vcmcuYXI=; Carolina Carrillo, Y2NhcnJpbGxvQGNlbnRyb21pbHN0ZWluLm9yZy5hcg==
†These authors have contributed equally to this work and share first authorship