- 1Department of Parasitology and Medical Entomology, Faculty of Medical Laboratory Sciences, University of Khartoum, Khartoum, Sudan
- 2Department of Pharmacology and Toxicology, Indiana University School of Medicine, Indianapolis, IN, United States
- 3The Kuvin Center for the Study of Infectious and Tropical Diseases, Department of Microbiology and Molecular Genetics, Institute for Medical Research Israel-Canada, Faculty of Medicine, Hebrew University of Jerusalem, Jerusalem, Israel
The deadly malaria parasite, Plasmodium falciparum, contains a unique subcellular organelle termed the apicoplast, which is a clinically-proven antimalarial drug target. The apicoplast is a plastid with essential metabolic functions that evolved via secondary endosymbiosis. As an ancient endosymbiont, the apicoplast retained its own genome and it must be inherited by daughter cells during cell division. During the asexual replication of P. falciparum inside human red blood cells, both the parasite, and the apicoplast inside it, undergo massive morphological changes, including DNA replication and division. The apicoplast is an integral part of the cell and thus its development is tightly synchronized with the cell cycle. At the same time, certain aspects of its dynamics are independent of nuclear division, representing a degree of autonomy in organelle biogenesis. Here, we review the different aspects of organelle dynamics during P. falciparum intraerythrocytic replication, summarize our current understanding of these processes, and describe the many open questions in this area of parasite basic cell biology.
Introduction
The Study of Fundamental Cell Biology of Malaria Parasites
Malaria is a worldwide leading cause of morbidity and mortality, infecting predominantly people in tropical and sub-tropical regions. In 2020, WHO estimated 250 million malaria cases and reported about 627000 deaths preponderantly in sub-Saharan African countries (World Health Organization, 2021). Malaria is caused by eukaryotic parasites of the genus Plasmodium, which are transmitted by female Anopheles mosquitoes (Garrido-Cardenas et al., 2019). It is primarily one species, P. falciparum, that is responsible for most of the mortality (World Health Organization, 2021). As yet, there are no effective vaccines and the parasite gained resistance to all clinically available antimalarial drugs, jeopardizing the progress that has been made in the last decade (Dondorp et al., 2009; Phillips et al., 2017; World Health Organization, 2019). Since the genome sequencing of P. falciparum in 2002 (Gardner et al., 2002), various surveys on population genetics, genomics, transcriptomics and proteomics brought into view the potential of targeting parasite-specific molecular pathways in eliminating malaria (Winzeler, 2008; Su et al., 2019). These strategies rely heavily on advanced techniques in cell biology and molecular genetics including various conditional-knockdown methods and the application of CRISPR/Cas9 genome engineering (Ghorbal et al., 2014; Wagner et al., 2014) [extensively reviewed in (Kudyba et al., 2021)]. Furthermore, a recently developed microscopy technique named ultrastructure expansion microscopy, allows the visualization of preserved expanded organelles with a 4-fold isotropic size increase, giving the resolution needed to monitor organelle dynamics of the microscopic malaria parasite during its replication (Bertiaux et al., 2021; Liffner and Absalon, 2021; Tomasina et al., 2021). Additional advanced microscopy techniques such as lattice light-sheet microscopy (LLSM) provide high-resolution in time and space that was used to determine the kinetics of parasite invasion into the erythrocyte and revealed detailed events in membrane remodeling (Geoghegan et al., 2021). Finally, some of the most advanced techniques in electron microscopy, for example Focused Ion Beam-Scanning Electron Microscopy (FIB-SEM), are used to gain the ultrastructure of nuclear division and subcellular organelle organization (Medeiros et al., 2012; Rudlaff et al., 2020). In this mini review, we describe P. falciparum mode of division in erythrocytes with a focus on a specialized plastid organelle named the apicoplast. This parasite-specific organelle, which is clinically targeted by various antimalarials, is essential for parasite viability due to its metabolic functions. Here we describe various open questions related to apicoplast biogenesis and discuss future research directions to examine the autonomous nature of apicoplast growth, fission, and segregation during Plasmodium cell division.
Plasmodium’s Cell Cycle and Division
The asexual replication of P. falciparum inside the red blood cell (RBC) begins with invasion by a single parasite into the host RBC and culminates 48 hours later in the egress of roughly 30 new daughter parasites (Garg et al., 2015). During this life cycle, the parasite grows and at a certain point begins a unique form of cell division called schizogony (Striepen et al., 2007; Francia and Striepen, 2014; Gubbels et al., 2021) (Figure 1). In this process, the parasite replicates its DNA and then follows with nuclear division inside an intact nuclear envelope to produce two nuclei (Absalon, 2020). This process is repeated multiple times asynchronously and produces a multi-nucleated cell (Gerald et al., 2011). Schizogony concludes in a single cytokinesis event, during which the multi-nucleated cell segments into ~30 daughter cells called merozoites that will egress and invade new host RBCs. This particular type of segmentation involves a membranous structure called the inner membrane complex (IMC) (Dearnley et al., 2012; Harding and Meissner, 2014). The IMC is a double lipid bilayer formed from a patchwork of flattened membrane vesicles that, together with associated proteins, lies closely underneath the parasite plasma membrane (Morrissette and Sibley, 2002; Kono et al., 2013). Beneath the IMC lies a network of alveolins—intermediate filament-like proteins that provide support to the IMC, and are common to all protists in the infrakingdom Alveolata, to which Plasmodium and other apicomplexan parasites belong (Khater et al., 2004; Gould et al., 2008; Al-Khattaf et al., 2014; Tremp et al., 2014). The IMC is involved in many essential parasite-specific functions including host cell invasion by anchoring many of the glideosome proteins required for actinomyosin-based gliding motility (Bergman et al., 2003; Keeley and Soldati, 2004; Baum et al., 2006; Frénal et al., 2010). Studies in the related parasite Toxoplasma gondii showed that the IMC defines the shape and structural stability of the parasite and is essential for parasite cell division (Mann and Beckers, 2001; Hu et al., 2002; Beck et al., 2010). In P. falciparum, it was shown that the IMC is associated with proteins inside the parasite and dictates the shape and rigidity of nascent merozoites, however, its biogenesis and mechanisms of action remain poorly understood (Absalon et al., 2016). Notably, it remains elusive how asynchronous nuclear replication coincides with a single event of segmentation where sub-cellular content must be equally distributed within daughter cells. The partitioning and distribution of organelles such as the endoplasmic reticulum, the Golgi apparatus and parasite-specific secretory organelles, are coordinated with segmentation (van Dooren et al., 2005). The fact that all these membranous compartments can be synthesized de novo, suggests some flexibility in their distribution to daughter cells which, in theory, can replenish the secretory pathway using inherited material as well as de novo synthesis. The case is different however for the parasite’s two endosymbiotic organelles, the mitochondrion and the apicoplast, which carry their own ancestral genomes. Unlike many other eukaryotic cells, the parasite carries only a single copy of each organelle that must be inherited accurately during cell division. As will be discussed below, the apicoplast organelle undergoes drastic morphological changes culminating in its division and sorting, while being coordinated with the different phases of schizogony.
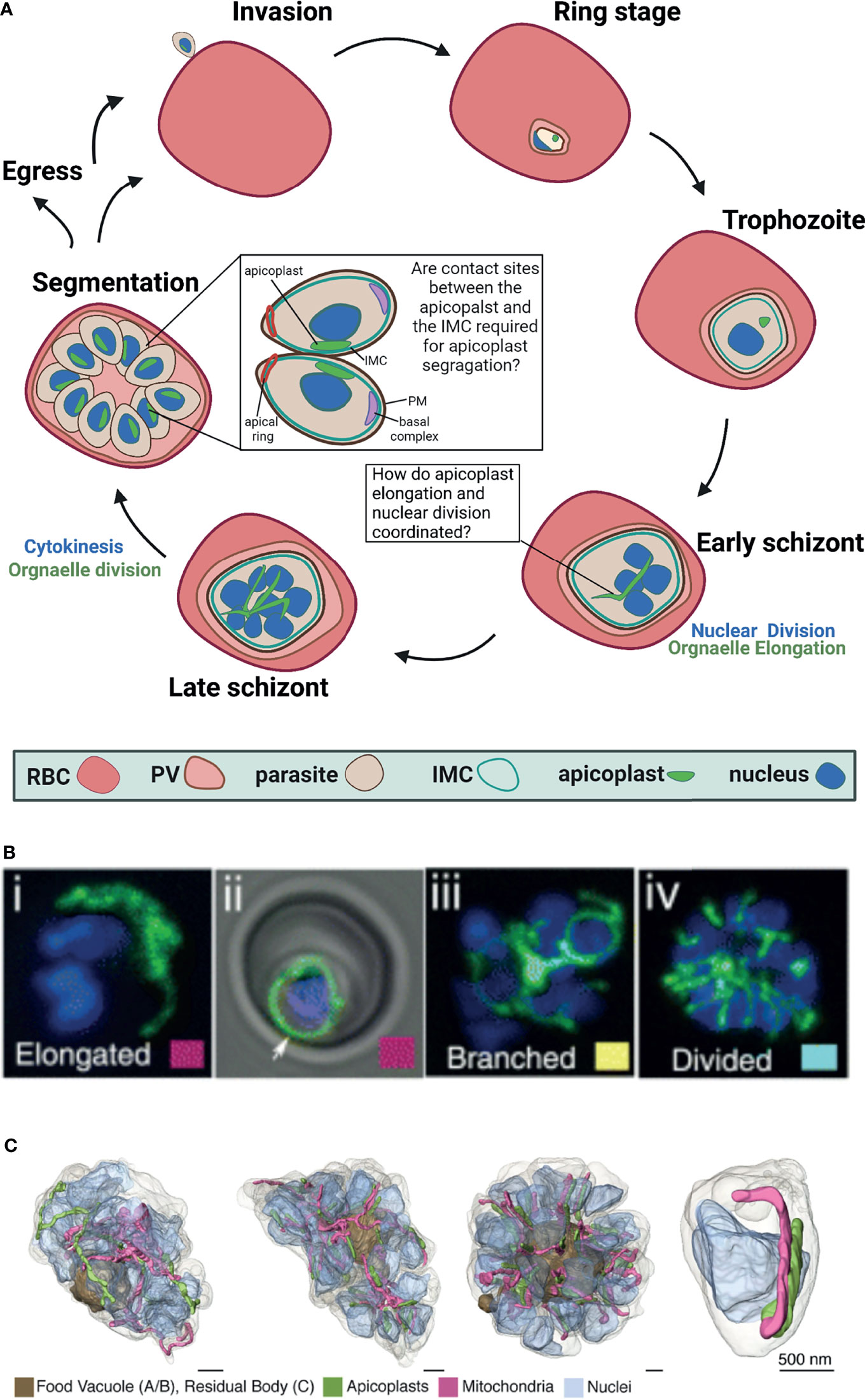
Figure 1 Apicoplast dynamics during intraerythrocytic development of Plasmodium falciparum. (A) Schematic representation of apicoplast dynamics during intraerythrocytic asexual replication of P. falciparum. RBC, red blood cell; PV, parasitophorous vacuole; IMC, inner membrane complex, PM, plasma membrane Created with BioRender.com. (B) Fluorescent microcopy of live apicoplast-tagged parasites (ACP-DsRed) co-labelled with a nuclear Hoechst dye. Apicoplast morphology was categorized into four groups based on developmental stage: (i) rounded (ii) elongated (iii) branched and (iv) divided. Image taken from van Dooren et al. (2005). (C). Apicoplast morphologies throughout segmentation. By mid-segmentation, apicoplasts have divided to form one organelle for each nascent daughter cell. Ultra-resolution images were obtained using FIB-SEM, taken from Rudlaff et al. (2020).
The Apicoplast, an Ancient Endosymbiont
Plasmodium falciparum is a remarkably complex unicellular parasite that, in addition to a mitochondrion, contains a second endosymbiont known as the apicoplast (McFadden et al., 1996; Köhler et al., 1997). This unique organelle evolved via a two-step endosymbiosis (Köhler et al., 1997). In the primary endosymbiotic event, a cyanobacterium was incorporated into a eukaryotic cell to form the modern chloroplast. During the second endosymbiotic event, a photosynthetic red alga was taken up by a protist, which led to the formation of a secondary plastid (van Dooren and Striepen, 2013). The subsequent evolution of the apicoplast resulted in the loss of all photosynthetic abilities, but retained important prokaryotic metabolic pathways including the synthesis of isoprenoids, fatty acids, iron-sulfur clusters, and heme (Ralph et al., 2004; Yeh and DeRisi, 2011; van Dooren and Striepen, 2013; Swift et al., 2021). In sharp contrast to its human host, the Plasmodium apicoplast shares molecular features with prokaryotes, plants and parasites, and therefore encompasses multiple parasite-specific drug targets (Fichera and Roos, 1997; Dahl and Rosenthal, 2007; Amberg-Johnson et al., 2017; Florentin et al., 2020). Despite its central cellular functions and clinical significance, little is known about the molecular mechanisms governing apicoplast biogenesis and development throughout the complex parasite’s cell cycle. The Plasmodium cell contains a single apicoplast organelle in all the different stages, and fusion and fission events that are common for other endosymbionts were never observed. However, the apicoplast undergoes striking morphological changes during the parasite’s intraerythrocytic growth, and develops from a small globular organelle into an elongated and branched structure (Figure 1). Moreover, the apicoplast cannot be synthesized de novo, and must be inherited. Therefore, the precise division of a single apicoplast into multiple organelles and their accurate segregation into the merozoites daughter cells are required to ensure that each nascent parasite contains a single complete apicoplast (Figure 1).
Apicoplast Elongation During Nuclear Division
Right after invasion into the RBC in the early ring stages, the apicoplast is found as a single, small globular shape (McFadden et al., 1996; Lim and McFadden, 2010). It remains this way during most of the parasite’s intraerythrocytic development including the trophozoite stages, up until schizogony begins. Only after the onset of the asynchronous nuclear divisions, the apicoplast begins to elongate (van Dooren et al., 2005). During the first rounds of nuclear replication and division (2-5 nuclei), the apicoplast organelle begins to elongate at a fast speed. It is estimated that the replication of the organelle genome begins more or less during this phase of early schizogony, but clear evidence is still missing (Williamson et al., 2002). It is clear, however, that the replication of the apicoplast genome is key to organelle inheritance and involves prokaryotic machinery (as demonstrated by its antibiotic-sensitivity (Milton and Nelson, 2016)) as well as eukaryotic components such as autophagy related protein 8 (ATG8) (Walczak et al., 2018). As was demonstrated by fluorescence in situ hybridization, ATG8 mutants fail to pass on apicoplast genome to their daughter cells, representing a unique, parasite-specific adaptation to conserved eukaryotic factors (Walczak et al., 2018). As seen in Figure 1, while schizogony proceeds (5-10 nuclei) the organelle branches out to form an intricate structure, spanning throughout the cell volume (van Dooren et al., 2005). The molecular mechanisms underlying these drastic morphological changes are completely unknown, and to date, not a single gene was shown to be directly involved in this process. Interestingly, cdc2-related protein kinase 4 (CRK4), the cell-cycle regulator that controls the decision to undergo the first round of nuclear DNA replication, does not regulate organelle development (Ganter et al., 2017). In CRK4 mutants the apicoplast elongates and branches indistinctively from wild type parasites, despite the fact that nuclear DNA replication is completely blocked (Ganter et al., 2017). This intriguing observation suggests that although apicoplast development is synced with schizogony, it is not dependent on nuclear replication. Alternatively, it may be that apicoplast and nuclear division are coupled in wildtype parasites but decoupled upon CRK4 knockdown. Additional experimentation is required to distinguish between these possibilities, and to test whether apicoplast elongation and branching is regulated by an autonomous, organelle-specific mechanism that does not rely on known cell cycle checkpoints. What is that mechanism, and how is overall cellular synchrony maintained remains to be investigated.
Apicoplast Division
The apicoplast elongates and branches, and reaches its most intricate structure during early segmentation (Rudlaff et al., 2020). As described above, segmentation is the Plasmodium equivalence of cytokinesis, and in this process the multinucleated schizont is separated into multiple daughter cells called merozoites. It is interesting to note that while the merozoites have already started individualization, the apicoplast still exists as a single organelle. Recent ultra-resolution studies revealed that it is only during mid segmentation that the apicoplast divides to produce daughter organelles (Rudlaff et al., 2020). This organelle division (or fission) needs to accurately result in a single apicoplast for each individual nascent merozoite (Figure 1). Because nuclear divisions are asynchronous and schizogony can produce varying numbers of daughter merozoites, it is unclear how apicoplast fission is regulated to produce the right number of organelles. It is clear though that at the end of this process each new daughter merozoite is equipped with a single new apicoplast organelle. The division machinery itself is unknown. The endosymbiotic evolution of the apicoplast suggests that this machinery may involve prokaryotic as well as eukaryotic components that have mostly remained elusive. The binary bacterial division involves an ancestral machinery that is based on a small GTPase called FtsZ that forms a contractile structure called a Z-ring at the fission site (Barrows and Goley, 2021). Plant chloroplasts also use an FtsZ homolog that forms a Z-ring at their inner membrane, which then recruits additional contracting proteins to the organelle outer membrane (TerBush et al., 2013). The outer ring is formed by eukaryotic dynamin-like proteins, and thus, the binary chloroplast division machinery progresses through reciprocal communication between inside and outside protein complexes across the two organellar membranes (Miyagishima, 2017). Despite the common origin, apicoplast division differs from those of its bacterial and chloroplast ancestors in several ways. First of all, it is not a binary division (i.e. a single organelle/cell splitting into two), rather a partitioning of a very long structure into multiple organelles, probably involving numerous fission sites. Second, unlike bacteria and chloroplasts, fission does not occur across one or two membranes, rather involves the contraction of four lipid bilayers. And most importantly, the Plasmodium genome does not encode any homologs to components of the FtsZ division machinery, suggesting that the apicoplast divergently evolved a distinct mode of division (Vaishnava et al., 2005; Dooren et al., 2006; Verhoef et al., 2021).
As stated above, in plants dynamin-like proteins contract the outer membrane and interact with the FtsZ ring in the inner membranes to facilitate chloroplast division (Miyagishima, 2017). Dynamins are large GTPases that mediate membrane remodeling and, similar to FtsZ, form ring-like structures in eukaryotic systems (Jimah and Hinshaw, 2019). In mammalian and yeast cells, dynamin-related proteins mediate mitochondrial fission (Nottia et al., 2021). Both Plasmodium and the related parasite Toxoplasma encode three dynamin-related genes that seem to diverge significantly from the chloroplasts orthologs and are more similar to the mitochondrial dynamin (Li et al., 2004; Charneau et al., 2007; Breinich et al., 2009; Heredero-Bermejo et al., 2019). A study in T. gondii revealed a role for a dynamin-related protein in apicoplast division (van Dooren et al., 2009). It remains to be investigated whether the Plasmodium orthologue is similarly involved in apicoplast division, whether a dynamin-ring is formed and whether other contractile rings are formed during this elusive process.
Apicoplast Segregation Into Daughter Merozoites
The segmentation of the multinucleated cell into dozens of merozoite daughter cells involves several parasite-specific cellular structures that control and facilitate this complicated process. The inner membrane complex (IMC), the associated basal complex, and the interaction of these structures with parasite nuclei are critical for segmentation. As described above, the IMC is a unique membranous structure with associated proteins inside the parasite that dictates its shape and rigidity (Beck et al., 2010; Dearnley et al., 2012). At the onset of segmentation, a ring of IMC proteins moves from the apical to the basal end of the nascent merozoite, leaving behind the incorporated IMC proteins that form a cylinder-like structure around its contents (Tran et al., 2010). At the apical end of the merozoite, the apical ring is hypothesized to nucleate the formation of sub-pellicular microtubules and that this polymerization may facilitate IMC progression from the apical to basal end (Pacheco et al., 2020). The basal complex is a group of proteins at the posterior end of the IMC, hypothesized to generate force to pull the IMC down the length of the daughter cell and mediate the final abscission step of cytokinesis (Rudlaff et al., 2019). Together, the IMC and basal complex orchestrate daughter parasite assembly and division through critical interactions with the parasite nuclei (Engelberg et al., 2016; Morano and Dvorin, 2021). Not only that IMC biogenesis and its mechanisms of action remain poorly understood; it is also completely unclear whether and how it is involved in accurate organelle sorting into daughter cells. Ultrastructure studies demonstrate that the apicoplast divides only after segmentation begins, when the IMC is roughly halfway through the cell volume (Rudlaff et al., 2020). At the end of the IMC movement, after daughter cells have been individualized, each one of them will also have a single apicoplast. How are the multiple daughter organelles sorted accurately between the nascent cells? It is particularly intriguing because nuclear divisions are asynchronous and can result in a different number of daughter cells. In Toxoplasma gondii, apicoplast division was shown to be associated with the centrosome (Striepen et al., 2000). Although Toxoplasma centrosome differs significantly from the Plasmodium microtubule organizing center in architecture and organization, they might serve a similar function with respect to apicoplast segregation. It is tempting to speculate that accurate organelle segregation during late schizogony is mediated through interactions between the apicoplast and the IMC. The rationale behind this hypothesis is that in the last decade, functional tethering between organelles has been described in most cellular eukaryotic systems, underlying the physiological significance of such interactions (Scorrano et al., 2019). Moreover, early ultrastructural analysis suggested contact sites between the Plasmodium ER and the apicoplast (Hopkins et al., 1999), as well as more recent observations between these organelles in T. gondii (Tomova et al., 2009). Similarly, contact sites between the mitochondrion and the apicoplast were reported (van Dooren et al., 2005), and it was suggested that these interactions might represent a mechanism to ensure accurate sorting of organelles (Verhoef et al., 2021). Therefore, it may very well be that the IMC serves as a central cellular hub that physically links the nuclei and organelles including the apicoplast during segmentation, to ensure that every daughter parasite receives a complete set of cellular content. This hypothesis needs to be tested experimentally, and if proven correct, will explain how accurate organelle sorting is achieved despite the asynchronous nature of nuclear division. Such physical tethering will also provide unique evidence of functional organelle contact sites in Plasmodium.
Apicoplast Dynamics in Other Plasmodium Life Stages
Due to limited culturing and complicated experimental settings, the study of apicoplast dynamics in P. falciparum has been mostly focused on the parasite’s asexual replication within the erythrocyte. A small subpopulation of the erythrocyte infecting parasites will undergo sexual differentiation in a process called gametocytogenesis, transforming into the infective gametocyte stages (Ngotho et al., 2019). The metabolic function of the apicoplast during this process is comparable to its roles during the intraerythrocytic asexual replication (Wiley et al., 2015), and the morphological changes that it undergoes were described by live microscopy (Okamoto et al., 2009). A detailed description and the molecular mechanisms underlying the timing and morphological transitions of apicoplast biogenesis and fission events are yet to be unveiled.
Most importantly, the complete parasite life cycle also includes massive replication in human hepatocytes as well as sexual development within the mosquito vector. The studies of organelle dynamics during these stages rely mostly on murine models, involving related Plasmodium species such as P. berghei (Stanway et al., 2009). The imaging of P. berghei-infecting mouse hepatocytes revealed remarkably complex organelle morphologies (Stanway et al., 2011). In these stages, a single infecting sporozoite divides and develops into thousands of daughter merozoites inside the hepatocyte, and the rapid growth and fission of the apicoplast during this process is astonishing (Stanway et al., 2011). These processes are reminiscent of the morphological changes that the apicoplast undergoes inside the erythrocyte but on a much larger scale, and are even less understood. Similarly, very little data have been gathered concerning apicoplast development during mosquito stages, which are characterized by additional metabolic requirements from the organelle (van Schaijk et al., 2013; Korbmacher et al., 2021). The questions remain whether similar processes occur during the apicoplast liver and mosquito development in P. falciparum, and what are the molecular mechanisms that control and execute these subcellular developments.
Conclusions and Open Questions
● The autonomous nature of apicoplast development during nuclear replication: Although apicoplast elongation happens together with nuclear replication, these two processes seem to occur independently of each other, as suggested by the normal apicoplast development documented in cell cycle mutants (Ganter et al., 2017), and the normal asexual replication observed in apicoplast-less parasites supplemented with essential metabolites (Yeh and DeRisi, 2011; Florentin et al., 2020). How is organelle biogenesis coordinated with nuclear replication and division? Is there an apicoplast-specific mechanism in the organelle itself that times and controls these morphological changes? If so, what is it? What other regulatory mechanisms are in place to ensure that nuclear replication and organelle development are coordinated?
● Obscure aspects of apicoplast fission: Despite the common origin, apicoplast division differs from those of bacteria and chloroplasts because it is not a binary division, it occurs across four lipid bilayers, and it does not involve a homolog of the FtsZ division machinery (Vaishnava et al., 2005; Dooren et al., 2006; Verhoef et al., 2021). All of these facts suggest that the apicoplast divergently evolved a distinct mode of division, which might represent an attractive target for drug development. What cellular components mediate this process? Are dynamin-related-proteins involved in Plasmodium apicoplast fission, similar to those that mediate this process in Toxoplasma? If so, on which of the four membranes do they act, and what other organellar components contract the membranes from within?
● Apicoplast-cytoskeleton contact sites during segmentation: After the apicoplast divides, the resulting multiple organelles are sorted accurately between the daughter merozoite cells. It is unclear how this exact sorting is mediated, particularly in light of the asynchronous nature of nuclear divisions that result in varying numbers of daughter cells. One intriguing hypothesis is that physical contact sites between the apicoplast and other cellular components guarantee precise division. The inner membrane complex (IMC) is a key mediator of segmentation, and thus might serve as a central tethering point for cellular content, including the apicoplast. Experimental evidence is still missing, and thus the process of accurate organelle sorting at the final stage of the Plasmodium cell cycle remains enigmatic.
Author Contributions
AE, SA, and AF wrote together the manuscript. All authors contributed to manuscript revision, read, and approved the submitted version.
Acknowledgments
AF is supported by The Abisch-Frenkel Faculty Development Lectureship. Manuscript preparation was supported by a training-travel grant by The Kuvin Foundation to AE.
Conflict of Interest
The authors declare that the research was conducted in the absence of any commercial or financial relationships that could be construed as a potential conflict of interest.
Publisher’s Note
All claims expressed in this article are solely those of the authors and do not necessarily represent those of their affiliated organizations, or those of the publisher, the editors and the reviewers. Any product that may be evaluated in this article, or claim that may be made by its manufacturer, is not guaranteed or endorsed by the publisher.
References
Absalon, S. (2020). Msphere of Influence: The Dynamic Nature of the Nuclear Envelope During Mitosis of Malaria Parasites. mSphere 5, e00815–e00820. doi: 10.1128/mSphere.00815-20
Absalon, S., Robbins, J. A., Dvorin, J. D. (2016). An Essential Malaria Protein Defines the Architecture of Blood-Stage and Transmission-Stage Parasites. Nat. Commun. 7, 11449. doi: 10.1038/ncomms11449
Al-Khattaf, F. S., Tremp, A. Z., Dessens, J. T. (2014). Plasmodium Alveolins Possess Distinct But Structurally and Functionally Related Multi-Repeat Domains. Parasitol Res. 114, 631–639. doi: 10.1007/s00436-014-4226-9
Amberg-Johnson, K., Hari, S. B., Ganesan, S. M., Lorenzi, H. M., Sauer, R. T., Niles, J. C., et al. (2017). Small Molecule Inhibition of Apicomplexan FtsH1 Disrupts Plastid Biogenesis in Human Pathogens. eLife 6, 4525. doi: 10.7554/eLife.29865
Barrows, J. M., Goley, E. D. (2021). FtsZ Dynamics in Bacterial Division: What, How, and Why? Curr. Opin. Cell Biol. 68, 163–172. doi: 10.1016/j.ceb.2020.10.013
Baum, J., Tonkin, C. J., Paul, A. S., Rug, M., Smith, B. J., Gould, S. V., et al. (2006). A Conserved Molecular Motor Drives Cell Invasion and Gliding Motility Across Malaria Life Cycle Stages and Other Apicomplexan Parasites. J. Biol. Chem. 281, 5197–5208. doi: 10.1074/jbc.M509807200
Beck, J. R., Rodriguez-Fernandez, I. A., Cruz de Leon, J., Huynh, M.-H., Carruthers, V. B., Morrisette, N. S., et al. (2010). A Novel Family of Toxoplasma IMC Proteins Displays a Hierarchical Organization and Functions in Coordinating Parasite Division. PloS Pathog. 6, e1001094. doi: 10.1371/journal.ppat.1001094
Bergman, L. W., Kaiser, K., Fujioka, H., Coppens, I., Daly, T. M., Fox, S., et al. (2003). Myosin A Tail Domain Interacting Protein (MTIP) Localizes to the Inner Membrane Complex of Plasmodium Sporozoites. J. Cell Sci. 116, 39–49. doi: 10.1242/jcs.00194
Bertiaux, E., Balestra, A. C., Bournonville, L., Louvel, V., Maco, B., Soldati-Favre, M., et al. (2021). Expansion Microscopy Provides New Insights Into the Cytoskeleton of Malaria Parasites Including the Conservation of a Conoid. PloS Biol. 19, e3001020. doi: 10.1371/journal.pbio.3001020
Breinich, M. S., Ferguson, D. J. P., Foth, B. J., van Dooren, G. G., Lebrun, M., Quon, D. V., et al. (2009). A Dynamin Is Required for the Biogenesis of Secretory Organelles in Toxoplasma Gondii. Curr. Biol. 19, 277–286. doi: 10.1016/j.cub.2009.01.039
Charneau, S., Dourado Bastos, I. M., Mouray, E., Morais Ribeiro, B., Santana, J. M., Grellier, P., et al. (2007). Characterization of PfDYN2, A Dynamin-Like Protein of Plasmodium Falciparum Expressed in Schizonts. Microbes Infect. 9, 797–805. doi: 10.1016/j.micinf.2007.02.020
Dahl, E. L., Rosenthal, P. J. (2007). Multiple Antibiotics Exert Delayed Effects Against the Plasmodium Falciparum Apicoplast. Antimicrob. Agents Chemother. 51, 3485–3490. doi: 10.1128/AAC.00527-07
Dearnley, M. K., Yeoman, J. A., Hanssen, E., Kenny, S., Turnbull, L., Whitchurch, C. B., et al. (2012). Origin, Composition, Organization and Function of the Inner Membrane Complex of Plasmodium Falciparum Gametocytes. J. Cell Sci. 125, 2053–2063. doi: 10.1242/jcs.099002
Di Nottia, M., Verrigni, D., Torraco, A., Rizza, T., Bertini, E., Carrozzo, R. (2021). Mitochondrial Dynamics: Molecular Mechanisms, Related Primary Mitochondrial Disorders and Therapeutic Approaches. Genes-Basel 12, 247. doi: 10.3390/genes12020247
Dondorp, A. M., Nosten, F., Yi, P., Das, D., Phae Phyo, A., Tarning, J., et al. (2009). Artemisinin Resistance in Plasmodium Falciparum Malaria. N. Engl. J. Med. 361, 455–467. doi: 10.1056/NEJMoa0808859
Dooren, G. G. V., Stimmler, L. M., McFadden, G. I. (2006). Metabolic Maps and Functions of the Plasmodium Mitochondrion. FEMS Microbiol. Rev. 30, 596–630. doi: 10.1111/j.1574-6976.2006.00027.x
Engelberg, K., Ivey, F. D., Lin, A., Kono, M., Lorestani, A., Faugno-Fusci, D., et al. (2016). A MORN1-Associated HAD Phosphatase in the Basal Complex Is Essential for Toxoplasma Gondii Daughter Budding: Toxoplasma Basal Complex HAD Phosphatase. Cell Microbiol. 18, 1153–1171. doi: 10.1111/cmi.12574
Fichera, M. E., Roos, D. S. (1997). A Plastid Organelle as a Drug Target in Apicomplexan Parasites. Nature 390, 407–409. doi: 10.1038/37132
Florentin, A., Stephens, D. R., Brooks, C. F., Baptista, R. P., Muralidharan, V. (2020). Plastid Biogenesis in Malaria Parasites Requires the Interactions and Catalytic Activity of the Clp Proteolytic System. Proc. Natl. Acad. Sci. U. S. A. 3, 201919501. doi: 10.1073/pnas.1919501117
Francia, M. E., Striepen, B. (2014). Cell Division in Apicomplexan Parasites. Nat. Rev. Microbiol. 12, 125–136. doi: 10.1038/nrmicro3184
Frénal, K., Polonais, V., Marq, J-B., Stratmann, R., Limenitakis, J., Soldati-Favre, D. (2010). Functional Dissection of the Apicomplexan Glideosome Molecular Architecture. Cell Host Microbe 8, 343–357. doi: 10.1016/j.chom.2010.09.002
Ganter, M., Goldberg, J. M., Dvorin, J., Paulo, J. A., King, J. G., Tripathi, A. K., et al. (2017). Plasmodium Falciparum CRK4 Directs Continuous Rounds of DNA Replication During Schizogony. Nat. Microbiol. 2, 17017. doi: 10.1038/nmicrobiol.2017.17
Gardner, M. J., Hall, N., Fung, E., White, O., Berriman, M., Hyman, R. W., et al. (2002). Genome Sequence of the Human Malaria Parasite Plasmodium Falciparum. Nature 419, 498–511. doi: 10.1038/nature01097
Garg, S., Agarwal, S., Dabral, S., Kumar, N., Sehrawat, S., Singh, S. (2015). Visualization and Quantification of Plasmodium Falciparum Intraerythrocytic Merozoites. Syst. Synthetic Biol. 9, 23–26. doi: 10.1007/s11693-015-9167-9
Garrido-Cardenas, J. A., González-Cerón, L., Manzano-Agugliaro, F., Mesa-Valle, C. (2019). Plasmodium Genomics: An Approach for Learning About and Ending Human Malaria. Parasitol. Res. 118, 1–27. doi: 10.1007/s00436-018-6127-9
Geoghegan, N. D., Evelyn, C., Whitehead, L. W., Pasternak, M., McDonald, P., et al. (2021). 4D Analysis of Malaria Parasite Invasion Offers Insights Into Erythrocyte Membrane Remodeling and Parasitophorous Vacuole Formation. Nat. Commun. 12, 3620. doi: 10.1038/s41467-021-23626-7
Gerald, N., Mahajan, B., Kumar, S. (2011). Mitosis in the Human Malaria Parasite Plasmodium Falciparum. Eukaryot Cell 10, 474–482. doi: 10.1128/EC.00314-10
Ghorbal, M., Gorman, M., Macpherson, C. R., Martins, R. M., Scherf, A., Lopez-Rubio, J-J. (2014). Genome Editing in the Human Malaria Parasite Plasmodium Falciparum Using the CRISPR-Cas9 System. Nat. Biotechnol. 32, 819–821. doi: 10.1038/nbt.2925
Gould, S. B., Tham, W.-H., Cowman, A. F., McFadden, G. I., Waller, R. F. (2008). Alveolins, A New Family of Cortical Proteins That Define the Protist Infrakingdom Alveolata. Mol. Biol. Evol. 25, 1219–1230. doi: 10.1093/molbev/msn070
Gubbels, M.-J., Coppens, I., Zarringhalam, K., Duraisingh, M. T., Engelberg, K. (2021). The Modular Circuitry of Apicomplexan Cell Division Plasticity. Front. Cell Infect. Microbiol. 11, 670049. doi: 10.3389/fcimb.2021.670049
Harding, C. R., Meissner, M. (2014). The Inner Membrane Complex Through Development of Toxoplasma Gondii and Plasmodium: The IMC in Plasmodium and Toxoplasma. Cell Microbiol. 16, 632–641. doi: 10.1111/cmi.12285
Heredero-Bermejo, I., Varberg, J. M., Charvat, R., Jacobs, K., Garbuz, T., Sullivan, W. J., et al. (2019). TgDrpC, an Atypical Dynamin-Related Protein in Toxoplasma Gondii, Is Associated With Vesicular Transport Factors and Parasite Division. Mol. Microbiol. 111, 46–64. doi: 10.1111/mmi.14138
Hopkins, J., Fowler, R., Krishna, S., Wilson, I., Mitchell, G., Bannister, L. (1999). The Plastid in Plasmodium Falciparum Asexual Blood Stages: A Three-Dimensional Ultrastructural Analysis. Protist 150, 283–295. doi: 10.1016/S1434-4610(99)70030-1
Hu, K., Mann, T., Striepen, B., Beckers, C J.M., Roos, D. S., Murray, J. M. (2002). Daughter Cell Assembly in the Protozoan Parasite Toxoplasma Gondii. Mol. Biol. Cell 13, 593–606. doi: 10.1091/mbc.01-06-0309
Jimah, J. R., Hinshaw, J. E. (2019). Structural Insights Into the Mechanism of Dynamin Superfamily Proteins. Trends Cell Biol. 29, 257–273. doi: 10.1016/j.tcb.2018.11.003
Keeley, A., Soldati, D. (2004). The Glideosome: A Molecular Machine Powering Motility and Host-Cell Invasion by Apicomplexa. Trends Cell Biol. 14, 528–532. doi: 10.1016/j.tcb.2004.08.002
Khater, E. I., Sinden, R. E., Dessens, J. T. (2004). A Malaria Membrane Skeletal Protein Is Essential for Normal Morphogenesis, Motility, and Infectivity of Sporozoites. J. Cell Biol. 167, 425–432. doi: 10.1083/jcb.200406068
Köhler, S., Delwiche, C. F., Denny, P. W., Tilney, L. G., Webster, P., Wilson, R. J., et al. (1997). A Plastid of Probable Green Algal Origin in Apicomplexan Parasites. Science 275, 1485–1489. doi: 10.1126/science.275.5305.1485
Kono, M., Prusty, D., Parkinson, J., Gilberger, T. W. (2013). A Membranous System in the Spotlight. Front. Biosci. 18, 982. doi: 10.2741/4157
Korbmacher, F., Drepper, B., Sanderson, T., Martin, P., Stach, T., Maier, A. G., et al. (2021). An Apicoplast-Resident Folate Transporter Is Essential for Sporogony of Malaria Parasites. Cell Microbiol. 23, e13266. doi: 10.1111/cmi.13266
Kudyba, H. M., Cobb, D. W., Vega-Rodríguez, J., Muralidharan, V. (2021). Some Conditions Apply: Systems for Studying. Plasmodium falciparum Protein Funct. PloS Pathog. 17, e1009442. doi: 10.1371/journal.ppat.1009442
Li, H., Han, Z., Lu, Y., Lin, Y., Zhang, L., Wu, Y., et al. (2004). Isolation and Functional Characterization of a Dynamin-Like Gene From Plasmodium Falciparum. Biochem. Bioph. Res. Co 320, 664–671. doi: 10.1016/j.bbrc.2004.06.010
Liffner, B., Absalon, S. (2021). Expansion Microscopy Reveals Plasmodium Falciparum Blood-Stage Parasites Undergo Anaphase With a Chromatin Bridge in the Absence of Mini-Chromosome Maintenance Complex Binding Protein. Microorg 9, 2306. doi: 10.3390/microorganisms9112306
Lim, L., McFadden, G. I. (2010). The Evolution, Metabolism and Functions of the Apicoplast. Philos. Trans. R Soc. B Biol. Sci. 365, 749–763. doi: 10.1098/rstb.2009.0273
Mann, T., Beckers, C. (2001). Characterization of the Subpellicular Network, A Filamentous Membrane Skeletal Component in the Parasite Toxoplasma Gondii. Mol. Biochem. Parasit 115, 257–268. doi: 10.1016/S0166-6851(01)00289-4
McFadden, G. I., Reith, M. E., Munholland, J., Lang-Unnasch, N. (1996). Plastid in Human Parasites. Nature 381, 482–482. doi: 10.1038/381482a0
Medeiros, L. C. S., Souza, W. D., Jiao, C., Barrabin, H., Miranda, K. (2012). Visualizing the 3d Architecture of Multiple Erythrocytes Infected With Plasmodium at Nanoscale by Focused Ion Beam-Scanning Electron Microscopy. PloS One 7, e33445. doi: 10.1371/journal.pone.0033445
Milton, M. E., Nelson, S. W. (2016). Replication and Maintenance of the Plasmodium Falciparum Apicoplast Genome. Mol. Biochem. Parasit 208, 56–64. doi: 10.1016/j.molbiopara.2016.06.006
Miyagishima, S. (2017). Chloroplast Division: A Handshake Across Membranes. Nat. Plants 3, 17025. doi: 10.1038/nplants.2017.25
Morano, A. A., Dvorin, J. D. (2021). The Ringleaders: Understanding the Apicomplexan Basal Complex Through Comparison to Established Contractile Ring Systems. Front. Cell. Infect. Microbiol. 11, 656976. doi: 10.3389/fcimb.2021.656976
Morrissette, N. S., Sibley, L. D. (2002). Cytoskeleton of Apicomplexan Parasites. Microbiol. Mol. Biol. R 66, 21–38. doi: 10.1128/MMBR.66.1.21-38.2002
Ngotho, P., Soares, A. B., Hentzschel, F., Achcar, F., Bertuccini, L., Marti, M. (2019). Revisiting Gametocyte Biology in Malaria Parasites. FEMS Microbiol. Rev. 43, 401–414. doi: 10.1093/femsre/fuz010
Okamoto, N., Spurck, T. P., Goodman, C. D., McFadden, G. I. (2009). Apicoplast and Mitochondrion in Gametocytogenesis of Plasmodium Falciparum. Eukaryot Cell 8, 128–132. doi: 10.1128/EC.00267-08
Pacheco, N. D. S., Tosetti, N., Koreny, L., Waller, R. F., Soldati-Favre, D. (2020). Evolution, Composition, Assembly, and Function of the Conoid in Apicomplexa. Trends Parasitol 36, 688–704. doi: 10.1016/j.pt.2020.05.001
Phillips, M. A., Burrows, J. N., Manyando, C., van Huijsduijnen, R. H., Van Voorhis, W. C., Wells, T. N.C. (2017). Malaria. Nat. Rev. Dis. Primers 3, 17050. doi: 10.1038/nrdp.2017.50
Ralph, S. A., van Dooren, G. G., Waller, R. F., Crawford, M. J., Fraunholz, M. J., Foth, B. J., et al. (2004). Tropical Infectious Diseases: Metabolic Maps and Functions of the Plasmodium Falciparum Apicoplast. Nat. Rev. Microbiol. 2, 203–216. doi: 10.1038/nrmicro843
Rudlaff, R. M., Kraemer, S., Marshman, J., Dvorin, J. D. (2020). Three-Dimensional Ultrastructure of Plasmodium Falciparum Throughout Cytokinesis. PloS Pathog. 16, e1008587. doi: 10.1371/journal.ppat.1008587
Rudlaff, R. M., Kraemer, S., Streva, V. A., Dvorin, J. D. (2019). An Essential Contractile Ring Protein Controls Cell Division in Plasmodium Falciparum. Nat. Commun. 10, 2181. doi: 10.1038/s41467-019-10214-z
Scorrano, L., De Matteis, M. A., Emr, S., Giordano, F., Hajnóczky, G., Kornmann, B., et al. (2019). Coming Together to Define Membrane Contact Sites. Nat. Commun. 10, 1287. doi: 10.1038/s41467-019-09253-3
Stanway, R. R., Mueller, N., Zobiak, B., Graewe, S., Froehlke, U., Zessin, P. J. M., et al. (2011). Organelle Segregation Into Plasmodium Liver Stage Merozoites: Plasmodium Organelle Segregation. Cell Microbiol. 13, 1768–1782. doi: 10.1111/j.1462-5822.2011.01657.x
Stanway, R. R., Witt, T., Zobiak, B., Aepfelbacher, M., Heussler, V. T. (2009). GFP-Targeting Allows Visualization of the Apicoplast Throughout the Life Cycle of Live Malaria Parasites. Biol. Cell 101, 415–435. doi: 10.1042/BC20080202
Striepen, B., Crawford, M. J., Shaw, M., Tilney, L. G., Seeber, F., Roos, D. S. (2000). The Plastid of Toxoplasma Gondii is Divided by Association With the Centrosomes. J. Cell Biol. 151, 1423–1434. doi: 10.1083/jcb.151.7.1423
Striepen, B., Jordan, C. N., Reiff, S., van Dooren, G. G. (2007). Building the Perfect Parasite: Cell Division in Apicomplexa. PloS Pathog. 3, e78. doi: 10.1371/journal.ppat.0030078
Su, X., Lane, K. D., Xia, L., Sá, J. M., Wellems, T. E. (2019). Plasmodium Genomics and Genetics: New Insights Into Malaria Pathogenesis, Drug Resistance, Epidemiology, and Evolution. Clin. Microbiol. Rev. 32 (4), e00019–e00019. doi: 10.1128/CMR.00019-19
Swift, R. P., Rajaram, K., Liu, H. B., Prigge, S. T. (2021). Dephospho-CoA Kinase, A Nuclear-Encoded Apicoplast Protein, Remains Active and Essential After Plasmodium Falciparum Apicoplast Disruption. EMBO J. 40, e107247. doi: 10.15252/embj.2020107247
TerBush, A. D., Yoshida, Y., Osteryoung, K. W. (2013). FtsZ in Chloroplast Division: Structure, Function and Evolution. Curr. Opin. Cell Biol. 25, 461–470. doi: 10.1016/j.ceb.2013.04.006
Tomasina, R., González, F., Francia, M. E. (2021). Structural and Functional Insights Into the Microtubule Organizing Centers of Toxoplasma Gondii and Plasmodium Spp. Microorganisms 9, 2503. doi: 10.3390/microorganisms9122503
Tomova, C., Humbel, B. M., Geerts, W. J.C., Entzeroth, R., Holthuis, J. C.M., Verkleij, A. J. (2009). Membrane Contact Sites Between Apicoplast and ER in Toxoplasma Gondii Revealed by Electron Tomography. Traffic 10, 1471–1480. doi: 10.1111/j.1600-0854.2009.00954.x
Tran, J. Q., de Leon, J. C., Li, C., Huynh, M-H., Beatty, W., Morrissette, N. S. (2010). RNG1 Is a Late Marker of the Apical Polar Ring in Toxoplasma Gondii. Cytoskeleton 67, 586–598. doi: 10.1002/cm.20469
Tremp, A. Z., Al-Khattaf, F. S., Dessens, J. T. (2014). Distinct Temporal Recruitment of Plasmodium Alveolins to the Subpellicular Network. Parasitol Res. 113, 4177–4188. doi: 10.1007/s00436-014-4093-4
Vaishnava, S., Morrison, D. P., Gaji, R. Y., Murray, J. M., Entzeroth, R., Howe, D. K., et al. (2005). Plastid Segregation and Cell Division in the Apicomplexan Parasite Sarcocystis Neurona. J. Cell Sci. 118, 3397–3407. doi: 10.1242/jcs.02458
van Dooren, G. G., Marti, M., Tonkin, C. J., Stimmler, L. M., Cowman, A. F., McFadden, G. I. (2005). Development of the Endoplasmic Reticulum, Mitochondrion and Apicoplast During the Asexual Life Cycle of Plasmodium Falciparum. Mol. Microbiol. 57, 405–419. doi: 10.1111/j.1365-2958.2005.04699.x
van Dooren, G. G., Reiff, S. B., Tomova, C., Meissner, M., Humbel, B. M., Striepen, B. (2009). A Novel Dynamin-Related Protein Has Been Recruited for Apicoplast Fission in Toxoplasma Gondii. Curr. Biol: CB 19, 267–276. doi: 10.1016/j.cub.2008.12.048
van Dooren, G. G., Striepen, B. (2013). The Algal Past and Parasite Present of the Apicoplast. Annu. Rev. Microbiol. 67, 271–289. doi: 10.1146/annurev-micro-092412-155741
van Schaijk, B. C. L., Kumar, T. R.S., Vos, M. W., Richman, A., van Gemert, G-J., Li, T., et al. (2013). Type II Fatty Acid Biosynthesis Is Essential for Plasmodium Falciparum Sporozoite Development in the Midgut of Anopheles Mosquitoes. Eukaryot Cell 13, 550–559. doi: 10.1128/EC.00264-13
Verhoef, J. M. J., Meissner, M., Kooij, T. W. A. (2021). Organelle Dynamics in Apicomplexan Parasites. mBio 12, e01409–e01421. doi: 10.1128/mBio.01409-21
Wagner, J. C., Platt, R. J., Goldfless, S. J., Zhang, F., Niles, J. C. (2014). Efficient CRISPR-Cas9-Mediated Genome Editing in Plasmodium Falciparum. Nat. Methods 11, 915–918. doi: 10.1038/nmeth.3063
Walczak, M., Ganesan, S. M., Niles, J. C., Yeh, E. (2018). ATG8 Is Essential Specifically for an Autophagy-Independent Function in Apicoplast Biogenesis in Blood-Stage Malaria Parasites. mBio 9, e02021–e02017. doi: 10.1128/mBio.02021-17
Wiley, J. D., Merino, E. F., Krai, P. M., McLean, K. J., Tripathi, A. K., Vega-Rodríguez, J., et al. (2015). Isoprenoid Precursor Biosynthesis Is the Essential Metabolic Role of the Apicoplast During Gametocytogenesis in Plasmodium Falciparum. Eukaryot Cell 14, 128–139. doi: 10.1128/EC.00198-14
Williamson, D. H., Preiser, P. R., Moore, P. W., McCready, S., Strath, M., Wilson, R. J. M. (2002). The Plastid DNA of the Malaria Parasite Plasmodium Falciparum is Replicated by Two Mechanisms. Mol. Microbiol. 45, 533–542. doi: 10.1046/j.1365-2958.2002.03033.x
Winzeler, E. A. (2008). Malaria Research in the Post-Genomic Era. Nature 455, 751–756. doi: 10.1038/nature07361
World Health Organization. (2019). World Malaria Report 2018. World Health Organization (February 12, 2019).
World Health Organization. (2021). World Malaria Report 2021. World Health Organization (December 6, 2021).
Keywords: plasmodium, malaria, apicoplast, cell cycle, schizogony, organelle dynamics
Citation: Elaagip A, Absalon S and Florentin A (2022) Apicoplast Dynamics During Plasmodium Cell Cycle. Front. Cell. Infect. Microbiol. 12:864819. doi: 10.3389/fcimb.2022.864819
Received: 28 January 2022; Accepted: 11 March 2022;
Published: 29 April 2022.
Edited by:
Tania F. De Koning-Ward, Deakin University, AustraliaReviewed by:
Paul Sigala, The University of Utah, United StatesGeoffrey McFadden, The University of Melbourne, Australia
Copyright © 2022 Elaagip, Absalon and Florentin. This is an open-access article distributed under the terms of the Creative Commons Attribution License (CC BY). The use, distribution or reproduction in other forums is permitted, provided the original author(s) and the copyright owner(s) are credited and that the original publication in this journal is cited, in accordance with accepted academic practice. No use, distribution or reproduction is permitted which does not comply with these terms.
*Correspondence: Sabrina Absalon, c2Fic2Fsb25AaXUuZWR1; Anat Florentin, YW5hdC5mbG9yZW50aW5AbWFpbC5odWppLmFjLmls