- 1Department of Infectious Diseases and Immunology, University of Florida, Gainesville, FL, United States
- 2Genetics Institute, University of Florida, Gainesville, FL, United States
- 3Emerging Pathogens Institute, University of Florida, Gainesville, FL, United States
Apicomplexan parasites live in hostile environments in which they are challenged chemically and their hosts attempt in many ways to kill them. In response, the parasites have evolved multiple mechanisms that take advantage of these challenges to enhance their survival. Perhaps the most impressive example is the evolutionary co-option of DNA repair mechanisms by the parasites as a means to rapidly manipulate the structure, antigenicity, and expression of the products of specific multigene families. The purpose of variant proteins that mediate cytoadhesion has long been thought to be primarily the avoidance of splenic clearance. Based upon known biology, I present an alternative perspective in which it is survival of the oxidative environment within which Babesia spp. parasites live that has driven integration of DNA repair, antigenic variation, and cytoadhesion, and speculate on how genome organization affects that integration. This perspective has ramifications for the development of parasite control strategies.
Introduction
Many unsuccessful years of effort aimed at continuous in vitro cultivation of Plasmodium falciparum came to fruition in 1976 at the Trager Laboratory of Rockefeller University. A key element turned out to be the fortuitous use of a “candle jar” to provide the elevated CO2 atmosphere assumed to be needed for growth, which also had lowered the O2 tension (Trager and Jensen, 1976). When parasites were found to continue replicating it was realized, and later established, that P. falciparum is a microaerophile (Scheibel et al., 1979). This lead was followed in establishing an in vitro culture system for the related hemoparasite, Babesia bovis, which also thrives under microaerophilic conditions (Levy and Ristic, 1980). This trait may be important in unobvious ways.
The shared trait of microaerophilia dovetails with another bit of shared biology in these two species: infected-erythrocytes (IE) of each species cytoadhere to the capillary and post-capillary venous endothelium of major organs [reviewed elsewhere (Allred and Al-Khedery, 2004)]. Cytoadhesion is thought to mediate avoidance of IE passage through the spleen, where disruption of normal erythrocyte membrane characteristics and rheologic properties might result in their removal (Berendt et al., 1994; Allred et al., 2003). However, it is likely that cytoadhesion also provides the opportunity to complete developmental maturation and genome replication under hypoxic conditions. While all Babesia and Plasmodium parasites studied to date benefit from reduced oxygen tension (Trager and Jensen, 1976; Levy and Ristic, 1980; Vega et al., 1985; Takagi and Waki, 1987; Long et al., 1989; Zweygarth et al., 1995; Chotivanich et al., 2001; Sunaga et al., 2002; McCormack et al., 2019), only a few are known to cytoadhere or sequester as blood stages. If hypoxic conditions during development are not essential, then why does cytoadhesion occur at all? I propose for consideration that the answer is the intersection of DNA repair, cytoadhesion, antigenic variation … and chance.
Why Cytoadhesion?
Cytoadhesion in B. bovis is mediated through the VESA1 protein (O’Connor and Allred, 2000), a heterodimeric protein with two subunits, VESA1a and 1b (Allred et al., 1993; Allred et al., 1994; O’Connor et al., 1997). By contrast, in P. falciparum this function is mediated by a monomeric protein, PfEMP1 (Baruch et al., 1995; Smith et al., 1995; Su et al., 1995). Despite the shared function and similar ecological niches occupied by each species, the proteins mediating this function and the genes encoding them are unrelated. They do, however, share the trait of being members of large multigene families that arose through amplification and diversification of progenitor genes. Among the Babesia sensu stricto, recognizable ves multigene families encoding VESA1-related proteins are consistently present but have undergone further evolutionary innovation and functional diversification into at least two unique branches in each species (Jackson et al., 2014). In B. bovis, this diversity is manifested by the two major branches, ves1α and ves1β, together comprised of over 110 members, and a third branch of three ves1γ genes. Other Babesia spp. are not known to undergo ligand-mediated cytoadhesion. Why are these amplified gene families retained when most species do not cytoadhere? The answer may be that cytoadhesion has repeatedly arisen independently during speciation in different parasite lineages. In the case of Babesia spp. the ves gene families of different species all differ significantly in sequence and sometimes structure, yet are consistent in these traits within a species. Despite these differences there is widespread synteny among Babesia spp. in chromosomal regions inhabited by ves loci. This indicates the presence of amplified ves loci in a common ancestor (Jackson et al., 2014), with subsequent innovation and selection, including evolution of ves gene sequences and structures capable of mediating cytoadhesion in B. bovis during or after speciation. By definition, speciation is the outcome of having arrived at different evolutionary solutions to survival challenges. One cannot expect all species to cytoadhere if this trait was not present in the progenitor, and most do not. It is significant that cytoadhesion also arose in P. falciparum and closely related species, whereas other Plasmodium spp. do not share var genes or cytoadhere.
How could cytoadhesion provide sufficient selection for it to repeatedly arise independently? The evolution of cytoadhesion in B. bovis was accompanied by formation of abnormal ridge-like structures in the IE membrane at which VESA1 cytoadhesion ligands are assembled (Aikawa et al., 1997; O’Connor and Allred, 2000; Xiao et al., 2010). As most VESA1 protein isoforms are not adhesive these ridge-like modifications would increase IE removal by the spleen and would be deleterious if they did not serve some beneficial purpose (O’Connor et al., 1999). Despite ready availability of many nutrients in the plasma, to live within a red blood cell is to survive in a harsh, difficult environment. Intraerythrocytic parasites must contend with significant challenges, such as surviving frequent rheologic contortions, obtaining nutrients despite being surrounded by membranes of host origin, and modifying those membranes to serve the parasite’s purposes. One major challenge is simply living within a pool of hemoglobin (Hb). The parasite first must compete with Hb for O2 needed for its own metabolism (Barry, 1984). As plasma pH drops within hypoxic tissues the parasite is then inundated with free O2 given up by Hb. As the O2 diffuses in all directions the parasite lives in the line of fire. During the brief bouts of high O2 partial pressure oxidative damage may occur to many components, perhaps exacerbated by hemoglobin peroxidase activity and associated radicals (Reeder, 2017; Zhang et al., 2020). It is clear that appreciable oxidative damage occurs, based upon the accumulation of the lipid oxidation product, malondialdehyde, in IE membranes and fragmentation of DNA (Commins et al., 1988; Murase et al., 1996; Esmaeilnejad et al., 2020), challenging parasite genome integrity. Malondialdehyde is itself carcinogenic, forming DNA base adducts (Marnett, 2000). Damage generated by reactive oxygen species or nitrosylation from innate immune responses also would contribute to selective pressure (Asada et al., 2015; Zhang et al., 2020). Cytoadhesive parasites avoid both clearance by the spleen and genotoxic damage from O2 acquired in the lungs. The advantage of enhanced genome integrity may have offset the accompanying enhanced susceptibility to splenic removal. Therefore, both the spleen and lungs would be applying different selective pressures, in a synergistic manner.
How Do the Parasites Respond to Genotoxic Stress?
Oxidation damage occurring to DNA typically occurs in the form of oxidized bases, most commonly 8-oxo-7,8-dihydroguanine (8-OG), although many forms are possible (Merta et al., 2019; Kumar et al., 2020). Reactive nitrogen species (RNS) may also damage DNA and affect parasite viability, including through direct deamination (Dong and Dedon, 2006; Ohshima et al., 2006; Asada et al., 2015; Li et al., 2017). Damaged bases must be replaced in order to allow replication to continue and to maintain integrity of the genome (Radak and Boldogh, 2010; Garcia-Rodriguez et al., 2018; Kumar et al., 2020). Mechanisms for base replacement involve a single- and often double-stranded DNA break- a potentially lethal event. Repair of these breaks is essential to maintaining genome integrity. Viewed differently, the cycles of damage and repair provide opportunities for loosely targeted sequence modifications during the repair process, an important point to which I will return.
Beyond sparse experimental evidence and what can be gleaned from genomic sequences, little is known about DNA repair among apicomplexan parasites, other than it is a robust example of the adage, “less is more”. For example, canonical non-homologous end-joining (cNHEJ) is a major repair process in higher eukaryotes (Rulten and Grundy, 2017) and would seem essential for haploid asexual parasites. Yet, while present in Toxoplasma gondii, most or all of the enzymatic machinery for cNHEJ is absent in Babesia and most apicomplexan parasites (Fox et al., 2011; Mack et al., 2019; Nenarokova et al., 2019). Homologous recombination (HR), another major repair process in eukaryotes, is also not utilized equivalently by B. bovis. Rad51 (and related) proteins are integral to HR (Symington et al., 2014), yet knock-out of the B. bovis Bbrad51 gene surprisingly had no effect on in vitro parasite survival, growth rate, or chromosome reassembly kinetics following γ-irradiation. Although the sensitivity phenotype of Bbrad51 knockouts for the alkylating agent, methylmethane sulfonate, is consistent, it is not large (Mack et al., 2019). Loss of BbRad51 does, however, eliminate the ability to recombine exogenous DNA into the B. bovis genome, an HR form of recombination (Mack et al., 2019). Thus, the overall in vitro viability of parasites is unaffected by simultaneous natural and induced lack of cNHEJ and HR, respectively, suggesting that alternative mechanisms are robust at genome maintenance in the absence of overt insult.
Cytoadhesion and DNA Repair Are Related?
It was recognized more than 50 years ago that the surface of B. bovis-IE is antigenically distinct from that of uninfected erythrocytes (Callow, 1968). This difference is due at least in part to parasite-derived proteins integrated into the IE membrane (Allred et al., 1993). Cytoadhesion depends upon parasite-derived components that are targets of host adaptive immunity. In an in vitro assay antibody recognition of the cytoadhesion ligand, the protein VESA1, was found to block or reverse this function (O’Connor and Allred, 2000). Under constant hydrodynamic shear in vivo this presumably would result in the IE re-entering the circulation covered in antibody, and removal by the spleen (Allred, 1995). This outcome was demonstrated directly for P. falciparum in vivo cytoadhesion and sequestration in Aotus monkeys (David et al., 1983). In the case of B. bovis the antigenicity of VESA1 was found to vary clonally over brief periods in the infected host, reflecting rapid antigenic variation (Allred et al., 1994). This finding led to identification of the ves multigene family encoding VESA1 polypeptides (Allred et al., 2000; Xiao et al., 2010), and determination that variation is achieved through a process of segmental gene conversion (SGC) (Al-Khedery and Allred, 2006). During SGC short segments (109 bp on average) of the actively transcribed ves genes are replaced with alternative sequences from silent ves genes in loci scattered about the genome (Al-Khedery and Allred, 2006; Mack et al., 2020). The outcome of this back-and-forth between recognition and destruction versus variation and escape is long-term persistence with wide population fluctuations (Allred et al., 1994; Calder et al., 1996). Not surprisingly, cytoadhesive function varies along with ligand antigenicity (O’Connor and Allred, 2000), and selection by survival may determine the major expressed isoform within the parasite population.
The ves multigene family is large and scattered over all B. bovis chromosomes (Al-Khedery and Allred, 2006; Brayton et al., 2007), yet only one ves locus (comprised of one ves1α and one ves1β) is transcriptionally active at a time, the locus of active ves transcription (LAT) (Al-Khedery and Allred, 2006; Żupańska et al., 2009; Mack et al., 2020). Importantly, transcriptionally active chromatin is more susceptible than silent chromatin to adoption of oxidation-prone higher-order structure, damage, and mutation (Makova and Hardison, 2015). It remains unclear how such damage is repaired, but we propose that the observed unidirectional movement of duplicated sequence patches that identifies SGC reflects the repair process. In most instances SGC appears as though it represents canonical HR, but this was not supported by knock-out of the Bbrad51 gene, which had little effect on SGC (Mack et al., 2019; Mack et al., 2020). As the Rad51 superfamily is pivotal to HR throughout all three biological Kingdoms (Jiang et al., 2018) this outcome raises real doubt that SGC is a product of classical HR. While the underlying mechanisms are unclear, the result of SGC is a seemingly endless variety of slightly modified alternative versions of the actively transcribed ves genes and the proteins they encode.
As a mechanism to create structural and antigenic diversity of VESA1 proteins, SGC seems ideal. VESA1 has minimal well-conserved sequences and, given its massive sequence variability, likely accommodates considerable tertiary and quaternary structural diversity despite maintaining consistent overall structural organization of each subunit (Allred et al., 2000; Al-Khedery and Allred, 2006; Brayton et al., 2007; Jackson et al., 2014). SGC occurs in both ves1α and ves1β genes (Al-Khedery and Allred, 2006), and VESA1 holoproteins are comprised of comparably variant VESA1a and 1b subunits. The variety that can be expressed, and thus the unique molecular space that can be sampled by the protein’s surface, is extremely large. Consistent with in vitro observations (O’Connor et al., 1999) one would anticipate that many- perhaps most- VESA1 variants are non-functional in cytoadhesion. However, the extreme diversity arising from the assembly of mosaic ves genes and proteins, and the correspondingly large molecular space that can be sampled results in selection of VESA1 isoforms capable of binding to one or more endothelial receptors. With the enhanced in vivo survival of cytoadhesive parasites and immunologic elimination of non-cytoadhesive parasites, episodic establishment of dominant variant populations differing in their adhesive specificities would be favored. Such extreme variability, coupled with repeated rounds of positive selection and amplification, is essentially the same mechanism as that underlying in vivo peptide phage display (Pasqualini and Ruoslahti, 1996; George et al., 2003), albeit on a larger structural scale, and is readily mimicked in the laboratory (O’Connor et al., 1999).
Discussion
The survival of B. bovis relies upon DNA repair mechanisms resulting in creation of variant protein structures. Thus, there is a real need to understand these mechanisms and their ramifications. How could sequence patches recombine into transcribed ves genes to replace existing sequences by a non-HR process without affecting the donor? One possibility is that this feat might be accomplished by repair enzymes with the capacity to switch templates repeatedly during replication or repair, similar to the formation of immunoglobulin genes in birds (Nakazato et al., 2018). In the B. bovis genome a single Polζ DNA polymerase can be identified, orthologs of which have template-switching capacity in yeast and mammalian cells (reviewed in (Brayton et al., 2007; Northam et al., 2013; Martin and Wood, 2019)). Interestingly, among Plasmodium spp. the presence of a Polζ ortholog influences the extent of antigenic variation (Kirkman and Deitsch, 2020; Siao et al., 2020). Template-switching involving a second ves locus as template would be facilitated by close proximity among ves genes. Evidence consistent with such proximity was found in a limited chromatin-conformation capture assay (Wang et al., 2012). Similarly, organization of P. falciparum var genes into “bouquets” at the nuclear periphery was observed (Freitas-Junior et al., 2000), likely facilitating the cascade of crossover reactions occurring during repair of double-strand DNA breaks in the var gene family encoding PfEMP1 (Zhang et al., 2019).
How are SGC-mediated sequence changes focused on ves genes? As mentioned, B. bovis lives within an environment that experiences periodic high levels of oxidative stress. The guanine bases of G-G dinucleotide pairs present in G-quadruplex (G4) DNA are highly susceptible to oxidation, being readily damaged to give 8-OG (Fleming et al., 2017; Merta et al., 2019). A simple search of the B. bovis genome for sequences predicted to be competent to form G4 structure reveals that such sequences are highly enriched within or near (≤ 8 Kbp) ves loci (Figure 1). The full extent of enrichment is difficult to predict, as G4 structure may form with only two stacked G-quartets (Figure 1A), or may involve three or more (Figure 1B). Due to a need for DNA strand separation in order to form, actively transcribed DNA is far more prone to G4 formation than non-transcribed DNA (Rodriguez et al., 2012). This would have the effect of focusing oxidative damage and mutation on the ves family and specifically the LAT, and could act as a trigger for the SGC process. The G4 “focusing” effect is so strong that it serves to regulate promoter function in some DNA repair protein genes (Clark et al., 2012; Fleming et al., 2019). In concert with a second translesion polymerase, Rev1, DNA Polζ activity is important to lesion bypass of damaged bases such as 8-OG and stalled replication forks (Freisinger et al., 2004; Northam et al., 2013). Orthologs of both Rev1 and Polζ translesion polymerases are transcribed by asexual stage B. bovis parasites (Brayton et al., 2007; Pedroni et al., 2013). The significance of these components to SGC, antigenic variation, and cytoadhesion is not known, but we are pursuing this potential connection.
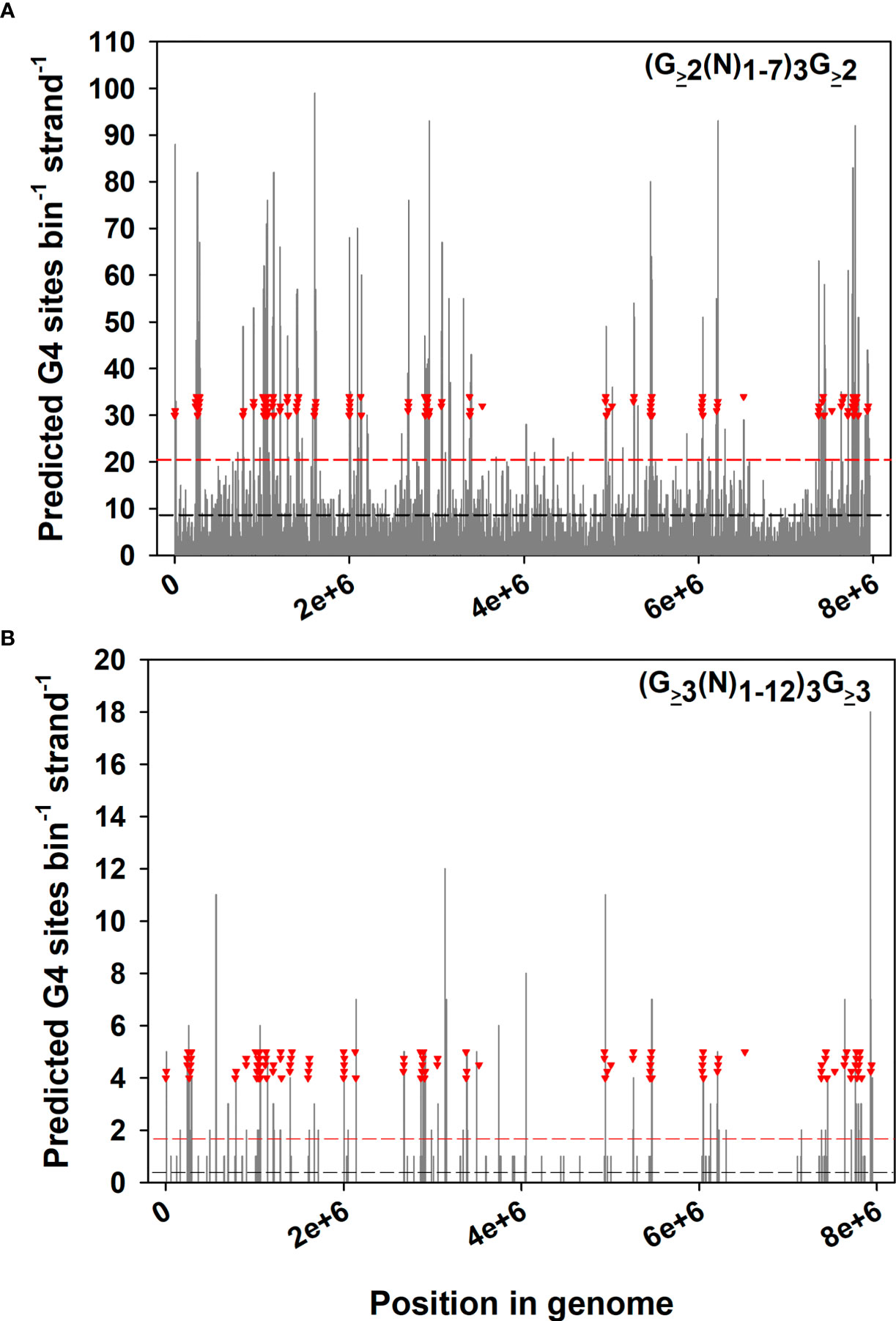
Figure 1 Distribution of predicted G-quadruplex sequences relative to ves genes in the B. bovis genome. The B. bovis C9.1 genome (Jackson et al., 2014) was concatenated and surveyed for G4 motifs, using (A) the low stringency motif (G≥2(N)1-7)3G≥2 or (B) a high-stringency motif (G≥3(N)1-12)3G≥3. These results were plotted as histograms of G4 density per 8 Kbp genome segments. Inverted red triangles indicate the “left”-most ends of ves coding sequences. The dashed horizontal lines represent the mean G4 density across the genome (black), or +2 s.d. (red).
To summarize, hemoparasites live in a highly oxidative environment, and I suggest that cytoadhesion evolved to mitigate this damage. The B. bovis genome is greatly enriched in sequences with the potential to form G4 structure within and/or near ves genes. As G4 DNA is formed primarily within actively-transcribed sequences, can disrupt replication, and is overtly susceptible to oxidative damage this could make the LAT a sensitive target for damage. Repair of damage via a translesion polymerase with template-switching capabilities could both help to maintain overall genome integrity and result in local inclusion of ectopic sequence patches, the basis of SGC. Mosaic VESA1 protein isoforms created by SGC enable selection for adhesion, simultaneously avoiding immune recognition and oxidative damage. The ability to disrupt important mediators of SGC could not only affect parasite viability directly, but also diminish antigenic variation and cytoadhesion, reducing parasite survival and pathology.
Data Availability Statement
Publicly available datasets were analyzed in this study. This data can be found here: https://www.sanger.ac.uk/resources/downloads/protozoa/babesia-bovis.html.
Author Contributions
The author confirms being the sole contributor of this work and has approved it for publication.
Funding
The author was supported by a University of Florida College of Veterinary Medicine intramural grant, account 00131124.
Conflict of Interest
The author declares that the research was conducted in the absence of any commercial or financial relationships that could be construed as a potential conflict of interest.
Publisher’s Note
All claims expressed in this article are solely those of the authors and do not necessarily represent those of their affiliated organizations, or those of the publisher, the editors and the reviewers. Any product that may be evaluated in this article, or claim that may be made by its manufacturer, is not guaranteed or endorsed by the publisher.
Acknowledgments
The author wishes to thank Erin Mack for critically reviewing early drafts of this manuscript.
References
Aikawa, M., Dawazu, S. I., Kamio, T., Matsumoto, Y., Naya, T., Torii, M., et al. (1997). Membrane Knobs of Unfixed Babesia Bovis-Infected Erythrocytes: New Findings as Revealed by Atomic Force Microscopy and Surface Potential Spectroscopy. Parasitol. Int. 46 (4), 241–246. doi: 10.1016/S1383-5769(97)00031-7
Al-Khedery, B., Allred, D. R. (2006). Antigenic Variation in Babesia Bovis Occurs Through Segmental Gene Conversion of the Ves Multigene Family, Within a Bidirectional Site of Transcription. Mol. Microbiol. 59, 402–414. doi: 10.1111/j.1365-2958.2005.04993.x
Allred, D. R. (1995). Immune Evasion by Babesia Bovis and Plasmodium Falciparum: Cliff-Dwellers of the Parasite World. Parasitol. Today 11, 100–105. doi: 10.1016/0169-4758(95)80166-9
Allred, D. R., Al-Khedery, B. (2004). Antigenic Variation and Cytoadhesion in Babesia Bovis and Plasmodium Falciparum: Different Logics Achieve the Same Goal. Mol. Biochem. Parasitol. 134, 27–35. doi: 10.1016/j.molbiopara.2003.09.012
Allred, D. R., Al-Khedery, B., O’Connor, R. M. (2003). “Antigenic Variation and its Significance to Babesia,” in Antigenic Variation. Eds. Craig, A., Scherf, A. (Oxford: Elsevier Science Ltd), 273–290.
Allred, D. R., Carlton, J. M.-R., Satcher, R. L., Long, J. A., Brown, W. C., Patterson, P. E., et al. (2000). The Ves Multigene Family of B. Bovis Encodes Components of Rapid Antigenic Variation at the Infected Erythrocyte Surface. Mol. Cell 5, 153–162. doi: 10.1016/S1097-2765(00)80411-6
Allred, D. R., Cinque, R. M., Lane, T. J., Ahrens, K. P. (1994). Antigenic Variation of Parasite-Derived Antigens on the Surface of Babesia Bovis-Infected Erythrocytes. Infect. Immun. 62, 91–98. doi: 10.1128/iai.62.1.91-98.1994
Allred, D. R., Hines, S. A., Ahrens, K. P. (1993). Isolate-Specific Parasite Antigens of the Babesia Bovis-Infected Erythrocyte Surface. Mol. Biochem. Parasitol. 60, 121–132. doi: 10.1016/0166-6851(93)90035-V
Asada, M., Yahata, K., Hakimi, H., Yokoyama, N., Igarashi, I., Kaneko, O., et al. (2015). Transfection of Babesia Bovis by Double Selection With WR99210 and Blasticidin-S and its Application for Functional Analysis of Thioredoxin Peroxidase-1. PloS One 10 (5), e0125993. doi: 10.1371/journal.pone.0125993
Barry, D. N. (1984). Metabolism of Babesia Parasites In Vitro. Glucose and Energy Metabolism of B. Bovis. Aust. J. Exp. Biol. Med. Sci. 62 (1), 53–61. doi: 10.1038/icb.1984.5
Baruch, D. I., Pasloske, B. L., Singh, H. B., Bi, X., Ma, X. C., Feldman, M., et al. (1995). Cloning the P. Falciparum Gene Encoding PfEMP1, A Malarial Variant Antigen and Adherence Receptor on the Surface of Parasitized Human Erythrocytes. Cell 82, 77–87. doi: 10.1016/0092-8674(95)90054-3
Berendt, A. R., Turner, G. D. H., Newbold, C. I. (1994). Cerebral Malaria: The Sequestration Hypothesis. Parasitol. Today 10, 412–414. doi: 10.1016/0169-4758(94)90238-0
Brayton, K. A., Lau, A. O. T., Herndon, D. R., Hannick, L., Kappmeyer, L. S., Berens, S. J., et al. (2007). Genome Sequence of Babesia Bovis and Comparative Analysis of Apicomplexan Hemoprotozoa. PloS Pathog. 3, e148. doi: 10.1371/journal.ppat.0030148
Calder, J. A. M., Reddy, G. R., Chieves, L., Courtney, C. H., Littell, R., Livengood, J. R., et al. (1996). Monitoring Babesia Bovis Infections in Cattle by Using PCR-Based Tests. J. Clin. Microbiol. 34, 2748–2755. doi: 10.1128/jcm.34.11.2748-2755.1996
Callow, L. L. (1968). A Note on Homologous Strain Immunity in Babesia Argentina Infections. Aust. Vet. J. 44, 268–269. doi: 10.1111/j.1751-0813.1968.tb04976.x
Chotivanich, K., Silamut, K., Udomsangpetch, R., Stepniewska, K. A., Pukrittayakamee, S., Looareesuwan, S., et al. (2001). Ex-Vivo Short-Term Culture and Developmental Assessment of Plasmodium Vivax. Trans. R. Soc. Trop. Med. Hyg. 95, 677–680. doi: 10.1016/S0035-9203(01)90113-0
Clark, D. W., Phang, T., Edwards, M. G., Geraci, M. W., Gillespie, M. N. (2012). Promoter G-Quadruplex Sequences are Targets for Base Oxidation and Strand Cleavage During Hypoxia-Induced Transcription. Free Radical Biol. Med. 53 (1), 51–59. doi: 10.1016/j.freeradbiomed.2012.04.024
Commins, M. A., Goodger, B. V., Waltisbuhl, D. J., Wright, I. G. (1988). Babesia Bovis: Studies of Parameters Influencing Microvascular Stasis of Infected Eryrthrocytes. Res. Vet. Sci. 44, 226–228. doi: 10.1016/S0034-5288(18)30844-0
David, P. H., Hommel, M., Miller, L. H., Udeinya, I. J., Oligino, L. D. (1983). Parasite Sequestration in Plasmodium Falciparum Malaria: Spleen and Antibody Modulation of Cytoadherence of Infected Erythrocytes. Proc. Natl. Acad. Sci. (USA) 80, 5075–5079. doi: 10.1073/pnas.80.16.5075
Dong, M., Dedon, P. C. (2006). Relatively Small Increases in the Steady-State Levels of Nucleobase Deamination Products in DNA From Human TK6 Cells Exposed to Toxic Levels of Nitric Oxide. Chem. Res. Toxicol. 19, 50–57. doi: 10.1021/tx050252j
Esmaeilnejad, B., Tavassoli, M., Dalir-Naghadeh, B., Samiei, A., Rajabi, S., Mohammadi, V., et al. (2020). Status of Oxidative Stress, Trace Elements, Sialic Acid and Cholinesterase Activity in Cattle Naturally Infected With Babesia Bigemina. Comp. Immunol. Microbiol. Infect. Dis. 71, 101503. doi: 10.1016/j.cimid.2020.101503
Fleming, A. M., Zhu, J., Ding, Y., Burrows, C. J. (2017). 8-Oxo-7,8-Dihydroguanine in the Context of a Gene Promoter G-Quadruplex is an on-Off Switch for Transcription. ACS Chem. Biol. 12, 2417–2426. doi: 10.1021/acschembio.7b00636
Fleming, A. M., Zhu, J., Ding, Y., Burrows, C. J. (2019). Location Dependence of the Transcriptional Response of a Potential G-Quadruplex in Gene Promoters Under Oxidative Stress. Nucleic Acids Res. 47 (10), 5049–5060. doi: 10.1093/nar/gkz207
Fox, B. A., Falla, A., Rommereim, L. M., Tomita, T., Gigley, J. P., Mercier, C., et al. (2011). Type II Toxoplasma Gondii KU80 Knockout Strains Enable Functional Analysis of Genes Required for Cyst Development and Latent Infection. Eukaryot. Cell 10, 1193–1206. doi: 10.1128/EC.00297-10
Freisinger, E., Grollman, A. P., Miller, H., Kisker, C. (2004). Lesion (in)Tolerance Reveals Insights Into DNA Replication Fidelity. EMBO J. 23, 1493–1505. doi: 10.1038/sj.emboj.7600158
Freitas-Junior, L. H., Bottius, E., Pirrit, L. A., Deitsch, K. W., Scheidig, C., Guinet, F., et al. (2000). Frequent Ectopic Recombination of Virulence Factor Genes in Telomeric Chromosome Clusters in P. Falciparum. Nature 407, 1018–1022. doi: 10.1038/35039531
Garcia-Rodriguez, N., Wong, R. P., Ulrich, H. D. (2018). The Helicase Pif1 Functions in the Template Switching Pathway of DNA Damage Bypass. Nucleic Acids Res. 46 (16), 8347–8356. doi: 10.1093/nar/gky648
George, A. J. T., Lee, L., Pitzalis, C. (2003). Isolating Ligands Specific for Human Vasculature Using In Vivo Phage Selection. Trends Biotechnol. 21, 199–203. doi: 10.1016/S0167-7799(03)00079-9
Jackson, A. P., Otto, T. D., Darby, A., Ramaprasad, A., Xia, D., Echaide, I. E., et al. (2014). The Evolutionary Dynamics of Variant Antigen Genes in Babesia Reveal a History of Genomic Innovation Underlying Host-Parasite Interaction. Nucleic Acids Res. 42, 7113–7131. doi: 10.1093/nar/gku322
Jiang, S., Lin, T. L., Xie, Q., Wang, L. (2018). Network Analysis of RAD51 Proteins in Metazoa and the Evolutionary Relationships With Their Archaeal Homologs. Front. Genet. 9, 383. doi: 10.3389/fgene.2018.00383
Kirkman, L. A., Deitsch, K. W. (2020). Vive La Difference: Exploiting the Differences Between Rodent and Human Malarias. Trends Parasitol. 36 (6), 504–511. doi: 10.1016/j.pt.2020.03.008
Kumar, N., Raja, S., Van Houten, B. (2020). The Involvement of Nucelotide Excision Repair Proteins in the Removal of Oxidative DNA Damage. Nucleic Acids Res. 48 (20), 11227–11243. doi: 10.1093/nar/gkaa777
Levy, M. G., Ristic, M. (1980). Babesia Bovis: Continuous Cultivation in a Microaerophilous Stationary Phase Culture. Science 207, 1218–1220. doi: 10.1126/science.7355284
Li, M., Chen, Q., Ma, T., Yu, X. (2017). Targeting Reactive Nitrogen Species Suppresses Hereditary Pancreatic Cancer. Proc. Natl. Acad. Sci. U S A 114 (27), 7106–7111. doi: 10.1073/pnas.1702156114
Long, G. W., Leath, S., Schuman, R., Hollingdale, M. R., Ballou, W. R., Sim, B. K. L., et al. (1989). Cultivation of the Exoerythrocytic Stage of Plasmodium Berghei in Primary Cultures of Mouse Hepatocytes and Continuous Mouse Cell Lines. Vitro Cell. Dev. Biol. 25 (9), 857–862. doi: 10.1007/BF02623670
Mack, E. A., Tagliamonte, M. S., Xiao, Y.-P., Quesada, S., Allred, D. R. (2020). Babesia Bovis Rad51 Ortholog Influences Switching of Ves Genes But is Not Essential for Segmental Gene Conversion in Antigenic Variation. PloS Pathog. 16 (8), e1008772. doi: 10.1371/journal.ppat.1008772
Mack, E. A., Xiao, Y.-P., Allred, D. R. (2019). Knockout of Babesia Bovis Rad51 Ortholog and its Complementation by Expression From the BbACc3 Artificial Chromosome Platform. PloS One 14 (8), e0215882. doi: 10.1371/journal.pone.0215882
Makova, K. D., Hardison, R. C. (2015). The Effects of Chromatin Organization on Variation in Mutation Rates in the Genome. Nat. Rev. Genet. 16, 213–233. doi: 10.1038/nrg3890
Marnett, L. J. (2000). Oxyradicals and DNA Damage. Carcinogenesis 21 (3), 361–370. doi: 10.1093/carcin/21.3.361
Martin, S. K., Wood, R. D. (2019). DNA Polymerase ζ in DNA Replication and Repair. Nucleic Acids Res. 47, 8348–8361. doi: 10.1093/nar/gkz705
McCormack, K. A., Alhaboubi, A., Pollard, D. A., fFuller, L., Holman, P. J. (2019). In Vitro Cultivation of Babesia Duncani (Apicomplexa: Babesiidae), A Zoonotic Hemoprotozoan, Using Infected Blood From Syrian Hamsters (Mesocricetus Auratus). Parasitol. Res. 118, 2409–2417. doi: 10.1007/s00436-019-06372-0
Merta, T. J., Geacintov, N. E., Shafirovich, V. (2019). Generation of 8-Oxo-7,8-Dihydroguanine in G-Quadruplexes Models of Human Telomere Sequences by One-Electron Oxidation. Photochem. Photobiol. 95, 244–251. doi: 10.1111/php.12926
Murase, T., Ueda, T., Yamato, O., Tajima, M., Maede, Y. (1996). Oxidative Damage and Enhanced Erythrophagocytosis in Canine Erythrocytes Infected With Babesia Gibsoni. J. Vet. Med. Sci. 58, 259–261. doi: 10.1292/jvms.58.259
Nakazato, A., Kajita, K., Ooka, M., Akagawa, R., Abe, T., Takeda, S., et al. (2018). SPARTAN Promotes Genetic Diversification of the Immunoglobulin-Vaiable Gene Locus in Avian DT40 Cells. DNA Repair 68, 50–57. doi: 10.1016/j.dnarep.2018.06.003
Nenarokova, A., Záhonová, K., Krasilnikova, M., Gahura, O., McCulloch, R., Ziková, A., et al. (2019). Causes and Effects of Loss of Classical Nonhomlogous End Joining Pathway in Parasitic Eukaryotes. mBio 10, e01541–e01519. doi: 10.1128/mBio.01541-19
Northam, M. R., Moore, E. A., Mertz, T. M., Binz, S. K., Stith, C. M., Stepchenkova, E. I., et al. (2013). DNA Polymerases ζ and Rev1 Mediate Error-Prone Bypass of non-B DNA Structures. Nucleic Acids Res. 42 (1), 290–306. doi: 10.1093/nar/gkt830
O’Connor, R. M., Allred, D. R. (2000). Selection of Babesia Bovis-Infected Erythrocytes for Adhesion to Endothelial Cells Co-Selects for Altered Variant Erythrocyte Surface Antigen Isoforms. J. Immunol. 164, 2037–2045. doi: 10.4049/jimmunol.164.4.2037
O’Connor, R. M., Lane, T. J., Stroup, S. E., Allred, D. R. (1997). Characterization of a Variant Erythrocyte Surface Antigen (VESA1) Expressed by Babesia Bovis During Antigenic Variation. Mol. Biochem. Parasitol. 89, 259–270. doi: 10.1016/s0166-6851(97)00125-4
O’Connor, R. M., Long, J. A., Allred, D. R. (1999). Cytoadherence of Babesia Bovis-Infected Erythrocytes to Bovine Brain Capillary Endothelial Cells Provides an In Vitro Model for Sequestration. Infect. Immun. 67, 3921–3928. doi: 10.1128/iai.67.8.3921-3928.1999
Ohshima, H., Sawa, T., Akaike, T. (2006). 8-Nitroguanine, A Product of Nitrative DNA Damage Caused by Reactive Nitrogen Species: Formation, Occurrence, and Implications in Inflammation and Carcinogenesis. Antioxid. Redox Signaling 8 (5&6), 1033–1045. doi: 10.1089/ars.2006.8.1033
Pasqualini, R., Ruoslahti, E. (1996). Organ Targeting In Vivo Using Phage Display Peptide Libraries. Nature 380, 364–366. doi: 10.1038/380364a0
Pedroni, M. J., Sondgeroth, K. S., Gallego-Lopez, G. M., Echaide, I., Lau, A. O. T. (2013). Comparative Transcriptome Analysis of Geographically Distinct Virulent and Attenuated Babesia Bovis Strains Reveals Similar Gene Expression Changes Through Attenuation. BMC Genomics 14, 763.761–713. doi: 10.1186/1471-2164-14-763
Radak, Z., Boldogh, I. (2010). 8-Oxo-7,8-Dihydroguanine: Links to Gene Expression, Aging, and Defense Against Oxidative Stress. Free Radical Biol. Med. 49, 587–596. doi: 10.1016/j.freeradbiomed.2010.05.008
Reeder, B. J. (2017). Redox and Peroxidase Activities of the Hemoglobin Superfamily: Relevance to Health and Disease. Antioxid. Redox Signaling 26 (14), 763–776. doi: 10.1089/ars.2016.6803
Rodriguez, R., Miller, K. M., Forment, J. V., Bradshaw, C. R., Nikan, M., Britton, S., et al. (2012). Small-Molecule-Induced DNA Damage Identifies Alternative DNA Structures in Human Genes. Nat. Chem. Biol. 8, 301–310. doi: 10.1038/nchembio.780
Rulten, S. L., Grundy, G. J. (2017). Non-Homologous End Joining: Common Interaction Sites and Exchange of Multiple Factors in the DNA Repair Process. Bioessays 39 (3), 1600209.1-1600209.12. doi:10.1002/bies.201600209
Scheibel, L. W., Ashton, S. H., Trager, W. (1979). Plasmodium Falciparum: Microaerophilic Requirements in Human Red Blood Cells. Exp. Parasitol. 47, 410–418. doi.10.1016/0014-4894(79)90094-8
Siao, M. C., Borner, J., Perkins, S. L., Deitsch, K. W., Kirkman, L. A. (2020). Evolution of Host Specificity by Malaria Parasites Through Altered Mechanisms Controlling Genome Maintenance. mBio 11 (2), e03272–e03219. doi 10.1128/mBio.03272-19
Smith, J. D., Chitnis, C. E., Craig, A. G., Roberts, D. J., Hudson-Taylor, D. E., Peterson, D. S., et al. (1995). Switches in Expression of Plasmodium Falciparum Var Genes Correlate With Changes in Antigenic and Cytoadherent Phenotypes of Infected Erythrocytes. Cell 82, 101–110. doi: 10.1016/0092-8674(95)90056-x
Su, X.-z., Heatwole, V. M., Wertheimer, S. P., Guinet, F., Herrfeldt, J. A., Peterson, D. S., et al. (1995). The Large Diverse Gene Family Var Encodes Proteins Involved in Cytoadherence and Antigenic Variation of Plasmodium Falciparum-Infected Erythrocytes. Cell 82, 89–100doi: 10.1016/0092-8674(95)90055-1.
Sunaga, F., Namikawa, K., Kanno, Y. (2002). Continuous In Vitro Culture of Erythrocytic Stages of Babesia Gibsoni and Virulence of the Cultivated Parasite. J. Vet. Med. Sci. 64, 571–575doi: 10.1292/jvms.64.571.
Symington, L. S., Rothestein, R., Lisby, M. (2014). Mechanisms and Regulation of Mitotic Recombination in Saccharomyces Cerevisiae. Genetics 198, 795–835. doi: 10.1534/genetics.114.166140
Takagi, T., Waki, S. (1987). Requirement of Low Oxygen Tension for Adaptation of a New Isolate of Plasmodium Falciparum to Continuous In Vitro Culture. J. Parasitol. 73 (1), 238–240. doi: 10.2307/3282379
Trager, W., Jensen, J. B. (1976). Human Malaria Parasites in Continuous Culture. Science 193, 673–675. doi: 10.1126/science.781840
Vega, C. A., Buening, G. M., Green, T. J., Carson, C. A. (1985). In Vitro Cultivation of Babesia Bigemina. Am. J. Vet. Res. 46, 416–420.
Wang, X., Bouchut, A., Xiao, Y.-P., Al-Khedery, B., Allred, D. R. (2012). Characterization of the Unusual Bidirectional Ves Promoters Driving VESA1 Expression and Associated With Antigenic Variation in Babesia Bovis. Eukaryot. Cell 11, 260–269. doi: 10.1128/EC.05318-11
Xiao, Y.-P., Al-Khedery, B., Allred, D. R. (2010). The Babesia Bovis VESA1 Virulence Factor Subunit 1b is Encoded by the 1β Branch of the Ves Multigene Family. Mol. Biochem. Parasitol. 171, 81–88. doi: 10.1016/j.molbiopara.2010.03.001
Zhang, X., Alexander, N., Leonardi, I., Mason, C., Kirkman, L. A., Deitsch, K. W. (2019). Rapid Antigen Diversification Through Mitotic Recombination in the Human Malaria Parasite Plasmodium Falciparum. PloS Biol. 17 (5), e3000271. doi: 10.1371/journal.pbio.3000271
Zhang, H., Wang, Z., Huang, J., Cao, J., Zhou, Y., Zhou, J. (2020). A Novel Thioredoxin-Dependent Peroxiredoxin (TPx-Q) Plays an Important Role in Defense Against Oxidative Stress and is a Possible Drug Target in Babesia Microti. Front. Vet. Sci. 7, 76. doi: 10.3389/fvets.2020.00076
Żupańska, A. K., Drummond, P. B., Swetnam, D. M., Al-Khedery, B., Allred, D. R (2009). Universal Primers Suitable to Assess Population Dynamics Reveal Apparent Mutually Exclusive Transcription of the Babesia bovis Ves1α Gene. Mol. Biochem. Parasitol. 166, 47–53. doi: 10.1016/j.molbiopara.2009.02.008
Keywords: antigenic variation, Babesia, cytoadhesion, DNA Repair, immune evasion, oxidative stress
Citation: Allred DR (2022) Integration of DNA Repair, Antigenic Variation, Cytoadhesion, and Chance in Babesia Survival: A Perspective. Front. Cell. Infect. Microbiol. 12:869696. doi: 10.3389/fcimb.2022.869696
Received: 04 February 2022; Accepted: 24 March 2022;
Published: 14 April 2022.
Edited by:
Lan He, Huazhong Agricultural University, ChinaReviewed by:
Hassan Hakimi, Texas A&M University College Station, United StatesMingming Liu, Hubei University of Arts and Science, China
Copyright © 2022 Allred. This is an open-access article distributed under the terms of the Creative Commons Attribution License (CC BY). The use, distribution or reproduction in other forums is permitted, provided the original author(s) and the copyright owner(s) are credited and that the original publication in this journal is cited, in accordance with accepted academic practice. No use, distribution or reproduction is permitted which does not comply with these terms.
*Correspondence: David R. Allred, YWxscmVkZEB1ZmwuZWR1