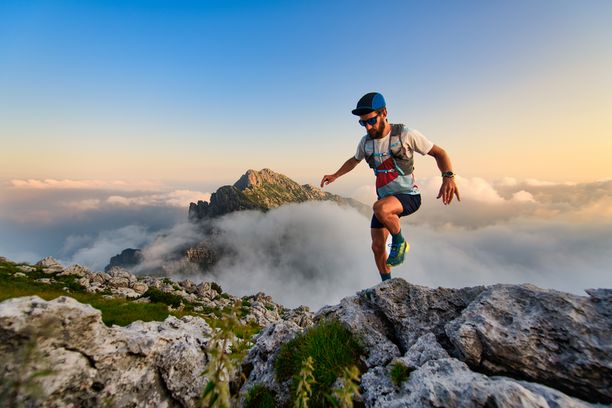
95% of researchers rate our articles as excellent or good
Learn more about the work of our research integrity team to safeguard the quality of each article we publish.
Find out more
ORIGINAL RESEARCH article
Front. Cell. Infect. Microbiol., 28 March 2022
Sec. Bacteria and Host
Volume 12 - 2022 | https://doi.org/10.3389/fcimb.2022.866357
To discover novel microbial pesticide for controlling rice bacterial disease, polymyxin B1 and E2 were firstly isolated from the supernatant of fermentation broth of Paenibacillus polymyxa Y-1 by bioactivity tracking separation. It is shown that polymyxin B1 and E2 had remarkable in vitro inhibitory activities to Xanthomonas oryzae pv. oryzae (Xoo) and Xanthomonas oryzae pv. oryzicola (Xoc) with the EC50 values of 0.19 μg/ml and 0.21 μg/ml against Xoo, and 0.32 μg/ml and 0.41 μg/ml against Xoc, respectively, which were better than those of Zhongshengmycin (0.31 μg/ml and 0.73 μg/ml) and Bismerthiazol (77.48 μg/ml and 85.30 μg/ml). Polymyxins B1 and E2 had good protection and curative activities against rice bacterial leaf blight (BLB) and rice bacterial leaf streak (BLS) in vivo. The protection and curative activities of polymyxins B1 (45.8 and 35.8%, respectively) and E2 (41.2 and 37.0%, respectively) to BLB were superior to those of Zhongshengmycin (34.8 and 29.8%, respectively) and Bismerthiazol (38.0 and 33.5%, respectively). Meanwhile, the protection and curative activities of polymyxins B1 (44.8 and 39.8%, respectively) and E2 (42.9 and 39.9%, respectively) to BLS were also superior to those of Zhongshengmycin (39.7 and 32.0%, respectively) and Bismerthiazol (41.5 and 34.3%, respectively). Polymyxin B1 exerted the anti-pesticide properties via destroying the cell integrity of Xoo, reducing its infectivity and enhancing rice resistance against pathogens through activating the phenylpropanoid biosynthesis pathway of rice. It is indicated that polymyxin B1 and E2 were potential microbial pesticides for controlling rice bacterial disease.
Plant bacterial diseases are caused by a large number of microorganisms, leading to a substantial economic loss of crops worldwide each year. Two important rice bacterial diseases, rice bacterial leaf streak (BLS) and rice bacterial blight (BLB) caused by Xanthomonas oryzae pv. oryzicola (Xoc) and Xanthomonas oryzae pv. oryzae (Xoo), seriously affect the yield and quality of rice. BLB and BLS result in at least 80 and 40% yield loss annually, respectively (Huang et al., 1997; Li et al., 2012; Jiang et al., 2020). The long-term use of traditional bactericides like Bismerthiazol, Zinc thiazole, and Thiodiazole copper causes drug resistance and adverse events on the safety of the environment (Wang et al., 2019). However, microbial pesticide with low toxicity and easy metabolism properties has been a hot research topic in recent years. As a successful microbial pesticide, Zhongshengmycin has been widely used to control plant bacterial diseases, although its control efficacy is relatively low (Wang et al., 2021). Therefore, it is urgent to discover the alternative to conventional antibiotics with high efficacy, environmental friendliness and low toxicity.
Dendrobium nobile (D. nobile) is a medicinal and edible plant native to China. It belongs to the Orchidaceae, and is rich in endophytic bacteria. Paenibacillus polymyxa (P. polymyxa) is one of important endophytic bacteria of D. nobile with various physiological activators. Among them, peptide antibiotics, a kind of main secondary metabolites derived from various species of P. polymyxa, present an acceptable inhibitory effect against pathogens, namely, polymyxin (Landman et al., 2008; Deng et al., 2011; Niu et al., 2013), saltavidin and gavaserin (Pichard et al., 1995), gatavalin (Nakajima et al., 1971), jolipeptin (Ito and Koyama, 1972), and fusaricidin (Kajimura and Kaneda, 1996; Kajimura and Kaneda, 1997). Five polymyxins (polymyxin A to E) have been widely analyzed and applied as antimicrobial agents. Meanwhile, there are some other metabolites produced from P. polymyxa like cytokinins, auxins, chitinase, and hydrolase, which can promote plant growth, improve plant utilization of nutrients, and induce plant systemic resistance (Mavingui and Heulin, 1994; Lebuhn et al., 1997; Pham et al., 1998; Timmusk et al., 1999; Budi et al., 2000). Recently, P. polymyxa was used to control plant pathogens, namely, Xanthomonas campestris and X. axonopodis (Hahm et al., 2012; Mageshwaran et al., 2012). However, anti-plant pathogens metabolites of P. polymyxa have been rarely reported.
Our previous study, an endophytic bacterium of D. nobile living in the Chishui County of Guizhou Province in China, P. polymyxa Y-1 was firstly isolated. Meanwhile, three pairs of fusaricidin-type compounds were isolated from P. polymyxa Y-1 and their antifungal activities against Pestalotiopsis were assessed, and showed the inhibitory effects on energy generation and amino acid biosynthesis of Pestalotiopsis (Yang et al., 2018). In the current study, to further discover the anti-plant bacterial metabolites from P. polymyxa, two polymyxin metabolites of P. polymyxa were isolated by bioactivity tracking separation and their anti-plant bacterial activities were evaluated. The results showed excellent anti-plant bacterial activities of polymyxin B1 and E2. Furthermore, morphological, conductivity, biofilm, exopolysaccharides (EPS) and differentially expressed genes in polymyxin B1 were explored to clarify the potential mechanisms.
P. polymyxa Y-1 was isolated and identified in our previous study (Yang et al., 2018) and cultured at 28°C in potato dextrose broth (PDB) medium and stored at –80°C in Luria-Bertani with 30% (v/v) glycerol. The pathogenic bacterial strain was cultured in 500 ml of PDB at 28°C until bacteria PDB medium has grown into the logarithmic phase. The supernatant was inoculated into 30 L of PDB medium and grown for 14 days until the OD595 reached more than 1.1. The fermentation broth was centrifuged at 8,000 rpm for 30 min at room temperature, and then collected the supernatant for testing the antibacterial activity and isolating the antibacterial metabolites.
The inhibitory effects of fermentation broth and metabolites of P. polymyxa Y-1 against Xoo and Xoc were examined by paper disk method and turbidimetric method, respectively (Dalgaard et al., 1994; Happy, 2017). Then, the protective and curative activities of metabolites to BLS and BLB were measured in potted plants by Schaad’s method (Li et al., 2018). Bismerthiazol (20% wettable powder) and Zhongshengmycin (12% wettable powder) were served as positive controls. The EC50 values and the in vivo curative and protective effect of metabolites were analyzed using SPSS 17.0.
The antibacterial metabolites were isolated from P. polymyxa Y-1 supernatant by bioactivity tracking separation (Figure 1). The supernatant was separated by an Amberlite XAD-16 column eluted with CH3OH−H2O (0:100, 25:75, 50:50, 80:20, and 100:0 in turn, v/v, 400 ml each) and four fractions (Fr.A to Fr.D) were obtained. The Fr.B (4.3 g) was purified with Sephadex LH-20 column eluted with 20, 50, and 80% CH3OH–H2O in turn (50 ml each), and the same segments were enriched and concentrated to afford Fr.B1 to Fr.B3. The Fr.B3 (816.2 mg) was purified with Sephadex LH-20 and Sephadex G-10 column eluted with methanol and H2O for separating the active fractions, respectively. Finally, the active fraction was further purified by HPLC eluted with 30% CH3CN in 0.1% trifluoroacetic acid H2O, and Y1 (65.4 mg) and Y2 (78.2 mg) were obtained. The structures were identified by 1H NMR, 13C NMR, and HRMS.
The Xoo was inoculated into 20 ml of nutrient broth (NB) medium, and cultured to the logarithmic phase (Nollin and Borgers, 1975). The fermentation liquid was centrifuged and Xoo cells in the precipitant were washed, re-suspended in PBS (pH = 7.2) and treated with the active metabolite at 10, 5, and 1 µg/ml for 10 h at 28°C, respectively, with Tween buffer as the negative control. Ten hours later, Xoo cells were washed in PBS and fixed by the 2.5% glutaraldehyde overnight at 4°C. Subsequently, Xoo cells were sequentially dehydrated in 10, 30, 50, 70, 90, and 100% ethanol. Samples were finally freeze-dried, coated, and visualized using Nova Nano SEM 450.
The Xoo was inoculated into 20 ml of NB medium, and cultured to the logarithmic phase. The fermentation liquid was centrifuged and Xoo cells in the precipitant were washed three times with sterile water, and re-suspended in 20 ml of sterile water. Subsequently, Xoo cells were treated with the active metabolite at 10, 5, and 1 µg/ml, respectively, with Tween 20 solution as the negative control. The conductivity at 0, 30, 60, 120, 180, 240, 300, 360, and 420 min were measured, respectively. Finally, the suspension was boiled for 10 min to kill Xoo, followed by detection of the conductivity. The experiment was repeated in triplicate.
Approximately 200 µl of Xoo suspension in the logarithmic phase was added to 2 ml of NB medium. The mixture in NB medium was prepared at the active metabolite concentration of 10, 5, and 1 µg/ml, respectively, which was cultured at 28°C for 5 d. The effects of biofilm formation treated with active metabolite were performed according to the previous method (Pan et al., 2018). Tween 20 solution was used as the negative control. The experiment was repeated in triplicate.
Approximately 150 µl of Xoo suspension in the logarithmic phase was added to 15 ml of NB medium to prepare the mixture containing 10, 5, and 1 µg/ml active metabolites, and cultured for 72 h at 28°C with 180 rpm shaking. The content of EPS was detected by previously reported method (Denny, 1995). Tween 20 solution was used as the negative control. The experiment was repeated in triplicate.
Under greenhouse conditions, Fengyouxiangzhan (seeds were purchased from the Guizhou Seed Management Station) rice was cultivated for 8 weeks, and 200 μg/ml active metabolite solutions were uniformly sprayed onto rice leaves of the treatment group until dripping down, whereas Tween 20 solution was used as control groups. One day after spraying, Xoo in the logarithmic phase was inoculated onto rice leaves with scissors method. Then, the rice plants were cultivated in greenhouse at 28°C and 90% RH. Five days after inoculation, rice leaves were extracted and enzymatically digested for LC–MS/MS (Bin et al., 2020). DEPs were analyzed using the gene ontology (GO) annotation (http://www.geneontology.org/), and the Kyoto Encyclopedia of Genes and Genomes (KEGG) annotation (http://www.genome.jp/Pathway). Then, the databases were searched using the Uniprot software (http://www.uniprot.org/) (Gene, 2004).
The activities of dehydrogenase (CAD) and peroxidase (POD) in rice leaves were determined on the 1st, 3rd, and 5th days after inoculation by the instructions of the manufacturer using commercial enzyme assay reagent kits (Beijing Solarbao Life Sciences, China).
Rice leaves were extracted and enzymatically digested, and then analyzed by LC–MS/MS. Label-free proteomics samples were taken from the same batch of rice leaves. MS data were processed using Skyline (v.3.6). Peptide parameters were set as follows: Trypsin [KR/P]; Max missed cleavage = 0; Peptide length = 7–25 amino acid residues; Fixed modification of cysteine alkylation. Transition parameters were set as follows: Precursor charges = 2, 3; Ion charges = 1, 2; Ion types = b, y, p; Product ions = ion 3 to last ion I; Ion match tolerance = 0.02 Da (Tsuchiya et al., 2013; Yu et al., 2014; Du et al., 2015).
A series of bioassay results showed that the supernatant of fermentation broth from P. polymyxa Y-1 presented remarkable antibacterial activities against Xoo (33.3%) and Xoc (31.5%) (Figure S1). After purification, two antibacterial metabolites coded Y1 and Y2 were isolated for the supernatant. The 1H NMR (600 MHz, D2O) chemical shifts of antibacterial active metabolites Y1, chemical shifts of δ 7.36–7.23 (m, 5H) indicated the existence of aromatic groups. Meanwhile, the chemical shift of δ 4.58–4.39 (m, 5H), 4.34–4.14 (m, 7H) indicated the presence of Hα and Thr-Hβ. The 13C NMR chemical shifts of δ 177.7, 175.1, 173.6, 173.4, 173.1, 173.0, 172.9, 172.4, 171.9, 171.8, and 171.5 indicated the presence of carbonyl carbon, and the chemical shift of δ 135.3, 129.0, 129.0, 128.9, 128.9, and 127.4 confirmed the presence of aromatic groups. HRMS exhibited ion peaks [M + H]+ at m/z 1,203.69739, by 1H NMR, 13C NMR, and HRMS analysis, the compounds were identified as polymyin B1 (calculated for C56H99O13N16, [M + H]+, 1,203.75720). Similarly, the 1H NMR (600 MHz, D2O) chemical shifts of antibacterial active metabolites Y2, δ 4.55–4.50 (m, 3H), 4.41–4.35 (m, 3H), 4.30–4.22 (m, 5H), and 4.18 (d, J = 4.78 Hz, 1H) suggested Hα and Thr-Hβ. The 13C NMR chemical shifts of δ 177.7, 175.0, 174.9, 173.5, 173.1, 172.9, 172.9, 172.4, 172.2, 171.9, and 171.5 represented the carbonyl carbon. HRMS exhibited ion peaks [M + H]+ at m/z 1,155.76685, by 1H NMR, 13C NMR, and HRMS analysis, and the compounds were identified as polymyin E2 (calculated for C52H99O13N16, [M + H]+, 1,155.75720) (Figure 2) (Ikai et al., 1998; Bruch et al., 1999; Pristovsek and Kidric, 1999; Orwa et al., 2001; Cui et al., 2018) (Supplementary Data). The study for the first time reported the structures of polymyins from P. polymyxa Y-1.
The in vitro antibacterial activities of polymyxin B1 and E2 against Xoo and Xoc were evaluated by the turbidimeter method, and the results are listed in Table 1. It is shown that polymyxin B1 and E2 exhibited excellent antibacterial activities (100%) against Xoo and Xoc at the concentration of 200 and 100 μg/ml, respectively, which were similar to Zhongshengmycin, but superior to Bismerthiazol (74.1 and 53.1%, 81.0 and 65.24%, respectively). The antibacterial activities of polymyxin B1 and E2 were confirmed with the EC50 values against Xoo of 0.19 and 0.21 μg/ml, respectively, and 0.32 and 0.41 μg/ml against Xoc, respectively, which were better than those of Zhongshengmycin (0.31 and 0.73 μg/ml) and Bismerthiazol (77.48 and 85.30 μg/mL) against Xoo and Xoc, respectively. It is indicated that polymyxin B1 and E2 can be considered as new antibacterial agents.
As shown in Table 2 and Figure 3, polymyxin B1 (45.8%) and E2 (41.2%) exhibited a better protection activity against BLB than that of Zhongshengmycin (34.8%) and Bismerthiazol (38.0%) under the greenhouse condition at 200 μg/ml. Compared with the curative activity of Zhongshengmycin (29.8%) and Bismerthiazol (33.5%), polymyxin B1 (35.8%) and E2 (37.0%) also showed an excellent curative effect to BLB. In addition, polymyxin B1 (44.8 and 39.8%) and E2 (42.9 and 39.9%) also exhibited excellent protection and curative activities against BLS under the greenhouse condition at 200 μg/ml, which were better than those of Zhongshengmycin (39.7 and 32.0%) and Bismerthiazol (41.50 and 34.3%) (Table 3 and Figure 4). The results indicated that polymyxin B1 and E2 significantly reduced the occurrence of BLB and BLS diseases.
Figure 3 Protection activity and curative activity of polymyxin B1 and E2 against BLB under greenhouse conditions at 200 μg/ml.
Figure 4 Protection activity and curative activity of polymyxin B1 and E2 against BLS under greenhouse conditions at 200 μg/ml.
The effects of polymyxin B1 and E2 on Xoo cell membranes were examined by SEM. As shown in Figure 5, the thallus of the CK groups was plump and uniform on the surface (Figure 5A). However, the morphologies of Xoo have been deformed and wrinkled (Figures 5B, E) after treatment with polymyxin B1 and E2 at 1 μg/ml, respectively. Meanwhile, the morphologies of Xoo were transformed corrugated structure, and partially broken state at 5 μg/ml (Figures 5C, F). A pore-like shape on the bacterial surface and a lot of fragmentized bacteria were observed at the concentration of polymyxin B1 and E2 of 10 μg/ml (Figures 5D, G). SEM results confirmed that polymyxin B1 and E2 could act on cell membranes and damage the cell integrity of Xoo.
Figure 5 Cell surface morphologies of Xoo treated with polymyxin B1 and E2. (A) CK, (B) Xoo cells treated with polymyxin B1 (1 μg/mL), (C) Xoo cells treated with polymyxin B1 (5 μg/mL), (D) Xoo cells treated with polymyxin B1 (10 μg/mL), (E) Xoo cells treated with polymyxin E2 (1 μg/mL), (F) Xoo cells treated with polymyxin E2 (5 μg/mL), (G) Xoo cells treated with polymyxin E2 (10 μg/mL).
Conductivity was determined to reveal the membrane permeability. As shown in Figure 6A, increased effects of polymyxin B1 and E2 on the cell membrane permeability of Xoo with time and concentration were observed. There were significant differences in the treatment concentrations. The relative permeability of polymyxin B1 and E2 were 23.7 and 22.4% at 10 μg/ml for 30 min, which were 12.4 and 13.1% at 5 μg/ml, and 2.1 and 1.3% at 1 μg/ml, respectively. All of them were significantly higher than those of CK groups (0.4%). In addition, the relative permeability of the bacterial liquid increased with time. Notably, the conductivity rapidly increased within 60 min, which slowly increased with the treatment of polymyxin B1 and E2. Among them, the relative permeability of the bacterial solution treated with polymyxin B1 and E2 were much higher than that of the CK group. Seriously damaged cell membrane might be the main reason for the antibacterial effect of polymyxin B1 and E2.
Figure 6 The effects of polymyxin B1 and E2 on relative conductivity (A), biofilm formation (B), and EPS (C) to Xoo.
The effect of the agent on cell biofilm was determined by crystal violet staining. As shown in Figure 6B, the biofilm formation was inhibited after treatment with polymyxin B1 and E2. The inhibition ratios of polymyxin B1 and E2 at 10, 5, and 1 μg/ml were 87.56, 47.28, 39.26% and 87.37, 62.78, 31.18%, respectively, which were superior to CK groups. It can be seen that polymyxin B1 and E2 might act on Xoo biofilm and the effect was positively correlated with the concentration.
The effects of polymyxin B1 and E2 on EPS were determined and the results were shown in Figure 6C. Polymyxin B1 and E2 at 10 μg/ml significantly inhibited the production of EPS. Meanwhile, the inhibition ratios of polymyxin B1 and E2 at 5 μg/ml were 55.93 and 70.42%, respectively, which were 28.19 and 29.34% at 1 μg/ml. It is shown that polymyxin B1 and E2 had significant inhibitory effects on the production of EPS from Xoo and then decreased the pathogenicity of Xoo.
A total of 2,772 proteins were identified in both polymyxin B1 + Xoo (PB) groups and CK + Xoo (CK) groups, which are shown in the Supplementary LC–MS data. There were 2,706 and 2,569 proteins identified in PB and CK groups, respectively, including 203 (7.32%) and 66 (2.38%) specifically expressed proteins, respectively (Figure 7A). A total of 460 proteins were DEPs, including 251 (54.57%) upregulated proteins and 209 (45.43%) downregulated ones (Figure 7B). GO analysis results showed that biological processes were mainly enriched in “cellular process”, “metabolic process”, “response to stimulus”, and “biological regulation”. Cellular component only enriched in “cell”, “intracellular”, and “protein-containing complex” (Figure 7C). Molecular functions were mostly enriched in “catalytic activity” and “binding”. It is demonstrated that polymyxin B1 could affect rice proteome in many aspects of plant physiology, namely, induced resistance, metabolic process, and cellular process.
Figure 7 DEPs. (A) Venn diagram for proteins identified in the CK + Xoo (CK) and PB1 + Xoo (PB) groups, (B) Differential protein volcano map (|log2 foldchange| >1, P <0.05), (C) GO annotation and enrichment analysis of the DEPs between PB1 + Xoo (PB) and CK + Xoo (CK) groups, (D) KEGG analysis of the DEPs between PB1 + Xoo (PB) and CK + Xoo (CK) groups.
KEGG analysis results showed that a total of 12 pathways were enriched (Figure 7D), namely, the phenylpropanoid biosynthesis, MAPK signaling pathway, fatty acid degradation, tryptophan metabolism, lysine degradation, and others. Among them, 16 DEPs was enriched in the phenylpropane biosynthetic pathway, namely, trans-cinnamate-4-monooxygenase (CYP73A), β-glucosidase (GLU), CAD, and POD. There were 9 upregulated proteins (A0A0N7KI36, A0A0P0XR31, Q5JMS4, Q94DM2, A2YPX2, Q6ZCC2, Q6K4J4, Q7XSU7, and Q7XSU8) and 2 downregulated ones (A0A0P0V2C2, Q9AS12) involved in the regulation of POD. Meanwhile, CAD (Q8H859, Q6ERW9, and Q6ERW7) and GLU (Q60DX8) proteins were upregulated, but CYP73A (A2Y375) was downregulated (Figure 8). It is well-known that POD and CAD are able to regulate the formation of lignin (Hrubcová et al., 2000; Fan et al., 2009; Sui et al., 2019), which can provide structural support, prevent pathogen invasion through a physical barrier and act as defensive components in plants (Weng and Chapple, 2010; Aehle et al., 2011; Labeeuw et al., 2015). Upregulation of CAD and POD can increase the resistance of plants to invaded pathogens and reduce the infection incidence (Chen et al., 2019; Yadav et al., 2020). The results indicated that polymyxin B1 could enhance the resistance of rice to Xoo pathogen through upregulating CAD and POD in rice.
Figure 8 DEPs related to phenylpropanoid biosynthesis in rice leaf. The log2 fold change of genes is indicated as a gradient between red (upregulated) and green (downregulated). (1≤ log2 fold change ≤−1, P < 0.05).
As shown in Figure 9A, there was a significant difference of CAD activity between CK + PB and CK groups on the 3rd and 5th days. Meanwhile, CK + PB groups improved 25.8% of CAD activity than that of CK groups on the 5th day. However, there was no significant difference between Xoo + PB and Xoo groups on the 1st, 3rd, and 5th days. As shown in Figure 9B, the enzyme activity of POD significantly increased after treatment with polymyxin B1, yielding the maximum on the 5th day. In detail, CK + PB treatment improved 46.5% of POD activity that of CK treatment. In contrast, the significant difference in POD activity between Xoo + PB and Xoo groups was revealed on the 3rd day at 42.5%. The results showed that polymyxin B1 could increase the defensive enzyme activity and then activate the systemic acquired resistance of rice, and inhibit Xoo infection.
Figure 9 Effects of polymyxin B1 (PB) on CAD (A) and POD (B)k activities in rice leaves. Data presented are averages of pooled data (n = 3). Bars represent standard deviations of the means.
PRM was performed to confirm the potential relationship of 16 DEPs to the phenylpropanoid biosynthesis pathway. As shown in Table 4 and Figure 10, the expression levels of 16 DEPs were consistent with proteomic analysis results. Three POD proteins were significantly upregulated, namely, Q94DM2, Q6ZCC2, and Q7XSU7. Notably, the expression level of Q7XSU7 increased 17.64-fold after Xoo + PB treatment than that of Xoo group (PB/CK). Meanwhile, the PB/CK ratio of Q94DM2 and Q6ZCC2 protein was 9.89 and 8.34, respectively. Expression levels of Q8H859 and Q6ERW9 of CAD upregulated 2.34-fold and 2.19-fold versus CK groups. Among DEPs of phenylpropanoid biosynthesis pathway, A0A0P0V2C2, Q9AS12, and A2Y375 were downregulated. The PRM results demonstrated that polymyxin B1 could significantly affect the expression level of POD and then regulate the phenylpropanoid biosynthesis pathway, which were consistent with DEPs analysis.
Figure 10 The fragment ion peak area distribution map of unique peptides of some POD and CAD. PB is the Xoo + polymyxin B1 group and CK is the Xoo group of rice.
In conclusion, polymyxin B1 and E2 were firstly isolated from P polymyxa Y-1 and later were evaluated on their antibacterial activities in vitro and in vivo. Notably, polymyxin B1 and E2 possessed outstanding inhibitory activities against Xoo and Xoc through destroying the cell integrity of Xoo, reducing its infectivity and enhancing rice resistance to defense pathogen infections. This study implied that polymyxin B1 and E2 can serve as new microbial pesticides for controlling rice bacterial disease and provide an eco-friendly method for developing new pesticides.
The original contributions presented in the study are included in the article/Supplementary Material. Further inquiries can be directed to the corresponding author.
XG and WY conceived and designed the research. XG and WY wrote the manuscript. CC analyzed the data. All authors listed have made a substantial, direct, and intellectual contribution to the work and approved it for publication.
This work was supported by the National Key Research and Development Program of China (2018YFD0200100), the Construction Project of Key Laboratories from the Education Department of Guizhou Province (QJHKY[2018]001), the Subsidy Project for Outstanding Key Laboratory of Guizhou Province in China (20154004), and the Program of Introducing Talents of Discipline to Universities of China (111 Program, D20023).
The authors declare that the research was conducted in the absence of any commercial or financial relationships that could be construed as a potential conflict of interest.
All claims expressed in this article are solely those of the authors and do not necessarily represent those of their affiliated organizations, or those of the publisher, the editors and the reviewers. Any product that may be evaluated in this article, or claim that may be made by its manufacturer, is not guaranteed or endorsed by the publisher.
The Supplementary Material for this article can be found online at: https://www.frontiersin.org/articles/10.3389/fcimb.2022.866357/full#supplementary-material
Supplementary data includes 13C NMR, 1H NMR and HRMS of polymyxin B1 and E2. Antibacterial activity assay of P. polymyxa supernatant. LC-MS/MS identified 4431 proteins, which were shown in Appendix LC-MS data.
Aehle, E., Müller, U., Eklund, P. C., Willför, S. M., Sippl, W., Dräger, B. (2011). Lignans as Food Constituents With Estrogen and Antiestrogen Activity. Phytochemistry 72, 2396–2405. doi: 10.1016/j.phytochem.2011.08.013
Bin, T., Ying, Z., Chen, Z. (2020). Quantitative Analysis of Allelic Differences in the Grain Proteome Between the Wxg2 and Wxg3 Alleles in Rice (Oryza Sativa L.). Euphytica 216, 150. doi: 10.1007/s10681-020-02685-9
Bruch, M. D., Cajal, Y., Koh, J. T., Jain, M. K. (1999). Higher-Order Structure of Polymyxin B: The Functional Significance of Topological Flexibility. J. Am. Chem. Soc. 121, 11993–12004. doi: 10.1021/ja992376m
Budi, S. W., Van, T. D., Arnould, C., Dumas, G. E., Gianinazzi, P. V., Gianinazzi, S. (2000). Hydrolytic Enzyme Activity of Paenibacillus Sp. Strain B2 and Effects of the Antagonistic Bacterium on Cell Integrity of Two Soil-Borne Pathogenic Fungi. Appl. Soil Ecol. 15, 191–199. doi: 10.1016/S0929-1393(00)00095-0
Chen, C., Cai, N., Chen, J., Wan, C. (2019). Clove Essential Oil as an Alternative Approach to Control Postharvest Blue Mold Caused by Penicillium Italicum in Citrus Fruit. Biomolecules 9, 197. doi: 10.3390/biom9050197
Cui, A. L., Hu, X. X., Gao, Y., Jin, J., Yi, H., Wang, X. K., et al. (2018). Synthesis and Bioactivity Investigation of the Individual Components of Cyclic Lipopeptide Antibiotics. J. Med. Chem. 61, 1845–1857. doi: 10.1021/acs.jmedchem.7b01367
Dalgaard, P., Ross, T., Kamperman, L., Neumeyer, K., McMeekin, T. A. (1994). Estimation of Bacterial Growth Rates From Turbidimetric and Viable Count Data. Int. J. Food. Microbiol. 23, 391–404. doi: 10.1016/0168-1605(94)90165-1
Deng, Y., Lu, Z., Bi, H., Lu, F., Zhang, C., Bie, X. (2011). Isolation and Characterization of Peptide Antibiotics LI-F04 and Polymyxin B6 Produced by Paenibacillus Polymyxa Strain JSa-9. Peptides 32, 1917–1923. doi: 10.1016/j.peptides.2011.08.004
Denny, T. P. (1995). Involvement of Bacterial Polysaccharide in Plant Pathogenesis. Annu. Rev. Phytopathol. 33, 173–197. doi: 10.1146/annurev.py.33.090195.001133
Du, C., Liu, H. F., Lin, Y. Z., Wang, X. F., Ma, J., Li, Y. J., et al. (2015). Proteomic Alteration of Equine Monocyte-Derived Macrophages Infected With Equine Infectious Anemia Virus. Proteomics 15, 1843–1858. doi: 10.1002/pmic.201400279
Fan, L., Shi, W. J., Hu, W. R., Hao, X. Y., Wang, D. M., Yuan, H., et al. (2009). Molecular and Biochemical Evidence for Phenylpropanoid Synthesis and Presence of Wall-Linked Phenolics in Cotton Fibers. J. Integr. Plant Biol. 51, 626–637. doi: 10.1111/j.1744-7909.2009.00840.x
Gene, O. C. (2004). The Gene Ontology (GO) Database and Informatics Resource. Nucleic Acids Res. 32, D258–D261. doi: 10.1093/nar/gkh036
Hahm, M. S., Sumayo, M., Hwang, Y. J., Jeon, S. A., Park, S. J., Lee, J. Y., et al. (2012). Biological Control and Plant Growth Promoting Capacity of Rhizobacteria on Pepper Under Greenhouse and Field Conditions. J. Microbiol. 50, 380–385. doi: 10.1007/s12275-012-1477-y
Happy, N. (2017). Antibacterial Activity of Metabolites Products of Vibrio Alginolyticus Isolated From Sponge Haliclona Sp. Against Staphylococcus Aureus. Ital. J. Food. Saf. 6, 18–22. doi: 10.4081/ijfs.2017.6237
Hrubcová, M., Cvikrová, M., Eder, J., Zoń, J., Macháčková, I. (2000). Effect of Inhibition of Phenylpropanoid Biosynthesison Peroxidase and IAA-Oxidase Activities and Auxin Contentin Alfalfa Suspension Cultures. Plant Physiol. Biochem. 38, 949–956. doi: 10.1016/S0981-9428(00)01208-0
Huang, N., Angeles, E. R., Domingo, J., Magpantay, G., Singh, S., Zhang, G., et al. (1997). Pyramiding of Bacterial Blight Resistance Genes in Rice: Marker-Assisted Selection Using RFLP and PCR. Theor. Appl. Genet. 95, 313–320. doi: 10.1007/s001220050565
Ikai, Y., Oka, H., Hayakawa, J., Kawamura, N., Harada, K., Suzuki, M., et al. (1998). Isolation of Colistin A and B Using High-Speed Countercurrent Chromatography. J. Liq. Chrom. Rel. Technol. 21, 143–155. doi: 10.1080/10826079808001943
Ito, M., Koyama, Y. (1972). Jolipeptin, a New Peptide Antibiotic. II. @ the Mode of Action of Jolipeptin. J. Antibiot. 25, 309–314. doi: 10.7164/antibiotics.25.309
Jiang, N., Yan, J., Liang, Y., Shi, Y. L., He, Z. Z., Wu, Y. T., et al. (2020). Resistance Genes and Their Interactions With Bacterial Blight/Leaf Streak Pathogens (Xanthomonas Oryzae) in Rice (Oryza Sativa L.)-An Updated Review. Rice 13, 1–12. doi: 10.1186/s12284-019-0358-y
Kajimura, Y., Kaneda, M. (1996). Fusaricidin A, a New Depsipeptide Antibiotic Produced by Bacillus Polymyxa KT-8 Taxonomy, Cultivation, Isolation, Structure Elucidation and Biological Activity. J. Antibiot. 49, 129–135. doi: 10.7164/antibiotics.49.129
Kajimura, Y., Kaneda, M. (1997). Fusaricidins B, C and D, New Depsipeptide Antibuotics Produced by Bacillus Polymyxa KT-8: Isolation, Structure Elucidation and Biological Activity. J. Antibiot. 50, 220–228. doi: 10.7164/antibiotics.50.220
Labeeuw, L., Martone, P. T., Boucher, Y., Case, R. J. (2015). Ancient Origin of the Biosynthesis of Lignin Precursors. Biol. Direct. 10, 23. doi: 10.1186/s13062-015-0052-y
Landman, D., Georgescu, C., Martin, D. A., Quale, J. (2008). Polymyxins Revisited. Clin. Microbiol. Rev. 21, 449–465. doi: 10.1128/cmr.00006-08
Lebuhn, M., Heulin, T., Hartmann, A. (1997). Production of Auxin and Other Indolic and Phenolic Compounds by Paenibacillus Polymyxa Strains Isolated From Different Proximity to Plant Roots. FEMS Microbiol Ecol 22, 325–334. doi: 10.1111/j.1574-6941.1997.tb00384.x
Li, P., Hu, D. Y., Xie, D. D., Chen, J. X., Jin, L. H., Song, B. A. (2018). Design, Synthesis, and Evaluation of New Sulfone Derivatives Containing a 1, 3, 4-Oxadiazole Moiety as Active Antibacterial Agents. J. Agric. Food Chem. 66, 3093–3100. doi: 10.1021/acs.jafc.7b06061
Li, D. X., Wang, L. J., Teng, S. L., Zhang, G. G., Guo, L. J., Mao, Q., et al. (2012). Proteomics Analysis of Rice Proteins Up-Regulated in Response to Bacterial Leaf Streak Disease. J. Plant Biol. 55, 316–324. doi: 10.1007/s12374-011-0346-2
Mageshwaran, V., Walia, S., anf Annapurna, K. (2012). Isolation and Partial Characterization of Antibacterial Lipopeptide Produced by Paenibacillus Polymyxa HKA-15 Against Phytopathogen Xanthomonas Campestris Pv. Phaseoli M-5. World J. Microb. Biot. 28, 909–917. doi: 10.1007/s11274-011-0888-y
Mavingui, P., Heulin, T. (1994). In Vitro Chitinase and Antifungal Activity of a Soil, Rhizosphere and Rhizoplane Population of Bacillus Polymyxa. Soil. Biol. Biochem. 26, 801–803. doi: 10.1016/0038-0717(94)90277-1
Nakajima, N., Chihara, S., Koyama, Y. (1971). A New Antibiotic, Gatavalin I. Isolation and Characterization. J. Antibiot. 25, 243–247. doi: 10.1111/j.1559-3584.1927.tb04229.x
Niu, B., Vater, J., Rueckert, C., Blom, J., Lehmann, M., Ru, J. J., et al. (2013). Polymyxin P is the Active Principle in Suppressing Phytopathogenic Erwinia Spp. By the Biocontrol Rhizobacterium Paenibacillus Polymyxa M-1. BMC Microbiol. 13, 1–13. doi: 10.1186/1471-2180-13-137
Nollin, S., Borgers, M. (1975). Scanning Electron Microscopy of Candida Albicans After In Vitro Treatment With Miconazole. Antimicrob. Agents Chemother. 7, 704–711. doi: 10.1128/aac.7.5.704
Orwa, J. A., Govaerts, C., Busson, R., Roets, E., Schepdae, A. V., Hoogmartens, J. (2001). Isolation and Structural Characterization of Polymyxin B Components. J. Chromatogr. A. 912, 369–373. doi: 10.1016/s0021-9673(01)00585-4
Pan, X. Y., Xu, S., Wu, J., Luo, J. Y., Duan, Y. B., Wang, J. X., et al. (2018). Screening and Characterization of Xanthomonas Oryzae Pv. Oryzae Strains With Resistance to Pheazine-1-Carboxylic Acid. Pestic. Biochem. Phys. 145, 8–14. doi: 10.1016/j.pestbp.2017.12.003
Pham, P. L., Taillandier, P., Delmas, M., Strehaiano, P. (1998). Production of Xylanases by Bacillus Polymyxa Using Lignocellulosic Wastes. Ind 7, 195–203. doi: 10.1016/S0926-6690(97)00048-4
Pichard, B., Larue, J. P., Thouvenot, D. (1995). Gavaserin and Saltavalin, New Peptide Antibiotics Produced by Bacillus Polymyxa. FEMS. Microbiol. Lett. 133, 215–218. doi: 10.1111/j.1574-6968.1995.tb07887.x
Pristovsek, P., Kidric, J. (1999). Solution Structure of Polymyxins B and E and Effect of Binding to Lipopolysaccharide: An NMR and Molecular Modeling Study. J. Med. Chem. 42, 4604–4613. doi: 10.1021/jm991031b
Sui, J. J., Qu, C. Q., Yang, J. X., Zhang, W. N., Ji, Y. T. (2019). Transcriptome Changes in the Phenylpropanoid Pathway in Senescing Leaves of Toona Sinensis. Acta Physiol. Plant 41, 1–15. doi: 10.1007/s11738-019-2915-9
Timmusk, S., Nicander, B., Granhall, U., Tillberg, E. (1999). Cytokinin Production by Paenibacillus Polymyxa. Biol. Biochem. 31, 1847–1852. doi: 10.1016/S0038-0717(99)00113-3
Tsuchiya, H., Tanaka, K., Saeki, Y. (2013). The Parallel Reaction Monitoring Method Contributes to a Highly Sensitive Polyubiquitin Chain Quantification. Biochem. Biophys. Res. Commun. 436, 223–229. doi: 10.1016/j.bbrc.2013.05.080
Wang, S. B., Gan, X. H., Wang, Y. J., Li, S. Y., Yi, C. F., Chen, J. X., et al. (2019). Novel 1,3,4-Oxadiazole Derivatives Containing a Cinnamic Acid Moiety as Potential Bactericide for Rice Bacterial Diseases. Int. J. Mol. Sci. 20, 1–17. doi: 10.3390/ijms20051020
Wang, Q. P., Zhang, C., Long, Y. H., Wu, X. M., Su, Y., Lei, Y., et al. (2021). Bioactivity and Control Efficacy of the Novel Antibiotic Tetramycin Against Various Kiwifruit Diseases. Antibiotics 10, 289. doi: 10.3390/antibiotics10030289
Weng, J. K., Chapple, C. (2010). The Origin and Evolution of Lignin Biosynthesis. New Phytol. 187, 273–285. doi: 10.1111/j.1469-8137.2010.03327.x
Yadav, V., Wang, Z., Wei, C., Amo, A., Ahmed, B., Yang, X., et al. (2020). Phenylpropanoid Pathway Engineering: An Emerging Approach Towards Plant Defense. Pathogens 9, 1–25. doi: 10.3390/pathogens9040312
Yang, A. M., Zeng, S., Yu, L., He, M., Yang, Y. Y., Zhao, X. Z., et al. (2018). Characterization and Antifungal Activity Against Pestalotiopsis of a Fusaricidin-Type Compound Produced by Paenibacillus Polymyxa Y-1. Pestic. Biochem. Physiol. 147, 67–74. doi: 10.1016/j.pestbp.2017.08.012
Keywords: Paenibacillus polymyxa Y-1, polymyxin, rice bacterial blight, antibacterial activity, phenylpropanoid biosynthesis
Citation: Yi W, Chen C and Gan X (2022) Polymyxin B1 and E2 From Paenibacillus polymyxa Y-1 for Controlling Rice Bacterial Disease. Front. Cell. Infect. Microbiol. 12:866357. doi: 10.3389/fcimb.2022.866357
Received: 09 February 2022; Accepted: 22 February 2022;
Published: 28 March 2022.
Edited by:
Pei Li, Kaili University, ChinaReviewed by:
Song Bai, Guizhou Institute of Technology, ChinaCopyright © 2022 Yi, Chen and Gan. This is an open-access article distributed under the terms of the Creative Commons Attribution License (CC BY). The use, distribution or reproduction in other forums is permitted, provided the original author(s) and the copyright owner(s) are credited and that the original publication in this journal is cited, in accordance with accepted academic practice. No use, distribution or reproduction is permitted which does not comply with these terms.
*Correspondence: Xiuhai Gan, Z3hoMjAwNzE5QDE2My5jb20=
Disclaimer: All claims expressed in this article are solely those of the authors and do not necessarily represent those of their affiliated organizations, or those of the publisher, the editors and the reviewers. Any product that may be evaluated in this article or claim that may be made by its manufacturer is not guaranteed or endorsed by the publisher.
Research integrity at Frontiers
Learn more about the work of our research integrity team to safeguard the quality of each article we publish.