- 1Department of Central Laboratory, Department of Laboratory Medicine, the Second People’s Hospital of Lianyungang City (Cancer Hospital of Lianyungang), Lianyungang, China
- 2School of Biotechnology, Jiangsu University of Science and Technology, Zhenjiang, China
- 3Department of Acupuncture and Moxibustion, Lianyungang Affiliated Hospital of Nanjing University of Traditional Chinese Medicine, Lianyungang, China
Acinetobacter baumannii is a worldwide, primary cause of respiratory tract infections, septicemia, urinary apparatus infections, and secondary meningitis. It can be fatal. Rapid and accurate detection methods are needed to control the spread of carbapenem-resistant A. baumannii (CRAB). Current molecular diagnostic methods are limited and not suitable for on-site detection. In this study, an isothermal detection method using recombinase polymerase amplification (RPA) combined with a lateral flow strip (LFS) was developed to target the blaOXA-51 and blaOXA-23 genes of A. baumannii. The reaction was completed in about 40 min at 37°C. This method can also effectively distinguish A. baumannii and CRAB. The limit of detection of 100-101 CFU/reaction was equal to that of other detection methods. The detection accuracy was equal to that of the qPCR method with the use of clinical samples. The RPA-LFS assay is portable, rapid, and accurate and could replace existing detection methods for on-site detection of A. baumannii and CRAB.
Introduction
Acinetobacter baumannii is a common pathogen of nosocomial infections (Chung et al., 2011; Mirshekar et al., 2018). The 2020 China Antimicrobial Surveillance Network reported that number of infections caused by A. baumannii continues to increase, accounting for 17.07% of lower respiratory tract infections and a mortality rate of 35% (Shaheen et al., 2017). A. baumannii is categorized by the World Health Organization as among the most dangerous bacteria (Song et al., 2016; Tacconelli et al., 2018). In addition, A. baumannii is resistant to several antibiotics and, thus, has attracted the attention of microbiologists and doctors (Tekin et al., 2014). A. baumannii is a major cause of respiratory tract infections, septicemia, urinary apparatus infections, and secondary meningitis (Ranjbar et al., 2020). A. baumannii is widely distributed and can survive for long periods in hospital settings, thereby posing a serious threat to patients in the intensive care unit (Perez et al., 2007). Notably, carbapenem-resistant A. baumannii (CRAB) continues to rapidly spread globally (Villegas and Hartstein, 2003).
A. baumannii is categorized into five associated subgroups based on the production of oxacillinase (OXA): OXA-51, which is intrinsic, and OXA-143, OXA-58, OXA-40, and OXA-23, which are acquired (Woodford et al., 2006; Lee et al., 2009). CARB is resistant to various antimicrobial agents, mainly due to the production of OXA and metallo-β-lactamase (Azimi et al., 2013; Ei et al., 2019). The most common CRAB isolates produce OXA-23 carbapenemase (Chen et al., 2013). The blaOXA-51 gene is an established marker for detection of A. baumannii, while the blaOXA-23 gene is the most frequent carbapenemase gene detected in CRAB isolates (Turton et al., 2006; Chen et al., 2013; Djahmi et al., 2014; Karampatakis et al., 2017).
Rapid detection of CRAB can facilitate early treatment and minimize the severity of infection. Several diagnostic methods have been reported for the detection of A. baumannii, including loop-mediated isothermal amplification (LAMP), polymerase chain reaction (PCR), quantitative PCR (qPCR), and culture-based methods (Huang et al., 2012; Wang et al., 2014; Mu et al., 2016; Nirwati et al., 2018). Although these methods have unique advantages, all are limited by time requirements, low sensitivity, need for thermocycling equipment, and dependence on trained personnel. These drawbacks may inhibit the application of these methods in the field for everyday monitoring. To combat the extensive spread of CRAB, it is important to establish an on-site diagnostic method that is simple, rapid, accurate, and inexpensive.
Recombinase polymerase amplification (RPA) is an isothermal DNA amplification technology first reported in 2006 and widely used in recent years (Piepenburg et al., 2006). The RPA system relies on recombinase (UvsX and UvsY), single-stranded binding protein (gp32), and strand-displacing DNA polymerase (Bsu) for nucleic acid amplification. The reaction is completed in about 30 min at a constant temperature of 25–42°C, usually at 37°C, (Dong et al., 2020; Wang et al., 2020; Wang et al., 2022). The amplification products of RPA can be detected using gel electrophoresis, a fluorescence detector, and a lateral flow strip (LFS) (Khater et al., 2019; Ma et al., 2021; Wang et al., 2022). However, the sensitivity of gel electrophoresis and fluorescence detection is limited outside of a laboratory. On the contrary, a LFS is suitable for simple testing and the detection results can be analyzed visually without the need for complex thermocycling equipment and trained personnel (Li et al., 2020). The RPA-LFS assay can achieve a quick response time and good accuracy when used as a diagnostic test for a variety of infectious diseases (Dong et al., 2020; Yang et al., 2021).
In this study, an accurate RPA-LFS assay for detection of CRAB was established by designing specific primers and probes for detection of the blaOXA-51 and blaOXA-23 genes. This is the first report of the detection of A. baumannii in sputum with the use of the RPA-LFS assay and to distinguish CRAB via detection of A. baumannii (blaOXA-51 without OXA-23) and A. baumannii (blaOXA-51 and OXA-23). This method can confirm infection of CRAB, but not common A. baumannii, to facilitate early treatment and prevent severe illness.
Materials and Methods
Collection of Samples and DNA Extraction
A. baumannii and Candida albicans were obtained from the American Type Culture Collection (Manassas, VA, USA). In addition, isolates of A. baumannii (blaOXA-51 without OXA-23), isolates of A. baumannii (blaOXA-51 and OXA-23) strains, isolates of other Acinetobacter species, and isolates of common infectious pathogens were provided by The Second People’s Hospital of Lianyungang (Lianyungang, China). The sputum isolates of A. baumannii were collected from patients aged 20–50 years and hospitalised for at least one week. Swab of the wound, sputum, and urine clinical samples were obtained from the ICU hospitalized patients with clinically suspected multi-resistant infections. Information of all strains and samples are listed in Table 1. The identities of all isolates were confirmed by 16S rRNA PCR and qPCR (Huang et al., 2012; Misbah et al., 2015). All strains were cultured in Luria–Bertani broth at 37°C while shaking at 200 rpm. Cultures of 107 colony-forming units (CFU)/μL were boiled at 100°C for 10 min as DNA templates. The DNA templates were confirmed as originating from the respective pathogens by qPCR as described previously. The PCR products amplified with 16S rRNA primers were sequenced using the first generation sequencing techniques by ABI 3730XL Genetic Sequencer, and confirmed by General Biosystems Co. Ltd. (Anhui, China).
Design of Primers and Probes
Primers and probes for the RPA-LFS assay were designed to target the sequences of the blaOXA-51 gene (National Center for Biotechnology Information [NCBI] reference sequence: CP043953.1) and blaOXA-23 gene (NCBI reference sequence: NG_049525.1). Forward and reverse primers were designed with the Primer-Basic Local Alignment Search Tool (BLAST) (https://www.ncbi.nlm.nih.gov/tools/primer-blast/). The key parameter settings were as follows: minimum and maximum product sizes, 50 and 250 bp, respectively; minimum and maximum primer sizes, 31 and 35 nt, respectively; and minimum and maximum guanine-cytosine (GC) content, 20% and 70%, respectively. Other parameters were applied at default settings. The RPA amplification products were analyzed on a 1.5% agarose gel.
The probes were designed using Primer Premier 5 software (Premier Biosoft, Palo Alto, CA, USA) based on the sequences of regions defined by the selected primer pairs. The minimum and maximum sizes of the probes were 45 and 50 bp, the minimum and maximum melting temperatures (Tm) were 50°C and 100°C, and the minimum and maximum GC contents were 20% and 80%, respectively. In addition, if the probes and primers had three consecutive matching bases, the probes were mutated to avoid false-positive results.
RPA-LFS Procedure
RPA reactions were conducted in accordance with the manufacturer’s instructions of the Twist Amp® DNA Amplification nfo Kit (TwistDx Ltd., Maidenhead, UK). Each 25-μL reaction mixture contained 1.05 μL of each primer (10 μM), 0.3 μL of the probe (10 μM), 1.0 μL of the template, and other standard reaction components. Primers and probes were synthesized by General Biosystems Co. Ltd. To initiate the reaction, 1.25 μL of magnesium acetate (280 mM) were added. The reaction mixture was incubated for 30 min at 37°C. Then, 5 μL of the amplification product were spotted on the LFS (Ustar Biotechnologies Ltd., Hangzhou, China). The LFS was composed of a sample pad, conjugate pad (soaked with mouse-originated AuNP-tagged anti-FITC antibody), test line (coated with streptavidin), control line (coated with anti-mouse antibody), and absorbent pad that lined up through the solvent migration route. The RPA amplification product was added to the sample pad of the LFS and the stick of the LFS was inserted into 100 μL of the solvent (Ustar Biotechnologies Ltd.) for about 10 min until the test and control lines were visualized. Totally, the reaction is completed in 30 min isothermally at 37°C and the result can be observed on a LFS in 10 min.
To determine the suitability of the RPA-LFS assay to specifically detect blaOXA-51 and blaOXA-23, 20 A. baumannii (blaOXA-51 without OXA-23) and A. baumannii (blaOXA-51 without OXA-23) isolates from sputum were used as templates (107 CFU). The specificity of the primer-probe set blaOXA-51-F3/P/R2B was tested with the RPA-LFS assay using different sample templates of microbes isolated from sputum. Reference strains of C. albicans and A. baumannii were also tested (Table 1). The amount of the templates was set at 107 CFU. The LOD was first determined with pure A. baumannii (blaOXA-51 without OXA-23). The amount of template was tested at 107–100 CFU/μL (1 μL for each reaction).
qPCR Procedure
qPCR detection procedure of A. baumannii and CRAB were performed as previously reported (Martín-Peña et al., 2013). The primers blaOXA-51-qF (5’-GCA ACC ACC ACA GAA G-3’) and blaOXA-51-qR (5’-TCC AAT ACG ACG AGC T-3’) were designed to detect A. baumannii, while the primers blaOXA-23-qF (5’-ATC GGA TTG GAG AAC C-3’) and blaOXA-23-qR (5’-CCT GAT AGA CTG GGA CT-3’) were used to detect CRAB.
Results
Design of RPA Primers and Probes for CRAB Detection
The results showed that the four primer pairs produced no obvious primer-dependent artifacts when the DNA template was excluded (Figure 1A). The primer sets blaOXA-51-F2 and blaOXA-23-F2 were used to design the probes as 5’ end. Possible pairing between the probe and reverse primer of blaOXA-51 was analyzed to identify the cause of false-positive signals. False-positive signals could result if the probe and reverse primer share three or five consecutive matching bases (Figure 1B). To disrupt consecutive matching, two bases were substituted on the probe (C < G and T < G). Likewise, false-positive signals could result if the probe and reverse primer of blaOXA-23 shared three or four consecutive matching bases (Figure 1C), thus five bases were substituted on the probe (A < T, A < C, T < C, A < T, and A < G). Screening two more primers in front of probes according to the primer design principle, they were named as blaOXA-51-F3 and blaOXA-23-F3. The sequences of the primers and modified probes are listed in Table 2, where base substitutions are highlighted in red. The use of these two modified primer-probe sets for the RPA-LFS assay prevented false-positive signals with no DNA template control (NTC). Since the results indicate that the amplifications were not affected (Figure 1D), the modified primer-probe sets were used in this study.
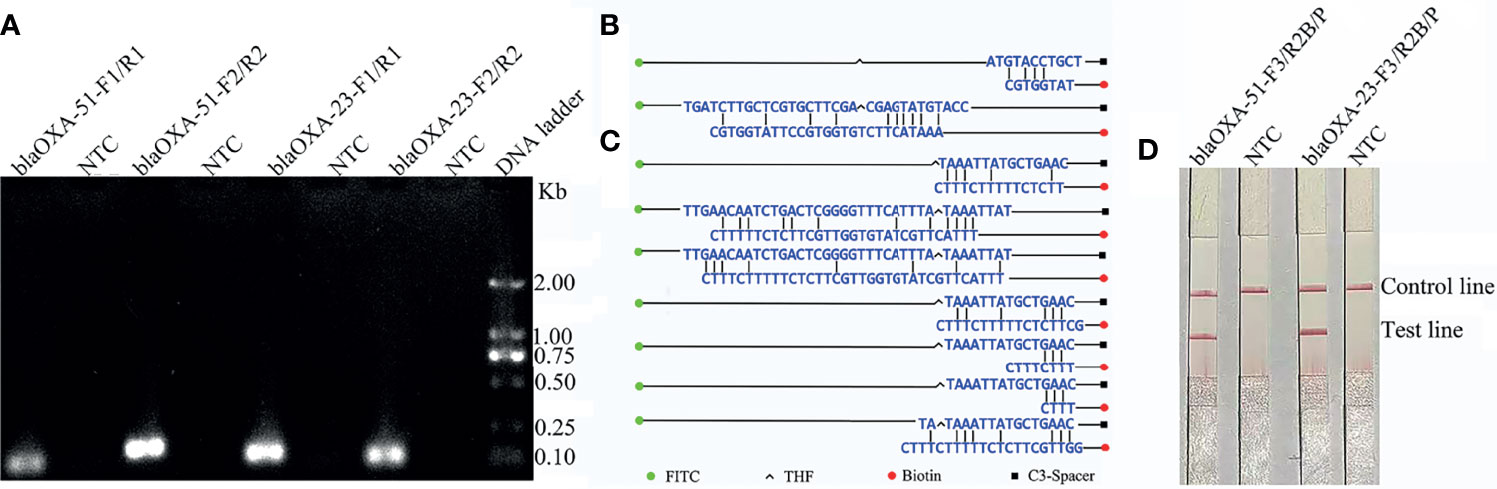
Figure 1 Screening of the primer-probe sets. (A) The RPA results of the four different primer sets targeting blaOXA-51 and blaOXA-23. The name of each set is shown at the top of each lane. A NTC was used in the reactions. All reactions were performed at 37°C for 30 min. The image represents the results of three independent experiments. (B, C) Pairing analysis and sequence modifications of the primer-probe sets for detection of blaOXA-51 and blaOXA-23 with Primer Premier 5 software. Relevant DNA bases of the probes and primers were excluded. The DNA strands are shown as horizontal lines and matching bases are indicated with vertical lines. Molecular markers are listed under the (C). (D) Effectiveness of the primer-probe sets for the RPA-LFS assay. The name of each set is shown at the top of each lane. A NTC was used in the reactions. The positions of the test and control lines are indicated on the right. All reactions were performed at 37°C for 30 min. The images represent the results of three independent experiments.
Suitability of the RPA-LFS Assay on blaOXA-51 and blaOXA-23
The use of the two primer-probe sets (blaOXA-51-F3/P/R2B and blaOXA-23-F3/P/R2B) to detect A. baumannii (blaOXA-51 without OXA-23) demonstrated that the primer-probe set targeting the blaOXA-23 gene did not yield a positive signal, while only the primer-probe set targeting the blaOXA-51 gene obtained a positive signal and the NTC did not yield a false-positive signal (Figure 2A). Then, the primer-probe sets blaOXA-51-F3/P/R2B and blaOXA-23-F3/P/R2B were used to detect the 10 A. baumannii (blaOXA-51 and OXA-23) isolates. All RPA-LFS reactions yielded positive signals without NTC. These results indicate that the primer-probe sets blaOXA-51-F3/P/R2B and blaOXA-23-F3/P/R2B can effectively distinguish strains coding for the blaOXA-51 and blaOXA-23 genes, respectively (Figure 2B). The suitability of the primer-probe sets used in this study was deemed good.
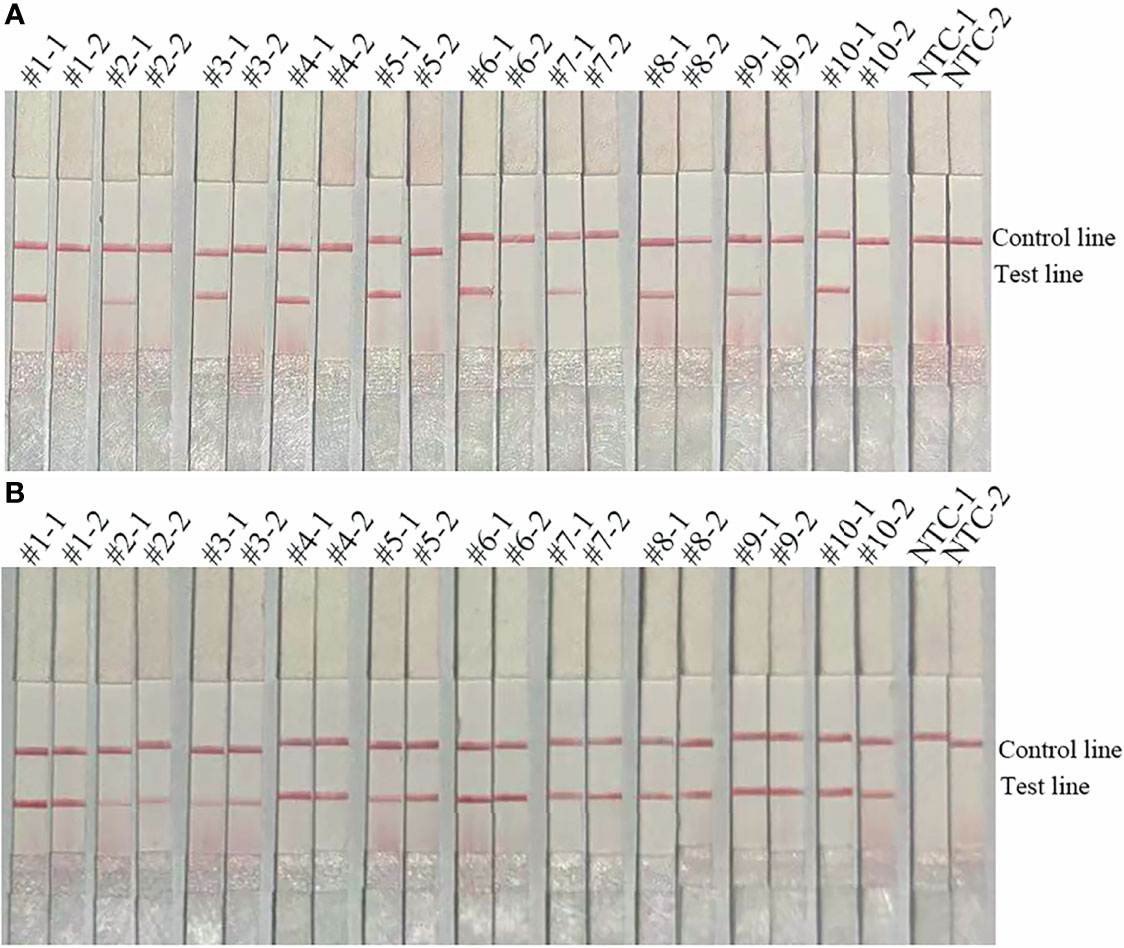
Figure 2 Applicability of the primer-probe sets. (A) The image shows the detection results of the RPA-LFS assay for 10 A. baumannii (blaOXA-51 without OXA-23) isolates using the primer-probe sets blaOXA-51-F3/R2B/P (#1-1, #2-1, #3-1, #4-1, #5-1, #6-1, #7-1, #8-1, #9-1, #10-1) and blaOXA-23-F3/R2B/P (#1-2, #2-2, #3-2, #4-2, #5-2, #6-2, #7-2, #8-2, #9-2, #10-2). (B) The image shows the detection results of the RPA-LFS assay for 10 A. baumannii (blaOXA-51 and OXA-23) isolates using the primer-probe sets blaOXA-51-F3/R2B/P (#1-1, #2-1, #3-1, #4-1, #5-1, #6-1, #7-1, #8-1, #9-1, #10-1) and blaOXA-23-F3/R2B/P (#1-2, #2-2, #3-2, #4-2, #5-2, #6-2, #7-2, #8-2, #9-2, #10-2). NTC-1, no template control with the primer-probe set blaOXA-51-F3/R2B/P. NTC-2, no template control with the primer-probe set blaOXA-23-F3/R2B/P. The name of each set is shown at the top of each lane. The positions of the test and control lines are indicated on the right. All reactions were performed at 37°C for 30 min. The images represent the results of three independent experiments.
Specificity of the RPA-LFS Assay for Detection of blaOXA-51
The primer-probe set blaOXA-51-F3/P/R2B showed good specificity (Figure 3). The primer-probe set blaOXA-51-F3/P/R2B targeting the blaOXA-51 gene was highly specific and, thus, used throughout the rest of the study.
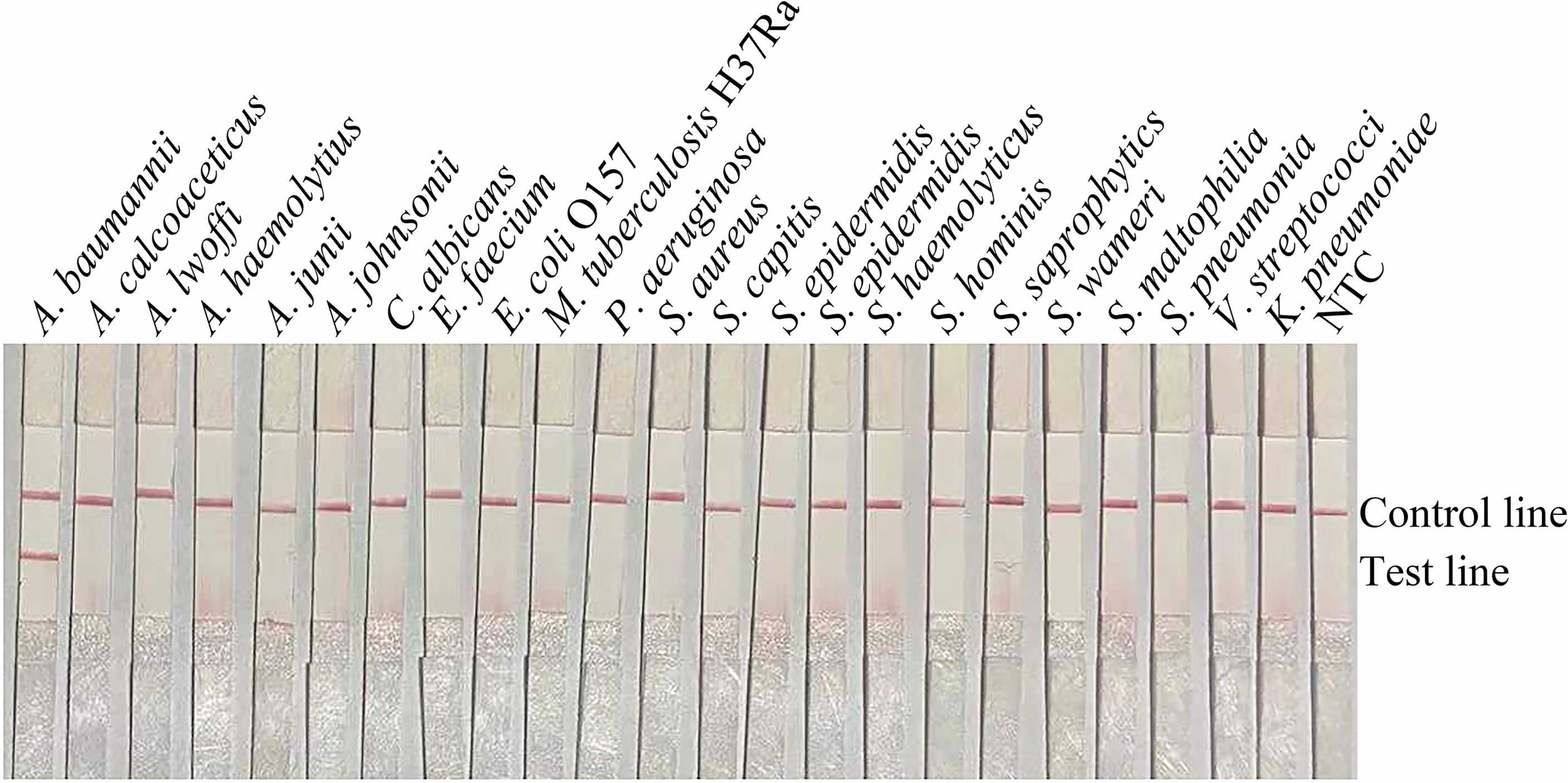
Figure 3 Specificity of the primer-probe sets. The image shows the detection results of the RPA-LFS assay with different bacterial templates using the primer-probe set blaOXA-51-F3/R2B/P. The name of the bacterium used for each reaction is shown at the top of each lane. NTC, no template control. The positions of the test and control lines are indicated on the right. All reactions were performed at 37°C for 30 min. The image represents the results of three independent experiments.
Limit of Detection (LOD) of the RPA-LFS Assay for the blaOXA-51 and blaOXA-23 Genes
The results of the RPA-LFS assay showed that the LOD was 100 CFU per reaction (Figure 4A). To mimic conditions of complex contamination, pure A. baumannii (blaOXA-51 without OXA-23) was spiked with 107 CFU/μL of Acinetobacter lwoffi and Escherichia coli O157. In addition, 107–100 CFU/μL of spiked A. baumannii (blaOXA-51 without OXA-23) were tested with the RPA-LFS assay. The results indicated that the RPA-LFS assay can tolerate interference from other bacteria and the LOD was 100 CFU/μL (Figure 4B). Thus, the LOD of the RPA-LFS assay for the blaOXA-51 gene was 100 CFU. Then, the LOD of the RPA-LFS assay for A. baumannii (blaOXA-51 and OXA-23) was tested using the primer-probe set blaOXA-23-F3/P/R2B against 107–100 CFU/μL of A. baumannii (blaOXA-51 and OXA-23) (1 μL for each reaction). The results showed that the LOD was 101 CFU per reaction (Figure 4C). In addition, the LOD of A. baumannii (blaOXA-51 and OXA-23) spiked with 107 CFU/μL of A. lwoffi and E. coli O157 was also 101 CFU/μL (Figure 4D).
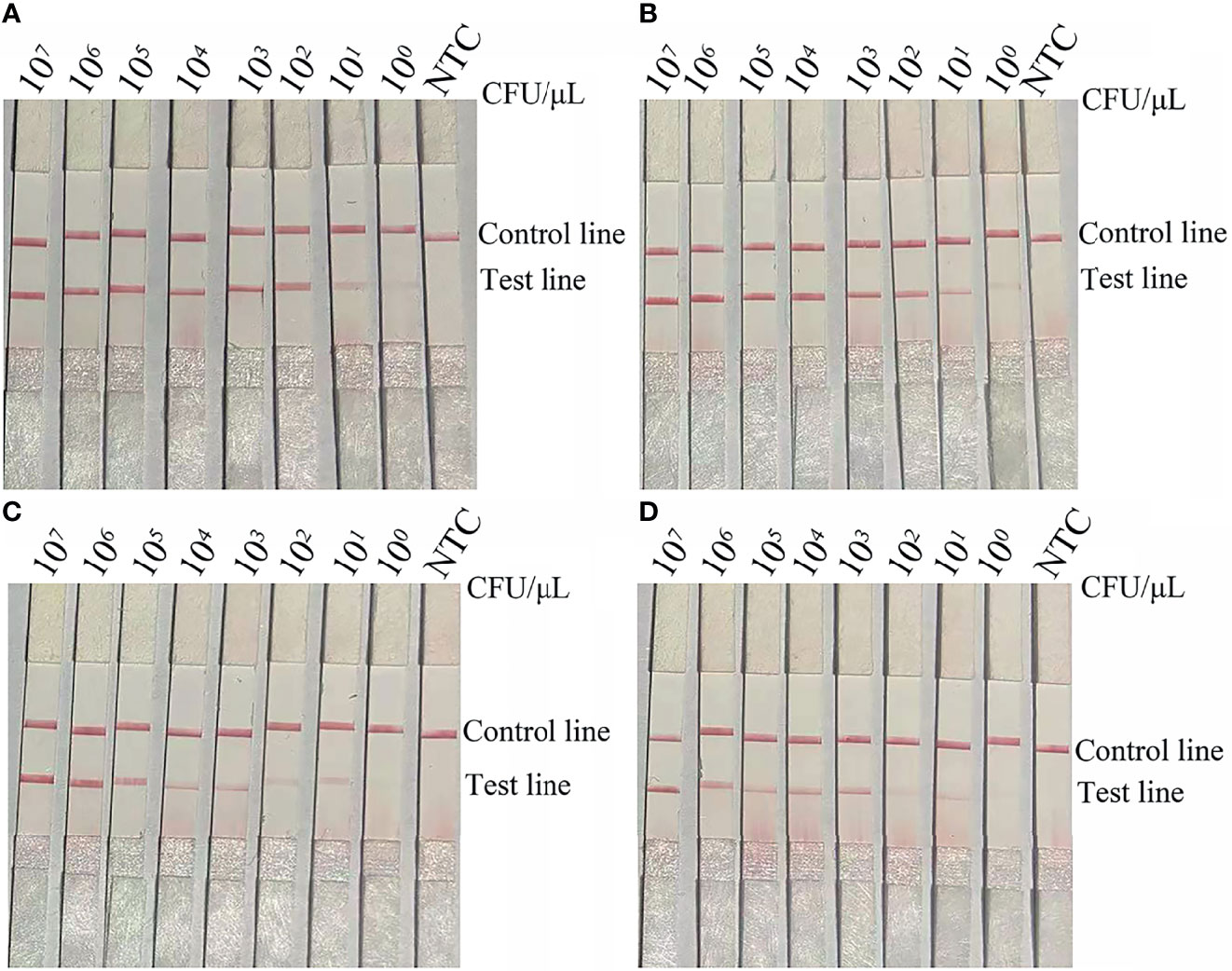
Figure 4 LOD of the RPA-LFS assay. (A) The image shows the detection results of the RPA-LFS assay with different CFUs of A. baumannii (blaOXA-51) using the primer-probe set blaOXA-51-F3/R2B/P. (B) The image shows the detection results of the RPA-LFS assay with different CFUs of A. baumannii (blaOXA-51) and 107 CFU of E. coli O157 using the primer-probe set blaOXA-51-F3/R2B/P. (C) The image shows the detection results of the RPA-LFS assay with different CFUs of A. baumannii (blaOXA-23) using the primer-probe set blaOXA-23-F3/R2B/P. (D) The image shows the detection results of the RPA-LFS assay with different CFUs of A. baumannii (blaOXA-23) and 107 CFU of E. coli O157 using the primer-probe set blaOXA-23-F3/R2B/P. NTC, no template control. All reactions were performed at 37°C for 30 min. The CFUs are indicated at the top of the strips. The positions of the test and control lines are indicated on the right. The images represent the results of three independent experiments.
Application of the RPA-LFS Assay for Detection of the blaOXA-51 and blaOXA-23 Genes in Clinical Samples
To mimic an actual application, the RPA-LFS assay was evaluated with 213 clinical samples. All samples were tested for the blaOXA-51 and blaOXA-23 genes with the RPA-LFS assay and compared with qPCR. The detection results of RPA-LFS were inconsistent with those of qPCR. In addition, the results showed that the detection rate of blaOXA-23 was 44.6% (Table 3).

Table 3 Prevalence of carbapenemase genes in 213 clinical samples of A. baumannii using RPA-LFS and PCR (summarized).
Discussion
CRAB poses a serious threat to hospitalized patients worldwide. CRAB infections in hospitals can cause highly mortality (Tekin et al., 2014; Shaheen et al., 2017). Thus, rapid and sensitive diagnosis of CRAB in the early stage of infection is important to ensure patient safety. Current detection methods, including PCR, qPCR, and LAMP, require specific equipment that is not readily available in smaller hospitals. In addition, long periods are required to obtain the results with these methods.
Molecular detection technologies require the selection of a diagnostic amplification target for effective detection of a particular species. Many studies have evaluated various methods for detection of A. baumannii infection in sputum samples. With these methods, the blaOXA-51 gene is most often used as the detection target (Abhari et al., 2021). Although specific to A. baumannii, the blaOXA-51 gene cannot be used to identify CRAB. Hence, the blaOXA-23 gene has been reported as a reliable target for detection of CRAB (Tekin et al., 2014). Therefore, two primer-probe sets were designed to detect A. baumannii and CRAB.
The results of this study indicated that base modifications had no obvious effects on the LOD and the RPA-LFS assay accurately detected A. baumannii and CRAB. The LOD of the RPA-LFS assay was 100 CFU for A. baumannii and 101 CFU for CRAB. This sensitivity was the same as that of the real-time RPA method, which was in the range of 100–101 CFU per reaction (Liu et al., 2020). In addition, the RPA-LFS assay for detection of CRAB was simple and fast, as detection can be completed within 40 min (30 min for amplification and 10 min for LFS analysis). This method requires an isothermal temperature of 37°C, which can be achieved by heating with the hands. The detection results can be easily read without instrumentation in accordance with relatively simple instructions. In contrast, the PCR, qPCR, and LAMP methods require temperature control equipment and relatively long periods for the reaction. The real-time RPA method requires a shorter time than PCR, but requires the use of a fluorescence detector. The total cost of real-time RPA is higher than that of the RPA-LFS assay.
Evaluation of clinical samples showed that the accuracy of the RPA-LFS assay was good. Testing of samples from different patients showed that detection of positive samples with the RPA-LFS was equal to that of qPCR, indicating that the RPA-LFS assay presents an alternative detection method. In addition, the two LFSs used to detect A. baumannii and CRAB can be combined into one LFS in the future, which will reduce the cost.
In conclusion, the established RPA-LFS assay is simple, rapid, and accurate, does not require a laboratory facility, and can be combined with a simple and fast DNA extraction method (heat boiling) for home detection of CRAB. Timely diagnosis can facilitate early treatment of nosocomial A. baumannii infections.
Data Availability Statement
The original contributions presented in the study are included in the article/supplementary material. Further inquiries can be directed to the corresponding authors.
Author Contributions
LW, GH, and XG designed the experiments and wrote the manuscript. YW, DS, and PZ collected the clinical samples. FW, DS, LC, KW, and LW performed the main experiments. YL, XL, and LW analyzed the data. All authors reviewed and approved the final version of the manuscript. All authors contributed to the article and approved the submitted version.
Funding
This study was supported by grants from the High-level Innovation and Entrepreneurship Talents Introduction Program of Jiangsu Province of China (grant number 2019-30345), the “521 Project” scientific research funding project of Lianyungang City (grant number LYG06521202157), the “HaiYan Plan” scientific research funding project of Lianyungang City (grant number 2019-QD-008), and the Clinical Medical Science and Technology Development Fund of Jiangsu University (grant number JLY20180020).
Conflict of Interest
The authors declare that the research was conducted in the absence of any commercial or financial relationships that could be construed as a potential conflict of interest.
Publisher’s Note
All claims expressed in this article are solely those of the authors and do not necessarily represent those of their affiliated organizations, or those of the publisher, the editors and the reviewers. Any product that may be evaluated in this article, or claim that may be made by its manufacturer, is not guaranteed or endorsed by the publisher.
Acknowledgments
We thank International Science Editing (http://www.internationalscienceediting.com) for editing this manuscript.
References
Abhari, S., Azizi, O., Modiri, L., Aslani, M., Assmar, M., Fereshteh, S., et al. (2021). Two New Rapid PCR-Based Methods for Identification of Acinetobacter baumannii Isolated From Clinical Samples. Mol. Cell. Probes 58, 101732. doi: 10.1016/j.mcp.2021.101732
Azimi, L., Lari, A., Talebi, M., Namvar, A., Jabbari, M. (2013). Comparison Between Phenotypic and PCR for Detection of OXA-23 Type and Metallo-Beta-Lactamases Producer Acinetobacter spp. GMS Hyg. Infect. Control 8 (2). doi: 10.3205/dgkh000216
Chen, Z., Liu, W., Zhang, Y., Li, Y., Jian, Z., Deng, H., et al. (2013). Molecular Epidemiology of Carbapenem-Resistant Acinetobacter Spp. From Xiang Ya Hospital, in Hunan Province, China. J. Basic Microbiol. 53 (2), 121–127. doi: 10.1002/jobm
Chung, D., Song, J., Kim, S., Thamlikitkul, V., Huang, S., Wang, H., et al. (2011). Asian Network for Surveillance of Resistant Pathogens Study Group. High Prevalence of Multidrug-Resistant Nonfermenters in Hospital-Acquired Pneumonia in Asia. Am. J. Respir. Crit. Care Med. 184 (12), 1409–1417. doi: 10.1164/rccm.201102-0349OC
Djahmi, N., Dunyach, C., Pantel, A., Dekhil, M., Sotto, A., Lavigne, J. (2014). Epidemiology of Carbapenemase-Producing Enterobacteriaceae and Acinetobacter baumannii in Mediterranean Countries. BioMed. Res. Int. 2014, 305784. doi: 10.1155/2014/305784
Dong, Y., Zhao, P., Chen, L., Wu, H., Si, X., Shen, X., et al. (2020). Fast, Simple and Highly Specific Molecular Detection of Vibrio alginolyticus Pathogenic Strains Using a Visualized Isothermal Amplification Method. BMC Vet. Res. 16 (1), 76. doi: 10.1186/s12917-020-02297-4
El, H., Nayme, K., El, H., Maroui, I., Sbiti, M., Zerouali, K., et al. (2019). Dissemination of Carbapenem-Resistant Acinetobacter baumannii Strains Carrying the blaGES, blaNDM and Blaoxa23 in Morocco. Germs 9 (3), 133–141. doi: 10.18683/germs.2019.1168
Huang, X., Cash, D., Chahine, M., Nikolich, M., Craft, D. (2012). Development and Validation of a Multiplex TaqMan Real-Time PCR for Rapid Detection of Genes Encoding Four Types of Class D Carbapenemase in Acinetobacter baumannii. J. Med. Microbiol. 61, 1532–1537. doi: 10.1099/jmm.0.045823-0
Karampatakis, T., Antachopoulos, C., Tsakris, A., Roilides, E. (2017). Molecular Epidemiology of Carbapenem-Resistant Acinetobacter baumannii in Greece: An Extended Revie-2015). Future Microbiol. 12, 801–815. doi: 10.2217/fmb-2016-0200
Khater, M., Escosura-Muñiz, A., Altet, L., Merkoçi, A. (2019). In Situ Plant Virus Nucleic Acid Isothermal Amplification Detection on Gold Nanoparticle-Modified Electrodes. Anal. Chem. 91 (7), 4790–4796. doi: 10.1021/acs.analchem.9b00340
Lee, Y., Turton, J., Chen, T., Wu, R., Chang, W., Fung, C., et al. (2009). First Identification of blaOXA-51-Like in Non-Baumannii acinetobacter spp. J. Chemother. 21 (5), 514–520. doi: 10.1179/joc.2009.21.5.514
Li, J., Macdonald, J., Stetten, F. (2020). Review: A Comprehensive Summary of a Decade Development of the Recombinase Polymerase Amplification. Analyst 144 (1), 31–67. doi: 10.1039/c8an01621f
Liu, S., Huang, G., Gong, Y., Jin, X., Meng, Y., Peng, Y., et al. (2020). Rapid and Accurate Detection of Carbapenem-Resistance Gene by Isothermal Amplification in Acinetobacter baumannii. Burns Trauma 2, 8. doi: 10.1093/burnst/tkaa026
Ma, C., Fan, S., Wang, Y., Yang, H., Qiao, Y., Jiang, G., et al. (2021). Rapid Detection of Enterocytozoon hepatopenaei Infection in Shrimp With a Real-Time Isothermal Recombinase Polymerase Amplification Assay. Front. Cell Infect. Microbiol. 11. doi: 10.3389/fcimb.2021.631960
Martín-Peña, R., Domínguez-Herrera, J., Pachón, J., McConnell, M. (2013). Rapid Detection of Antibiotic Resistance in Acinetobacter baumannii Using Quantitative Real-Time PCR. J. Antimicrob. Chemother. 68 (7), 1572–1575. doi: 10.1093/jac/dkt057
Mirshekar, M., Shahcheraghi, F., Azizi, O., Solgi, H., Badmasti, F. (2018). Diversity of Class 1 Integrons, and Disruption of carO and dacD by Insertion Sequences Among Acinetobacter baumannii Isolates in Tehran, Iran. Microb. Drug Resist. 24 (4), 359–366. doi: 10.1089/mdr.2017.0152
Misbah, S., Hassan, H., Yusof, M., Hanifah, Y., AbuBakar, S. (2015). Genomic Species Identification of Acinetobacter of Clinical Isolates by 16S rDNA Sequencing. Singapore Med. J. 46 (9), 461–464.
Mu, X., Nakano, R., Nakano, A., Ubagai, T., Kikuchi-Ueda, T., Tansho-Nagakawa, S., et al. (2016). Loop-Mediated Isothermal Amplification: Rapid and Sensitive Detection of the Antibiotic Resistance Gene ISAba1-blaOXA-51-Like in Acinetobacter baumannii. J. Microbiol. Methods 121, 36–40. doi: 10.1016/j.mimet.2015.12.011
Nirwati, H., Hakim, M., Darma, S., Mustafa, M., Nuryastuti, T. (2018). Detection of Blaoxa Genes and Identification of Biofilm-Producing Capacity of Acinetobacter Baumannii in a Tertiary Teaching Hospital, Klaten, Indonesia. Med. J. Malaysia 73 (5), 291–296.
Perez, F., Hujer, A., Hujer, K., Decker, B., Rather, P., Bonomo, R. (2007). Global Challenge of Multidrug-Resistant Acinetobacter baumannii. Antimicrob. Agents Chemother. 51 (10), 3471–3484. doi: 10.1128/AAC.01464-06
Piepenburg, O., Williams, C., Stemple, D., Armes, N. (2006). DNA Detection Using Recombination Proteins. PloS Biol. 4 (7), e204. doi: 10.1371/journal.pbio.0040204
Ranjbar, R., Zayeri, S., Mirzaie, A. (2020). Development of Multiplex PCR for Rapid Detection of Metallo-β-Lactamase Genes in Clinical Isolates of Acinetobacter baumannii. Iran J. Microbiol. 12 (2), 107–112. doi: 10.18502/ijm.v12i2.2615
Shaheen, A., Somayaji, R., Myers, R., Mody, C. (2017). Epidemiology and Trends of Cryptococcosis in the United States From 2000 to 2007: A Population-Based Study. Int. J. STD AIDS 29 (5), 453–460. doi: 10.1177/0956462417732649
Song, C., Hwee, J., Song, C., Tan, B., Chong, S. (2016). Burns Infection Profile of Singapore: Prevalence of Multidrug-Resistant Acinetobacter baumannii and the Role of Blood Cultures. Burns Trauma 21, 4–13. doi: 10.1186/s41038-016-0038-8
Tacconelli, E., Carrara, E., Savoldi, A., Harbarth, S., Mendelson, M., Monnet, D., et al. (2018). WHO Pathogens Priority List Working Group. Discovery, Research, and Development of New Antibiotics: The WHO Priority List of Antibiotic-Resistant Bacteria and Tuberculosis. Lancet Infect. Dis. 18 (3), 318–327. doi: 10.1016/S1473-3099(17)30753-3
Tekin, R., Dal, T., Bozkurt, F., Deveci, O., Palanc, Y., Arslan, E., et al. (2014). Risk Factors for Nosocomial Burn Wound Infection Caused by Multidrug Resistant Acinetobacter baumannii. J. Burn Care Res. 35 (1), e73–e80. doi: 10.1097/BCR.0b013e31828a493f
Turton, J., Woodford, N., Glover, J., Yarde, S., Kaufmann, M., Pitt, T. (2006). Identification of Acinetobacter baumannii by Detection of the blaOXA-51-Like Carbapenemase Gene Intrinsic to This Species. J. Clin. Microbiol. 44 (8), 2974–2976. doi: 10.1128/JCM.01021-06
Villegas, M., Hartstein, A. (2003). Acinetobacter Outbreak-2000. Infect. Control Hosp. Epidemiol. 24 (4), 284–295. doi: 10.1086/502205
Wang, H., Kim, S., Kim, J., Park, S., Uh, Y., Lee, H. (2014). Multiplex Real-Time PCR Assay for Rapid Detection of Methicillin-Resistant Staphylococci Directly From Positive Blood Cultures. J. Clin. Microbiol. 52 (6), 1911–1920. doi: 10.1128/JCM.00389-14
Wang, L., Wang, Y., Wang, F., Zhao, M., Gao, X., Chen, H., et al. (2022). Development and Application of Rapid Clinical Visualization Molecular Diagnostic Technology for Cryptococcus neoformans/C. gattii Based on Recombinase Polymerase Amplification Combined With a Lateral Flow Strip. Front. Cell Infect. Microbiol. 11. doi: 10.3389/fcimb.2021.803798
Wang, L., Zhao, P., Si, X., Li, J., Dai, X., Zhang, K., et al. (2020). Rapid and Specific Detection of Listeria monocytogenes With an Isothermal Amplification and Lateral Flow Strip Combined Method That Eliminates False-Positive Signals From Primer-Dimers. Front. Microbiol. 10. doi: 10.3389/fmicb.2019.02959
Woodford, N., Ellington, M., Coelho, J., Turton, J., Ward, M., Brown, S., et al. (2006). Multiplex PCR for Genes Encoding Prevalent OXA Carbapenemases in Acinetobacter spp. Int. J. Antimicrob. Agents 27 (4), 351–353. doi: 10.1016/j.ijantimicag.2006.01.004
Keywords: carbapenem-resistant Acinetobacter baumannii, recombinase polymerase amplification, lateral flow strip, blaOXA-51 gene, blaOXA-23 gene
Citation: Wang L, Sun D, Chen L, Zhou P, Wang K, Wang F, Lei X, Wang Y, Lu Y, Huang G and Gao X (2022) Development and Clinical Application of a Recombinase Polymerase Amplification-Lateral Flow Strip Assay for Detection of Carbapenem-Resistant Acinetobacter baumannii. Front. Cell. Infect. Microbiol. 12:876552. doi: 10.3389/fcimb.2022.876552
Received: 15 February 2022; Accepted: 17 March 2022;
Published: 11 May 2022.
Edited by:
Costas C. Papagiannitsis, University of Thessaly, GreeceReviewed by:
Katerina Tsilipounidaki, University of Thessaly, GreeceTheodoros Karampatakis, Papanikolaou General Hospital of Thessaloniki, Greece
Reem Mostafa Hassan, Cairo University, Egypt
Copyright © 2022 Wang, Sun, Chen, Zhou, Wang, Wang, Lei, Wang, Lu, Huang and Gao. This is an open-access article distributed under the terms of the Creative Commons Attribution License (CC BY). The use, distribution or reproduction in other forums is permitted, provided the original author(s) and the copyright owner(s) are credited and that the original publication in this journal is cited, in accordance with accepted academic practice. No use, distribution or reproduction is permitted which does not comply with these terms.
*Correspondence: Yingzhi Lu, ZG9jdG9ybHV5ekAxMjYuY29t; Guanhong Huang, aGdobHlnMDAwMkBzaW5hLmNu; Xuzhu Gao, YWxleGd3YW5AMTYzLmNvbQ==
†These authors have contributed equally to this work and share first authorship