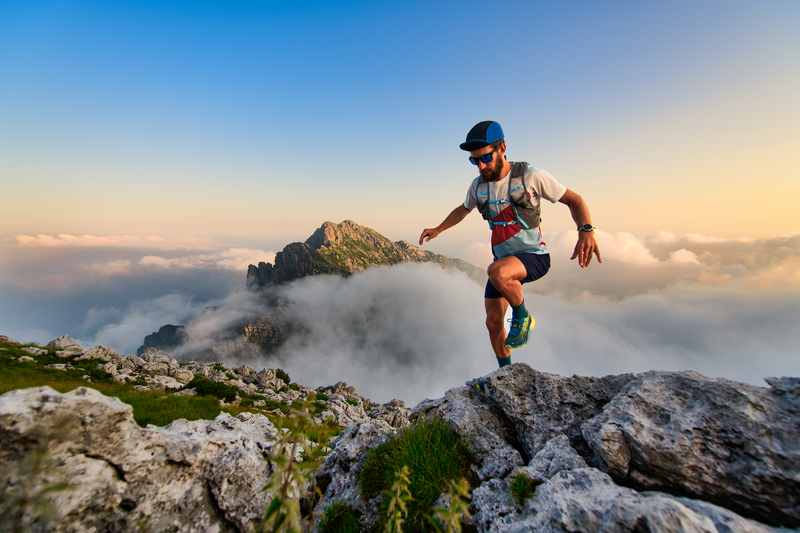
95% of researchers rate our articles as excellent or good
Learn more about the work of our research integrity team to safeguard the quality of each article we publish.
Find out more
ORIGINAL RESEARCH article
Front. Cell. Infect. Microbiol. , 07 July 2022
Sec. Clinical Microbiology
Volume 12 - 2022 | https://doi.org/10.3389/fcimb.2022.874401
This article is part of the Research Topic Developing Therapeutics for Antimicrobial Resistant Pathogens View all 12 articles
Objective: The study aimed to evaluate and compare the pharmacokinetic/pharmacodynamic (PK/PD) exposure to vancomycin in the novel optimal two-step infusion (OTSI) vs. intermittent infusion (II) vs. continuous infusion (CI) mode, for MRSA bloodstream infections occurring in critical patients.
Methods: With PK/PD modeling and Monte Carlo simulations, the PK/PD exposure of 15 OTSI, 13 II, and 6 CI regimens for vancomycin, at 1, 2, 3, 4, 5, and 6 g daily dose, was evaluated. Using the Monte Carlo simulations, the vancomycin population PK parameters derived from critical patients, the PD parameter for MRSA isolates [i.e., minimum inhibitory concentration (MIC)], and the dosing parameters of these regimens were integrated into a robust mdel of vancomycin PK/PD index, defined as a ratio of the daily area under the curve (AUC0–24) to MIC (i.e., AUC0–24/MIC), to estimate the probability of target attainment (PTA) of these regimens against MRSA isolates with an MIC of 0.5, 1, 2, 4, and 8 mg/L in patients with varying renal function. The PTA at an AUC0–24/MIC ratio of >400, 400–600, and >600 was estimated. A regimen with a PTA of ≥90% at an AUC0–24/MIC ratio of 400–600, which is supposed to maximize both efficacy and safety, was considered optimal.
Results: At the same daily dose, almost only the OTSI regimens showed a PTA of ≥90% at an AUC0–24/MIC ratio of 400–600, and this profile seems evident especially in patients with creatinine clearance (CLcr) of ≥60 ml/min and for isolates with an MIC of ≤2 mg/L. However, for patients with CLcr of <60 ml/min and for isolates with an MIC of ≥4 mg/L, the II regimens often displayed a higher or even ≥90% PTA at an AUC0–24/MIC ratio of >400 and of >600. The CI regimens frequently afforded a reduced PTA at an AUC0–24/MIC ratio of >400 and of >600, regardless of CLcr and MIC.
Conclusions: The data indicated that the OTSI regimens allowed preferred PK/PD exposure in terms of both efficacy and safety, and thus should be focused more on, especially in patients with CLcr of ≥60 ml/min and for isolates with an MIC of ≤2 mg/L.
Methicillin-resistant Staphylococcus aureus (MRSA) is a leading cause of infection worldwide, responsible for a wide range of both hospital and community-acquired infections. The most recent data regarding MRSA incidence, obtained from 85 (44%) of the World Health Organization member states, reported values exceeding 20% in all World Health Organization regions, and even 80% in some countries (Álvarez et al., 2019). The resulting infections due to MRSA often severely limit treatment options because MRSA is often cross-resistant to multiple existing antibiotics.
Some traditional alternatives to vancomycin for MRSA infections, such as trimethoprim–sulfamethoxazole, teicoplanin, daptomycin, linezolid, etc., and new antibiotics in the pipeline for MRSA therapy, such as ceftaroline, ceftobiprole, telavancin, dalbavancin, oritavancin, tedizolid, delafloxacin, radezolid, eravacycline, omadacycline, lefamulin, etc., exhibit good potency for MRSA infections (Lee et al., 2018); however, the traditional agents are unfortunately limited in practice since, compared with vancomycin, these drugs display nonnegligible disadvantages (e.g., inferior efficacy in S. aureus endovascular infections for trimethoprim–sulfamethoxazole, less suitability in acute severe infection for teicoplanin, ineffectiveness in pneumonia and central nervous system infections for daptomycin, and bone marrow suppression for linezolid) (Lee et al., 2018). Likewise, those new ones are also limited due to their geographical availability restrictions or unlisting (especially in resource-poor or low-ranking healthcare settings), non-licensing approval, or the lack of high-level evidence for MRSA treatment (Álvarez et al., 2019; Holubar et al., 2020). These predicaments preclude definitive conclusions regarding optimal therapy for such infections and often force clinicians to rely on the suboptimal options derived from high-dose or optimized regimens of existing antimicrobials extrapolated from PK/PD models.
This may be the case for vancomycin. Currently, in MRSA bloodstream infections occurring in critically ill patients, vancomycin is still recommended as a first-line antibiotic by the 2020 vancomycin therapeutic guideline issued by the American Society of Health-System Pharmacists (Rybak et al., 2020b), although the abovementioned potentially effective drugs exist (Lee et al., 2018) and the increase of MRSA isolates with high vancomycin MIC (i.e., ≥1 mg/L) has arisen over the past decade (European Committee on Antimicrobial Susceptibility Testing (EUCAST); The Micron Group). As described in the 2020 vancomycin therapeutic guideline (Rybak et al., 2020b), vancomycin, at an aggressive dosing strategy [i.e., a loading dose of 15 to 20 mg/kg, followed by daily maintenance continuous infusion (CI) of 30 to 40 mg/kg (up to 60 mg/kg)] which is derived from PK/PD prediction and aimed to achieve requisite PK/PD exposure (Rybak et al., 2020b), still remains the standard of care for MRSA infections occurring in critically ill patients, although the approved regimens of 2 g/day vancomycin have little evidence supporting its efficacy for MRSA infections due to isolates with an MIC of even 1 mg/L (Deryke and Alexander, 2009). Understandably, this infusion strategy for maintaining the role of vancomycin in the treatment of MRSA infections seems important.
However, this infusion strategy including CI may be a bit difficult to perform since CI often requires timely therapeutic drug monitoring and monitoring-based dose adjustment to maintain the desired drug exposure. These requirements, however, are often difficult to achieve due to the resistance of the patient to frequent blood sampling and in medical institutions where therapeutic drug monitoring devices are lacking. This results in a common phenomenon that clinicians prefer using the intermittent infusion (II) mode, although the CI mode has the advantages of safety, PK target, and steady-state attainment (Flannery et al., 2020; Yamada et al., 2020). Besides the CI and II modes, a novel infusion mode, i.e., the OTSI mode [a combined infusion mode with an initial loading-rate rapid infusion (LRRI) in the first step and afterwards with immediate low-rate continuous infusion (LRCI) in the second step], for vancomycin, has been recently presented in our previous study, and it showed great attractiveness in terms of PK/PD exposure in non-critically ill patients (Song et al., 2021).
Proverbially, critically ill patients often show distorted and high PK variability compared with non-critically ill patients (Di Giantomasso et al., 2003; del Mar Fernández de Gatta Garcia et al., 2007; Kees et al., 2011; The Surviving Sepsis Campaign Guidelines Committee including The Pediatric Subgroup* et al., 2013). This phenomenon may result in frequent insufficient vancomycin exposure (Udy et al., 2013; Roberts et al., 2014) and thus increased failure, especially when vancomycin is at an inappropriate infusion mode (since different infusion modes can have a large impact on drug exposure). Therefore, this group and changes in vancomycin exposure due to different infusion modes used in this population should be focused more on. However, it seems that few studies have focused on this issue. Thus, this study aimed to observe the PK/PD exposure of vancomycin at the CI vs. II vs. OTSI mode (Figure 1) for treating MRSA bloodstream infections occurring in critically ill patients to illustrate the concern of which infusion mode has sufficient superiority to resist MRSA infections occurring in critically ill patients, with the concurrent intent of defining optimal dosing regimens for such cases if possible.
Figure 1 Three infusion modes of vancomycin. (A) OTSI, optimal two-step infusion; “□”, loading-rate rapid infusion (LRRI) phase in OTSI mode; “◇”, low-rate continuous infusion (LRCI) phase in OTSI mode; “○”, elimination phase in OTSI mode. (B) II, intermittent infusion; τ, dosing interval; and (C) CI, continuous infusion. “↓”, start of the dose; AUC, area under the curve; C, drug concentration; Ctrough, trough concentration.
With the PK/PD modeling and Monte Carlo simulations, vancomycin population PK models derived from critically ill patients, MIC values fitting those reported in antimicrobial susceptibility testing, and dosing data fitting the clinical administration practice were incorporated as simulated variables into the mathematical model of AUC0–24/MIC to observe the probability of target attainment (PTA) provided by vancomycin regimens at the approved PK/PD target, defined as an AUC0–24/MIC ratio of 400–600. A 5,000-subject Monte Carlo simulation was performed on AUC0–24/MIC under these simulated variables. A regimen with a PTA of ≥90% at an AUC0–24/MIC ratio of 400–600 was optimal and acceptable. Based on the PTA obtained, (1) superiority of the three infusion modes and (2) determination of optimal or inferior regimens were further observed.
According to the 2020 vancomycin therapeutic guideline on MRSA infections published by the American Society of Health-System Pharmacists, an AUC0–24/MIC target of 400–600 (400 is for the efficacy threshold and 600 is for the safety ceiling) is presently recommended as the primary PK/PD target of vancomycin response considering the efficacy and safety, and traditional trough-only monitoring, with a target of 15–20 mg/L, is no longer recommended (Rybak et al., 2020b). Thus, an AUC0–24/MIC ratio of 400–600 was used as the optimal vancomycin PK/PD “efficacy” target in this study. Of note, this AUC0–24/MIC target value refers to the total rather than the free AUC0–24/MIC value, since they have been interchangeably reported (Rybak et al., 2009). Regarding the mathematical models, under different infusion mode, for calculating the AUC0–24/MIC value, they are derived from previous studies as follows.
(I) In the OTSI mode,
(II) In the II mode
(III) In the CI mode,
Note: (1) These equations were built based on steady state and one-compartment intravenous infusion model, (2) Equation 1 and 2 are derived and modified from our previous studies (Song et al., 2021; Song and Wu, 2022) and Equation 3 from the published literatures (Jeurissen et al., 2011; Rybak et al., 2020b).
Where Dvan (mg) is the daily dose, (mg/L) is the targeted steady-state concentration in the CI mode, AUC0–24 (mg·h/L) is the daily area under the concentration-time curve; MIC (mg/L) is the minimum inhibitory concentration; CLvan (L/h) is the vancomycin clearance; Vd (L) is the distribution volume; t1 (h) is the infusion time in LRRI phase of the OTSI mode; tinf (h) is the infusion time in the II mode; v1 (mg/h) is the zero-order infusion rate in LRRI phase of the OTSI mode, calculated as the dose in LRRI phase divided by t1; vII (mg/h) is the zero-order infusion rate in the II mode, calculated as each dose divided by tinf; vCI (mg/h) is the zero-order infusion rate in the CI mode, calculated as Dvan divided by 24 h; τ (h) is the dosing interval in the II mode; e is the natural constant.
Vancomycin population PK parameters (mainly CLvan and Vd) models constructed by Roberts et al. (2011), i.e., CLvan (L/h) = 4.58 × CLcr (ml/min)/100, and Vd (L/kg) = 1.53 × body weight (kg), were used for our analysis since these models (1) revealed good predictive performance for critically ill patients, with minimum mean prediction error of 5.1% [95% confidence interval: −1.2 to 11.4] and minimum median prediction error of −7.5% (95% confidence interval: −34.8 to 28) among six popular vancomycin models in an external validation evaluation (Guo et al., 2019); and (2) were derived from a large cohort study of including 206 intensive care unit patients with various degrees of renal function. Considering renal function changes in critically ill patients and the influence of body weight in Vd, various stages of CLcr ranging from 10 to 150 ml/min, with a 30 ml/min increment, were herein simulated, and an adult standard body weight of (mean of 65 kg ± standard deviation of 9.38 kg) (95% confidence interval; 40 to 100) was used for analysis in each stage of CLcr.
Considering the safety, generally per dose of ≤2 g and daily dose of ≤4 g for vancomycin are recommended when vancomycin was used in adults with normal renal function (Lodise et al., 2008; Filippone et al., 2017; Rybak et al., 2020a; USP). However, to predict the interest of increased doses in vancomycin exposure, higher doses of up to 6 g/day were studied in a previous study (del Mar Fernández de Gatta Garcia et al., 2007). Therefore, these doses would be simulated in this study. To accelerate targeted concentration attainment in critically ill patients, a loading dose of vancomycin of 15 to 20 mg/kg when the CI mode for vancomycin was used or even 20 to 35 mg/kg when the II mode for vancomycin was used can be considered according to the 2020 vancomycin therapeutic guideline (Rybak et al., 2020b). Understandably, in the OTSI mode, an initial loading dose of ≥1 g should be thus administered based on 65 kg of standard body weight. Usually, to minimize infusion-related adverse events, vancomycin should be diluted to ≤5 mg/ml and infused over ≥1 h or at a rate of 10 to 15 mg/min (≥1 h per 1 g) according to the 2020 vancomycin therapeutic guideline (Rybak et al., 2020b). Collectively, due to the limit of ≤2 g per dose and of ≥1 h infusion per 1 g, it is understandable that 2–3 h of conventional infusion time for a routine dose of vancomycin is the most frequent. Here, 34 dosing regimens, including 13 II, 15 OTSIs, and 6 CI regimens, are simulated and presented in Table 1, along with their dosing parameters.
Monte Carlo simulations, performed by the Oracle Crystal Ball software (version 11.1.2; Decisioneering, Inc., Denver, CO, USA) in this study, were used to estimate the PTA of each regimen against isolates with an MIC of 0.5, 1, 2, 4, and 8 mg/L at an AUC0–24/MIC target of >400, 400–600, and >600. Regarding the application of the Monte Carlo simulation method in PK/PD study of antibiotics and the principles, software application, and specific implementation of this method, it has been well studied and described elsewhere (Moine et al., 2016; Song and Long, 2018; Song et al., 2021). Briefly, the Monte Carlo simulation method includes the following four steps: (1) setting the distribution patterns of the simulated variables according to their characteristics; (2) setting the confidence interval; (3) incorporating the simulated variables into the mathematical model of AUC0–24/MIC; and (4) performing the Monte Carlo simulations on AUC0–24/MIC and exporting the PTA values.
Since the Monte Carlo simulation method simulates thousands of patients at given simulated parameters, it is important to acknowledge assumptions made regarding the variability in these parameter estimates. Based on the characteristics of the simulated variables, herein an uniform distribution for CLcr, vII, v1, v2, t1, t2, tinf, a log-normal distribution for body weight, and a custom distribution for Dvan, vCI, tCI, and MIC, were assumed. For example, for infected patients with a body weight of 65 ± 9.38 kg and a CLcr of 60–90 ml/min due to MRSA isolates with an MIC of 0.5 mg/L, if the II regimen of 0.25 g q 6 h (i.e., Dvan = 1 g) was used, a uniform distribution for CLcr in the interval of 60–90 ml/min, for vII in the interval of 83–125 mg/h and for tinf in the interval of 2–3 h, a log-normal distribution of 65 ± 9.38 kg for body weight, and a custom distribution with a probability of 100% for Dvan at 1 g and for MIC at 0.5 mg/L, were assumed. The confidence interval was set at 95%. With the incorporation of these parameters into the mathematical model of AUC0–24/MIC, a 5,000-subject Monte Carlo simulation was performed on AUC0–24/MIC to obtain PTA-AUC0–24/MIC diagrams with AUC0–24/MIC as the abscissa and PTA as the ordinate. The PTA at the AUC0–24/MIC target was obtained by assigning the abscissa as the designated target value. In Monte Carlo simulations, the PTA, i.e., the likelihood of a dosage regimen resisting the bacterial isolate at a designated AUC0–24/MIC target, is often used to measure the clinical acceptability of a dosage regimen. A regimen with the highest PTA would be optimal as it would provide the highest likelihood of obtaining the targeted exposure for the infectious isolate. Herein, a regimen that maximized the PTA of simulated patients to at least 90% at an AUC0–24/MIC ratio of 400–600, which is supposed to maximize both efficacy and safety, was defined as optimal. Regimen that achieved a PTA of ≥90% at an AUC0–24/MIC ratio of >400 but <90% (better as low as possible) at an AUC0–24/MIC ratio of >600, which is supposed to ensure efficacy but relatively reduce safety, was defined as inferior. Based on the PTA obtained, (1) superiority of the three infusion modes and (2) determination of optimal or inferior regimens were further observed.
The PTA of 34 dosage regimens at various CLcr and MICs under an AUC0–24/MIC ratio of >400, 400–600, and >600 is displayed in Figure 2. It can be seen that, under an AUC0–24/MIC ratio of 400–600 and for MRSA isolates with an MIC of ≤1 mg/L, only the OTSI regimen of 0.5 g LRRI + 0.5 g LRCI for isolates with an MIC of 0.5 mg/L, 1 g LRRI + 1 g LRCI for isolates with an MIC of 1 mg/L in patients with CLcr of >90 ml/min, and the CI regimen of 3 g q 24 h for isolates with an MIC of 1 mg/L in patients with a CLcr of 120–150 ml/min, yielded a PTA of ≥90%.
Figure 2 PTA values of 34 dosage regimens for various MICs and CLcr at various AUC0–24/MIC targets. AUC0–24, daily area under the curve; MIC, minimum inhibitory concentration; AUC0–24/MIC, ratio of daily area under the curve to minimum inhibitory concentration; CLcr, creatinine clearance; LRRI, loading-rate rapid infusion in OTSI mode; LRCI, low-rate continuous infusion in OTSI mode.
Under an AUC0–24/MIC ratio of 400–600 and for MRSA isolates with an MIC of ≥2 mg/L, (I) in patients with a CLcr of ≥90 ml/min, all vancomycin regimens at ≤3 g/day failed to achieve a PTA of ≥90%, regardless of the infusion mode. Vancomycin 4 g/day at the OTSI regimen of 1 g LRRI + 3 g LRCI, 1.5 g LRRI + 2.5 g LRCI and 2 g LRRI + 2 g LRCI in patients with CLcr of >90 ml/min, 5 g/day at the CI regimen of 5 g q 24 h in patients with CLcr of 90–120 ml/min, and 6 g/day at the CI regimen of 6 g q 24 h in patients with CLcr of 120–150 ml/min for MRSA isolates with an MIC of 2 mg/L, afforded a PTA of ≥90%. However, no regimen obtained this optimal PTA for those with an MIC of 4 mg/L; (II) in patients with CLcr of 60–90 ml/min, only the OTSI regimen of 1 g LRRI + 2 g LRCI for isolates with an MIC of 2 mg/L, 1 g LRRI + 5 g LRCI, 1.5 g LRRI + 4.5 g LRCI and 2 g LRRI + 4 g LRCI for isolates with an MIC of up to 4 mg/L, reached the desired PTA; (III) in patients with CLcr of <60 ml/min, no regimen provided a PTA of ≥90%, regardless of the doses, the MICs and infusion mode.
Under an AUC0–24/MIC ratio of >400 and >600 and for MRSA isolates with an MIC of ≤1 mg/L, all of the vancomycin regimens at ≥3 g/day achieved a PTA of ≥90% under an AUC0–24/MIC ratio of >400, and almost all of these regimens reached this PTA under an AUC0–24/MIC ratio of >600, regardless of the infusion mode and CLcr. No regimen at 1 g/day in patients with CLcr of ≥60 ml/min reached a PTA of ≥90% under an AUC0–24/MIC ratio of >400. However, the II regimen of 0.25 g q 6 h and 0.5 g q 12 h in patients with CLcr of 60–90 ml/min, and the OTSI regimen of 0.5 g LRRI + 0.5 g LRCI in patients with CLcr of 30–10 ml/min achieved the requisite PTA under an AUC0–24/MIC ratio of >400 but failed under an AUC0–24/MIC ratio of >600. All of the vancomycin regimens at 2 g/day obtained a PTA of ≥90% under an AUC0–24/MIC ratio of >400 except the II regimen of 1 g q 12 h and the CI regimen of 2 g q 24 h in patients with a CLcr of ≥90 ml/min. However, these regimens also achieved this PTA under an AUC0–24/MIC ratio of >600 in patients with a CLcr of <60 ml/min, except for the CI regimen of 2 g q 24 h.
Under an AUC0–24/MIC ratio of >400 and >600 and for MRSA isolates with an MIC of ≥2 mg/L, all vancomycin regimens at ≥4 g/day for isolates with an MIC of 2 mg/L reached a PTA of ≥90% under an AUC0–24/MIC ratio of >400 except the II regimens of 1 g q 6 h and 2 g q 12 h and the CI regimens of 4 g q 24 h and 5 g q 24 h in patients with a CLcr of ≥90 ml/min. Unexpectedly, these regimens also achieved this PTA under an AUC0–24/MIC ratio of >600 in patients with a CLcr of <60 ml/min. For isolates with an MIC of 4 mg/L, vancomycin regimens at ≥5 g/day in patients with CLcr of only <60 ml/min and those at 6 g/day in patients with CLcr of only <90 ml/min yielded the optimal PTA under an AUC0–24/MIC ratio of >400, and some of these regimens, such as 1.25 g q 6 h, 1.67 g q 8 h, 1.5 g q 6 h, and 2 g q 8 h in patients with CLcr of <60 ml/min provided the desired PTA under an AUC0–24/MIC ratio of >600. However, these high-dose regimens in patients with CLcr of 30–10 ml/min, achieved a PTA of ≥90% for MRSA isolates with an MIC of up to 8 mg/L under an AUC0–24/MIC ratio of even > 600.
It can be seen from Figure 2 that relative to the II and CI regimens with the same daily dose, only the OTSI regimen reached a PTA of ≥90% under a safe and effective PK/PD target (i.e., an AUC0–24/MIC ratio of 400–600), and this profile seems evident especially in patients with a CLcr of ≥60 ml/min and for MRSA isolates with an MIC of ≤2 mg/L. These findings suggest that the OTSI mode has certain advantages in terms of efficacy and safety. However, little superiority was shown in patients with a CLcr of <60 ml/min and for MRSA isolates with an MIC of ≥4 mg/L. In contrast, the II regimens for these patients and these isolates often displayed a superior and even ≥90% PTA under an AUC0–24/MIC ratio of >400 relative to the OTSI and CI regimens, implying that the II mode exhibited an extrapolated increase in terms of efficacy. However, these regimens also displayed a higher or even ≥90% PTA under an AUC0–24/MIC ratio of >600, implying that the II mode exhibited a concomitant increase in terms of safety risk. Interestingly, the CI regimens frequently afforded a reduced PTA under an AUC0-24/MIC ratio of >400 and of >600, regardless of the CLcr and MICs. This implied that the CI mode presented reduced efficacy and safety risk. Contrastively, the OTSI mode allowed the optimal PK/PD target attainment with both efficacy and safety.
In the absence of better options for treating MRSA bloodstream infections occurring in critically ill patients, this study summarizes the optimal or inferior vancomycin regimens that we considered may be effective based on the PTA obtained. Table 2 displays these regimens for such infections occurring in critically ill patients with different CLcr and caused by MRSA isolates with different MICs.
Table 2 The optimal or inferior vancomycin regimens that the present study considered may be effective for the treatment of MRSA bloodstream infections occurring in critically ill patients, at different CLcr and MICs.
To our knowledge, this is the first study to evaluate the PK/PD exposure of vancomycin at CI, II, and OTSI modes, for MRSA bloodstream infections occurring in critically ill patients with various CLcr and caused by MRSA isolates with different MICs. The data here supported that in critically ill patients: (1) the II mode displayed competitiveness for MRSA isolates with an MIC of ≥4 mg/L in efficacy but also increased risk in safety; (2) the CI mode presented no superiority in efficacy but reduced risk in safety; and (3) the OTSI mode showed certain advantages for MRSA isolates with an MIC of ≤2 mg/L in both efficacy and safety. The data, included in Table 2, can better inform tentative vancomycin regimens for treating MRSA infections occurring in critically ill patients in the absence of better options for such infections.
In critically ill patients, more current studies on vancomycin II vs. CI have focused mainly on the comparison in terms of the efficacy and safety of vancomycin (Vuagnat et al., 2004; Hutschala et al., 2009; Akers et al., 2012; Saugel et al., 2013; Schmelzer et al., 2013; Hanrahan et al., 2014; Tafelski et al., 2015; Bissell et al., 2020). However, comparative studies on the outcomes of vancomycin II vs. CI against MRSA isolates with a specific MIC are still scarce. A study (Akers et al., 2012) compared the clinical outcomes of vancomycin at the II regimen of 1 g q 8 h and the CI regimen of 3 g/day used in critically ill patients and indicated that an average of 2.3 g/day vancomycin, at the II regimens or with the II mode, fell below a trough of 15 mg/L more than half of the time. It implied that this dosage was insufficient and poor efficacy was thus obtained. However, this study did not provide the outcomes of vancomycin II vs. CI against MRSA isolates with a specific MIC. Theoretically, a vancomycin level of 25 mg/L for increasing MICs (≥1 mg/L) in S. aureus seems more appropriate (Wang et al., 2006), and would maintain an AUC0–24/MIC ratio of ≥400 against isolates with an MIC of 1.5 mg/L, assuming constant vancomycin levels over 24 h. To achieve this level, vancomycin 3 g/day, if with the CI mode, should be required when MRSA infections occur in critically ill patients with a CLcr of ≥120 ml/min (Jeurissen et al., 2011). Similarly, the data presented in the present study suggested that in such critically ill patients, 3 g/day vancomycin, with the CI mode, obtained a PTA of ≥90% for isolates with an MIC of 1 mg/L under an AUC0–24/MIC ratio of >400; however, whether this regimen (i.e., 3 g q 24 h) can achieve this optimal PTA for an MIC of 1.5 mg/L has not been studied. However, for critically ill patients with a CLcr of <60 ml/min, this regimen achieved a PTA of ≥90% for isolates with an MIC of up to 2 mg/L under an AUC0–24/MIC ratio of >400. Another study, which was aimed to evaluate the clinical outcomes of vancomycin CI vs. II in the treatment of severe staphylococcal infections, observed vancomycin response on 40 randomly selected strains (of which 18 in II group and 22 in CI group) with an MIC of ≤2 mg/L (Wysocki et al., 2001). In this study, although 31 cases were treated successfully, the kinds of MICs and the II or CI regimens were not reported. The current lack of comparative studies on clinical outcomes of vancomycin II vs. CI against isolates with a specific MIC has impeded the comparison of the superiority of the II mode vs. CI mode, especially against those with high MICs. However, this study indicated that the II mode for vancomycin displayed its competitiveness against isolates with an MIC of ≥4 mg/L relative to the CI mode and may therefore be a preferred dosing strategy in such cases when alternatives to vancomycin are unavailable.
Of interest, in most current clinical studies in vancomycin, one challenge when evaluating its clinical outcomes is preferring reporting of concentration—rather than AUC-indicated vancomycin exposure, regardless of vancomycin in the CI (usually reporting steady-state concentration) or II (usually reporting trough concentration) mode. However, these reported concentration values may not be adequate surrogates for AUC-indicated efficacy exposure in critically ill patients (Turner et al., 2018), as the AUC is the integrated quantity of cumulative drug exposure (i.e., the serum drug concentration-time curve over a defined interval), while the trough represents a single exposure point at the end of the dosing interval. Moreover, in clinical practice, monitoring of trough concentrations will be often be translated into the achievement of one specific minimum daily AUC value (Rybak et al., 2020b). Although trough-only monitoring is practical, the potential limitations surrounding the practice suggest that trough monitoring is insufficient to guide vancomycin dosing in all patients (Rybak et al., 2020b).
Nevertheless, in some simulated studies, AUC- or AUC/MIC-based vancomycin exposure was partially observed. A Monte Carlo simulation study conducted by del Mar Fernández de Gatta Garcia et al. (2007) indicated that in critically ill patients with a mean CLcr of 65.5 ml/min, 3–4 g/day vancomycin, if with the II mode, against isolates with an MIC of 1 mg/L, would be required to provide a PTA of 90% under an AUC0–24/MIC ratio of 400, thus questioning the standard regimens of 2 g/day vancomycin II against isolates with such MICs. However, this study suggested that in critically ill patients with a CLcr of 60–90 ml/min, 2 g/day vancomycin at the II regimen of 0.5 g q 6 h or 1 g q 12 h and 3–4 g/day vancomycin at a II regimen of 1 g q 8 h or 2 g q 12 h against isolates with an MIC of 1 and 2 mg/L, respectively, is sufficient to achieve a PTA of 90%. Another Monte Carlo simulation study, conducted by Setiawan et al., (2019), on vancomycin exposure against MRSA, reported that in patients with CLcr of 60–120 ml/min, 2 g/day vancomycin at the II regimen of 1 g q 12 h, 3 g/day vancomycin at a II regimen of 1 g q 8 h or 1.5 g q 12 h and 4 g/day vancomycin at a II regimen of 1 g q 6 h or 2 g q 12 h provided a PTA of 100% for an MIC of 0.5 mg/L at an AUC0–24/MIC ratio of 400. However, if with II mode 3 g/day vancomycin for an MIC of 1 mg/L and 4 g/day vancomycin for an MIC of 1.5 mg/L should be required for attaining a PTA of ≥90%, thus doubting the approved 2 g/day vancomycin, at the II regimens, against isolates with such MICs. Consistently, these regimens displayed similar outcomes in the present study, especially for patients with a CLcr of 120–150 ml/min. Inconsistently, however, 2 g/day vancomycin at the II regimen of 0.5 g q 6 h for an MIC of 1 mg/L and 4 g/day vancomycin at a II regimen of 1 g q 6 h for an MIC of up to 2 mg/L exhibited a PTA of nearly 100% in patients with CLcr of 60–120 ml/min. Discordance of the results between these and this study may be due to the used vancomycin PK models. The study by del Mar Fernández de Gatta Garcia et al. (2007) used a PK model of CLvan (ml/min/kg) = 0.660 − 0.016 × age(years) − 0.006 × Acute Physiology and Chronic Health Evaluation System score + 0.380 × serum albumin (g/dl) + 0.562 × CLcr (ml/min/kg) and the study by Setiawan et al., (2019) used a PK model of CLvan (L/h) = 0.0444 × CLcr (ml/min) to predict PTA of vancomycin regimens. Herein, a PK model of CLvan (L/h) = 4.58 × CLcr (ml/min)/100 was used. Understandably, using different PK models may result in different predicted results. We believe that the data herein are believable because the chosen vancomycin PK models were considered to have broad applicability for critical population since these models were derived from a large cohort study of including 206 intensive care unit patients with various degree of renal function, and revealed good predictive performance for critically ill patients (Guo et al., 2019).
Eguchi et al. proposed the strategy of optimal two-step infusion therapy and established the corresponding PK/PD index model in 2010 (Eguchi et al., 2010). However, this strategy was for time-dependent antibiotics. OTSI mode is a new infusion mode recently proposed and built for vancomycin, a concentration-dependent antibiotic, in our previous study (Song et al., 2021). Currently, little clinical data on this infusion mode exist. However, its theoretical superiority was exhibited both in efficacy and safety, and both in critically ill patients and non-critically ill patients. The present study indicated that at the same daily dose, almost only the OTSI regimens showed a PTA of ≥90% at an AUC0–24/MIC ratio of 400–600, especially for patients with a CLcr of ≥60 ml/min and against isolates with an MIC of ≤2 mg/L. It implies that this infusion mode maximizes both the efficacy and safety of vancomycin. Although the II regimens displayed a higher or even ≥90% PTA at an elevated MIC of ≥4 mg/L under an AUC0–24/MIC ratio of >400, they also obtained this PTA under an AUC0–24/MIC ratio of >600. It suggested that the II mode presented concomitant increased efficacy and safety risks. The CI regimens did not afford a higher PTA at an AUC0–24/MIC ratio of >400 but obtained a reduced PTA at an AUC0–24/MIC ratio of >600, implying that no increase in efficacy but a lower risk of safety was displayed. This outcome in the CI mode for vancomycin is consistent with that obtained by previous studies (Van Herendael et al., 2012; Schlobohm et al., 2021). In non-critically ill patients, 2 g/day vancomycin at the OTSI regimen of 1.95 g LRRI + 0.05 g LRCI and 4 g/day vancomycin at the OTSI regimen of 2 g LRRI + 2 g LRCI for an MIC of up to 2 mg/L and 4 mg/L, respectively, achieved a PTA of ≥90% at an AUC0–24/MIC ratio of 400, but failed if at the II regimens (Song et al., 2021). This suggests the superiority of OTSI mode in improving efficacy. However, reduced PK/PD target attainment is still observed in critically ill patients compared with non-critically ill patients. This may be due to the distorted vancomycin PK variability in critically ill patients, as demonstrated in previous studies (Di Giantomasso et al., 2003; del Mar Fernández de Gatta Garcia et al., 2007; Kees et al., 2011; The Surviving Sepsis Campaign Guidelines Committee including The Pediatric Subgroup* et al., 2013).
The theoretical superiority of the OTSI mode in efficacy and safety is understandable because, according to the design of this mode, it cannot only rapidly reach the initial drug concentration of the multifold MIC but also reduce the fluctuation of peak and trough concentration. This not only rapidly inhibits the target strains but also alleviates the toxic side effects caused by high peak concentrations and the bacterial resistance caused by low trough concentrations. With increasing antibiotic resistance, this mode of vancomycin would be helpful in the clinic, particularly considering delays in the development of new alternatives and a lack of better treatment options. However, due to the lack of clinical data on this infusion mode, these theoretical advantages still lack experimental validation.
For MRSA infections occurring in critically ill patients, Table 2 summarizes some potentially effective vancomycin regimens based on our analysis, and these regimens can better inform us of tentative treatment in the absence of better options for such infections. Of note, despite this significant case of Monte Carlo simulation prediction, these potentially effective regimens based on Monte Carlo simulations cannot be considered certainly effective given the difference in action profiles among antibiotics and in resistance mechanisms among bacteria. Additionally, the modification of vancomycin delivery in severe infections may not be sufficient, by itself, to change the clinical outcome for critically ill patients. Moreover, risk factors associated with a novel vancomycin delivery, such as safety, may be points of concern. However, all of the dosage regimens here were set under a safe PK/PD index and dosing parameters (including dose, infusion rate or time, etc.). Understandably, this novel OTSI mode should be safe.
The main limitation of this study lies in its theoretical nature. This study relied on the PTA to evaluate the efficacy, which has potential limitations as it is only a probability value and therefore lacks sufficient power to detect clinical outcomes. However, Monte Carlo simulation-based feasibility for optimizing exposure to improve antimicrobial effectiveness has been expounded and applied in OPTAMA studies (Kuti and Nicolau, 2005) and PTA-indicated theoretical efficacy has been demonstrated by Eguchi et al. in an in vitro PD model study on meropenem against P. aeruginosa, in which in vitro viable cell counts of P. aeruginosa strain were used as a measure for the in vitro bactericidal activity of meropenem (Eguchi et al., 2010). Thus, we believe that our approach is appropriate since the vancomycin population PK model used here was derived from critically ill patients and PK variability was taken into account; the PD target was adopted from the 2020 vancomycin therapeutic guideline; the MIC values corresponded to those reported in antimicrobial susceptibility testing; and the emulational dosing parameters were close to clinical practice. Therefore, the results on vancomycin dosage could be applied if patient and pathogen populations match those considered here. If this was not the case, the same methodological procedure could be followed, but the actual PK (relationship between CLvan and CLcr, Vd, and body weight due to patient variables) and PD modeling (MIC values) would have to be used. Nevertheless, large clinical trials would be of benefit to determine the competency of CI, II, and OTSI regimens in critically ill patients. Also, therapeutic drug monitoring for vancomycin might be necessary considering its high PK variability in critically ill patients, especially involving the efficacy and safety at a high dose.
Critically ill patients manifest physiology that is unlikely to be encountered in an ambulatory or ward-based environment. Due to the distorted PK profile of vancomycin in these patients, the II and CI modes for vancomycin used in these groups may be unable to achieve an optimal balance in terms of both efficacy and safety. Based on the PK/PD end points, the data presented here show that the OTSI mode for vancomycin allows optimal PK/PD target attainment in terms of both efficacy and safety and it should be therefore focused more on when vancomycin is used for treating MRSA bloodstream infections occurring in these groups. However, large trials are needed to validate these regimens and their clinical implications, especially involving the balance of efficacy and nephrotoxicity at a high dose. Therefore, we agree with the opinion that therapeutic drug monitoring for vancomycin might be necessary considering the high PK variability of vancomycin in critically ill patients.
The original contributions presented in the study are included in the article/supplementary material. Further inquiries can be directed to the corresponding author.
Ethical approval/written informed consent was not required for the study of animals/human participants in accordance with the local legislation and institutional requirements.
XS performed the modeling simulations and wrote the manuscript. HM conceptualized and supervised the manuscript. All authors listed have made a substantial, direct, and intellectual contribution to the work and approved it for publication.
The authors declare that the research was conducted in the absence of any commercial or financial relationships that could be construed as a potential conflict of interest.
All claims expressed in this article are solely those of the authors and do not necessarily represent those of their affiliated organizations, or those of the publisher, the editors and the reviewers. Any product that may be evaluated in this article, or claim that may be made by its manufacturer, is not guaranteed or endorsed by the publisher.
The authors are grateful to the anti-infection experts in our hospital for their direction on vancomycin therapy, and we thank all members of our hospital library for access to their information resources.
Akers, K. S., Cota, J. M., Chung, K. K., Renz, E. M., Mende, K., Murray, C. K. (2012). Serum Vancomycin Levels Resulting From Continuous or Intermittent Infusion in Critically Ill Burn Patients With or Without Continuous Renal Replacement Therapy. J. Burn. Care Res. 33, e254–e262. doi: 10.1097/BCR.0b013e31825042fa
Álvarez, A., Fernández, L., Gutiérrez, D., Iglesias, B., Rodríguez, A., García, P. (2019). Methicillin-Resistant Staphylococcus Aureus in Hospitals: Latest Trends and Treatments Based on Bacteriophages. J. Clin. Microbiol. 57, e01006–e01019. doi: 10.1128/JCM.01006-19
Bissell, B. D., Riggi, G., Morrison, C. (2020). Evaluation of Continuous Infusion Vancomycin Administration in a Critically Ill Trauma Population. J. Intensive Care Med. 35, 570–575. doi: 10.1177/0885066618768749
del Mar Fernández de Gatta Garcia, M., Revilla, N., Calvo, M. V., Domínguez-Gil, A., Sánchez Navarro, A. (2007). Pharmacokinetic/pharmacodynamic Analysis of Vancomycin in ICU Patients. Intensive Care Med. 33, 279–285. doi: 10.1007/s00134-006-0470-5
Deryke, C. A., Alexander, D. P. (2009). Optimizing Vancomycin Dosing Through Pharmacodynamic Assessment Targeting Area Under the Concentration-Time Curve/Minimum Inhibitory Concentration. Hosp. Pharm. 44, 751–765. doi: 10.1310/hpj4409-751
Di Giantomasso, D., May, C. N., Bellomo, R. (2003). Vital Organ Blood Flow During Hyperdynamic Sepsis. Chest 124, 1053–1059. doi: 10.1378/chest.124.3.1053
Eguchi, K., Kanazawa, K., Shimizudani, T., Kanemitsu, K., Kaku, M. (2010). Experimental Verification of the Efficacy of Optimized Two-Step Infusion Therapy With Meropenem Using an In Vitro Pharmacodynamic Model and Monte Carlo Simulation. J. Infect. Chemother. 16, 1–9. doi: 10.1007/s10156-009-0001-8
European Committee on Antimicrobial Susceptibility Testing (EUCAST) Antimicrobial Wild Type Distributions of Microorganisms. Available at: https://mic.eucast.org/search/?search%5Bmethod%5D=mic&search%5Bantibiotic%5D=200&search%5Bspecies%5D=-1&search%5Bdisk_content%5D=-1&search%5Blimit%5D=50 (Accessed June 14, 2021).
Filippone, E., Kraft, W., Farber, J. (2017). The Nephrotoxicity of Vancomycin. Clin. Pharmacol. Ther. 102, 459–469. doi: 10.1002/cpt.726
Flannery, A. H., Bissell, B. D., Bastin, M. T., Morris, P. E., Neyra, J. A. (2020). Continuous Versus Intermittent Infusion of Vancomycin and the Risk of Acute Kidney Injury in Critically Ill Adults: A Systematic Review and Meta-Analysis. Crit. Care Med. 48, 912–918. doi: 10.1097/CCM.0000000000004326
Guo, T., van Hest, R. M., Roggeveen, L. F., Fleuren, L. M., Thoral, P. J., Bosman, R. J., et al. (2019). External Evaluation of Population Pharmacokinetic Models of Vancomycin in Large Cohorts of Intensive Care Unit Patients. Antimicrob. Agents Chemother. 63 (5), e02543–18. doi: 10.1128/AAC.02543-18
Hanrahan, T. P., Harlow, G., Hutchinson, J., Dulhunty, J. M., Lipman, J., Whitehouse, T., et al. (2014). Vancomycin-Associated Nephrotoxicity in the Critically Ill: A Retrospective Multivariate Regression Analysis*. Crit. Care Med. 42, 2527–2536. doi: 10.1097/CCM.0000000000000514
Holubar, M., Meng, L., Alegria, W., Deresinski, S. (2020). Bacteremia Due to Methicillin-Resistant Staphylococcus Aureus: An Update on New Therapeutic Approaches. Infect. Dis. Clin. North Am. 34, 849–861. doi: 10.1016/j.idc.2020.04.003
Hutschala, D., Kinstner, C., Skhirdladze, K., Thalhammer, F., Müller, M., Tschernko, E. (2009). Influence of Vancomycin on Renal Function in Critically Ill Patients After Cardiac Surgery: Continuous Versus Intermittent Infusion. Anesthesiology 111, 356–365. doi: 10.1097/ALN.0b013e3181a97272
Jeurissen, A., Sluyts, I., Rutsaert, R. (2011). A Higher Dose of Vancomycin in Continuous Infusion is Needed in Critically Ill Patients. Int. J. Antimicrob. Agents 37, 75–77. doi: 10.1016/j.ijantimicag.2010.09.004
Kees, M. G., Vögeler, S., Hilpert, J. W. (2011). Initial Dosing of Vancomycin in Critically Ill Patients. Int. J. Antimicrob. Agents 38, 91–92. doi: 10.1016/j.ijantimicag.2011.03.009
Kuti, J. L., Nicolau, D. P. (2005). Making the Most of Surveillance Studies: Summary of the OPTAMA Program. Diagn. Microbiol. Infect. Dis. 53, 281–287. doi: 10.1016/j.diagmicrobio.2005.10.004
Lee, A. S., de Lencastre, H., Garau, J., Kluytmans, J., Malhotra-Kumar, S., Peschel, A., et al. (2018). Methicillin-Resistant Staphylococcus Aureus. Nat. Rev. Dis. Primers 4, 18033. doi: 10.1038/nrdp.2018.33
Lodise, T. P., Lomaestro, B., Graves, J., Drusano, G. L. (2008). Larger Vancomycin Doses (at Least Four Grams Per Day) Are Associated With an Increased Incidence of Nephrotoxicity. Antimicrob. Agents Chemother. 52, 1330–1336. doi: 10.1128/AAC.01602-07
Moine, P., Mueller, S. W., Schoen, J. A., Rothchild, K. B., Fish, D. N. (2016). Pharmacokinetic and Pharmacodynamic Evaluation of a Weight-Based Dosing Regimen of Cefoxitin for Perioperative Surgical Prophylaxis in Obese and Morbidly Obese Patients. Antimicrob. Agents Chemother. 60, 5885–5893. doi: 10.1128/AAC.00585-16
Roberts, J. A., Abdul-Aziz, M. H., Lipman, J., Mouton, J. W., Vinks, A. A., Felton, T. W., et al. (2014). Individualised Antibiotic Dosing for Patients Who Are Critically Ill: Challenges and Potential Solutions. Lancet Infect. Dis. 14, 498–509. doi: 10.1016/S1473-3099(14)70036-2
Roberts, J. A., Taccone, F. S., Udy, A. A., Vincent, J.-L., Jacobs, F., Lipman, J. (2011). Vancomycin Dosing in Critically Ill Patients: Robust Methods for Improved Continuous-Infusion Regimens. Antimicrob. Agents Chemother. 55, 2704–2709. doi: 10.1128/AAC.01708-10
Rybak, M. J., Le, J., Lodise, T. P., Levine, D. P., Bradley, J. S., Liu, C., et al. (2020a). Executive Summary: Therapeutic Monitoring of Vancomycin for Serious Methicillin-Resistant Staphylococcus Aureus Infections: A Revised Consensus Guideline and Review of the American Society of Health-System Pharmacists, the Infectious Diseases Society of America, the Pediatric Infectious Diseases Society, and the Society of Infectious Diseases Pharmacists. Pharmacotherapy 40, 363–367. doi: 10.1002/phar.2376
Rybak, M. J., Le, J., Lodise, T. P., Levine, D. P., Bradley, J. S., Liu, C., et al. (2020b). Therapeutic Monitoring of Vancomycin for Serious Methicillin-Resistant Staphylococcus Aureus Infections: A Revised Consensus Guideline and Review by the American Society of Health-System Pharmacists, the Infectious Diseases Society of America, the Pediatric Infectious Diseases Society, and the Society of Infectious Diseases Pharmacists. Am. J. Health Syst. Pharm. 77, 835–864. doi: 10.1093/ajhp/zxaa036
Rybak, M., Lomaestro, B., Rotschafer, J. C., Moellering, R., Craig, W., Billeter, M., et al. (2009). Therapeutic Monitoring of Vancomycin in Adult Patients: A Consensus Review of the American Society of Health-System Pharmacists, the Infectious Diseases Society of America, and the Society of Infectious Diseases Pharmacists. Am. J. Health-Syst. Pharm. 66, 82–98. doi: 10.2146/ajhp080434
Saugel, B., Nowack, M. C. M., Hapfelmeier, A., Umgelter, A., Schultheiss, C., Thies, P., et al. (2013). Continuous Intravenous Administration of Vancomycin in Medical Intensive Care Unit Patients. J. Crit. Care 28, 9–13. doi: 10.1016/j.jcrc.2012.02.003
Schlobohm, C. J., Zhu, E., Duby, J. J. (2021). Continuous Infusion Versus Intermittent Infusion Vancomycin in a Burn Center Intensive Care Unit. Burns 47, 1495–1501. doi: 10.1016/j.burns.2021.08.016
Schmelzer, T. M., Christmas, A. B., Norton, H. J., Heniford, B. T., Sing, R. F. (2013). Vancomycin Intermittent Dosing Versus Continuous Infusion for Treatment of Ventilator-Associated Pneumonia in Trauma Patients. Am. Surg. 79, 1185–1190. doi: 10.1177/000313481307901123
Setiawan, E., Suwannoi, L., Montakantikul, P., Chindavijak, B. (2019). Optimization of Intermittent Vancomycin Dosage Regimens for Thai Critically Ill Population Infected by MRSA in the Era of the “MIC Creep” Phenomenon. Acta Med. Indon. 51, 10–18.
Song, X., Long, M. (2018). Pharmacodynamic Model for β-Lactam Regimens Used in Surgical Prophylaxis: Model-Based Evaluation of Standard Dosing Regimens. Int. J. Clin. Pharm. 40, 1059–1071. doi: 10.1007/s11096-018-0720-y
Song, X., Zeng, M., Wu, Y., Pan, Y. (2021). Competence Mining of Vancomycin (VAN) in the Management of Infections Due to Bacterial Strains With High VAN Minimum Inhibitory Concentrations (MICs): A Novel Dosing Strategy Based on Pharmacokinetic/Pharmacodynamic Modeling. Front. Microbiol. 12. doi: 10.3389/fmicb.2021.649757
Song, X., Wu, Y. (2022). Predicted Vancomycin Dosage Requirement in Patients With Hematological Malignancies and Dosage Dynamic Adjustment. Front. Pharmacol. 13, 890748. doi: 10.3389/fphar.2022.890748
Tafelski, S., Nachtigall, I., Troeger, U., Deja, M., Krannich, A., Günzel, K., et al. (2015). Observational Clinical Study on the Effects of Different Dosing Regimens on Vancomycin Target Levels in Critically Ill Patients: Continuous Versus Intermittent Application. J. Infect. Public Health 8, 355–363. doi: 10.1016/j.jiph.2015.01.011
The Micron Group Antimicrobial Testing Leadership and Surveillance(ATLAS) Antibacterials Database. Available at: https://atlas-surveillance.com/#/database/mic-distribution (Accessed June 14, 2021).
The Surviving Sepsis Campaign Guidelines Committee including The Pediatric Subgroup*, Dellinger, R. P., Levy, M. M., Rhodes, A., Annane, D., Gerlach, H., et al. (2013). Surviving Sepsis Campaign: International Guidelines for Management of Severe Sepsis and Septic Shoc. Intensive Care Med. 39, 165–228. doi: 10.1007/s00134-012-2769-8
Turner, R. B., Kojiro, K., Won, R., Chang, E., Chan, D., Elbarbry, F. (2018). Prospective Evaluation of Vancomycin Pharmacokinetics in a Heterogeneous Critically Ill Population. Diagn. Microbiol. Infect. Dis. 92, 346–351. doi: 10.1016/j.diagmicrobio.2018.06.022
Udy, A. A., Roberts, J. A., Lipman, J. (2013). Clinical Implications of Antibiotic Pharmacokinetic Principles in the Critically Ill. Intensive Care Med. 39, 2070–2082. doi: 10.1007/s00134-013-3088-4
USP VANCOMYCIN Hydrochloride for Injection, USP. Available at: https://www.sagentpharma.com/wpcproduct/vancomycin-hydrochloride-for-injection-usp/ (Accessed February 20, 2022).
Van Herendael, B., Jeurissen, A., Tulkens, P. M., Vlieghe, E., Verbrugghe, W., Jorens, P. G., et al. (2012). Continuous Infusion of Antibiotics in the Critically Ill: The New Holy Grail for Beta-Lactams and Vancomycin? Ann. Intensive Care 2, 22. doi: 10.1186/2110-5820-2-22
Vuagnat, A., Stern, R., Lotthe, A., Schuhmacher, H., Duong, M., Hoffmeyer, P., et al. (2004). High Dose Vancomycin for Osteomyelitis: Continuous vs. Intermittent Infusion. J. Clin. Pharm. Ther. 29, 351–357. doi: 10.1111/j.1365-2710.2004.00572.x
Wang, G., Hindler, J. F., Ward, K. W., Bruckner, D. A. (2006). Increased Vancomycin MICs for Staphylococcus Aureus Clinical Isolates From a University Hospital During a 5-Year Period. J. Clin. Microbiol. 44, 3883–3886. doi: 10.1128/JCM.01388-06
Wysocki, M., Delatour, F., Faurisson, F., Rauss, A., Pean, Y., Misset, B., et al. (2001). Continuous Versus Intermittent Infusion of Vancomycin in Severe Staphylococcal Infections: Prospective Multicenter Randomized Study. Antimicrob. Agents Chemother. 45, 2460–2467. doi: 10.1128/AAC.45.9.2460-2467.2001
Yamada, C. H., Telles, J. P., Oliveira, D. D. S., Cieslinski, J., Ribeiro, V. S. T., Gasparetto, J., et al. (2020). Comparison of Intermittent Versus Continuous-Infusion Vancomycin for Treating Severe Patients in Intensive Care Units. Braz. J. Infect. Dis. 24, 356–359. doi: 10.1016/j.bjid.2020.07.001
Keywords: vancomycin, methicillin-resistant Staphylococcus aureus, pharmacokinetic/pharmacodynamic, continuous infusion, intermittent infusion, optimal infusion
Citation: Song X and Han M (2022) Pharmacokinetic/Pharmacodynamic Target Attainment of Vancomycin, at Three Reported Infusion Modes, for Methicillin-Resistant Staphylococcus aureus (MRSA) Bloodstream Infections in Critically Ill Patients: Focus on Novel Infusion Mode. Front. Cell. Infect. Microbiol. 12:874401. doi: 10.3389/fcimb.2022.874401
Received: 12 February 2022; Accepted: 20 May 2022;
Published: 07 July 2022.
Edited by:
Bennett Tochukwu Amaechi, The University of Texas Health Science Center at San Antonio, United StatesReviewed by:
Thiruselvam Viswanathan, Florida International University, United StatesCopyright © 2022 Song and Han. This is an open-access article distributed under the terms of the Creative Commons Attribution License (CC BY). The use, distribution or reproduction in other forums is permitted, provided the original author(s) and the copyright owner(s) are credited and that the original publication in this journal is cited, in accordance with accepted academic practice. No use, distribution or reproduction is permitted which does not comply with these terms.
*Correspondence: Xiangqing Song, c3hxbWFzdGVyQDE2My5jb20=
Disclaimer: All claims expressed in this article are solely those of the authors and do not necessarily represent those of their affiliated organizations, or those of the publisher, the editors and the reviewers. Any product that may be evaluated in this article or claim that may be made by its manufacturer is not guaranteed or endorsed by the publisher.
Research integrity at Frontiers
Learn more about the work of our research integrity team to safeguard the quality of each article we publish.