- Research Department, Sidra Medicine, Doha, Qatar
Pregnancy causes physiological and immunological adaptations that allow the mother and fetus to communicate with precision in order to promote a healthy pregnancy. At the same time, these adaptations may make pregnant women more susceptible to infections, resulting in a variety of pregnancy complications; those pathogens may also be vertically transmitted to the fetus, resulting in adverse pregnancy outcomes. Even though the placenta has developed a robust microbial defense to restrict vertical microbial transmission, certain microbial pathogens have evolved mechanisms to avoid the placental barrier and cause congenital diseases. Recent mechanistic studies have begun to uncover the striking role of the maternal microbiota in pregnancy outcomes. In this review, we discuss how microbial pathogens overcome the placental barrier to cause congenital diseases. A better understanding of the placental control of fetal infection should provide new insights into future translational research.
1 Introduction
Pregnancy is a critical “formative period” that has a significant impact on an individual’s health trajectory from fetal life to adulthood (Lash, 2015). Pregnancy is governed by a series of interconnected physiological and cellular mechanisms that promote maternal homeostasis and maintain optimal maternal-fetal interface while boosting fetal growth (Ander et al., 2019). These mechanisms enable the woman’s body to undergo, physiological and immunologic adaptations to host fetal antigens. From the mother’s immune system perspective, the fetus is an allograft that contains foreign antigens from the father (Robinson and Klein, 2012). To protect the fetus from immune rejection, the maternal immune must strike a delicate balance between maintaining tolerance to the fetal allograft by inducing anti-inflammatory properties at the maternal-fetal interface and maintaining an elevated inflammatory response with rising levels of pro-inflammatory cytokines at mucosal surfaces such as the gut to protect against microbial challenges (Koren et al., 2012; Erlebacher, 2013; PrabhuDas et al., 2015; Nuriel-Ohayon et al., 2016; Marchant et al., 2017). Concurrently, the transition of the maternal immune system during pregnancy from more inflammatory states at the start of pregnancy to lower levels of inflammation in mid-pregnancy makes pregnant women more vulnerable to infections (Mor and Cardenas, 2010) and pregnancy complications. Although the exact etiology of pregnancy complications remains elusive, the complex interaction of microbial or other factors with host immune system is thought to be the underlying pathogenesis of pregnancy complications (Megli and Coyne, 2021).
The emerging findings from the various pregnancy cohorts (Piler et al., 2017; Pansieri et al., 2020), as well as many animal studies, demonstrated that pregnancy complications are heterogeneous and depend on a variety of factors, including intra- or extra-uterine infection, microbial dysbiosis, and aberrant immune system (Romero et al., 2014a; MacIntyre et al., 2015; Waken et al., 2017; Fettweis et al., 2019; Serrano et al., 2019; Jehan et al., 2020; Kumar et al., 2021a). During pregnancy, multiple immune signaling pathways and cytokines normally act as mediators to promote a healthy and successful pregnancy and to arbitrate defense against pathogens (Mor and Cardenas, 2010). However, the complexity of interaction between multiple host factors, including maternal infection or aberrant activation of the immune response during pregnancy, could lead to severe pregnancy complications and have a negative impact on pregnancy health or the developing fetus (Kumar et al., 2021a). Indeed, the emerging evidence indicates that these pregnancy complications may pose significant challenges to fetal growth and development during pregnancy, as well as susceptibility to a variety of diseases later in life (Rahman et al., 2012; Nimeri et al., 2013).
In this article, we review the complexity of the interaction between various host factors associated with different maternal infections and dynamic fluctuation of the maternal immune system in both inducing pregnancy complications and eliciting detrimental effects on the developing fetus.
2 Maternal Infections During Pregnancy
Complications from various bacterial, viral, parasitic or fungal maternal infections can occur at any stage of pregnancy. Indeed, several studies suggest that pregnant women are more vulnerable to certain infections as a result of compensatory physiological and immunologic adaptations. The “TORCH” pathogens including Toxoplasma gondii, Other agents (syphilis, varicella-zoster, parvovirus B19), Rubella, Cytomegalovirus (CMV), and Herpes simplex virus, are known to cause various pregnancy complications such as congenital infections, abortion, and intrauterine fetal growth restrictions (Megli and Coyne, 2021). In addition to these most common infections linked to congenital defects, ZIKA infection, one of the newest TORCH pathogens, has recently sparked public concern, resulting in severe pregnancy complications ranging from fetal growth restriction to miscarriages in 2015-2017 (Coyne and Lazear, 2016). Most TORCH pathogens cause mild to moderate morbidity, but infections during pregnancy can have serious fetal consequences due to stimulation of systemic or local factors (Table 1). Emerging studies indicate that various microbial pathogens and neurotropic viruses can cross the placenta barrier, and an aberrant immune response to pathogens can cause various pregnancy complications (Platt et al., 2018), such as:
● Acute maternal infection during pregnancy: may cause maternal morbidity and/or mortality or a wide range of obstetric complications, including low birth weight, stillbirth, miscarriage, and preterm labor.
● Vertical transmission during pregnancy: which can result in congenital infection, intrauterine death, or permanent disability.
● Perinatal transmission during delivery: which can lead to severe neonatal diseases.
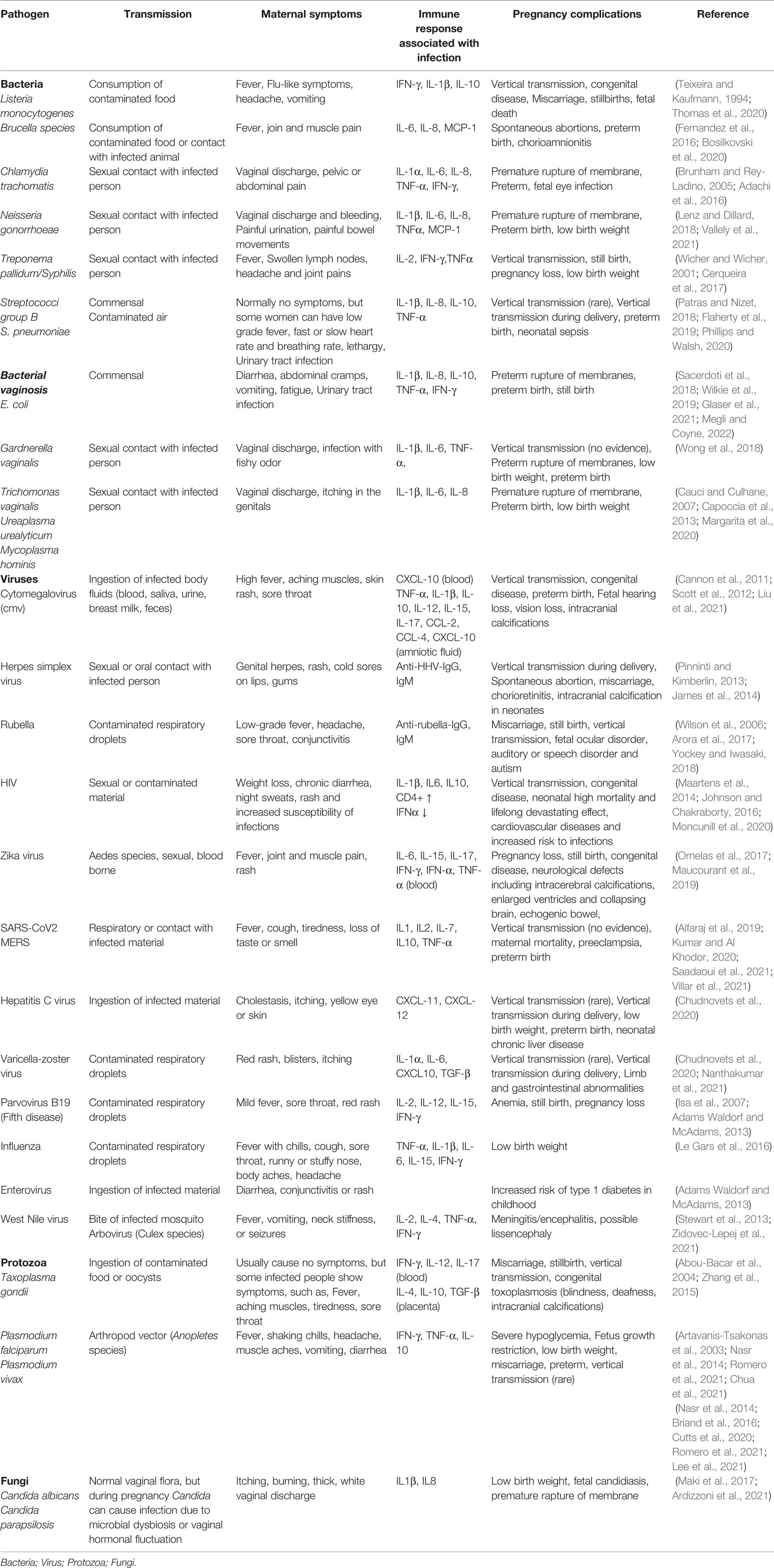
Table 1 Pathogens associated with pregnancy complications and their pathological role in adverse pregnancy outcomes.
To better understand the pathophysiology and consequences of TORCH pathogens and other maternal infections during pregnancy, as well as their impact on pregnancy outcomes, we classified these pathogens into the following categories:
2.1 Bacterial Infections
Acute bacterial infections during pregnancy can increase pregnancy complications and even have a negative pregnancy outcome (Table 1). Bacterial infections, such as listeriosis, bacterial vaginosis, and sexually transmitted infections (STIs), can be caused by a single bacterial pathogen or by a microbial dysbiosis and can result in inflammasome signaling at the maternal-fetal interface and/or severe congenital anomalies in the developing fetus.
2.1.1 Listeriosis
Listeriosis is a foodborne bacterial infection caused by Listeria monocytogenes (Wang et al., 2021). Although this infection is uncommon in healthy people, pregnant women are particularly vulnerable to L. monocytogenes infection, possibly due to their altered immune status (Wang et al., 2021). Once transmitted through contaminated food, L. monocytogenes can cross the intestinal barrier to reach the placenta causing pregnancy complications such as preterm birth, stillbirth, congenital diseases, and sepsis (Mateus et al., 2013). A recent listeriosis outbreak in South Africa reported exceptionally high mortality rates among infected infants (>28%) and pregnant women (Thomas et al., 2020). Although the pathophysiology of L. monocytogenes placental transmission is still largely unknown, emerging studies show that the bacterium binds to E-cadherin on primary trophoblasts via the internalis protein InIA and InIB or InIP (Disson et al., 2008; Faralla et al., 2018), to survive in a hostile environment, suggesting that the bacterium uses trophoblast-specific virulence factors for placental colonization and fetal tissues infection (Bakardjiev et al., 2006). Concurrently, bacterial colonization in placental tissues leads to abscess development, innate immune cells recruitment, and aberrant IFN-γ secretion at the maternal-fetal interface (Charlier et al., 2020; Maudet et al., 2021) and subsequently stimulates inflammasome signaling and increases severity of neonatal outcomes. A.
2.1.2 Bacterial Vaginosis
Bacterial vaginosis (BV) is characterized by the loss of healthy vaginal microbiome composition and an increase in the abundance of pathogenic microbes (Isik et al., 2016). BV is the most common gynecological infection among women during reproductive age and pregnancy (Isik et al., 2016; Kumar et al., 2021a), resulting in serious pregnancy complications such as miscarriage and preterm birth (Table 1) (Leitich et al., 2003). Vaginal infections caused by group B Streptococcus (GBS), Escherichia coli, Bacteroides species, C. trachomatis, and N. gonorrhoeae can ascend to the genital tract and intraamniotic fluid causing chorioamnionitis (Galinsky et al., 2013; Jain et al., 2022). Infections caused by ascending genito-urinary tract pathogens are typically polymicrobial (Mendz et al., 2013) and often associated with microbial biofilm and antimicrobial cervical mucous plug to reach the intra-amniotic fluid or maternal-fetal interface and induce inflammation locally, which then endangers the fetus due to aberrant inflammation at the fetal membrane (Ayala et al., 2019). There is no clear evidence of how dysbiotic flora crosses the maternal barriers to reach the fetus, but GBS and E. coli are the most common pathogens found in the placenta and late-onset sepsis in neonates (Wilkie et al., 2019; Glaser et al., 2021). GBS and E. coli can both adhere to the fetal membrane via various virulence factors and stimulate neutrophils and macrophages to produce inflammatory cytokines and potentially develop extracellular traps to cause premature fetal membrane rupture (Armistead et al., 2020; Coleman et al., 2021; Deshayes de Cambronne et al., 2021).
2.1.3 Sexually Transmitted Infections
Changing the vaginal microenvironment during pregnancy may increase vaginal susceptibility to opportunistic STIs, which are frequently asymptomatic, but can cause severe pregnancy complications if left untreated. Ascending transmission of Chlamydia trachomatis and Neisseria gonorrhoeae can lead to pelvic inflammatory disease and endocarditis, as well as serious pregnancy complications like ectopic pregnancy, preterm birth, and low birth weight (Adachi et al., 2016; Heumann et al., 2017). Syphilis is another common STI (caused by Treponema pallidum). Although the pathophysiology of T. pallidum ascending transmission is unknown, it may be dependent on both the gestational age of the fetus and the maternal stage of infection (Kimball et al., 2020; Primus et al., 2020). Vertical transmission of this bacterium can cause excessive inflammation at the maternal-fetal interface resulting in mild to severe pregnancy complications such as low birth weight, preterm birth, congenital anomalies, and sometimes fetal loss (Primus et al., 2020; Megli and Coyne, 2021).
2.1.4 Maternal Microbiome
The maternal microbiome undergoes significant changes during the course of pregnancy and has been suggested to play an influencing role in the health of pregnant women and their neonates during pregnancy and beyond (Prince et al., 2015; Fettweis et al., 2019). The maternal microbiome consists of distinct microbial communities dominated by different bacterial taxa. For example, a vaginal microbial community dominated with Lactobacillus species are suggested to be associated with a healthy pregnancy, whereas the abundance of a complex vaginal microbial community of CST-IV including Gardnerella, Prevotella, Chlamydia and bacterial vaginosis (BV)-associated bacterium-I (BVAB-I) are associated with increased risk for adverse pregnancy outcomes and fetal infection (Ravel et al., 2011; Kumar et al., 2021a; Saadaoui et al., 2021). The gut and oral microbial communities, like the vaginal microbiome, undergo significant changes during pregnancy, including a significant decrease in alpha diversity and a significant enrichment in Actinobacteria and Proteobacteria species in the gut and oral environment (Figure 1A) (Offenbacher et al., 2006; Aagaard et al., 2012).
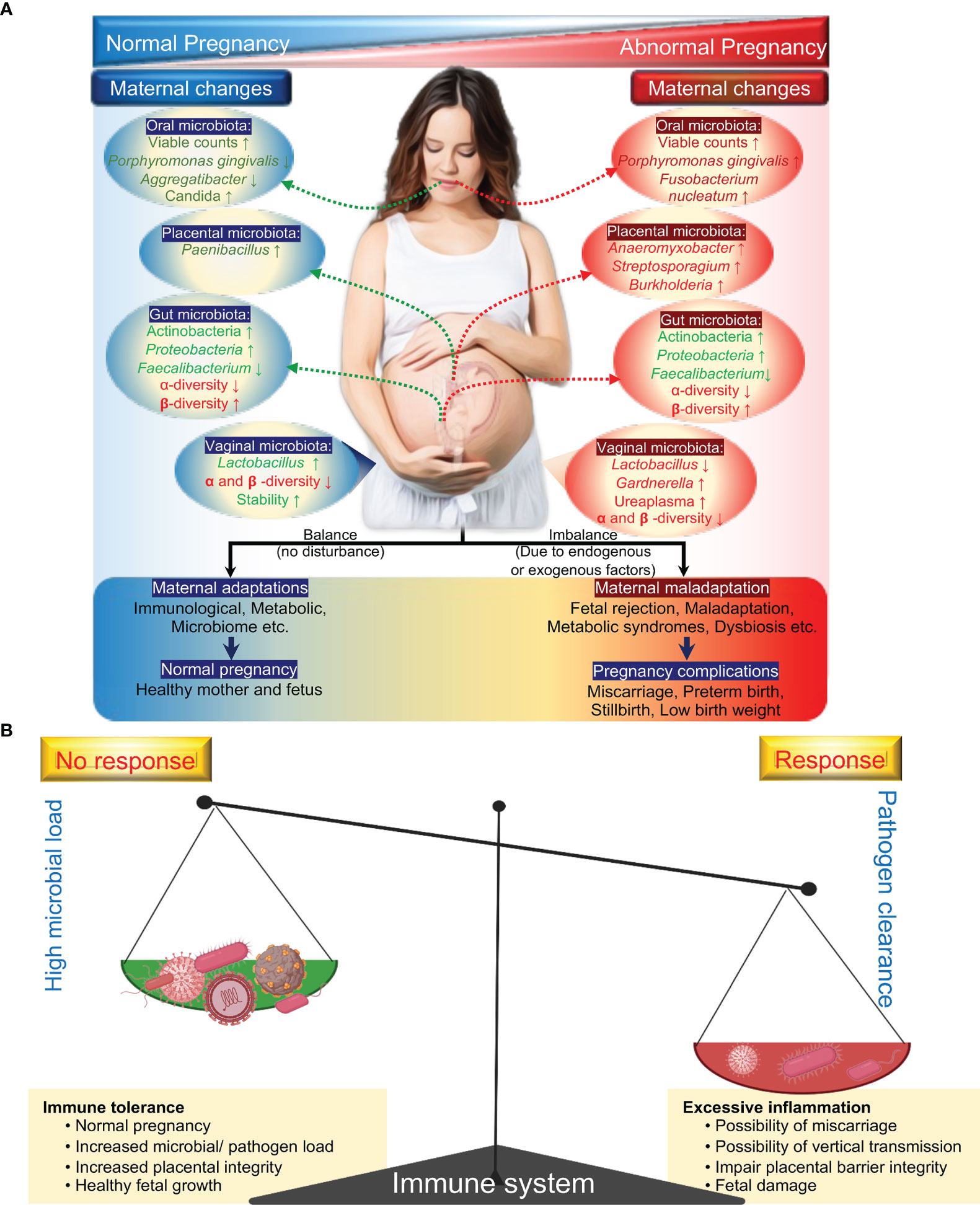
Figure 1 General microbial dynamics during health pregnancy and complicated pregnancy. Known changes in the microbial composition: changes in a specific taxonomy (green) and changes in community diversity (red) (A). Immune response during pregnancy: double-edged sword (B). During pregnancy, the maternal immune system has to balance between sustaining the growth of the fetus and protecting both mother and fetus from pathogens.
To ensure healthy pregnancy outcomes, this delicate balance between microbial communities and immune tolerance or immune response must be maintained (Figure 1B). Numerous studies have suggested that microbial dysbiosis is linked to a variety of pregnancy complications and fetal development (Seong et al., 2008; Han et al., 2010). For example, abnormal changes in the oral microbiota during pregnancy, such as a decrease in Lactobacillus species or an increase in the abundance of Porphyromonas gingivalis, may lead to further infections and the production of pro-inflammatory cytokines, which is thought to be a contributory factor to various pregnancy complications such as early labor, pregnancy loss, and low birth weight, among others (Aagaard et al., 2012; Koren et al., 2012; de Weerth et al., 2013; Romero et al., 2014b; DiGiulio et al., 2015; Goltsman et al., 2018). While the link between microbial dysbiosis and pregnancy complications is clear, the exact nature of these interactions is unknown. It is unclear whether dysbiosis impairs the maternal immune system or influences other mechanisms (Zhang et al., 2015; Kumar et al., 2020) to promote pregnancy complications and fetal development. These findings suggest that intra- or extra-uterine infection or vaginal dysbiosis induces an abnormal immune response in pregnant women and may be an important predictor marker for adverse outcomes of congenital infections.
2.2 Viral Infections
The human microbiome has a significant virome component, which includes a diverse collection of endogenous retroviruses, eukaryotic viruses, and bacteriophages (Wylie et al., 2012), and is increasingly recognized as an orchestrator of bacterial diversity and functionality (Mills et al., 2013; Barr, 2017). Although the majority of viruses are harmless, some pathogenic viruses can cross the maternal-fetal interface and influence placental functions, potentially causing fetal disease (Table 1).
2.2.1 Cytomegalovirus
Cytomegalovirus (CMV) is a DNA virus that belongs to the Herpesviridae family. CMV is the most common viral infection transmitted vertically in utero, causing a wide range of congenital disorders such as hearing and vision loss, intracranial calcifications, microcephaly, organ dysfunction, and intellectual disability (Liu et al., 2021). CMV is typically transmitted from person to person via infected bodily fluids such as blood, saliva, urine, and breast milk (Cannon et al., 2011). Once infected, the virus can live in bone marrow hematopoietic cells for the rest of one’s life (Collins-McMillen et al., 2018). However, it is a primary infection during pregnancy, rather than a reactivation of a persistent infection, that causes adverse pregnancy outcomes (Boppana et al., 2001; Maidji et al., 2006). Although the exact pathophysiology of CMV is unknown, the severity of the infection and fetal consequences are dependent on gestational age at the time of maternal infection, implying that changes in maternal immune status and the maternal-fetal interface play an important role in CMV vertical transmission. According to new research, CMV may first infect placental pericytes before infecting the fetus (Aronoff et al., 2017). Additionally, CMV infected pregnant women have elevated level of cytokines including TNF-α, IL-1β, IL-10, IL-12, IL-15, IL-17, and CXCL10 which may cause various pregnancy complications or serious health problems to the baby, such as preterm birth or low birth weight, or hearing loss at birth or later in life, depending on the pregnancy (Scott et al., 2012).
2.2.2 Herpes Simplex Virus
Herpes simplex virus (HSV) infections are often asymptomatic or cause mild symptoms in adults; however, the changing maternal immune system from higher inflammatory status at the beginning of pregnancy to a lower level of inflammation in mid-pregnancy may predispose the pregnant women to different viral infections, including HSVs (Straface et al., 2012). Although the mechanism of its transplacental transmission is unknown, vertical transmission via direct contact with viral lesions in the genital tract during delivery is a more common route of neonatal infection (James et al., 2014). As a result, maternal HSV infection near the time of delivery increases the risk of vertical transmission, which can result in herpes simplex encephalitis, chorioretinitis, and intracranial calcification in neonates, with a 50-80% mortality rate in untreated cases (Pinninti and Kimberlin, 2013).
2.2.3 Rubella Virus
Rubella virus is a contagious virus in the Togaviridae family. Rubella virus is primarily transmitted via respiratory droplets, and in healthy adults, the infection causes mild illness with a low-grade fever; however, pregnant women who acquire rubella infection are 85 percent more likely to have a miscarriage or stillbirth, and the virus can induce necrosis in the syncytiotrophoblasts allowing it to cross the placental barrier (Lambert et al., 2015; Arora et al., 2017). The neonatal infection can cause severe birth defects with devastating, lifelong consequences such as ocular disorder, auditory problems, cardiovascular defects, speech disorder, and autism (Lambert et al., 2015).
2.2.4 Human Immunodeficiency Virus
Despite the availability of effective anti-HIV therapies, approximately 38 million people are still infected with HIV; among these 53% are women (Data, 2020). HIV can be transmitted through the placenta, perinatally (from direct contact to maternal vaginal fluids or blood during delivery), or postnatally (from breast milk or other sources) (Milligan and Overbaugh, 2014). As a result, congenital HIV transmission remains the leading cause of neonatal infections and the associated neonatal mortality or life-long devastation. Although it is unknown how HIV crosses the placental barrier, neonates born to HIV-infected women are always at a significantly high risk of vertical transmission (25 percent in the absence of antiretroviral therapy) (Bernstein and Wegman, 2018), which predispose them to serious health consequences, including developing acquired immunodeficiency syndrome (AIDS) and cardiovascular diseases (Maartens et al., 2014). Additionally, HIV infection is often associated with opportunistic infections, further increasing the risk of adverse pregnancy outcomes or vertical transmission (Johnson and Chakraborty, 2016).
2.2.5 Zika Virus
Zika virus (ZIKV) is an emerging arbovirus that is endemic in Africa, America, Asia, and Europe (Khaiboullina et al., 2018). ZIKV is primarily transmitted by the bite of an infected mosquito (Khaiboullina et al., 2018). Though ZIKV infection in adults causes mild symptoms with low-grade fever, headache, rash (Javanian et al., 2018), infection during pregnancy can cross the placenta and increase the risk of adverse pregnancy outcomes and postnatal developmental sequelae, such as miscarriage or stillbirth, or surviving infants show lifelong neurological defects such as enlarged ventricles, collapsing brains, and microcephaly. Emerging studies indicate that ZIKV can selectively infect decidual fibroblasts and macrophages, trophoblasts, hofbauer cells (fetal macrophages), and umbilical cord (Quicke et al., 2016; Tabata et al., 2016) and can significantly induce cytokine levels of IL-6, IL-15, IL-17, IFN-α, CXCL10 and IFN-γ at the maternal-fetal interface and in amniotic fluid, which may result in severe fetal neurological abnormalities (Ornelas et al., 2017; Maucourant et al., 2019). Accumulating evidence shows a link between ZIKV infection and congenital microcephaly (Tang et al., 2016; Gladwyn-Ng et al., 2018). ZIKV infection during gestation can trigger endoplasmic reticulum stress in the embryonic brain, which may perturb physiological unfolded protein response in the cerebral cortex and lead to microcephaly in the babies born from mothers infected with ZIKV (Mlakar et al., 2016; Gladwyn-Ng et al., 2018).
2.2.6 COVID-19
The most recent COVID-19 pandemic, caused by the Severe Acute Respiratory Syndrome Coronavirus 2 (SARS-CoV-2), infected over 308 million subjects, and killed 5.5 million people worldwide, highlighting the importance of focusing on women’s health. SARS-CoV2 is primarily spread through close contact with an infected person, as well as through aerosols and respiratory droplets (Saadaoui et al., 2021) and can severely impact a variety of physiological and immunological processes, including pregnancy health and outcomes (Kumar and Al Khodor, 2020; Saadaoui et al., 2021). SARS-CoV-2 binds to host cells through the angiotensin-converting enzyme 2 (ACE2) receptor (Yan et al., 2020), which is expressed on the surface of various trophoblasts including, cytotrophoblast and syncytiotrophoblast cells at the maternal-fetal interface (Gengler et al., 2021). Although the virion genome has been observed in placental and vaginal samples (Dong et al., 2020), but the majority of recent reports show no evidence of vertical transmission (Saadaoui et al., 2021), suggesting that SARS-CoV2 cannot cross the placental barriers even in severely infected women. Despite the magnitude of the pandemic, pregnant women do not appear to vertically transfer the SARS-CoV2 to the fetus, but the inflammatory storm during SARS-CoV2 infection might indirectly induce pregnancy complications and even fetal developmental obstacles. For example, increasing levels of inflammatory cytokines during infection, such as IL-1, IL-2, IL-7, IL-10, and TNF-α in the maternal blood, at the maternal-fetal interface may lead to adverse pregnancy complications, including maternal mortality, preeclampsia, and preterm birth (Villar et al., 2021).
2.3 Parasites
Despite the fact that emerging knowledge and practices on prevention of mosquito-borne diseases have significantly reduced parasitic infections worldwide (Nguyen-Tien et al., 2021), some parasitic infections are still common during pregnancy due to the living conditions (Brummaier et al., 2019) or decreased host immunity. Due to reduced maternal immunity during pregnancy, parasitic infections are common among pregnant women living in low resource settings (Brummaier et al., 2019) and therefore can influence maternal and fetal health (Table 1).
2.3.1 Toxoplasmosis
Toxoplasmosis is caused by Toxoplasma gondii resulting in more than 200,000 cases of congenital toxoplasmosis worldwide each year (Bigna et al., 2020). T. gondii can be vertically transmitted during pregnancy to cause toxoplasmosis and can lead to a high risk of congenital diseases (Bigna et al., 2020). Although, vertical transmission of toxoplasmosis can occur only in 30-40% of patients, but T. gondii infection during pregnancy could lead to an aberrant immune response in blood to control the infection (Sasai and Yamamoto, 2019). Immune response toward the T. gondii infected cells leads to aberrant production of IFN-γ, IL-12, IL-17 which can result in miscarriage and stillbirth (Smith et al., 2021).
2.3.2 Malaria
Malaria parasites, mainly Plasmodium falciparum and Plasmodium vivax, are other pathogens associated with an elevated risk of pregnancy complications, including fetal growth restriction and preterm birth (Briand et al., 2016; Romero et al., 2021). Malaria parasite-infected erythrocytes during pregnancy can adhere to placental receptors and trigger placental inflammation and subsequent damage, causing harm to both mother and her infant (Chua et al., 2021). Emerging evidence suggests that malaria parasite-infected women have significantly higher systemic levels of pro-inflammatory cytokines and chemokines, including TNF-α, IFN-γ, IL-10, which appear to be a key mediators of pregnancy complications (Nasr et al., 2014). IFN-γ response during pregnancy is a double-edged sword. It plays both protective and pathological roles during malaria infection (Nasr et al., 2014). IFN-γ response in malaria parasite-infected women is crucial for parasite clearance in both the liver and blood stages (Inoue et al., 2013), however high levels of IFN-γ may also exacerbate the disease severity, including cerebral malaria and other pregnancy complications such as embryotoxicity or abnormal placenta as shown in Figure 3 (King and Lamb, 2015).
2.4 Fungal Infections
The vast majority of fungi are harmless, and serious fungal infections are uncommon during pregnancy; however, they may occur with higher frequency in pregnant women, which potentially can increase maternal complications, including prematurity or, in some cases, even fetal loss (Rasti et al., 2014).
2.4.1 Candidiasis
Candidiasis is the most common cause of infection worldwide and is caused by Candida, an opportunistic yeast (Manolakaki et al., 2010). Under normal conditions, most Candida species are commensals or endosymbionts, but some species, such as Candida albicans and Candida parapsilosis, can cause candidiasis (AN and Rafiq, 2021). Vaginal candidiasis is the most common gynecological infection during reproductive age and pregnancy. According to emerging studies, up to 40% of women have vaginal colonization with Candida spp. during pregnancy (DiGiulio, 2012), which can easily transmit to the maternal-fetal barrier and progress to intra-amniotic infection which may lead to severe pregnancy complications including low birth weight or fetal candidiasis (Siriratsivawong et al., 2014; Drummond and Lionakis, 2018).
3 Pregnancy Complications Associated With Maternal Infections
Although complications caused by maternal infections or extrinsic abnormalities can occur at any stage of pregnancy, the first trimester is critical for placental development and the formation of a selective barrier between maternal and fetal tissue (Burton et al., 2016). The placental barrier, which is made up of multiple layers of maternal and fetal tissues, serves as a strong barrier against human pathogens reaching the fetus (Burton et al., 2016). Syncytiotrophoblasts (SYNs) are multinucleated cells that form a strong barrier between maternal and fetal blood within the placenta (Ander et al., 2019). Despite the fact that SYNs are highly resistant to bacterial or viral infections and produce type III IFNs (Ander et al., 2019), some pathogens can still cross these barriers and reach the fetus (Figure 2). Although the mechanism(s) by which pathogens breach the strong barriers remains unknown, intrauterine infection and associated inflammation are significant contributors to pregnancy complications. Surprisingly, approximately 25% of preterm births are microbially induced, either through intrauterine infection or maternal extrauterine infection (Agrawal and Hirsch, 2012).
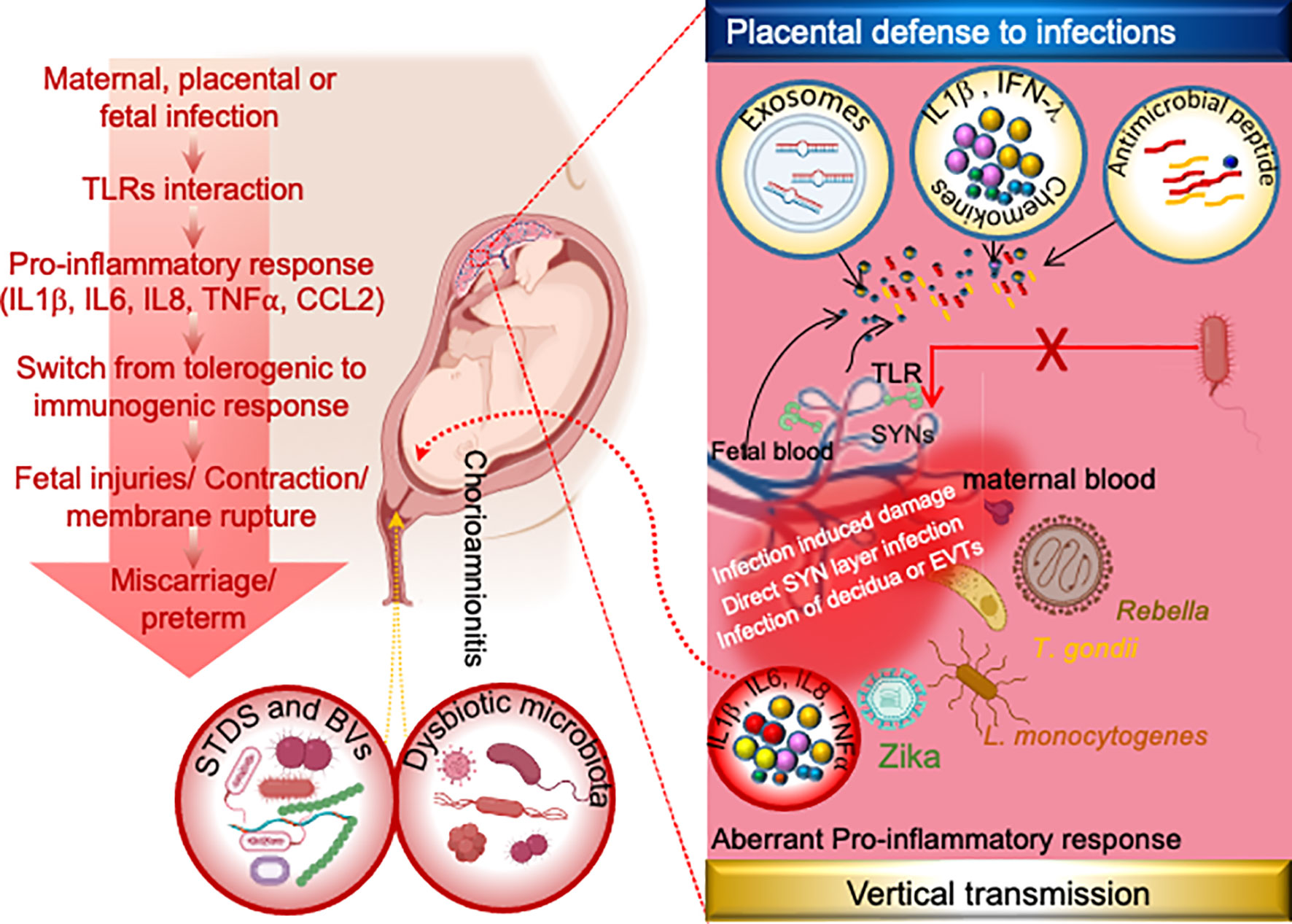
Figure 2 Mechanism of placental physical and immune defense and possible mechanism of vertical transmission of TORCH and other pathogens during pregnancy. The human placenta has evolved several layers of defense including antimicrobial effectors such as exosomes, antimicrobial peptides, and/or innate immune response to infection by release of cytokines and/or highly integrated syncytiotrophoblast. Syncytiotrophoblast is the placental barrier between maternal and fetal blood that allows selective exchanges in nutrients and gases between the embryo and the mother but inhibits the microbial invasion. Although the exact mechanisms by which TORCH pathogens cross the placental barrier are still unclear. However emerging studies indicates these pathogens reach the fetus through infected maternal decidua, infected extracellular trophoblasts (EVTs) and/or through direct infection of the syncytium, while the vaginal pathogens gain access to the amniotic cavity via ascending transmission. Following the amniotic cavity infection, toll-like receptors (TLRs) at the fetal-maternal interface get activated and induce pro-inflammatory cytokines and chemokines, leading to further immune cells recruitment. Switching of maternal immune response from tolerogenic to inflammatory state leads to the premature activation of cervical ripening proteins and onset of labor. Common STDs and BVs infections are C. trachomatis, N. gonorrhoeae, T. pallidum, GBS, E. coli etc. Dysbiotic microbiota infections are GBS, E. coli, C. trachomatis, Gardnerella, Prevotella etc. EVTs: Extravillous trophoblasts, GBS: Group B Streptococcus, SYNs: syncytiotrophoblast.
4 Pregnancy Complication as a Result of Aberrant Immune Response
According to the findings of recent pathological and advanced metagenomic studies, which have been supplemented by cellular and experimental animal studies, a significant amount of pathogens can bypass the placental barrier integrity and modulate an abnormal immune response at the maternal-fetal interface or in the amniotic fluid (Megli and Coyne, 2021). Microbial pathogens commonly associated with periodontal disease or found in the lower genital tract can cross the placental barrier and react to amniotic fluid in women who had preterm labor, possibly via hematogenous dissemination via the transplacental passage or ascending microbial invasion into the amniotic fluid (chorioamnionitis) from the urinary tract (Cobb et al., 2017). Normally, microbial-induced pregnancy complications are mediated by an aberrant inflammatory process. Many studies have revealed an elevated level of proinflammatory cytokines such as IL-1, IL-6, IL-8, and TNF-α in cervicovaginal lavage or amniotic fluid of women experiencing pregnancy complications (Agrawal and Hirsch, 2012; Romero et al., 2014a; Gee et al., 2021). Interestingly, emerging evidence suggests that microbial infection or injection of microbial products such as PAMPs or recombinant inflammatory cytokines in pregnancy mice could lead to adverse pregnancy complications, including preterm birth or even fetal demise (Romero et al., 2014a). Microorganisms or their ligands such as LPS, CpG, Poly (I:C) are recognized by toll-like receptors (TLRs), to induce the production of chemokines (e.g., IL-8, and C-C motif legend 2 (CCL2), cytokines (e.g., IL-1β, and TNF-α), which act on the prostaglandins and proteases to induce the common pathway of parturition (Figure 3, Table 2) (Racicot et al., 2016; Yockey et al., 2018). Indeed, murine models revealed that microbial ligands or recombinant cytokines are likely to elicit miscarriage and preterm labor (Gonzalez et al., 2011), and can be used as a predictive biomarker of the onset of preterm labor (Romero et al., 2014a), emphasizing the role of microbial induced inflammation in pregnancy complications. These studies, when taken together, highlighted the role of microbial-induced inflammation in pregnancy complications and congenital disease.
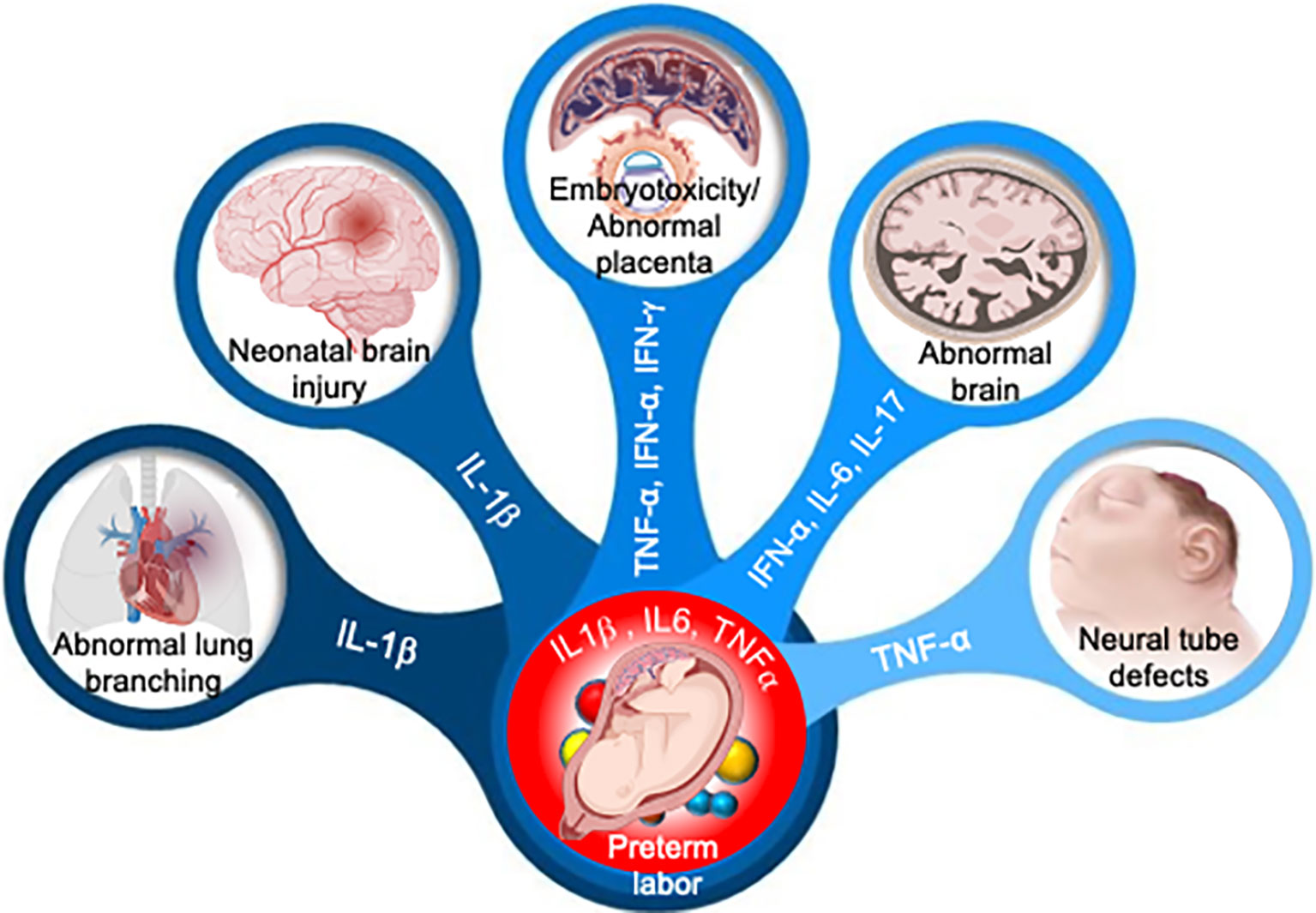
Figure 3 Adverse pregnancy outcomes induced by aberrant cytokine response. Aberrant levels of IL-1β, IL-6, IL-17, TNF-α, IFN-α and IFN-γ in amniotic fluid can induce multiple organ development failure in fetus or induce premature activation of cervical ripening proteins and onset of preterm labor.
5 Future Directions
Although technological advances over the past decade have made significant advances on multiple fronts, including a better understanding of molecular mechanisms, more precise diagnostics, and significantly improved therapeutic outcomes, the increasing incidences of pregnancy-related complications continue to pose daunting challenges in understanding their underlying pathogenesis, host-pathogen interaction at the maternal-fetal interface. As the incidence of maternal infections and associated pregnancy complications rises, a better understanding of the developmental events that result in host-pathogen interaction at the maternal-fetal interface and aberrant immune response is critical for the development of rational intervention strategies. With the help of advanced molecular techniques, the TORCH pathogens and their ability to cross the maternal-fetal barrier to cause congenital fetus disease, which was first proposed decades ago, have now been expanded to include emerging maternal infections and the effects of microbial dysbiosis.
Despite the progress made, there are still many unanswered and widely debated questions. For example, how the placental barrier remains uncompromised to multiple microbial pathogens that cause maternal systemic illness and bacteremia, such as methicillin-resistant Staphylococcus aureus, E. coli, SARS-CoV2 virus, while other pathogens have mastered a variety of evasion mechanisms leading to serious maternal and fetal complications? Another controversial question is the whether the placenta harbors its own microbiome or not? (Aagaard et al., 2014), and how/when does the priming of the fetal immune system with the maternal microbiome occur? (Wampach et al., 2018; de Goffau et al., 2019). The intriguing question now is, what levels of proinflammatory cytokines are required systematically or locally at the maternal-fetal interface to modulate placental integrity and allow vertical transmission of pathogens? Finally, how does maternal dysbiotic microbiota influence the maternal-fetal interface or immune response to cause pregnancy complications? While emerging multi-omics have provided us with comprehensive information about the maternal microbiome (Jehan et al., 2020; Kumar et al., 2021a), their translational impact on women’s health is still far from being achieved and requires more research.
Future research into the mechanism of host-pathogen interaction at the maternal-fetal interface, as well as how these interactions modulate immune responses and placental integrity, will have broader implications in understanding the mechanism of adverse pregnancy complications, such as miscarriage, preterm birth, and vertical transmission of pathogens. Additionally, it may lead to future therapeutic strategies to improve maternal health and prevent vertical transmission of pathogens. Advanced, cutting-edge statistical models, as well as high-throughput molecular multi-omics techniques, can be used to integrate various datasets for assessing their role in biological processes (Kumar et al., 2021b). It should be noted that numerous specific microbial therapies, such as bacteriophage or narrow-spectrum therapies that kill the specific pathogen without affecting other health microbes, are being developed and proving to be effective (Kumar et al., 2018; Brives and Pourraz, 2020). Studies are currently being conducted to determine whether these strategies will be effective for TORCH (Rodriguez-Melcon et al., 2018). Next-generation mRNA vaccines to control different maternal infections are being actively explored (Healy et al., 2019; Kumar and Al Khodor, 2021). These efforts can ultimately facilitate the design of targeted strategies to engineer the vaginal microbiota to lead to antibiotic-sparing strategies to modulate and restore a robust vaginal micro-environment, which may ultimately improve the reproductive health of women and their children.
Author Contributions
MK, MS and SK Conceptualization, MK and SA. Writing—original draft preparation, MK and SA. Writing—review and editing, MK, MS and SA. All authors have read and agreed to the published version of the manuscript.
Funding
This research was funded by Sidra Medicine, Qatar, grant number SDR400161, and the APC was funded by Research Department, Sidra Medicine, Qatar.
Conflict of Interest
The authors declare that the research was conducted in the absence of any commercial or financial relationships that could be construed as a potential conflict of interest.
Publisher’s Note
All claims expressed in this article are solely those of the authors and do not necessarily represent those of their affiliated organizations, or those of the publisher, the editors and the reviewers. Any product that may be evaluated in this article, or claim that may be made by its manufacturer, is not guaranteed or endorsed by the publisher.
References
Aagaard, K., Ma, J., Antony, K. M., Ganu, R., Petrosino, J., Versalovic, J. (2014). The Placenta Harbors a Unique Microbiome. Sci. Transl. Med. 6 (237), 237ra65. doi: 10.1126/scitranslmed.3008599
Aagaard, K., Riehle, K., Ma, J., Segata, N., Mistretta, T. A., Coarfa, C., et al. (2012). A Metagenomic Approach to Characterization of the Vaginal Microbiome Signature in Pregnancy. PloS One 7 (6), e36466. doi: 10.1371/journal.pone.0036466
Abou-Bacar, A., Pfaff, A. W., Letscher-Bru, V., Filisetti, D., Rajapakse, R., Antoni, E., et al. (2004). Role of Gamma Interferon and T Cells in Congenital Toxoplasma Transmission. Parasit. Immunol. 26 (8-9), 315–318. doi: 10.1111/j.0141-9838.2004.00713.x
Adachi, K., Nielsen-Saines, K., Klausner, J. D. (2016). Chlamydia Trachomatis Infection in Pregnancy: The Global Challenge of Preventing Adverse Pregnancy and Infant Outcomes in Sub-Saharan Africa and Asia. BioMed. Res. Int. 2016, 9315757. doi: 10.1155/2016/9315757
Adams Waldorf, K. M., McAdams, R. M. (2013). Influence of Infection During Pregnancy on Fetal Development. Reproduction 146 (5), R151–R162. doi: 10.1530/REP-13-0232
Agrawal, V., Hirsch, E. (2012). Intrauterine Infection and Preterm Labor. Semin. Fetal Neonatal. Med. 17 (1), 12–19. doi: 10.1016/j.siny.2011.09.001
Alfaraj, S. H., Al-Tawfiq, J. A., Memish, Z. A. (2019). Middle East Respiratory Syndrome Coronavirus (MERS-CoV) Infection During Pregnancy: Report of Two Cases & Review of the Literature. J. Microbiol. Immunol. Infect. 52 (3), 501–503. doi: 10.1016/j.jmii.2018.04.005
Ander, S. E., Diamond, M. S., Coyne, C. B. (2019). Immune Responses at the Maternal-Fetal Interface. Sci. Immunol. 4 (31): eaat6114. doi: 10.1126/sciimmunol.aat6114
Ardizzoni, A., Wheeler, R. T., Pericolini, E. (2021). It Takes Two to Tango: How a Dysregulation of the Innate Immunity, Coupled With Candida Virulence, Triggers VVC Onset. Front. Microbiol. 12 (1449). doi: 10.3389/fmicb.2021.692491
Armistead, B., Herrero-Foncubierta, P., Coleman, M., Quach, P., Whidbey, C., Justicia, J., et al. (2020). Lipid Analogs Reveal Features Critical for Hemolysis and Diminish Granadaene Mediated Group B Streptococcus Infection. Nat. Commun. 11 (1), 1502. doi: 10.1038/s41467-020-15282-0
Aronoff, D. M., Correa, H., Rogers, L. M., Arav-Boger, R., Alcendor, D. J. (2017). Placental Pericytes and Cytomegalovirus Infectivity: Implications for HCMV Placental Pathology and Congenital Disease. Am. J. Reprod. Immunol. 78 (3)10.1111/aji.12728. doi: 10.1111/aji.12728
Arora, N., Sadovsky, Y., Dermody, T. S., Coyne, C. B. (2017). Microbial Vertical Transmission During Human Pregnancy. Cell Host Microbe 21 (5), 561–567. doi: 10.1016/j.chom.2017.04.007
Artavanis-Tsakonas, K., Tongren, J. E., Riley, E. M. (2003). The War Between the Malaria Parasite and the Immune System: Immunity, Immunoregulation and Immunopathology. Clin. Exp. Immunol. 133 (2), 145–152. doi: 10.1046/j.1365-2249.2003.02174.x
Ashkar, A. A., Black, G. P., Wei, Q., He, H., Liang, L., Head, J. R., et al. (2003). Assessment of Requirements for IL-15 and IFN Regulatory Factors in Uterine NK Cell Differentiation and Function During Pregnancy. J. Immunol. 171 (6), 2937–2944. doi: 10.4049/jimmunol.171.6.2937
Ayala, O. D., Doster, R. S., Manning, S. D., O'Brien, C. M., Aronoff, D. M., Gaddy, J. A., et al. (2019). Raman Microspectroscopy Differentiates Perinatal Pathogens on Ex Vivo Infected Human Fetal Membrane Tissues. J. Biophotonics. 12 (9), e201800449. doi: 10.1002/jbio.201800449
Bakardjiev, A. I., Theriot, J. A., Portnoy, D. A. (2006). Listeria Monocytogenes Traffics From Maternal Organs to the Placenta and Back. PloS Pathog. 2 (6), e66. doi: 10.1371/journal.ppat.0020066
Barr, J. J. (2017). A Bacteriophages Journey Through the Human Body. Immunol. Rev. 279 (1), 106–122. doi: 10.1111/imr.12565
Baud, V., Karin, M. (2001). Signal Transduction by Tumor Necrosis Factor and its Relatives. Trends Cell Biol. 11 (9), 372–377. doi: 10.1016/S0962-8924(01)02064-5
Bernstein, H. B., Wegman, A. D. (2018). HIV Infection: Antepartum Treatment and Management. Clin. Obstet. Gynecol. 61 (1), 122–136. doi: 10.1097/GRF.0000000000000330
Bigna, J. J., Tochie, J. N., Tounouga, D. N., Bekolo, A. O., Ymele, N. S., Youda, E. L., et al. (2020). Global, Regional, and Country Seroprevalence of Toxoplasma Gondii in Pregnant Women: A Systematic Review, Modelling and Meta-Analysis. Sci. Rep. 10 (1), 12102. doi: 10.1038/s41598-020-69078-9
Boppana, S. B., Rivera, L. B., Fowler, K. B., Mach, M., Britt, W. J. (2001). Intrauterine Transmission of Cytomegalovirus to Infants of Women With Preconceptional Immunity. N. Engl. J. Med. 344 (18), 1366–1371. doi: 10.1056/NEJM200105033441804
Bosilkovski, M., Arapovic, J., Keramat, F. (2020). Human Brucellosis in Pregnancy - An Overview. Bosn. J. Basic Med. Sci. 20 (4), 415–422. doi: 10.17305/bjbms.2019.4499
Briand, V., Saal, J., Ghafari, C., Huynh, B.-T., Fievet, N., Schmiegelow, C., et al. (2016). Fetal Growth Restriction Is Associated With Malaria in Pregnancy: A Prospective Longitudinal Study in Benin. J. Infect. Dis. 214 (3), 417–425. doi: 10.1093/infdis/jiw158
Brives, C., Pourraz, J. (2020). Phage Therapy as a Potential Solution in the Fight Against AMR: Obstacles and Possible Futures. Palgrave Commun. 6 (1), 100. doi: 10.1057/s41599-020-0478-4
Brummaier, T., Syed Ahamed Kabeer, B., Lindow, S., Konje, J. C., Pukrittayaamee, S., Utzinger, J., et al. (2019). A Prospective Cohort for the Investigation of Alteration in Temporal Transcriptional and Microbiome Trajectories Preceding Preterm Birth: A Study Protocol. BMJ Open 9 (1), e023417. doi: 10.1136/bmjopen-2018-023417
Brunham, R. C., Rey-Ladino, J. (2005). Immunology of Chlamydia Infection: Implications for a Chlamydia Trachomatis Vaccine. Nat. Rev. Immunol. 5 (2), 149–161. doi: 10.1038/nri1551
Burton, G. J., Fowden, A. L., Thornburg, K. L. (2016). Placental Origins of Chronic Disease. Physiol. Rev. 96 (4), 1509–1565. doi: 10.1152/physrev.00029.2015
Cannon, M. J., Hyde, T. B., Schmid, D. S. (2011). Review of Cytomegalovirus Shedding in Bodily Fluids and Relevance to Congenital Cytomegalovirus Infection. Rev. Med. Virol. 21 (4), 240–255. doi: 10.1002/rmv.695
Capoccia, R., Greub, G., Baud, D. (2013). Ureaplasma Urealyticum, Mycoplasma Hominis and Adverse Pregnancy Outcomes. Curr. Opin. Infect. Dis. 26 (3), 231–240. doi: 10.1097/QCO.0b013e328360db58
Cauci, S., Culhane, J. F. (2007). Modulation of Vaginal Immune Response Among Pregnant Women With Bacterial Vaginosis by Trichomonas Vaginalis, Chlamydia Trachomatis, Neisseria Gonorrhoeae, and Yeast. Am. J. Obstet. Gynecol. 196 (2), 133.e1–133.e7. doi: 10.1016/j.ajog.2006.08.033
Cerqueira, L. R. P., Monteiro, D. L. M., Taquette, S. R., Rodrigues, N. C. P., Trajano, A. J. B., Souza, F. M., et al. (2017). The Magnitude of Syphilis: From Prevalence to Vertical Transmission. Rev. Inst. Med. Trop. Sao Paulo 59, e78. doi: 10.1590/s1678-9946201759078
Charlier, C., Disson, O., Lecuit, M. (2020). Maternal-Neonatal Listeriosis. Virulence 11 (1), 391–397. doi: 10.1080/21505594.2020.1759287
Chavan, A. R., Bhullar, B. A., Wagner, G. P. (2016). What was the Ancestral Function of Decidual Stromal Cells? A Model for the Evolution of Eutherian Pregnancy. Placenta 40, 40–51. doi: 10.1016/j.placenta.2016.02.012
Chesler, D. A., Reiss, C. S. (2002). The Role of IFN-Gamma in Immune Responses to Viral Infections of the Central Nervous System. Cytokine Growth Factor Rev. 13 (6), 441–454. doi: 10.1016/S1359-6101(02)00044-8
Choi, G. B., Yim, Y. S., Wong, H., Kim, S., Kim, H., Kim, S. V., et al. (2016). The Maternal Interleukin-17a Pathway in Mice Promotes Autism-Like Phenotypes in Offspring. Science 351 (6276), 933–939. doi: 10.1126/science.aad0314
Chua, C. L. L., Khoo, S. K. M., Ong, J. L. E., Ramireddi, G. K., Yeo, T. W., Teo, A. (2021). Malaria in Pregnancy: From Placental Infection to Its Abnormal Development and Damage. Front. Microbiol. 12, 777343. doi: 10.3389/fmicb.2021.777343
Chudnovets, A., Liu, J., Narasimhan, H., Liu, Y., Burd, I. (2020). Role of Inflammation in Virus Pathogenesis During Pregnancy. J. Virol. 95 (2), e01381–19. doi: 10.1128/JVI.01381-19
Cobb, C. M., Kelly, P. J., Williams, K. B., Babbar, S., Angolkar, M., Derman, R. J. (2017). The Oral Microbiome and Adverse Pregnancy Outcomes. Int. J. Womens Health 9, 551–559. doi: 10.2147/IJWH.S142730
Coleman, M., Armistead, B., Orvis, A., Quach, P., Brokaw, A., Gendrin, C., et al. (2021). Hyaluronidase Impairs Neutrophil Function and Promotes Group B Streptococcus Invasion and Preterm Labor in Nonhuman Primates. mBio 12 (1): e03115–20. doi: 10.1128/mBio.03115-20
Collins-McMillen, D., Peppenelli, M., Goodrum, F. (2018). Molecular Determinants and the Regulation of Human Cytomegalovirus Latency and Reactivation. Viruses 10 (8), 444. doi: 10.3390/v10080444
Coyne, C. B., Lazear, H. M. (2016). Zika Virus - Reigniting the TORCH. Nat. Rev. Microbiol. 14 (11), 707–715. doi: 10.1038/nrmicro.2016.125
Cutts, J. C., Agius, P. A., Zaw, L., Powell, R., Moore, K., Draper, B., et al. (2020). Pregnancy-Specific Malarial Immunity and Risk of Malaria in Pregnancy and Adverse Birth Outcomes: A Systematic Review. BMC Med. 18 (1), 14. doi: 10.1186/s12916-019-1467-6
Data, U. (2020). UNAIDS. Available at: https://www.unaids.org/en/resources/fact-sheet
de Goffau, M. C., Lager, S., Sovio, U., Gaccioli, F., Cook, E., Peacock, S. J., et al. (2019). Human Placenta has No Microbiome But can Contain Potential Pathogens. Nature 572 (7769), 329–334. doi: 10.1038/s41586-019-1451-5
Deshayes de Cambronne, R., Fouet, A., Picart, A., Bourrel, A. S., Anjou, C., Bouvier, G., et al. (2021). CC17 Group B Streptococcus Exploits Integrins for Neonatal Meningitis Development. J. Clin. Invest 131 (5), e136737. doi: 10.1172/JCI136737
de Weerth, C., Fuentes, S., de Vos, W. M. (2013). Crying in Infants: On the Possible Role of Intestinal Microbiota in the Development of Colic. Gut Microbes 4 (5), 416–421. doi: 10.4161/gmic.26041
DiGiulio, D. B. (2012). Diversity of Microbes in Amniotic Fluid. Semin. Fetal Neonatal. Med. 17 (1), 2–11. doi: 10.1016/j.siny.2011.10.001
DiGiulio, D. B., Callahan, B. J., McMurdie, P. J., Costello, E. K., Lyell, D. J., Robaczewska, A., et al. (2015). Temporal and Spatial Variation of the Human Microbiota During Pregnancy. Proc. Natl. Acad. Sci. U. S. A. 112 (35), 11060–11065. doi: 10.1073/pnas.1502875112
Disson, O., Grayo, S., Huillet, E., Nikitas, G., Langa-Vives, F., Dussurget, O., et al. (2008). Conjugated Action of Two Species-Specific Invasion Proteins for Fetoplacental Listeriosis. Nature 455 (7216), 1114–1118. doi: 10.1038/nature07303
Dong, L., Tian, J., He, S., Zhu, C., Wang, J., Liu, C., et al. (2020). Possible Vertical Transmission of SARS-CoV-2 From an Infected Mother to Her Newborn. JAMA 323 (18), 1846–1848. doi: 10.1001/jama.2020.4621
Drummond, R. A., Lionakis, M. S. (2018). Candidiasis of the Central Nervous System in Neonates and Children With Primary Immunodeficiencies. Curr. Fungal Infect. Rep. 12 (2), 92–97. doi: 10.1007/s12281-018-0316-y
Erlebacher, A. (2013). Immunology of the Maternal-Fetal Interface. Annu. Rev. Immunol. 31, 387–411. doi: 10.1146/annurev-immunol-032712-100003
Faralla, C., Bastounis, E. E., Ortega, F. E., Light, S. H., Rizzuto, G., Gao, L., et al. (2018). Listeria Monocytogenes InlP Interacts With Afadin and Facilitates Basement Membrane Crossing. PloS Pathog. 14 (5), e1007094. doi: 10.1371/journal.ppat.1007094
Fernandez, A. G., Ferrero, M. C., Hielpos, M. S., Fossati, C. A., Baldi, P. C. (2016). Proinflammatory Response of Human Trophoblastic Cells to Brucella Abortus Infection and Upon Interactions With Infected Phagocytes. Biol. Reprod. 94 (2), 48. doi: 10.1095/biolreprod.115.131706
Fettweis, J. M., Serrano, M. G., Brooks, J. P., Edwards, D. J., Girerd, P. H., Parikh, H. I., et al. (2019). The Vaginal Microbiome and Preterm Birth. Nat. Med. 25 (6), 1012–1021. doi: 10.1038/s41591-019-0450-2
Flaherty, R. A., Borges, E. C., Sutton, J. A., Aronoff, D. M., Gaddy, J. A., Petroff, M. G., et al. (2019). Genetically Distinct Group B Streptococcus Strains Induce Varying Macrophage Cytokine Responses. PloS One 14 (9), e0222910. doi: 10.1371/journal.pone.0222910
Galinsky, R., Polglase, G. R., Hooper, S. B., Black, M. J., Moss, T. J. (2013). The Consequences of Chorioamnionitis: Preterm Birth and Effects on Development. J. Pregnancy 2013, 412831. doi: 10.1155/2013/412831
Gee, S., Chandiramani, M., Seow, J., Pollock, E., Modestini, C., Das, A., et al. (2021). The Legacy of Maternal SARS-CoV-2 Infection on the Immunology of the Neonate. Nat. Immunol. 22 (12), 1490–1502. doi: 10.1038/s41590-021-01049-2
Gengler, C., Dubruc, E., Favre, G., Greub, G., de Leval, L., Baud, D. (2021). SARS-CoV-2 ACE-Receptor Detection in the Placenta Throughout Pregnancy. Clin. Microbiol. Infect. 27 (3), 489–490. doi: 10.1016/j.cmi.2020.09.049
Gladwyn-Ng, I., Cordon-Barris, L., Alfano, C., Creppe, C., Couderc, T., Morelli, G., et al. (2018). Stress-Induced Unfolded Protein Response Contributes to Zika Virus-Associated Microcephaly. Nat. Neurosci. 21 (1), 63–71. doi: 10.1038/s41593-017-0038-4
Glaser, M. A., Hughes, L. M., Jnah, A., Newberry, D. (2021). Neonatal Sepsis: A Review of Pathophysiology and Current Management Strategies. Adv. Neonatal. Care 21 (1), 49–60. doi: 10.1097/ANC.0000000000000769
Goltsman, D. S. A., Sun, C. L., Proctor, D. M., DiGiulio, D. B., Robaczewska, A., Thomas, B. C., et al. (2018). Metagenomic Analysis With Strain-Level Resolution Reveals Fine-Scale Variation in the Human Pregnancy Microbiome. Genome Res. 28 (10), 1467–1480. doi: 10.1101/gr.236000.118
Gonzalez, J. M., Franzke, C. W., Yang, F., Romero, R., Girardi, G. (2011). Complement Activation Triggers Metalloproteinases Release Inducing Cervical Remodeling and Preterm Birth in Mice. Am. J. Pathol. 179 (2), 838–849. doi: 10.1016/j.ajpath.2011.04.024
Hamai, Y., Fujii, T., Yamashita, T., Nishina, H., Kozuma, S., Mikami, Y., et al. (1997). Evidence for an Elevation in Serum Interleukin-2 and Tumor Necrosis Factor-Alpha Levels Before the Clinical Manifestations of Preeclampsia. Am. J. Reprod. Immunol. 38 (2), 89–93. doi: 10.1111/j.1600-0897.1997.tb00281.x
Han, Y. W., Fardini, Y., Chen, C., Iacampo, K. G., Peraino, V. A., Shamonki, J. M., et al. (2010). Term Stillbirth Caused by Oral Fusobacterium Nucleatum. Obstet. Gynecol. 115 (2 Pt 2), 442–445. doi: 10.1097/AOG.0b013e3181cb9955
Healy, S. A., Fried, M., Richie, T., Bok, K., Little, M., August, A., et al. (2019). Malaria Vaccine Trials in Pregnant Women: An Imperative Without Precedent. Vaccine 37 (6), 763–770. doi: 10.1016/j.vaccine.2018.12.025
Heumann, C. L., Quilter, L. A., Eastment, M. C., Heffron, R., Hawes, S. E. (2017). Adverse Birth Outcomes and Maternal Neisseria Gonorrhoeae Infection: A Population-Based Cohort Study in Washington State. Sex Transm. Dis. 44 (5), 266–271. doi: 10.1097/OLQ.0000000000000592
Hogmalm, A., Bry, M., Strandvik, B., Bry, K. (2014). IL-1beta Expression in the Distal Lung Epithelium Disrupts Lung Morphogenesis and Epithelial Cell Differentiation in Fetal Mice. Am. J. Physiol. Lung Cell Mol. Physiol. 306 (1), L23–L34. doi: 10.1152/ajplung.00154.2013
Inoue, S., Niikura, M., Mineo, S., Kobayashi, F. (2013). Roles of IFN-Gamma and Gammadelta T Cells in Protective Immunity Against Blood-Stage Malaria. Front. Immunol. 4, 258. doi: 10.3389/fimmu.2013.00258
Isa, A., Lundqvist, A., Lindblom, A., Tolfvenstam, T., Broliden, K. (2007). Cytokine Responses in Acute and Persistent Human Parvovirus B19 Infection. Clin. Exp. Immunol. 147 (3), 419–425. doi: 10.1111/j.1365-2249.2006.03286.x
Isik, G., Demirezen, S., Donmez, H. G., Beksac, M. S. (2016). Bacterial Vaginosis in Association With Spontaneous Abortion and Recurrent Pregnancy Losses. J. Cytol. 33 (3), 135–140. doi: 10.4103/0970-9371.188050
Jain, V. G., Willis, K. A., Jobe, A., Ambalavanan, N. (2022). Chorioamnionitis and Neonatal Outcomes. Pediatr. Res. 91 (2), 289–296. doi: 10.1038/s41390-021-01633-0
James, S. H., Sheffield, J. S., Kimberlin, D. W. (2014). Mother-To-Child Transmission of Herpes Simplex Virus. J. Pediatr. Infect. Dis. Soc 3 Suppl 1, S19–S23. doi: 10.1093/jpids/piu050
Javanian, M., Masrour-Roudsari, J., Ebrahimpour, S. (2018). Clinical Diagnosis Challenges in Zika Virus Infection. Caspian. J. Intern. Med. 9 (4), 416–417. doi: 10.22088/cjim.9.4.416
Jehan, F., Sazawal, S., Baqui, A. H., Nisar, M. I., Dhingra, U., Khanam, R., et al. (2020). Multiomics Characterization of Preterm Birth in Low- and Middle-Income Countries. JAMA Netw. Open 3 (12), e2029655. doi: 10.1001/jamanetworkopen.2020.29655
Johnson, E. L., Chakraborty, R. (2016). HIV-1 at the Placenta: Immune Correlates of Protection and Infection. Curr. Opin. Infect. Dis. 29 (3), 248–255. doi: 10.1097/QCO.0000000000000267
Khaiboullina, S., Uppal, T., Martynova, E., Rizvanov, A., Baranwal, M., Verma, S. C. (2018). History of ZIKV Infections in India and Management of Disease Outbreaks. Front. Microbiol. 9, 2126. doi: 10.3389/fmicb.2018.02126
Kimball, A., Torrone, E., Miele, K., Bachmann, L., Thorpe, P., Weinstock, H., et al. (2020). Missed Opportunities for Prevention of Congenital Syphilis - United States, 2018. MMWR Morb. Mortal Wkly. Rep. 69 (22), 661–665. doi: 10.15585/mmwr.mm6922a1
King, T., Lamb, T. (2015). Interferon-Gamma: The Jekyll and Hyde of Malaria. PloS Pathog. 11 (10), e1005118. doi: 10.1371/journal.ppat.1005118
Koren, O., Goodrich, J. K., Cullender, T. C., Spor, A., Laitinen, K., Backhed, H. K., et al. (2012). Host Remodeling of the Gut Microbiome and Metabolic Changes During Pregnancy. Cell 150 (3), 470–480. doi: 10.1016/j.cell.2012.07.008
Kumar, M., Al Khodor, S. (2020). Pathophysiology and Treatment Strategies for COVID-19. J. Transl. Med. 18 (1), 353. doi: 10.1186/s12967-020-02520-8
Kumar, M., Al Khodor, S. (2021). “Armed for the Future Coronavirus Pandemic”: A Promising Use of the Multimeric SARS-CoV-2 Receptor Binding Domain Nanoparticle as a New Pan-Coronavirus Vaccine. Signal Transduct Target Ther. 6 (1), 305. doi: 10.1038/s41392-021-00721-1
Kumar, M., Mathur, T., Joshi, V., Upadhyay, D. J., Inoue, S. I., Masuda, N. (2018). Effect of DS-2969b, a Novel GyrB Inhibitor, on Rat and Monkey Intestinal Microbiota. Anaerobe 51, 120–123. doi: 10.1016/j.anaerobe.2018.04.017
Kumar, M., Murugesan, S., Singh, P., Saadaoui, M., Elhag, D. A., Terranegra, A., et al. (2021a). Vaginal Microbiota and Cytokine Levels Predict Preterm Delivery in Asian Women. Front. Cell Infect. Microbiol. 11, 639665. doi: 10.3389/fcimb.2021.639665
Kumar, M., Saadaoui, M., Elhag, D. A., Murugesan, S., Al Abduljabbar, S., Fagier, Y., et al. (2021b). Omouma: A Prospective Mother and Child Cohort Aiming to Identify Early Biomarkers of Pregnancy Complications in Women Living in Qatar. BMC Pregnancy Childbirth 21 (1), 570. doi: 10.1186/s12884-021-04029-4
Kumar, M., Singh, P., Murugesan, S., Vetizou, M., McCulloch, J., Badger, J. H., et al. (2020). Microbiome as an Immunological Modifier. Methods Mol. Biol. 2055, 595–638. doi: 10.1007/978-1-4939-9773-2_27
Lambert, N., Strebel, P., Orenstein, W., Icenogle, J., Poland, G. A. (2015). Rubella. Lancet 385 (9984), 2297–2307. doi: 10.1016/S0140-6736(14)60539-0
Lash, G. E. (2015). Molecular Cross-Talk at the Feto-Maternal Interface. Cold Spring Harb. Perspect. Med. 5 (12), a023010. doi: 10.1101/cshperspect.a023010
Lee, S., Choi, Y.-K., Goo, Y.-K. (2021). Humoral and Cellular Immune Response to Plasmodium Vivax VIR Recombinant and Synthetic Antigens in Individuals Naturally Exposed to P. Vivax in the Republic of Korea. Malaria J. 20 (1), 288. doi: 10.1186/s12936-021-03810-2
Le Gars, M., Kay, A. W., Bayless, N. L., Aziz, N., Dekker, C. L., Swan, G. E., et al. (2016). Increased Proinflammatory Responses of Monocytes and Plasmacytoid Dendritic Cells to Influenza A Virus Infection During Pregnancy. J. Infect. Dis. 214 (11), 1666–1671. doi: 10.1093/infdis/jiw448
Leitich, H., Bodner-Adler, B., Brunbauer, M., Kaider, A., Egarter, C., Husslein, P. (2003). Bacterial Vaginosis as a Risk Factor for Preterm Delivery: A Meta-Analysis. Am. J. Obstet. Gynecol. 189 (1), 139–147. doi: 10.1067/mob.2003.339
Lenz, J. D., Dillard, J. P. (2018). Pathogenesis of Neisseria Gonorrhoeae and the Host Defense in Ascending Infections of Human Fallopian Tube. Front. Immunol. 9, 2710. doi: 10.3389/fimmu.2018.02710
Liu, P. H., Hao, J. D., Li, W. Y., Tian, J., Zhao, J., Zeng, Y. M., et al. (2021). Congenital Cytomegalovirus Infection and the Risk of Hearing Loss in Childhood: A PRISMA-Compliant Meta-Analysis. Med. (Baltimore) 100 (36), e27057. doi: 10.1097/MD.0000000000027057
Maartens, G., Celum, C., Lewin, S. R. (2014). HIV Infection: Epidemiology, Pathogenesis, Treatment, and Prevention. Lancet 384 (9939), 258–271. doi; 10.1016/S0140-6736(14)60164-1
MacIntyre, D. A., Chandiramani, M., Lee, Y. S., Kindinger, L., Smith, A., Angelopoulos, N., et al. (2015). The Vaginal Microbiome During Pregnancy and the Postpartum Period in a European Population. Sci. Rep. 5, 8988. doi; 10.1038/srep08988
Maidji, E., McDonagh, S., Genbacev, O., Tabata, T., Pereira, L. (2006). Maternal Antibodies Enhance or Prevent Cytomegalovirus Infection in the Placenta by Neonatal Fc Receptor-Mediated Transcytosis. Am. J. Pathol. 168 (4), 1210–1226. doi: 10.2353/ajpath.2006.050482
Maki, Y., Fujisaki, M., Sato, Y., Sameshima, H. (2017). Candida Chorioamnionitis Leads to Preterm Birth and Adverse Fetal-Neonatal Outcome. Infect. Dis. Obstet. Gynecol. 2017, 9060138. doi: 10.1155/2017/9060138
Manolakaki, D., Velmahos, G., Kourkoumpetis, T., Chang, Y., Alam, H. B., De Moya, M. M., et al. (2010). Candida Infection and Colonization Among Trauma Patients. Virulence 1 (5), 367–375. doi: 10.4161/viru.1.5.12796
Marchant, A., Sadarangani, M., Garand, M., Dauby, N., Verhasselt, V., Pereira, L., et al. (2017). Maternal Immunisation: Collaborating With Mother Nature. Lancet Infect. Dis. 17 (7), e197–e208. doi: 10.1016/S1473-3099(17)30229-3
Margarita, V., Fiori, P. L., Rappelli, P. (2020). Impact of Symbiosis Between Trichomonas Vaginalis and Mycoplasma Hominis on Vaginal Dysbiosis: A Mini Review. Front. Cell Infect. Microbiol. 10, 179. doi: 10.3389/fcimb.2020.00179
Mateus, T., Silva, J., Maia, R. L., Teixeira, P. (2013). Listeriosis During Pregnancy: A Public Health Concern. ISRN Obstet. Gynecol. 2013, 851712. doi: 10.1155/2013/851712
Maucourant, C., Queiroz, G. A. N., Samri, A., Grassi, M. F. R., Yssel, H., Vieillard, V. (2019). Zika Virus in the Eye of the Cytokine Storm. Eur. Cytokine Netw. 30 (3), 74–81. doi; 10.1684/ecn.2019.0433
Maudet, C., Levallois, S., Disson, O., Lecuit, M. (2021). Innate Immune Responses to Listeria In Vivo. Curr. Opin. Microbiol. 59, 95–101. doi: 10.1016/j.mib.2020.11.006
Megli, C. J., Coyne, C. B. (2021). Infections at the Maternal-Fetal Interface: An Overview of Pathogenesis and Defence. Nat. Rev. Microbiol 20 (2), 67–82. doi: 10.1038/s41579-021-00610-y
Megli, C. J., Coyne, C. B. (2022). Infections at the Maternal–Fetal Interface: An Overview of Pathogenesis and Defence. Nat. Rev. Microbiol. 20 (2), 67–82. doi: 10.1038/s41579-021-00610-y
Mendz, G. L., Kaakoush, N. O., Quinlivan, J. A. (2013). Bacterial Aetiological Agents of Intra-Amniotic Infections and Preterm Birth in Pregnant Women. Front. Cell Infect. Microbiol. 3, 58. doi: 10.3389/fcimb.2013.00058
Milligan, C., Overbaugh, J. (2014). The Role of Cell-Associated Virus in Mother-to-Child HIV Transmission. J. Infect. Dis. 210 Suppl 3, S631–S640. doi: 10.1093/infdis/jiu344
Mills, S., Shanahan, F., Stanton, C., Hill, C., Coffey, A., Ross, R. P. (2013). Movers and Shakers. Gut Microbes 4 (1), 4–16. doi: 10.4161/gmic.22371
Mlakar, J., Korva, M., Tul, N., Popovic, M., Poljsak-Prijatelj, M., Mraz, J., et al. (2016). Zika Virus Associated With Microcephaly. N. Engl. J. Med. 374 (10), 951–958. doi: 10.1056/NEJMoa1600651
Mogensen, T. H. (2009). Pathogen Recognition and Inflammatory Signaling in Innate Immune Defenses. Clin. Microbiol. Rev. 22 (2), 240–273. doi: 10.1128/CMR.00046-08
Moncunill, G., Dobano, C., Gonzalez, R., Smolen, K. K., Manaca, M. N., Balcells, R., et al. (2020). Association of Maternal Factors and HIV Infection With Innate Cytokine Responses of Delivering Mothers and Newborns in Mozambique. Front. Microbiol. 11, 1452. doi: 10.3389/fmicb.2020.01452
Mor, G., Cardenas, I. (2010). The Immune System in Pregnancy: A Unique Complexity. Am. J. Reprod. Immunol. 63 (6), 425–433. doi; 10.1111/j.1600-0897.2010.00836.x
Murphy, S. P., Fast, L. D., Hanna, N. N., Sharma, S. (2005). Uterine NK Cells Mediate Inflammation-Induced Fetal Demise in IL-10-Null Mice. J. Immunol. 175 (6), 4084–4090. doi: 10.4049/jimmunol.175.6.4084
Murphy, S. P., Tayade, C., Ashkar, A. A., Hatta, K., Zhang, J., Croy, B. A. (2009). Interferon Gamma in Successful Pregnancies. Biol. Reprod. 80 (5), 848–859. doi: 10.1095/biolreprod.108.073353
Nanthakumar, M. P., Sood, A., Ahmed, M., Gupta, J. (2021). Varicella Zoster in Pregnancy. Eur. J. Obstet. Gynecol. Reprod. Biol. 258, 283–287. doi:10.1016/j.ejogrb.2021.01.009
Nasr, A., Allam, G., Hamid, O., Al-Ghamdi, A. (2014). IFN-Gamma and TNF Associated With Severe Falciparum Malaria Infection in Saudi Pregnant Women. Malaria J. 13 (1), 314. doi; 10.1186/1475-2875-13-314
Nguyen-Tien, T., Pham, L. T., Vu, D. T., Tran, S. H., Vu, L. T., Bui, V. N., et al. (2021). Knowledge and Practice on Prevention of Mosquito-Borne Diseases in Livestock-Keeping and non-Livestock-Keeping Communities in Hanoi City, Vietnam: A Mixed-Method Study. PloS One 16 (2), e0246032. doi: 10.1371/journal.pone.0246032
Ni, L., Lu, J. (2018). Interferon Gamma in Cancer Immunotherapy. Cancer Med. 7 (9), 4509–4516. doi: 10.1002/cam4.1700
Nimeri, N., Rahman, S., El Tinay, S., El Ansari, W., Tamano, E. A., Sellami, S., et al. (2013). The National Perinatal Mortality Rate in the State of Qatar During 2011; Trends Since 1990 and Comparative Analysis With Selected High-Income Countries: The PEARL Study Project. J. Perinat. Med. 41 (3), 323–330. doi: 10.1515/jpm-2012-0143
Nuriel-Ohayon, M., Neuman, H., Koren, O. (2016). Microbial Changes During Pregnancy, Birth, and Infancy. Front. Microbiol. 7, 1031. doi; 10.3389/fmicb.2016.01031
Offenbacher, S., Boggess, K. A., Murtha, A. P., Jared, H. L., Lieff, S., McKaig, R. G., et al. (2006). Progressive Periodontal Disease and Risk of Very Preterm Delivery. Obstet. Gynecol. 107 (1), 29–36. doi: 10.1097/01.AOG.0000190212.87012.96
Ornelas, A. M., Pezzuto, P., Silveira, P. P., Melo, F. O., Ferreira, T. A., Oliveira-Szejnfeld, P. S., et al. (2017). Immune Activation in Amniotic Fluid From Zika Virus-Associated Microcephaly. Ann. Neurol. 81 (1), 152–156. doi: 10.1002/ana.24839
Pansieri, C., Pandolfini, C., Clavenna, A., Choonara, I., Bonati, M. (2020). An Inventory of European Birth Cohorts. Int. J. Environ. Res. Public Health 17 (9), 3071. doi: 10.3390/ijerph17093071
Patras, K. A., Nizet, V. (2018). Group B Streptococcal Maternal Colonization and Neonatal Disease: Molecular Mechanisms and Preventative Approaches. Front. Pediatr. 6, 27. doi: 10.3389/fped.2018.00027
Phillips, C., Walsh, E. (2020). Group A Streptococcal Infection During Pregnancy and the Postpartum Period. Nurs. Womens Health 24 (1), 13–23. doi: 10.1016/j.nwh.2019.11.006
Piler, P., Kandrnal, V., Kukla, L., Andryskova, L., Svancara, J., Jarkovsky, J., et al. (2017). Cohort Profile: The European Longitudinal Study of Pregnancy and Childhood (ELSPAC) in the Czech Republic. Int. J. Epidemiol. 46 (5), 1379–137f. doi: 10.1093/ije/dyw091
Pinninti, S. G., Kimberlin, D. W. (2013). Neonatal Herpes Simplex Virus Infections. Pediatr. Clin. North Am. 60 (2), 351–365. doi: 10.1016/j.pcl.2012.12.005
Platt, D. J., Smith, A. M., Arora, N., Diamond, M. S., Coyne, C. B., Miner, J. J. (2018). Zika Virus-Related Neurotropic Flaviviruses Infect Human Placental Explants and Cause Fetal Demise in Mice. Sci. Transl. Med. 10 (426), eaao7090. doi: 10.1126/scitranslmed.aao7090
PrabhuDas, M., Bonney, E., Caron, K., Dey, S., Erlebacher, A., Fazleabas, A., et al. (2015). Immune Mechanisms at the Maternal-Fetal Interface: Perspectives and Challenges. Nat. Immunol. 16 (4), 328–334. doi: 10.1038/ni.3131
Primus, S., Rocha, S. C., Giacani, L., Parveen, N. (2020). Identification and Functional Assessment of the First Placental Adhesin of Treponema Pallidum That May Play Critical Role in Congenital Syphilis. Front. Microbiol. 11, 621654. doi: 10.3389/fmicb.2020.621654
Prince, A. L., Chu, D. M., Seferovic, M. D., Antony, K. M., Ma, J., Aagaard, K. M. (2015). The Perinatal Microbiome and Pregnancy: Moving Beyond the Vaginal Microbiome. Cold Spring Harb. Perspect. Med. 5 (6), a023051. doi: 10.1101/cshperspect.a023051
Prins, J. R., Gomez-Lopez, N., Robertson, S. A. (2012). Interleukin-6 in Pregnancy and Gestational Disorders. J. Reprod. Immunol. 95 (1-2), 1–14. doi: 10.1016/j.jri.2012.05.004
Quicke, K. M., Bowen, J. R., Johnson, E. L., McDonald, C. E., Ma, H., O'Neal, J. T., et al. (2016). Zika Virus Infects Human Placental Macrophages. Cell Host Microbe 20 (1), 83–90. doi: 10.1016/j.chom.2016.05.015
Racicot, K., Kwon, J. Y., Aldo, P., Abrahams, V., El-Guindy, A., Romero, R., et al. (2016). Type I Interferon Regulates the Placental Inflammatory Response to Bacteria and is Targeted by Virus: Mechanism of Polymicrobial Infection-Induced Preterm Birth. Am. J. Reprod. Immunol. 75 (4), 451–460. doi: 10.1111/aji.12501
Rahman, S., Al Rifai, H., El Ansari, W., Nimeri, N., El Tinay, S., Salameh, K., et al. (2012). A PEARL Study Analysis of National Neonatal, Early Neonatal, Late Neonatal, and Corrected Neonatal Mortality Rates in the State of Qatar During 2011: A Comparison With World Health Statistics 2011 and Qatar's Historic Data Over a Period of 36 Years (1975-2011). J. Clin. Neonatol. 1 (4), 195–201. doi: 10.4103/2249-4847.105990
Rasti, S., Asadi, M. A., Taghriri, A., Behrashi, M., Mousavie, G. (2014). Vaginal Candidiasis Complications on Pregnant Women. Jundishapur J. Microbiol. 7 (2), e10078. doi: 10.5812/jjm.10078
Ravel, J., Gajer, P., Abdo, Z., Schneider, G. M., Koenig, S. S., McCulle, S. L., et al. (2011). Vaginal Microbiome of Reproductive-Age Women. Proc. Natl. Acad. Sci. U. S. A. 108 Suppl 1, 4680–4687. doi: 10.1073/pnas.1002611107
Robinson, D. P., Klein, S. L. (2012). Pregnancy and Pregnancy-Associated Hormones Alter Immune Responses and Disease Pathogenesis. Horm. Behav. 62 (3), 263–271. doi: 10.1016/j.yhbeh.2012.02.023
Rodriguez-Melcon, C., Capita, R., Garcia-Fernandez, C., Alonso-Calleja, C. (2018). Effects of Bacteriophage P100 at Different Concentrations on the Structural Parameters of Listeria Monocytogenes Biofilms. J. Food Prot. 81 (12), 2040–2044. doi: 10.4315/0362-028X.JFP-18-177
Romero, R., Dey, S. K., Fisher, S. J. (2014a). Preterm Labor: One Syndrome, Many Causes. Science 345 (6198), 760–765. doi; 10.1126/science.1251816
Romero, R., Hassan, S. S., Gajer, P., Tarca, A. L., Fadrosh, D. W., Bieda, J., et al. (2014b). The Vaginal Microbiota of Pregnant Women Who Subsequently Have Spontaneous Preterm Labor and Delivery and Those With a Normal Delivery at Term. Microbiome 2, 18. doi: 10.1186/2049-2618-2-18
Romero, M., Leiba, E., Carrión-Nessi, F. S., Freitas-De Nobrega, D. C., Kaid-Bay, S., Gamardo, Á. F., et al. (2021). Malaria in Pregnancy Complications in Southern Venezuela. Malaria J. 20 (1), 186. doi: 10.1186/s12936-021-03728-9
Saadaoui, M., Kumar, M., Al Khodor, S. (2021). COVID-19 Infection During Pregnancy: Risk of Vertical Transmission, Fetal, and Neonatal Outcomes. J. Pers. Med. 11 (6), 483. doi: 10.3390/jpm11060483
Sacerdoti, F., Scalise, M. L., Burdet, J., Amaral, M. M., Franchi, A. M., Ibarra, C. (2018). Shiga Toxin-Producing Escherichia Coli Infections During Pregnancy. Microorganisms 6 (4), 111. doi: 10.3390/microorganisms6040111
Sadowsky, D. W., Adams, K. M., Gravett, M. G., Witkin, S. S., Novy, M. J. (2006). Preterm Labor is Induced by Intraamniotic Infusions of Interleukin-1beta and Tumor Necrosis Factor-Alpha But Not by Interleukin-6 or Interleukin-8 in a Nonhuman Primate Model. Am. J. Obstet. Gynecol. 195 (6), 1578–1589. doi: 10.1016/j.ajog.2006.06.072
Sasai, M., Yamamoto, M. (2019). Innate, Adaptive, and Cell-Autonomous Immunity Against Toxoplasma Gondii Infection. Exp. Mol. Med. 51 (12), 1–10. doi: 10.1038/s12276-019-0353-9
Scott, G. M., Chow, S. S., Craig, M. E., Pang, C. N., Hall, B., Wilkins, M. R., et al. (2012). Cytomegalovirus Infection During Pregnancy With Maternofetal Transmission Induces a Proinflammatory Cytokine Bias in Placenta and Amniotic Fluid. J. Infect. Dis. 205 (8), 1305–1310. doi: 10.1093/infdis/jis186
Seong, H. S., Lee, S. E., Kang, J. H., Romero, R., Yoon, B. H. (2008). The Frequency of Microbial Invasion of the Amniotic Cavity and Histologic Chorioamnionitis in Women at Term With Intact Membranes in the Presence or Absence of Labor. Am. J. Obstet. Gynecol. 199 (4), 375 e1–375 e5. doi: 10.1016/j.ajog.2008.06.040
Serrano, M. G., Parikh, H. I., Brooks, J. P., Edwards, D. J., Arodz, T. J., Edupuganti, L., et al. (2019). Racioethnic Diversity in the Dynamics of the Vaginal Microbiome During Pregnancy. Nat. Med. 25 (6), 1001–1011. doi: 10.1038/s41591-019-0465-8
Siriratsivawong, R., Pavlis, M., Hymes, S. R., Mintzer, J. P. (2014). Congenital Candidiasis: An Uncommon Skin Eruption Presenting at Birth. Cutis 93 (5), 229–232.
Smith, N. C., Goulart, C., Hayward, J. A., Kupz, A., Miller, C. M., van Dooren, G. G. (2021). Control of Human Toxoplasmosis. Int. J. Parasitol. 51 (2-3), 95–121. doi: 10.1016/j.ijpara.2020.11.001
Spence, T., Allsopp, P. J., Yeates, A. J., Mulhern, M. S., Strain, J. J., McSorley, E. M. (2021). Maternal Serum Cytokine Concentrations in Healthy Pregnancy and Preeclampsia. J. Pregnancy 2021, 6649608. doi: 10.1155/2021/6649608
Stewart, R. D., Bryant, S. N., Sheffield, J. S. (2013). West Nile Virus Infection in Pregnancy. Case Rep. Infect. Dis. 2013, 351872. doi: 10.1155/2013/351872
Straface, G., Selmin, A., Zanardo, V., De Santis, M., Ercoli, A., Scambia, G. (2012). Herpes Simplex Virus Infection in Pregnancy. Infect. Dis. Obstet. Gynecol. 2012, 385697. doi: 10.1155/2012/385697
Tabata, T., Petitt, M., Puerta-Guardo, H., Michlmayr, D., Wang, C., Fang-Hoover, J., et al. (2016). Zika Virus Targets Different Primary Human Placental Cells, Suggesting Two Routes for Vertical Transmission. Cell Host Microbe 20 (2), 155–166. doi: 10.1016/j.chom.2016.07.002
Tang, H., Hammack, C., Ogden, S. C., Wen, Z., Qian, X., Li, Y., et al. (2016). Zika Virus Infects Human Cortical Neural Progenitors and Attenuates Their Growth. Cell Stem Cell. 18 (5), 587–590. doi: 10.1016/j.stem.2016.02.016
Teixeira, H. C., Kaufmann, S. H. (1994). Role of NK1.1+ Cells in Experimental Listeriosis. NK1+ Cells are Early IFN-Gamma Producers But Impair Resistance to Listeria Monocytogenes Infection. J. Immunol. 152 (4), 1873–1882.
Thomas, J., Govender, N., McCarthy, K. M., Erasmus, L. K., Doyle, T. J., Allam, M., et al. (2020). Outbreak of Listeriosis in South Africa Associated With Processed Meat. N Engl. J. Med. 382 (7), 632–643. doi: 10.1056/NEJMoa1907462
Vallely, L. M., Egli-Gany, D., Wand, H., Pomat, W. S., Homer, C. S. E., Guy, R., et al. (2021). Adverse Pregnancy and Neonatal Outcomes Associated With Neisseria Gonorrhoeae: Systematic Review and Meta-Analysis. Sex Transm. Infect. 97 (2), 104–111. doi: 10.1136/sextrans-2020-054653
Villar, J., Ariff, S., Gunier, R. B., Thiruvengadam, R., Rauch, S., Kholin, A., et al. (2021). Maternal and Neonatal Morbidity and Mortality Among Pregnant Women With and Without COVID-19 Infection: The INTERCOVID Multinational Cohort Study. JAMA Pediatr. 175 (8), 817–826. doi: 10.1001/jamapediatrics.2021.1050
Waken, R. J., de Las Fuentes, L., Rao, D. C. (2017). A Review of the Genetics of Hypertension With a Focus on Gene-Environment Interactions. Curr. Hypertens. Rep. 19 (3), 23. doi: 10.1007/s11906-017-0718-1
Wampach, L., Heintz-Buschart, A., Fritz, J. V., Ramiro-Garcia, J., Habier, J., Herold, M., et al. (2018). Birth Mode Is Associated With Earliest Strain-Conferred Gut Microbiome Functions and Immunostimulatory Potential. Nat. Commun. 9 (1), 5091. doi: 10.1038/s41467-018-07631-x
Wang, Z., Tao, X., Liu, S., Zhao, Y., Yang, X. (2021). An Update Review on Listeria Infection in Pregnancy. Infect. Drug Resist. 14, 1967–1978. doi: 10.2147/IDR.S313675
Waters, J. P., Pober, J. S., Bradley, J. R. (2013). Tumour Necrosis Factor in Infectious Disease. J. Pathol. 230 (2), 132–147. doi: 10.1002/path.4187
Wicher, V., Wicher, K. (2001). Pathogenesis of Maternal-Fetal Syphilis Revisited. Clin. Infect. Dis. 33 (3), 354–363. doi: 10.1086/321904
Wilkie, G. L., Prabhu, M., Ona, S., Easter, S. R., Tuomala, R. E., Riley, L. E., et al. (2019). Microbiology and Antibiotic Resistance in Peripartum Bacteremia. Obstet. Gynecol. 133 (2), 269–275. doi: 10.1097/AOG.0000000000003055
Wilson, K. M., Di Camillo, C., Doughty, L., Dax, E. M. (2006). Humoral Immune Response to Primary Rubella Virus Infection. Clin. Vaccine Immunol. 13 (3), 380–386. doi: 10.1128/CVI.13.3.380-386.2006
Wong, Y. P., Tan, G. C., Wong, K. K., Anushia, S., Cheah, F. C. (2018). Gardnerella Vaginalis in Perinatology: An Overview of the Clinicopathological Correlation. Malays J. Pathol. 40 (3), 267–286.
Wylie, K. M., Weinstock, G. M., Storch, G. A. (2012). Emerging View of the Human Virome. Transl. Res. 160 (4), 283–290. doi: 10.1016/j.trsl.2012.03.006
Yan, R., Zhang, Y., Li, Y., Xia, L., Guo, Y., Zhou, Q. (2020). Structural Basis for the Recognition of SARS-CoV-2 by Full-Length Human ACE2. Science 367 (6485), 1444–1448. doi: 10.1126/science.abb2762
Yockey, L. J., Iwasaki, A. (2018a). Interferons and Proinflammatory Cytokines in Pregnancy and Fetal Development. Immunity 49 (3), 397–412. doi: 10.1016/j.immuni.2018.07.017
Yockey, L. J., Jurado, K. A., Arora, N., Millet, A., Rakib, T., Milano, K. M., et al. (2018b). Type I Interferons Instigate Fetal Demise After Zika Virus Infection. Sci. Immunol. 3 (19), eaao1680. doi: 10.1126/sciimmunol.aao1680
Zhang, D., Huang, Y., Ye, D. (2015). Intestinal Dysbiosis: An Emerging Cause of Pregnancy Complications? Med. Hypotheses 84 (3), 223–226. doi: 10.1016/j.mehy.2014.12.029
Zhang, L., Zhao, M., Jiao, F., Xu, X., Liu, X., Jiang, Y., et al. (2015). Interferon Gamma is Involved in Apoptosis of Trophoblast Cells at the Maternal-Fetal Interface Following Toxoplasma Gondii Infection. Int. J. Infect. Dis. 30, 10–16. doi: 10.1016/j.ijid.2014.10.027
Keywords: preterm labor, miscarriage, TORCH, pregnancy complications, microbiome
Citation: Kumar M, Saadaoui M and Al Khodor S (2022) Infections and Pregnancy: Effects on Maternal and Child Health. Front. Cell. Infect. Microbiol. 12:873253. doi: 10.3389/fcimb.2022.873253
Received: 10 February 2022; Accepted: 04 May 2022;
Published: 08 June 2022.
Edited by:
Maayan Levy, University of Pennsylvania, United StatesReviewed by:
Piyali Chatterjee, United States Department of Veterans Affairs, United StatesOlivier Disson, Institut Pasteur, France
Copyright © 2022 Kumar, Saadaoui and Al Khodor. This is an open-access article distributed under the terms of the Creative Commons Attribution License (CC BY). The use, distribution or reproduction in other forums is permitted, provided the original author(s) and the copyright owner(s) are credited and that the original publication in this journal is cited, in accordance with accepted academic practice. No use, distribution or reproduction is permitted which does not comply with these terms.
*Correspondence: Souhaila Al Khodor, c2Fsa2hvZG9yQHNpZHJhLm9yZw==