- Division of Bio-Resource Sciences, Bio-Herb Research Institute, and Interdisciplinary Program in Smart Agriculture, Kangwon National University, Chuncheon, South Korea
Colletotrichum scovillei is the major anthracnose fungus of sweet pepper and chili pepper (Capsicum annuum L.), causing significant losses in the yield and quality of the pepper fruits. Molecular mechanisms governing development and pathogenicity have been widely studied in many foliar fungal pathogens, but the information on fruit diseases is still limited. In this study, we determined the functional roles of the dual-specificity tyrosine phosphorylation-regulated kinase CsPOM1 in C. scovillei. Knockout mutant for CsPOM1 gene was obtained via homology-dependent gene replacement. The ΔCspom1 mutant exhibited a reduction in vegetative growth on osmotic stress, surface hydrophobicity, and conidiation compared with wild-type. Conidia of the ΔCspom1 mutant were already two-celled before inoculation on an induction surface, indicating that CsPOM1 negatively regulates conidial cell division. The ΔCspom1 mutant, similar to wild-type, formed appressoria on the plant surface, but was significantly reduced on hydrophobic coverslips, probably due to a defect in the recognition of surface hydrophobicity. Treatment of conidia with cutin monomers restored appressorium formation on hydrophobic coverslips in the ΔCspom1 mutant. On pepper fruits, the ΔCspom1 mutant exhibited delayed penetration and invasive growth, leading to significantly reduced virulence. Collectively, the results showed that CsPOM1 is important for stress tolerance, conidiation, surface hydrophobicity, appressorium formation, and virulence in C. scovillei.
Introduction
The molecular mechanisms governing fungal development and pathogenicity of many foliar fungal pathogens have been extensively studied through functional genomics (Asakura et al., 2009; Wilson and Talbot, 2009). However, compared with foliar diseases, there is still limited information on fruit diseases, such as anthracnose caused by Colletotrichum species. The genus Colletotrichum is a major group of phytopathogenic fungi in the filamentous ascomycetes, causing anthracnose disease in many vegetables and fruits. The number of species within the genus ranges from 29 to more than 700, depending on taxonomic interpretation (Cannon et al., 2007; Dean et al., 2012). Due to the wide host range of Colletotrichum species, which included important crops such as apple, banana, coffeeberry, mango, and pepper, the genus is considered the eighth most important group of phytopathogenic fungi in the world (Dean et al., 2012). Anthracnose of sweet pepper and chili pepper (Capsicum annuum L.) caused by several Colletotrichum species, including C. scovillei, C. truncatum, C. fructicola, C. dematium, C. gloeosporioides, C. coccodes, and C. capsici, leads to significant losses in the yield and quality of pepper fruits in both pre- and postharvest periods (Caires et al., 2014; Kanto et al., 2014; Liu et al., 2016; Zhao et al., 2016; Diao et al., 2017). Recent advances in sequencing technology have revealed that C. scovillei, which belongs to the C. acutatum species complex is the dominant fungal pathogen of pepper anthracnose disease worldwide (Caires et al., 2014; Zhao et al., 2016; Diao et al., 2017; Noor and Zakaria, 2018; Khalimi et al., 2019). As C. scovillei was shown to be the most dominant fungal pathogen in pepper anthracnose in many countries, whole-genome sequencing, annotation, and genetic transformation methods for this pathogen have been established to understand fungal biology and pathogenicity (Han et al., 2016; Shin et al., 2019).
Fungi possess conserved signal transduction pathways, such as mitogen-activated protein kinase (MAPK) signaling pathways and the cyclic adenosine monophosphate (cAMP)-dependent protein kinase signaling pathway, which regulates developmental processes, stress responses, and virulence in many fungi (Xu and Hamer, 1996; Adachi and Hamer, 1998; Takano et al., 2000; Hagiwara et al., 2009; Mehrabi et al., 2009). Studying the mechanisms of fungal signaling systems provides information important for understanding fungal development and pathogenicity. One of the best-studied MAPK pathways is the Pmk1 MAPK pathway, which is required for appressorium formation and plant infection in many phytopathogenic fungi (Xu and Hamer, 1996; Zhao et al., 2007; Liang et al., 2019; Fu et al., 2022). The high osmolarity glycerol (HOG) MAPK pathway is also one of the well-characterized MAPK pathways that mediate cellular adaptation to hyperosmotic stress (Van Wuytswinkel et al., 2000; Tatebayashi et al., 2006; Wu et al., 2006; Hohmann, 2009; Duran et al., 2010; Saito and Posas, 2012). Recently, it has been revealed that a DYRK-family kinase PomA (a homolog of Pom1) is involved in the negative regulation of the HOG MAPK pathway in Aspergillus nidulans, affecting the phosphorylation level of Hog1, suggests important roles for PomA in the regulation of HOG MAPK pathway (Zhou et al., 2019).
DYRKs are serine/threonine protein kinases that autophosphorylate a tyrosine residue in their activation loop and phosphorylate their substrates on serine and threonine residues (Kentrup et al., 1996; Becker et al., 1998). The DYRK family can be classified into three subfamilies, i.e., DYRK kinases, homeodomain-interacting protein kinases, and pre-mRNA processing protein 4 kinases, based on the homology within the kinase domain (Aranda et al., 2011). Among them, Yak1 and Pom1 are well-characterized DYRK kinases in yeast (Garrett et al., 1991; Bähler and Pringle, 1998). Yak1, the first DYRK kinase discovered, acts as a negative regulator of growth in the budding yeast Saccharomyces cerevisiae (Garrett et al., 1991). Pom1 is well studied in the fission yeast Schizosaccharomyces pombe, in which Pom1 provides positional information for both growth and division (Bähler and Pringle, 1998). However, the functional roles of Pom1 kinase in fungal development and virulence remain largely unknown in filamentous fungi. This prompted us to investigate the functional roles of Pom1 in C. scovillei.
In this study, we identified C. scovillei POM1 as a homolog of S. pombe POM1, which was designated CsPOM1. This study aimed to functionally characterize the roles of CsPOM1 in fungal development and pathogenicity. We generated a targeted deletion mutant of CsPOM1 and performed phenotypic analysis. The CsPOM1 deletion mutant exhibited defects in stress tolerance, hydrophobicity, cell division, conidiation, appressorium formation, and virulence. In addition, we found that the expression of glycogen debranching and class II hydrophobin genes was significantly altered in the CsPOM1 deletion mutant, which might affect the phenotypic defects of the mutant.
Materials and Methods
Fungal Strains and Culture Conditions
Colletotrichum scovillei strain KC05 (Han et al., 2016) was used as the wild-type (WT) strain in this study. Transformants were selected on TB3 agar (200 g of sucrose, 3 g of yeast extract, 3 g of casamino acids, 10 g of glucose, and 8 g of agar/L) containing 200 µg/ml of hygromycin B (EMD Millipore, USA) or 400 µg/ml of G418 geneticin (Gibco, USA). Complete agar (CMA, 10 g of sucrose, 6 g of yeast extract, 6 g of casamino acids, and 15 g of agar/L) was used for vegetative growth. V8 agar (V8A, 80 ml of V8 juice, 310 µl 10 N NaOH, and 15 g of agar/L) and oatmeal agar (OMA, 50 g oatmeal and 25 g agar/L) were used for conidiation. Experiments were conducted in triplicates and repeated three times. All data were processed with the SigmaStar statistical software package (SPSS Science, Chicago, IL).
Bioinformatics Analysis
All sequence information used in this study was obtained from the online database CFGP (http://cfgp.snu.ac.kr). Protein sequences of putative glycogen debranching enzyme (CAP_006079), class II hydrophobin (CAP_004537), and dual-specificity tyrosine phosphorylation-regulated kinase Pom1 (CAP_000731) in C. scovillei were obtained through BLAST searches conducted using the protein sequences of Gdb1 (AJW05075) in Saccharomyces cerevisiae, Mhp1 (MG01173) in Magnaporthe oryzae, and Pom1 (NP_592974) in Schizosaccharomyces pombe, respectively. Protein sequence alignment of CsPOM1 with related proteins from fungi was performed using the ClustalW program in MEGA 6.0 (Thompson et al., 1994; Tamura et al., 2013). Phylogenetic analysis of obtained protein sequences was performed using a neighbor-joining method (10,000 bootstrap replicates) (Tamura et al., 2013). Domain structure analysis was performed using InterPro Scan v83.0 (http://www.ebi.ac.uk/interpro/) (Mulder et al., 2005). Oligonucleotide primers used in this study were designed using PrimerQuest® Design Tool (http://sg.idtdna.com/site) and synthesized by Bioneer (Daejeon, South Korea).
Vector Construction for Fluorescence Microscopy
Plasmid pIGPAPA containing a GFP gene under the control of the Neurospora crassa lyase gene promoter and a geneticin resistance gene (gen) cassette under the control of the A. nidulans trpC promoter was provided from the Fungal Plant Pathology Laboratory of Seoul National University, Seoul, South Korea (Horwitz et al., 1999; Lee et al., 2008). To generate a CsPOM1-GFP vector, a 5.8-kb PCR product including 1.7 kb of the 5’-flanking promoter region and CsPOM1 gene sequence was amplified from WT genomic DNA using the primers GFP_Pom1_F/GFP_Pom1_R (Supplementary Table S1). The pIGPAPA vector containing a GFP gene was linearized by PCR amplification using the primers GFP_Pom1_VF/GFP_Pom1_VR. The amplified 5.8-kb PCR product was cloned into the linearized pIGPAPA vector using an overlap DNA Cloning Kit (Elpis Biotech, Daejeon, South Korea).
Generation of Knockout Mutant and Gene Expression Analysis
Oligonucleotide primers used for PCR reactions are listed in Supplementary Table S1. Genomic DNA was extracted according to a standard method (Sambrook et al., 1989). Approximately 1.5 kb fragments of upstream and downstream of CsPOM1 were amplified from WT genomic DNA using primers 5F/5R and 3F/3R, respectively. The amplified upstream and downstream fragments of CsPOM1 were fused to the 1.5-kb hyg cassette amplified from plasmid pBCATPH via the double-joint PCR method (Yu et al., 2004; Choi et al., 2009). The resultant fusion was finally amplified using primers NF/NR and used as a knockout construct. Protoplast generation and transformation of C. scovillei were carried out according to previous work (Shin et al., 2019). Targeted gene deletion was confirmed with Southern blot hybridization and RT-PCR. For complementation, CsPOM1 fragments including 1.9 kb upstream and 600 bp downstream were amplified from WT genomic DNA using primers CsPom1_cmF/R. The amplified fragments were transformed into protoplasts of CsPOM1 deletion mutant with plasmid pII99 containing a geneticin resistance gene (gen) cassette (Yi et al., 2009).
For quantitative PCR (qPCR), total RNA was extracted using the Easy-Spin Total RNA Extraction Kit (Intron Biotechnology, Seoul, South Korea) from frozen fungal tissues according to the manufacturer’s instructions. First-strand cDNA was synthesized with 5 µg of total RNA using the oligo (dT) primer with the SuperScriptTM III First-Strand Synthesis System kit (Invitrogen™ Life Technologies, CA, USA). qPCR was performed on the StepOne Real-Time PCR System (Applied Biosystems, CA, USA) using the HIPI Real-Time PCR 2x Master Mix (Elpis, Daejeon, South Korea) as previously described (Han et al., 2015). Ct values were normalized to that of β-tubulin (CAP_007327) and expressed as relative values, with 1 corresponding to WT (Kim et al., 2009). Experiments were conducted in triplicate and repeated three times.
Microscopy Methods
C. scovillei photographs were routinely obtained using a Carl Zeiss Axio Image A2 microscope (Carl Zeiss Microscope Division, Oberkochen, Germany) equipped with differential interference contrast (DIC) and fluorescence optics. Iodine solution (60 mg of Kl and 10 mg of l2/ml of sterile distilled water) was used to stain the glycogen in conidia (Thines et al., 2000). To stain nuclei, diamidino-2-phenylindole (DAPI) (Sigma-Aldrich, USA) was dissolved in sterile distilled water to yield a 10-µg/ml staining solution (10×) (Miyakawa et al., 1984). Conidial septa were stained with a combination of Calcofluor White (1 mg of Calcofluor White M2R and 0.5 mg of Evans blue) (Sigma-Aldrich, USA) and 10% potassium hydroxide (KOH) in a 1:1 ratio.
Phenotype Analysis
Vegetative growth was evaluated by measuring the diameter of colonies at 6 days postinoculation on CM agar. To assess the role of CsPOM1 in hydrophobicity of aerial hyphae, 10 µl drops of water or 0.1% sodium dodecyl sulfate (SDS), respectively, were placed on the surface of the CsPOM1 deletion mutant grown on V8 agar for 5 days in the dark. Conidiation was measured by counting the number of conidia harvested with 5 ml of sterile distilled water from 6-day-old V8 agar under continuous light, using a hemocytometer. Conidial morphology was observed under a microscope, and the conidial length was measured using the ZEN imaging software. Conidial germination and appressorium formation were measured after 6 and 16 h postinoculation of conidial drops (5 × 104 conidia/ml) on coverslips, respectively. To observe appressorium formation from hyphal tips, mycelial agar plugs were obtained from 3-day-old OMA plates and placed on slide glasses. The mycelial agar plugs were covered with coverslips and incubated for 3 days in a moistened box.
Infection Assays
For appressorial penetration and invasive growth assays, conidial drops (5 × 104 conidia/ml) collected from 5-day-old OMA were inoculated onto the surface of green pepper fruits and incubated in a moistened box. To visualize invasive hyphae, we used GFP-expressing strains generated with the pIGPAPA vector. The fruit surface was peeled off using a razor blade as thin as possible, and the appressorial penetration and invasive growth were examined under a microscope. For the disease development assay, conidial drops (15 × 104 conidia/ml) collected from 5-day-old OMA were inoculated onto the surface of pepper fruits and incubated for 14 days in a moistened box. All experiments were repeated three times with three replicates.
Results
Identification of CsPOM1 in C. scovillei KC05
We identified CsPOM1 (locus CAP_000731), a homolog of Schizosaccharomyces pombe POM1, through BLAST searches conducted using the Comparative Fungal Genomics Platform (Bähler and Pringle, 1998; Park et al., 2006). The CsPOM1 gene was predicted to encode a protein of 1,345 amino acids with a putative protein kinase catalytic domain near the C-terminus, and the protein shared 67.4% (positives 80.9%) amino acid identity with the S. pombe Pom1 in their kinase domains. Pom1 is a DYRK subfamily member (Aranda et al., 2011). Several members of the DYRK subfamily, including DYRK1A, DYRK1B (Mirk), DYRK2, DYRK3 (REDK), DYRK4, Yak1, and Pom1, have been identified in eukaryotes (Garrett et al., 1991; Bähler and Pringle, 1998; Goyard et al., 2008; Aranda et al., 2011). Among them, Pom1 and Yak1 were found in fungi (Garrett et al., 1991; Bähler and Pringle, 1998; Goyard et al., 2008). Phylogenetic analysis of CsPOM1 and related proteins in other fungal species revealed that Pom1 homologs form a separate lineage distinct from Yak1 homologs (Supplementary Figure S1A). Pom1 homologs of C. higginsianum, Fusarium oxysporum, M. oryzae, N. crassa, Botrytis cinerea, A. nidulans, and S. pombe showed 98.0%, 93.3%, 97.7%, 95.3%, 90.6%, and 84.9% amino acid identity, respectively, to CsPOM1 in their kinase domains (Supplementary Figure S1B). Domain structure analysis confirmed that all DYRKs contain a DYRK homology (DH) box that regulates kinase activity (Becker and Joost, 1998) in the N-terminal of the catalytic domain (Supplementary Figure S1B).
Generation of the ΔCspom1 mutant
We generated targeted gene deletion mutant ΔCspom1 to characterize the functions of CsPOM1 in fungal development and pathogenicity (Supplementary Figure S2A). Approximately 120 putative deletion transformants for the CsPOM1 gene were selected on TB3 agar containing hygromycin B (200 ppm). Among the putative transformants, five candidates were selected by screening PCR, and the ΔCspom1 mutant was finally selected based on Southern blot hybridization (Supplementary Figure S2B). RT-PCR confirmed that the expression of the CsPOM1 gene was completely abolished in the deletion mutant (Supplementary Figure S2C). The expression of the CsPOM1 gene was restored in the complemented strain.
Vegetative Growth of the ΔCspom1 Mutant
To investigate the roles of CsPOM1 in the vegetative growth of C. scovillei, we measured the vegetative growth of the wild-type (WT) and ΔCspom1 mutant on CM agar. After 6 days of incubation, the ΔCspom1 mutant exhibited reduced vegetative growth compared with WT (20% reduction in average) (Figure 1A). Tolerance to environmental stress is important for the normal growth of fungi (Huang et al., 2017). To investigate whether CsPOM1 is involved in stress tolerance, we inoculated mycelial agar plugs of the WT and mutant on CM agar supplemented with an osmotic stress agent, followed by incubation for 6 days. Relative growth was compared with WT on CM agar without supplements. The vegetative growth of mutant on CM agar supplemented with 0.5 M NaCl or 0.5 M KCl (osmotic stress) was significantly reduced compared with WT (38% and 41% reduction, respectively), which exhibits more severe reductions than that on CM agar without supplements (20% reduction) (Figure 1A). The results indicate that CsPOM1 is involved in the tolerance to osmotic stress of C. scovillei.
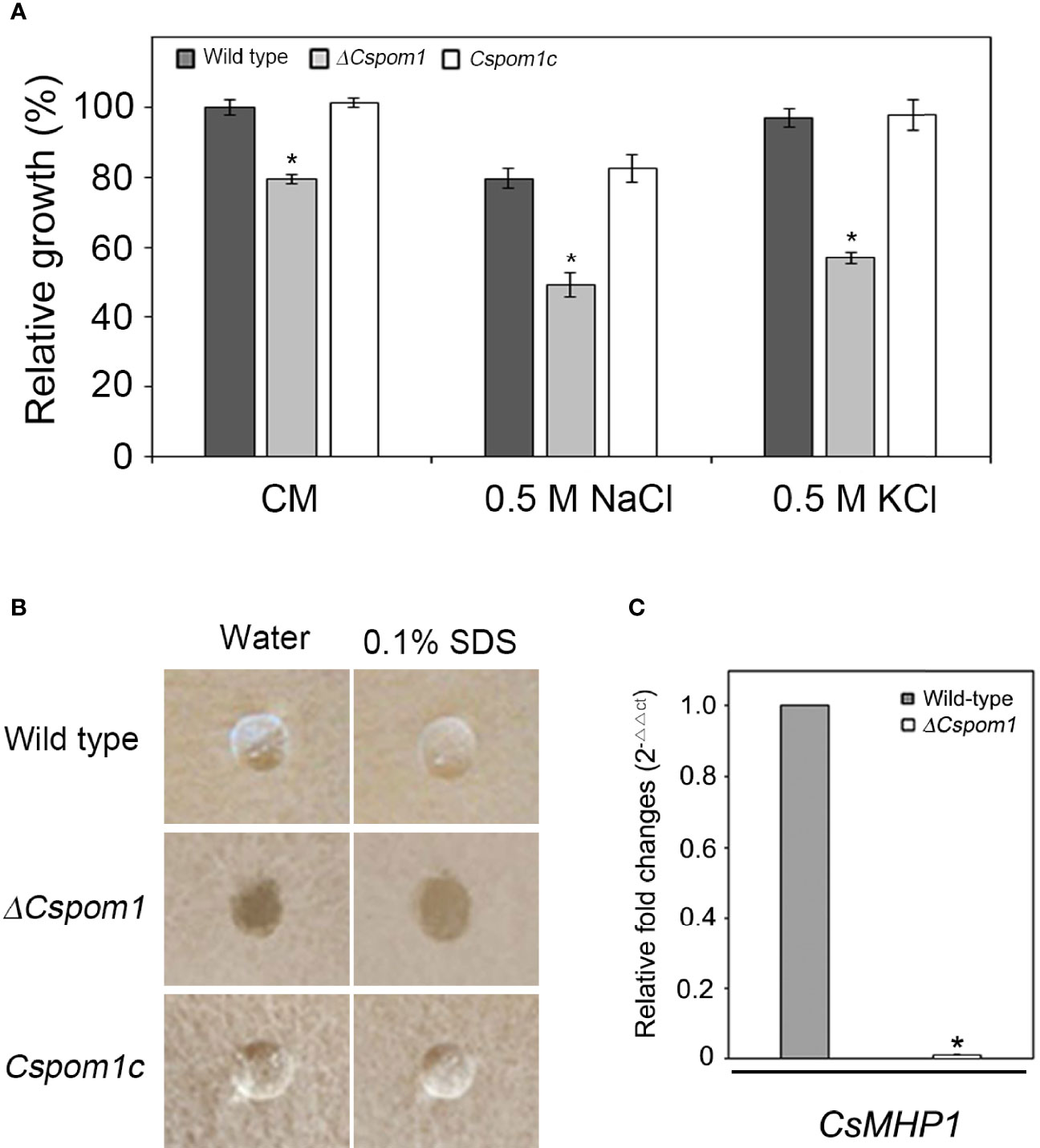
Figure 1 Vegetative growth and surface hydrophobicity of the ΔCspom1 mutant. (A) Fungal colonies were grown on CM agar with and without an osmotic stress agent (0.5 M NaCl or KCl) for 6 days at 25°C in the dark. Relative growth was compared with WT grown on CM agar. Asterisk indicates significant difference between WT and mutant according to Tukey’s test at p < 0.05. (B) First, 10 µl water or 0.1% SDS were placed on the surface of each strain grown on V8 agar for 5 days in the dark. (C) Measurement of the expression of the CsMHP1 gene encoding a putative class II hydrophobin (CAP_004537) in the ΔCspom1 mutant. Expression of the CsMHP1 gene was measured by qPCR, normalized to β-tubulin, and expressed as a relative value of 1 in the WT.
Surface Hydrophobicity of the ΔCspom1 Mutant
To assess the role of CsPOM1 in the surface hydrophobicity of C. scovillei, 10 µl drops of water or 0.1% SDS, respectively, were placed on the surface of WT and ΔCspom1 mutant grown on V8 agar for 5 days. We found that the drops of water and 0.1% SDS were suspended on the aerial hyphae of the WT, whereas they were immediately soaked into the mycelia of ΔCspom1 (Figure 1B). We identified a putative class II hydrophobin gene CsMHP1 (CAP_004537) in C. scovillei, a homolog of M. oryzae MHP1 (MG01173) sharing 46.1% amino acid identity (Kim et al., 2005), and compared the relative gene expression by quantitative PCR (qPCR). We found that expression of the CsMHP1 gene was significantly reduced in the ΔCspom1 mutant compared to WT (Figure 1C). Collectively, these results indicate that CsPOM1 is required for surface hydrophobicity, which is likely associated with the expression of the CsMHP1 gene.
CsPOM1 Is Important for Conidial Production, Conidial Morphology, and Glycogen Metabolism
Conidial production of the ΔCspom1 mutant was quantified on V8 agar. Quantitative analysis of conidial production showed that the ΔCspom1 mutant produced significantly fewer conidia than WT (Figure 2A). This phenotype of the ΔCspom1 mutant was rescued in complemented strain Cspom1c. Next, we collected conidia of the mutants from 10-day-old OMA and examined the conidial morphology. The ΔCspom1 mutant produced longer conidia (15.3 ± 0.17 in length) than those of the WT (10.3 ± 0.16 μm in length) (Figure 2B). In addition, approximately 34% of the ΔCspom1 mutant conidia were slightly bent, while most of the WT conidia were straight in shape (Figures 2B, C). Collectively, these data indicate that CsPOM1 is involved in the regulation of conidial production and conidial size.
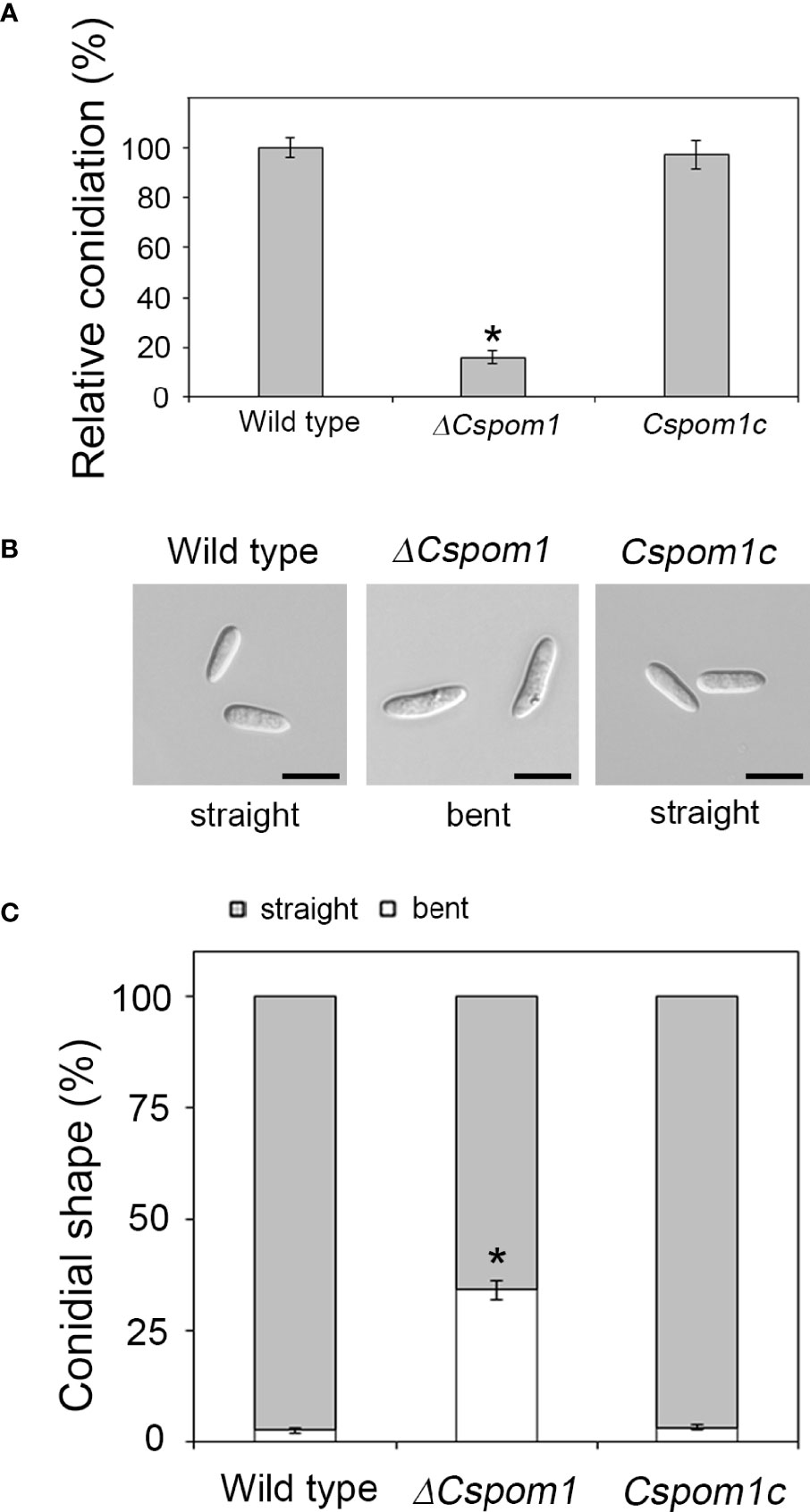
Figure 2 Abnormal conidial production of the ΔCspom1 mutant. (A) Quantitative measurement of conidia at 6 days postinoculation on V8 agar at 25°C under continuous light. Asterisk indicates a significant difference at p < 0.05 according to Tukey’s test. (B) Conidial morphology of the ΔCspom1 mutant. Scale bars, 10 µm. (C) Quantitative comparison of abnormal conidia (bent shape). Conidia were harvested from 10-day-old OMA. The shape of the conidia was observed under a microscope. Asterisk indicates a significant difference at p < 0.05 according to Tukey’s test.
We also examined glycogen deposits in conidia. Iodine staining of 10-day-old conidia revealed that only 71% of the ΔCspom1 mutant conidia contained glycogen, compared with more than 90% of WT conidia (Figures 3A, B). Longer incubation (up to 20 days) further decreased the rate of glycogen-containing conidia, down to 29% in the ΔCspom1 mutant, while most of the WT conidia still contained glycogen (Figures 3A, B). We identified a putative glycogen debranching gene in C. scovillei, i.e., CsGDB1, which is a homolog of S. cerevisiae GDB1 (AJW05075) sharing 47.0% amino acid identity, and compared the relative gene expression during vegetative growth in CM broth by qPCR. The qPCR analysis confirmed that expression of the glycogen debranching gene (CAP_006079) was significantly higher in the ΔCspom1 mutant compared with WT (Figure 3C). Taken together, these results indicate that CsPOM1 is involved in the regulation of glycogen metabolism in a conidial age-dependent manner.
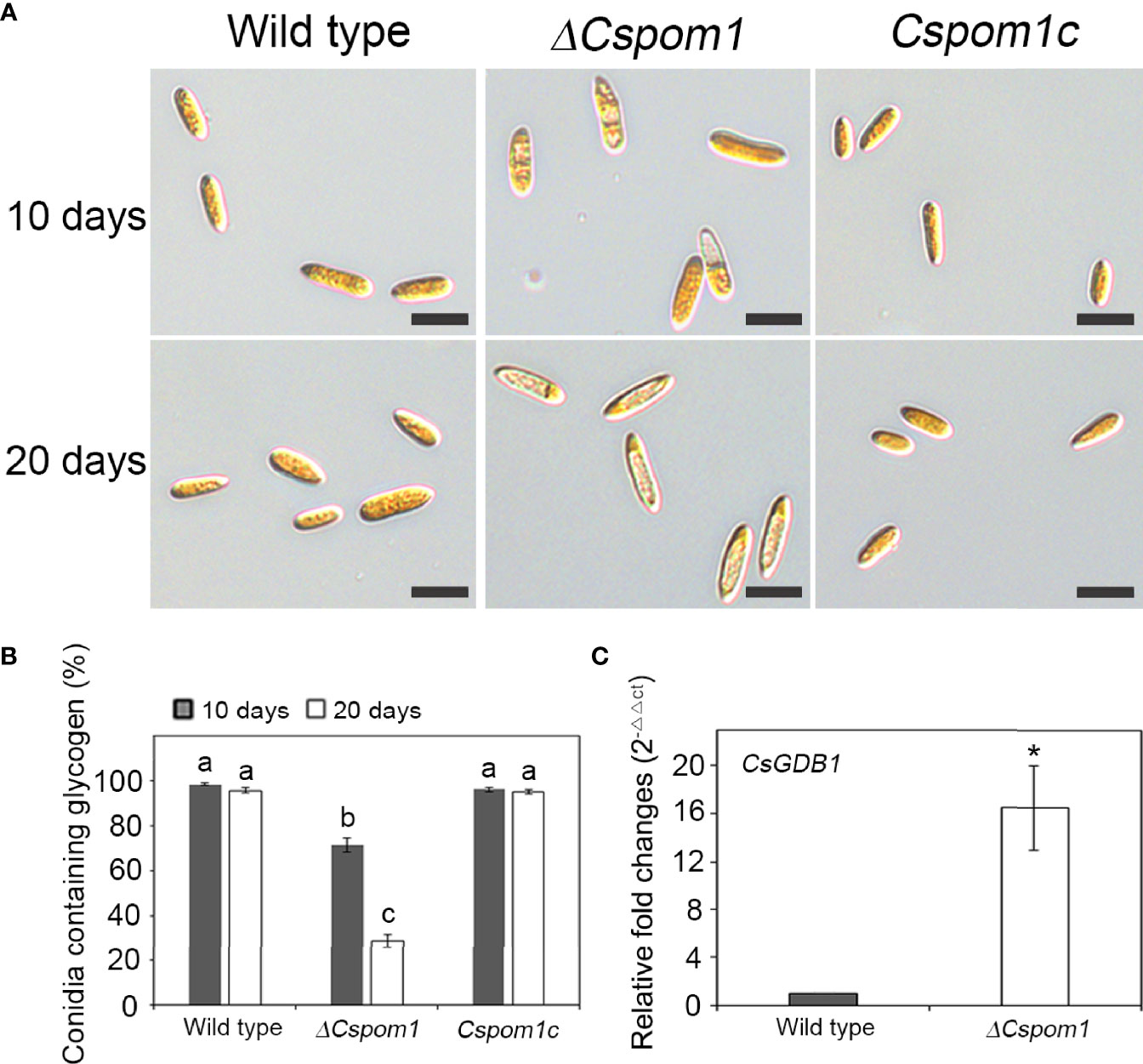
Figure 3 Glycogen metabolism of the ΔCspom1 mutant in conidia. (A) Conidia harvested from 10- or 20-day-old OMA were stained with iodine solution (2×, 60 mg of Kl and 10 mg of l2/ml of sterile distilled water). Scale bars, 10 µm. (B) Quantitative measurement of conidia containing glycogen. Different letters on bars indicate significant differences at p < 0.05 according to Tukey’s test. (C) Measurement of the expression of the CsGDB1 gene encoding a putative glycogen debranching enzyme (CAP_006079) in the ΔCspom1 mutant. Expression of the CsGDB1 gene was measured by qPCR, normalized to β-tubulin, and expressed as a relative value of 1 in the WT. Asterisk indicates a significant difference between the WT and mutant at p < 0.05 according to two-tailed t-test.
CsPOM1 Regulates Conidial Cell Division and Is Localized in the Region of Septum Formation
Pom1 kinase regulates cell division in the fission yeast S. pombe (Bähler and Pringle, 1998). Therefore, we examined conidial septation and nuclear division during appressorium formation on hydrophobic coverslips. The WT conidia were aseptate and uninucleate at 0 h, which was visualized by Calcofluor White (Figure 4A) and DAPI staining (Figure 4B), respectively. By 2 h, the conidia formed two uninuclear cells separated by a septum, and a single germ tube emerged (Figure 4B). The tip of the germ tube began to swell and produced an appressorium. A second round of nuclear division started, resulting in another nucleus in the appressorium by 8 h (Figure 4B). However, approximately 32% of the ΔCspom1 conidia were already septate (Figure 4A) and two-celled (Figure 4B) at 0 h, in which most of the two-celled conidia were slightly bent in shape. These results suggest that CsPOM1 may regulate conidial cell division in C. scovillei.
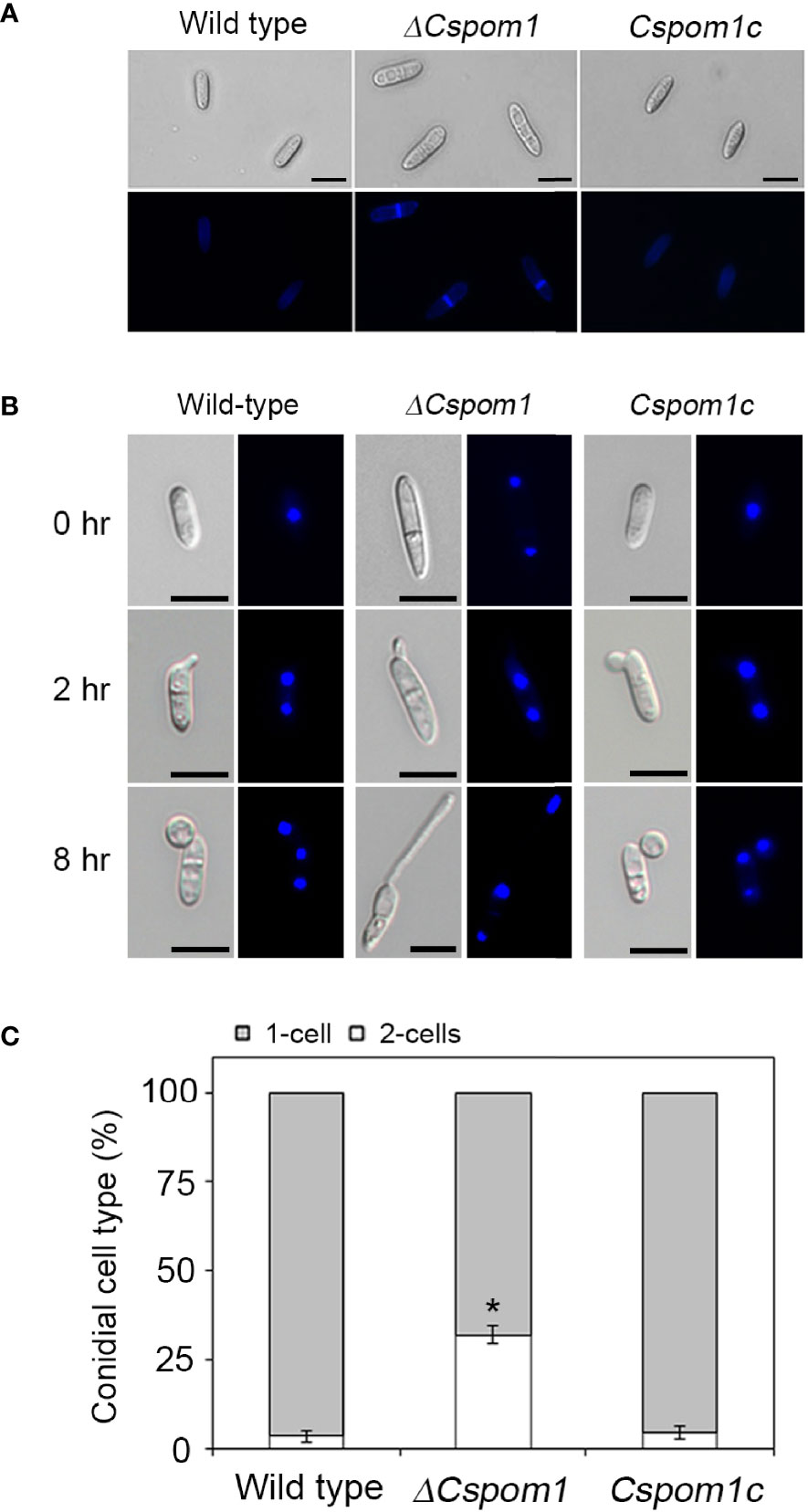
Figure 4 Conidial cell division of the ΔCspom1 mutant. (A) Septation of conidia on coverslips (0 h). The conidial septa were stained with Calcofluor White (1 mg Calcofluor White M2R and 0.5 mg Evans blue). Scale bars, 10 µm. (B) Nuclear division during conidial germination and appressorium development on coverslips. The conidial nuclei were stained with DAPI (1 µg/ml). Scale bars, 10 µm. (C) Quantitative comparison of the number of conidial cells (one- or two-celled) between the WT and mutant. Experiments were conducted in triplicate and repeated three times (n ≥ 100 conidia per strain). Asterisk indicates a significant difference according to Tukey’s test at p < 0.05.
Next, we tagged the 3’ end of CsPOM1 with sequences encoding a green fluorescent protein (GFP) and transformed the construct into WT protoplasts to investigate the intracellular localization of CsPOM1. Conidia of the WT transformant were inoculated on hydrophobic coverslips, and the GFP-tagged Pom1 was monitored. The fluorescent analysis demonstrated that CsPom1-GFP was localized to the nucleus in conidia (Figure 5A). The GFP signal at the nucleus in conidia became weaker during germination (Figure 5B). CsPOM1-GFP was also observed in the region of septum formation in conidia at 30 min postinoculation (Figure 5B). The GFP signal was very faint and became weaker during germination (Figure 5B).
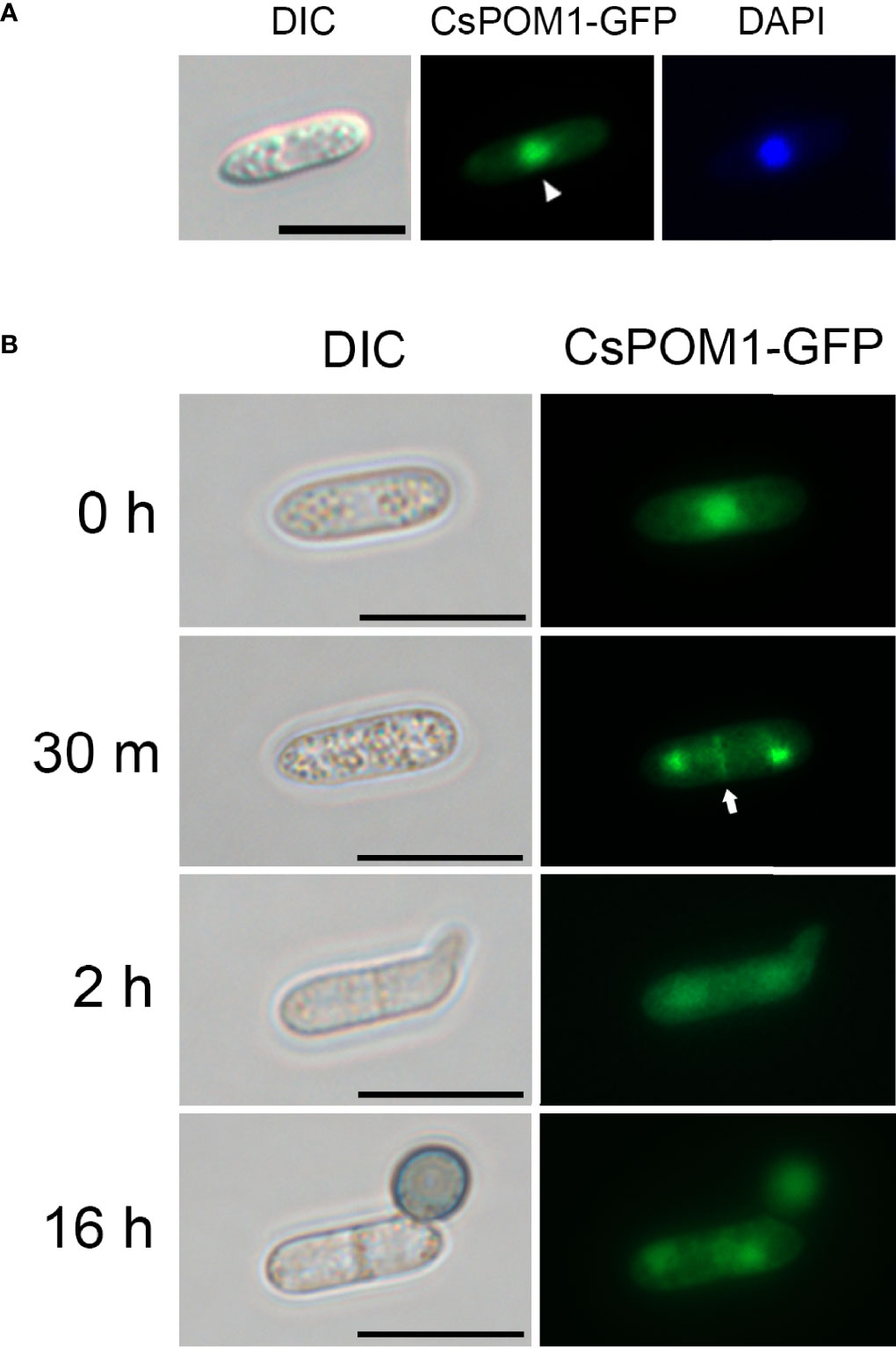
Figure 5 Intracellular localization of CsPom1-GFP fusion. Wild-type conidia expressing CsPom1-GFP fusion were inoculated on coverslips and incubated in a moistened box. (A) Nuclear localization of CsPOM1-GFP fusion. The conidial nuclei were stained with DAPI (1 µg/ml). Scale bar,10 µm. (B) Localization of CsPOM1-GFP fusion during conidial germination and appressorium formation. Arrow indicates the region of septum formation in conidia. Scale bars, 10 µm.
CsPOM1 Is Essential for Appressorium Formation in Conidia and Hyphal Tips
To investigate the functional role of CsPOM1 in appressorium formation, we collected conidia of the WT and mutants (5 × 105 conidia/ml) from 6-day-old OMA plates and inoculated conidial drops on coverslips. The experiment revealed that the ΔCspom1 mutant was normal in germination but defective in appressorium formation on hydrophobic coverslips (Figures 6A, B). At 16 h postinoculation, approximately 90% of the WT conidia formed melanized appressoria (Figures 6B, C). However, only 8% of the ΔCspom1 mutant conidia formed appressoria (Figures 6B, C). Similarly, appressorium formation from hyphal tips was significantly reduced in the ΔCspom1 mutant (Figure 6D). These results suggest that CsPOM1 is essential for appressorium formation from germ tube and hyphal tips. Treatment of conidia with synthetic cutin monomers or exogenous cyclic adenosine monophosphate (cAMP) is a method to induce appressorium formation in some fungi (Lee and Dean, 1994; Fu et al., 2021). We treated ΔCspom1 mutant conidia with 10 μM cutin monomers and 5 mM exogenous cAMP, respectively. The cutin monomer treatment partially restored appressorium formation of the ΔCspom1 mutant (Figures 6B, C). In contrast, the cAMP treatment did not restore appressorium formation of the ΔCspom1 mutant (Supplementary Figure S3), which indicates that CsPOM1 functions downstream of cAMP or in a cAMP-independent manner.
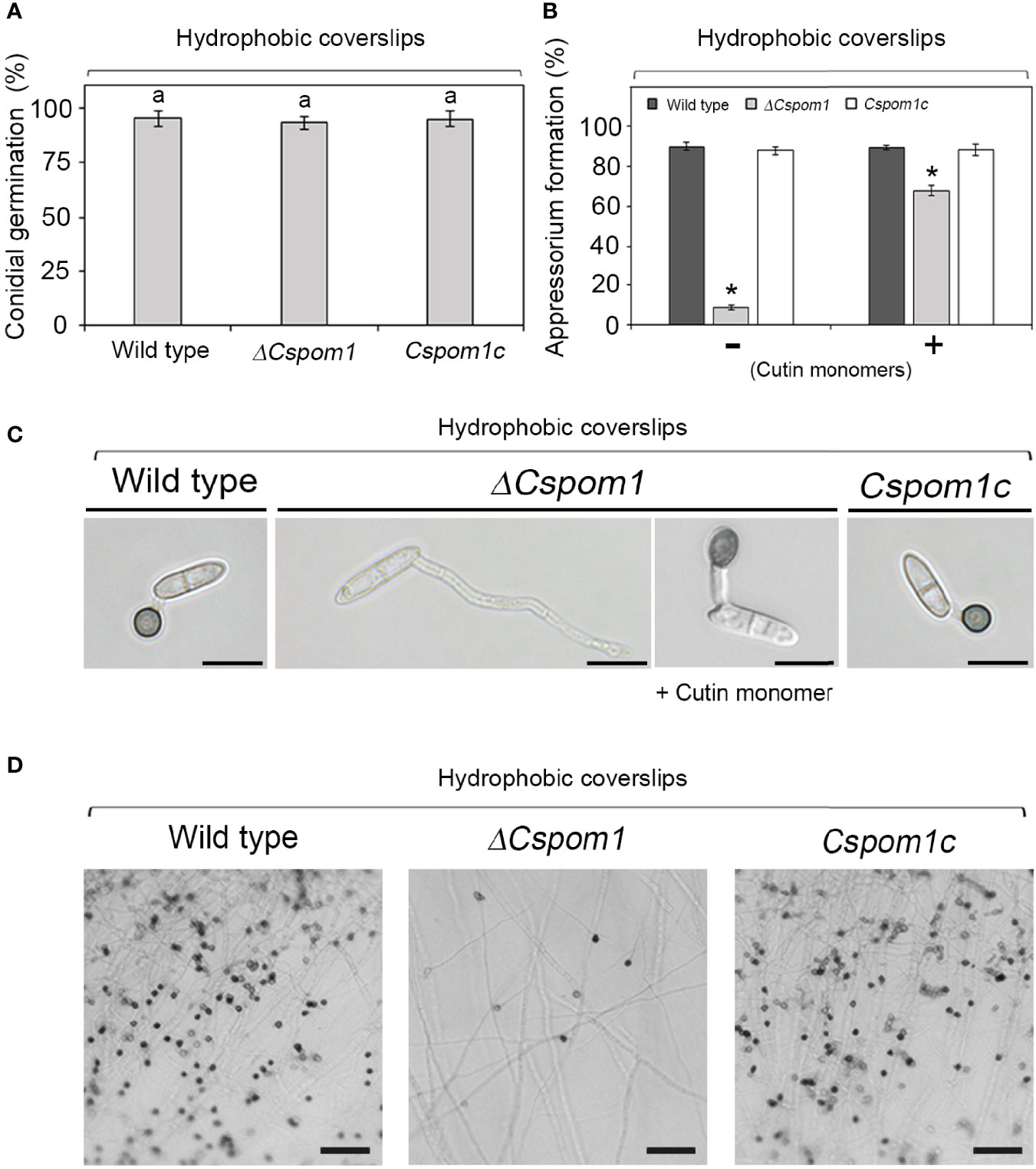
Figure 6 Appressorium formation of the ΔCspom1 mutant. (A) Quantitative measurement of conidial germination at 6 h postinoculation on coverslips. Experiments were conducted in triplicate and repeated three times (n ≥ 100 conidia per strain). The same letters on bars indicate there was no significant difference according to Tukey’s test. (B) Quantitative measurement of appressorium formation of conidia with or without 10 μM cutin monomers at 16 h postinoculation on coverslips. Experiments were conducted in triplicate and repeated three times (n ≥ 100 conidia per strain). Asterisks indicate significant differences according to Tukey’s test at p < 0.05. (C) Appressorium formation of conidia on coverslips. Scale bars, 10 µm. (D) Appressorium formation from hyphal tips. Mycelial agar plugs from 3-day-old OMA were placed on slide glasses and covered with coverslips. Photographs were taken at 3 days postinoculation on coverslips. Scale bars, 50 µm.
CsPOM1 Is Required for Early Infection
Next, we investigated the roles of CsPOM1 in fungal virulence on pepper fruit. We inoculated conidial drops (15 × 104 conidia/ml) collected from 5-day-old OMA plates onto the surface of pepper fruits (C. annuum L. cv. Gilsang) and incubated them in a moistened box. After 2 weeks, the WT caused typical anthracnose lesions on the pepper fruits, while the ΔCspom1 mutant produced tiny lesions on the fruits (Figure 7A). To investigate initial pepper fruit infection by the mutant, we generated GFP-expressing transformants and inoculated conidia on pepper fruits. Most of the WT conidia (>90%) formed appressoria on the pepper fruits, penetrated the plant cuticle by 24 h (Figure 7B), and formed a primary hypha in the plant epidermal cell by 72 h (Figure 7C). Unlike the appressorium formation on coverslips (Figure 6B), most of the ΔCspom1 conidia (>80%) formed appressoria on the pepper fruits by 24 h, although there was a slight reduction in appressorium formation compared with the WT (Supplementary Figure S4). However, the mutant conidia exhibited delayed penetration and invasive growth during infection compared with WT (Figures 7B, C). Collectively, these results suggest that CsPOM1 is involved in penetration and invasive growth during early infection on pepper fruits.
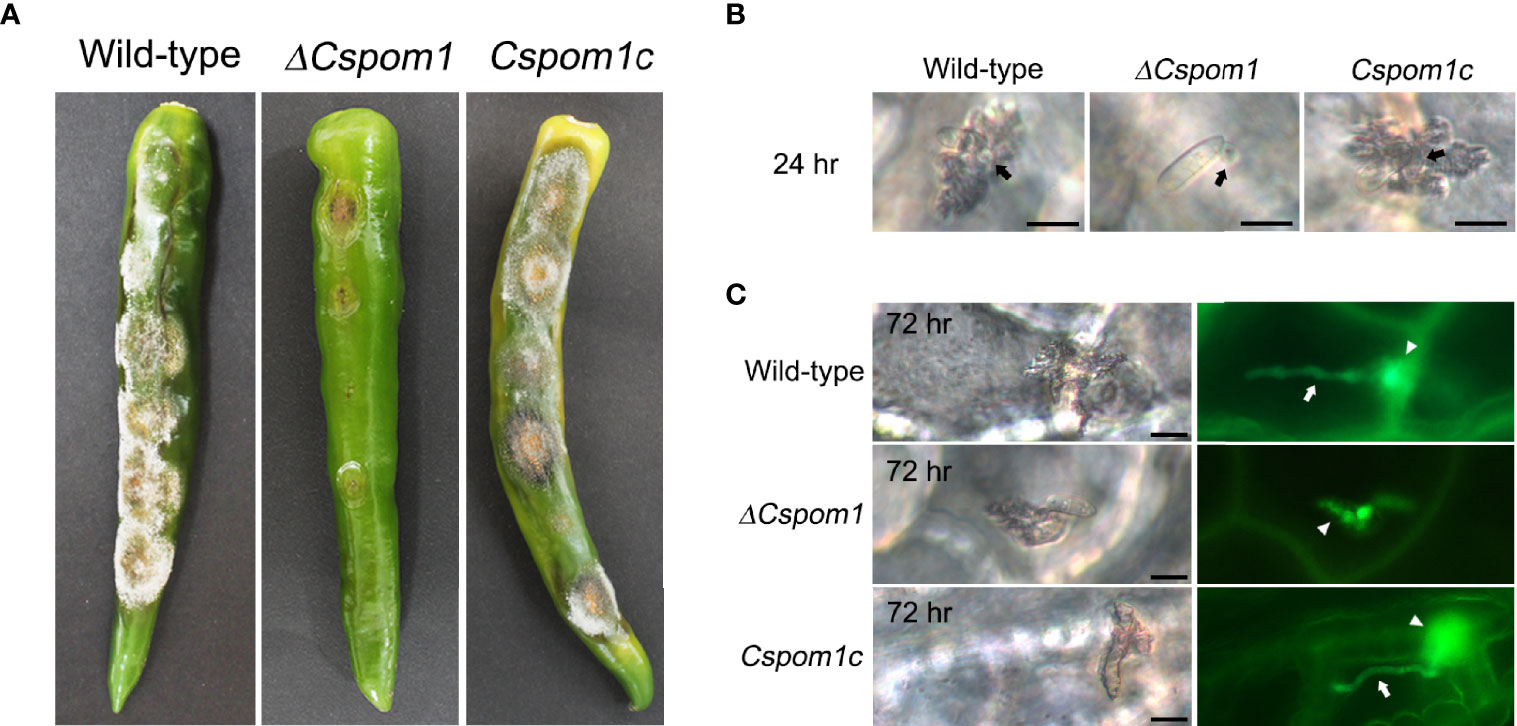
Figure 7 CsPom1 is important for plant penetration and invasive growth. (A) Conidial drops (15 × 104 conidia/ml) of WT and ΔCspom1 mutant were inoculated onto the surface of green pepper fruits (Capsicum annuum L. cv. Gilsang). Photographs were taken at 14 days postinoculation. (B) Green pepper fruits were inoculated with conidial drops (5 × 104 conidia/ml) of the WT and ΔCspom1 mutant. Photographs were taken at 24 h postinoculation (B) and 72 h postinoculation (C). Black arrows indicate appressorium. White arrows indicate primary hypha in a plant epidermal cell. White arrowheads indicate fungal hypha in plant cuticle. Scale bars, 10 µm.
Discussion
The DYRK kinase Pom1 is well-known to provide positional information for cell division in the fission yeast S. pombe, but the roles of Pom1 in filamentous fungi are not well characterized. In this study, we functionally characterized CsPOM1, a homolog of S. pombe POM1, in the anthracnose fungus C. scovillei. The ΔCspom1 mutant exhibited reduced vegetative growth on CM agar compared with the WT (Figure 1A). In the fission yeast S. pombe, deletion of POM1 results in failure of switching from unipolar to bipolar growth (Bähler and Pringle, 1998). In the filamentous fungus F. graminearum, the vegetative growth of the Fgpom1 mutant was slightly reduced on potato dextrose agar media (Wang et al., 2011). Thus, our results agree with previous reports that POM1 is involved in cell growth. In addition, the growth defect of the ΔCspom1 mutant became more severe when it was exposed to osmotic stress (NaCl or KCl). This suggests that CsPOM1 is involved in osmoregulation, which might affect the basic growth phenotype of the ΔCspom1 mutant.
Hydrophobins are small, hydrophobic proteins that provide a hydrophobic coating on aerial hyphae and conidia in fungi (Wessels et al., 1991; Kim et al., 2005; Pham et al., 2016). The hydrophobic surfaces of fungal pathogens are associated with conidial production, and the conidia surfaces are coated to reduce wettability and promote surface interaction (Pham et al., 2016). In M. oryzae, deletion of MHP1, a class II hydrophobin gene, results in a loss of surface hydrophobicity and reduction in conidiation (Kim et al., 2005). In this study, the ΔCspom1 mutant exhibited a loss of hydrophobicity, reduced conidiation, and reduced expression of CsMHP1 compared with WT (Figures 1, 2). Thus, we suggest that CsPOM1 is involved in the transcriptional regulation of the CsMHP1 gene in C. scovillei, in which the reduced expression of the CsMHP1 gene caused the defects in surface hydrophobicity and conidiation in the ΔCspom1 mutant.
Conidia of C. scovillei were uninucleated single cells that became two-celled just before germination and differentiated into an appressorium with one nucleus (Figures 4A, B). In the fission yeast S. pombe, Pom1 forms a polar gradient at the cell ends inhibiting Cdr2 activity, which causes a delay in mitotic entry (Moseley et al., 2009; Gerganova et al., 2019). Deletion of POM1 in S. pombe results in premature entry into mitosis (Moseley et al., 2009). Consistently, approximately 32% of the conidia produced by the ΔCspom1 mutant were already two-celled before inoculation of the conidia on an inductive surface (Figure 4A–C). Thus, we suggest that CsPOM1 has an important role in the negative regulation of cell division in C. scovillei. In S. pombe, Pom1 is localized at both cell ends and also localized to the cell division site (Bähler and Pringle, 1998). As cell division proceeds, the concentration of Pom1 at both cell ends decreases while the concentration at the cell center increases (Bähler and Pringle, 1998; Celton-Morizur et al., 2006). In our study, we found that CsPOM1-GFP fusion is localized in the region of septum formation in conidia after a 30-min incubation on hydrophobic coverslips, which is approximately the time at which nuclear division starts (Figure 5B). Consistent with S. pombe Pom1, this suggests that CsPOM1 provides positional information for cell division in C. scovillei. Unlike the S. pombe Pom1, the localization of CsPOM1-GFP fusion in the nuclei of ungerminated conidia were noticeable (Figure 5A), although some DYRK family kinases, including DYRK1A and Yak1, are localized to the nuclei (Moriya et al., 2001; Kaczmarski et al., 2014; Han et al., 2015). This may reflect divergent roles for CsPOM1 in the filamentous fungus, as shown in pleiotropic defects of the ΔCspom1 while the cellular roles of the protein await elucidation.
Glycogen is one of the major energy stores in fungi (Talbot, 1995). Glycogen is utilized during conidial germination and appressorium development, and is involved in the supply of energy during infection in fungi (Thines et al., 2000; Badaruddin et al., 2013). In our study, glycogen deposits in conidia of the ΔCspom1 mutant were already degraded before conidial germination (Figures 3A, B). In addition, the mutant exhibited delayed plant penetration, resulting in tiny and restricted lesions on pepper fruits (Figure 7). We found that expression of the CsGDB1 gene in the ΔCspom1 mutant was significantly increased compared with WT (Figure 3C). Thus, it is likely that CsPOM1 acts as a negative regulator inhibiting the expression of the glycogen debranching gene, CsGDB1 in C. scovillei, which is likely associated with the abnormal glycogen degradation and reduced virulence in the ΔCspom1 mutant.
Many phytopathogenic fungi recognize various host signaling factors including surface hydrophobicity, cutin monomers, and leaf waxes to develop appressoria (Adachi and Hamer, 1998; Kang et al., 1999; Dickman et al., 2003). In this study, the ΔCspom1 mutant exhibited significantly reduced appressorium formation on hydrophobic coverslips, but the defect was restored on the plant surface (Supplementary Figure S4) and by cutin monomer treatment (Figure 6). Therefore, we think that CsPOM1 contributes to the recognition of hydrophobic surface for appressorium formation in C. scovillei. In rice blast fungus M. oryzae, targeted deletion of class I hydrophobin MPG1 or class II hydrophobin MHP1 results in reduced appressorium formation on hydrophobic surface, and infectious growth in plant cells is significantly limited (Talbot et al., 1993; Kim et al., 2005). The addition of cAMP restored appressorium formation in the MPG1 deletion mutant, but not in the MHP1 deletion mutant, indicating that Mhp1 may function downstream of cAMP for appressorium formation in M. oryzae (Talbot et al., 1993; Kim et al., 2005). In our study, the addition of cAMP did not restore appressorium formation in the ΔCspom1 mutant (Supplementary Figure S3). Considering that the ΔCspom1 mutant exhibited significantly reduced expression of the CsMHP1 gene, CsPOM1 may function downstream of cAMP or a cAMP-independent manner for appressorium formation in C. scovillei. On pepper fruits, the ΔCspom1 mutant was able to form appressoria, probably via the sensing of signals on the plant surface, but the mutant showed a delay in penetration and infectious growth in plant cells (Figure 7). Therefore, the reduction of ΔCspom1 in virulence may be related to the defects in plant penetration and growth of invasive hyphae after penetration. POM1 has not been studied in the context of appressorium formation in other fungi, but the YAK1 kinase gene, belonging to the DYRK subfamily with POM1, is required for appressorium formation on the hydrophobic surface in M. oryzae (Han et al., 2015). Similar to the ΔCspom1 mutant, the ΔMoyak1 mutant in M. oryzae was able to form appressoria on the plant surface, but the appressoria were defective in plant penetration. Thus, these two genes belonging to the DYRK subfamily appear to have similar roles in the appressorium formation of phytophathogic fungi.
Based on the pleiotropic roles and nuclear localization of CsPOM1, we speculate that CsPOM1 may play diverse roles in phosphorylating regulators in signaling pathways for fungal development and metabolism. Supportively, a recent study reported that PomA regulates the HOG MAPK pathway in A. nidulans (Zhou et al., 2019). Two HOG MAPKs, SakA and MpkC, regulate the expression of gdbA, a glycogen debranching gene, during glycogen metabolism in A. fumigatus (de Assis et al., 2018). Our study revealed that CsPOM1, a DYRK kinase gene, plays important roles in fungal morphogenesis and disease development in C. scovillei, which provide fundamental basis to understand anthracnose diseases on fruits caused by Colletotrichum species.
Data Availability Statement
The raw data supporting the conclusions of this article will be made available by the authors, without undue reservation.
Author Contributions
Conceived and designed the experiments: JHS, HYK, TF, KHL, KSK. Performed the experiments: JHS, HYK, TF, KHL. Analyzed the data: JHS, HYK, TF, KSK. Wrote the paper: JHS, KSK. Originated research leading up to this paper and provided guidance and review: JHS, HYK, KSK.
Funding
This study was supported by Basic Science Research Program through the National Research Foundation of Korea grant (NRF-2020R1A2C100550700) funded by the Ministry of Education, Science and Technology.
Conflict of Interest
The authors declare that the research was conducted in the absence of any commercial or financial relationships that could be construed as a potential conflict of interest.
Publisher’s Note
All claims expressed in this article are solely those of the authors and do not necessarily represent those of their affiliated organizations, or those of the publisher, the editors and the reviewers. Any product that may be evaluated in this article, or claim that may be made by its manufacturer, is not guaranteed or endorsed by the publisher.
Supplementary Material
The Supplementary Material for this article can be found online at: https://www.frontiersin.org/articles/10.3389/fcimb.2022.861915/full#supplementary-material
References
Adachi, K., Hamer, J. E. (1998). Divergent cAMP Signaling Pathways Regulate Growth and Pathogenesis in the Rice Blast Fungus Magnaporthe Grisea. Plant Cell 10, 1361–1373. doi: 10.1105/tpc.10.8.1361
Aranda, S., Laguna, A., Luna, S. D. L. (2011). DYRK Family of Protein Kinases: Evolutionary Relationships, Biochemical Properties, and Functional Roles. FASEB J. 25, 449–462. doi: 10.1096/fj.10-165837
Asakura, M., Ninomiya, S., Sugimoto, M., Oku, M., Yamashita, S. I., Okuno, T., et al. (2009). Atg26-Mediated Pexophagy is Required for Host Invasion by the Plant Pathogenic Fungus Colletotrichum Orbiculare. Plant Cell 21, 1291–1304. doi: 10.1105/tpc.108.060996
Badaruddin, M., Holcombe, L. J., Wilson, R. A., Wang, Z.-Y., Kershaw, M. J., Talbot, N. J. (2013). Glycogen Metabolic Genes are Involved in Trehalose-6-Phosphate Synthase-Mediated Regulation of Pathogenicity by the Rice Blast Fungus Magnaporthe Oryzae. PloS Pathog. 9, e1003604. doi: 10.1371/journal.ppat.1003604
Bähler, J., Pringle, J. R. (1998). Pom1p, a Fission Yeast Protein Kinase That Provides Positional Information for Both Polarized Growth and Cytokinesis. Genes Dev. 12, 1356–1370. doi: 10.1101/gad.12.9.1356
Becker, W., Joost, H.-G. (1998). Structural and Functional Characteristics of Dyrk, a Novel Subfamily of Protein Kinases With Dual Specificity. Prog. Nucleic Acid Res. Mol. Biol. 62, 1–17. doi: 10.1016/S0079-6603(08)60503-6
Becker, W., Weber, Y., Wetzel, K., Eirmbter, K., Tejedor, F. J., Joost, H.-G. (1998). Sequence Characteristics, Subcellular Localization, and Substrate Specificity of DYRK-Related Kinases, a Novel Family of Dual Specificity Protein Kinases. J. Biol. Chem. 273, 25893–25902. doi: 10.1074/jbc.273.40.25893
Caires, N. P., Pinho, D. B., Souza, J. S. C., Silva, M. A., Lisboa, D. O., Pereira, O. L., et al. (2014). First Report of Anthracnose on Pepper Fruit Caused by Colletotrichum Scovillei in Brazil. Plant Dis. 98, 1437–1437. doi: 10.1094/PDIS-04-14-0426-PDN
Cannon, P., Damm, U., Johnston, P., Weir, B. (2007). Colletotrichum–current Status and Future Directions. Stud. Mycol. 59, 129–145. doi: 10.3114/sim0014
Celton-Morizur, S., Racine, V., Sibarita, J. B., Paoletti, A. (2006). Pom1 Kinase Links Division Plane Position to Cell Polarity by Regulating Mid1p Cortical Distribution. J. Cell Sci. 119, 4710–4718. doi: 10.1242/jcs.03261
Choi, J., Kim, Y., Kim, S., Park, J., Lee, Y.-H. (2009). MoCRZ1, a Gene Encoding a Calcineurin-Responsive Transcription Factor, Regulates Fungal Growth and Pathogenicity of Magnaporthe Oryzae. Fungal Genet. Biol. 46, 243–254. doi: 10.1016/j.fgb.2008.11.010
Dean, R., Van Kan, J. A., Pretorius, Z. A., Hammond-Kosack, K. E., Di Pietro, A., Spanu, P. D., et al. (2012). The Top 10 Fungal Pathogens in Molecular Plant Pathology. Mol. Plant Pathol. 13, 414–430. doi: 10.1111/j.1364-3703.2011.00783.x
de Assis, L. J., Manfiolli, A., Mattos, E., Fabri, J. H. M., Malavazi, I., Jacobsen, I. D., et al. (2018). Protein Kinase A and High-Osmolarity Glycerol Response Pathways Cooperatively Control Cell Wall Carbohydrate Mobilization in Aspergillus Fumigatus. MBio 9, e01952-18. doi: 10.1128/mBio.01952-18
Diao, Y.-Z., Zhang, C., Liu, F., Wang, W.-Z., Liu, L., Cai, L., et al. (2017). Colletotrichum Species Causing Anthracnose Disease of Chili in China. Pers.: Mol. Phylogeny. Evol. Fungi. 38, 20. doi: 10.3767/003158517X692788
Dickman, M. B., Ha, Y. S., Yang, Z., Adams, B., Huang, C. (2003). A Protein Kinase From Colletotrichum Trifolii is Induced by Plant Cutin and is Required for Appressorium Formation. Mol. Plant-Microbe Interact. 16, 411–421. doi: 10.1094/MPMI.2003.16.5.411
Duran, R., Cary, J. W., Calvo, A. M. (2010). Role of the Osmotic Stress Regulatory Pathway in Morphogenesis and Secondary Metabolism in Filamentous Fungi. Toxins 2, 367–381. doi: 10.3390/toxins2040367
Fu, T., Han, J. H., Shin, J. H., Song, H., Ko, J., Lee, Y. H., et al. (2021). Homeobox Transcription Factors are Required for Fungal Development and the Suppression of Host Defense Mechanisms in the Colletotrichum Scovillei-Pepper Pathosystem. Mbio 12, e01620-21. doi: 10.1128/mBio.01620-21
Fu, T., Shin, J. H., Lee, N. H., Lee, K. H., Kim, K. S. (2022). Mitogen-Activated Protein Kinase CsPMK1 is Essential for Pepper Fruit Anthracnose by Colletotrichum Scovillei. Front. Microbiol. 13. doi: 10.3389/fmicb.2022.770119
Garrett, S., Menold, M. M., Broach, J. R. (1991). The Saccharomyces Cerevisiae YAK1 Gene Encodes a Protein Kinase That is Induced by Arrest Early in the Cell Cycle. Mol. Cell. Biol. 11, 4045–4052. doi: 10.1128/mcb.11.8.4045-4052.1991
Gerganova, V., Floderer, C., Archetti, A., Michon, L., Carlini, L., Reichler, T., et al. (2019). Multi-Phosphorylation Reaction and Clustering Tune Pom1 Gradient Mid-Cell Levels According to Cell Size. Elife 8, e45983. doi: 10.7554/eLife.45983
Goyard, S., Knechtle, P., Chauvel, M., Mallet, A., Prévost, M. C., Proux, C., et al. (2008). The Yak1 Kinase is Involved in the Initiation and Maintenance of Hyphal Growth in Candida Albicans. Mol Biol. Cell 19, 2251–2266. doi: 10.1091/mbc.e07-09-0960
Hagiwara, D., Asano, Y., Marui, J., Yoshimi, A., Mizuno, T., Abe, K. (2009). Transcriptional Profiling for Aspergillus Nidulans HogA MAPK Signaling Pathway in Response to Fludioxonil and Osmotic Stress. Fungal Genet. Biol. 46, 868–878. doi: 10.1016/j.fgb.2009.07.003
Han, J.-H., Chon, J.-K., Ahn, J.-H., Choi, I.-Y., Lee, Y.-H., Kim, K. S. (2016). Whole Genome Sequence and Genome Annotation of Colletotrichum Acutatum, Causal Agent of Anthracnose in Pepper Plants in South Korea. Genom. Data 8, 45–46. doi: 10.1016/j.gdata.2016.03.007
Han, J. H., Lee, H. M., Shin, J. H., Lee, Y. H., Kim, K. S. (2015). Role of the MoYAK1 Protein Kinase Gene in Magnaporthe Oryzae Development and Pathogenicity. Environ. Microbiol. 17, 4672–4689. doi: 10.1111/1462-2920.13010
Hohmann, S. (2009). Control of High Osmolarity Signalling in the Yeast Saccharomyces Cerevisiae. FEBS Lett. 583, 4025–4029. doi: 10.1016/j.febslet.2009.10.069
Horwitz, B. A., Sharon, A., Lu, S. W., Ritter, V., Sandrock, T. M., Yoder, O. C., et al. (1999). AG Protein Alpha Subunit From Cochliobolus Heterostrophus Involved in Mating and Appressorium Formation. Fungal Genet. Biol. 26, 19–32. doi: 10.1006/fgbi.1998.1094
Huang, L., Zhang, S., Yin, Z., Liu, M., Li, B., Zhang, H., et al. (2017). MoVrp1, a Putative Verprolin Protein, is Required for Asexual Development and Infection in the Rice Blast Fungus Magnaporthe Oryzae. Sci Rep 7, 1–16. doi: 10.1038/srep41148
Kaczmarski, W., Barua, M., Mazur-Kolecka, B., Frackowiak, J., Dowjat, W., Mehta, P., et al. (2014). Intracellular Distribution of Differentially Phosphorylated Dual-Specificity Tyrosine Phosphorylation-Regulated Kinase 1A (DYRK1A). J. Neurosci. Res. 92, 162–173. doi: 10.1002/jnr.23279
Kang, S. H., Khang, C. H., Lee, Y. H. (1999). Regulation of cAMP-Dependent Protein Kinase During Appressorium Formation in Magnaporthe Grisea. FEMS Microbiol. Lett. 170, 419–423. doi: 10.1111/j.1574-6968.1999.tb13403.x
Kanto, T., Uematsu, S., Tsukamoto, T., Moriwaki, J., Yamagishi, N., Usami, T., et al. (2014). Anthracnose of Sweet Pepper Caused by Colletotrichum Scovillei in Japan. J. Gen. Plant Pathol. 80, 73–78. doi: 10.1007/s10327-013-0496-9
Kentrup, H., Becker, W., Heukelbach, J., Wilmes, A., Schürmann, A., Huppertz, et al. (1996). Dyrk, a Dual Specificity Protein Kinase With Unique Structural Features Whose Activity is Dependent on Tyrosine Residues Between Subdomains VII and VIII. J. Biol. Chem. 271, 3488–3495. doi: 10.1074/jbc.271.7.3488
Khalimi, K., Darmadi, A. A. K., Suprapta, D. N. (2019). First Report on the Prevalence of Colletotrichum Scovillei Associated With Anthracnose on Chili Pepper in Bali, Indonesia. Int. J. Agric. Biol. 22, 363–368. doi: 10.17957/IJAB/15.1072
Kim, S., Ahn, I. P., Rho, H. S., Lee, Y. H. (2005). MHP1, a Magnaporthe Grisea Hydrophobin Gene, is Required for Fungal Development and Plant Colonization. Mol. Microbiol. 57, 1224–1237. doi: 10.1111/j.1365-2958.2005.04750.x
Kim, S., Park, S.-Y., Kim, K. S., Rho, H.-S., Chi, M.-H., Choi, J., et al. (2009). Homeobox Transcription Factors are Required for Conidiation and Appressorium Development in the Rice Blast Fungus Magnaporthe Oryzae. PloS Genet. 5, e1000757. doi: 10.1371/journal.pgen.1000757
Lee, Y.-H., Dean, R. A. (1994). Hydrophobicity of Contact Surface Induces Appressorium Formation in Magnaporthe Grisea. FEMS Microbiol. Lett. 115, 71–75. doi: 10.1111/j.1574-6968.1994.tb06616.x
Lee, J., Leslie, J. F., Bowden, R. L. (2008). Expression and Function of Sex Pheromones and Receptors in the Homothallic Ascomycete Gibberella Zeae. Eukaryot. Cell 7, 1211–1221. doi: 10.1128/EC.00272-07
Liang, X., Wei, T., Cao, M., Zhang, X., Liu, W., Kong, Y., et al. (2019). The MAP Kinase CfPMK1 is a Key Regulator of Pathogenesis, Development, and Stress Tolerance of Colletotrichum Fructicola.Front. Microbiol. 10. doi: 10.3389/fmicb.2019.01070
Liu, F., Tang, G., Zheng, X., Li, Y., Sun, X., Qi, X., et al. (2016). Molecular and Phenotypic Characterization of Colletotrichum Species Associated With Anthracnose Disease in Peppers From Sichuan Province, China. Sci. Rep. 6, 1–17. doi: 10.1038/srep32761
Mehrabi, R., Zhao, X., Kim, Y., Xu, J.-R. (2009). “The cAMP Signaling and MAP Kinase Pathways in Plant Pathogenic Fungi,” in Plant Relationships, Vol. 5 (Berlin: Springer-Heidelberg), 157–172. doi: 10.1007/978-3-540-87407-2_8
Miyakawa, I., Aoi, H., Sando, N., Kuroiwa, T. (1984). Fluorescence Microscopic Studies of Mitochondrial Nucleoids During Meiosis and Sporulation in the Yeast, Saccharomyces Cerevisiae. J. Cell Sci. 66, 21–38. doi: 10.1242/jcs.66.1.21
Moriya, H., Shimizu-Yoshida, Y., Omori, A., Iwashita, S., Katoh, M., Sakai, A. (2001). Yak1p, a DYRK Family Kinase, Translocates to the Nucleus and Phosphorylates Yeast Pop2p in Response to a Glucose Signal. Genes Dev. 15, 1217–1228. doi: 10.1101/gad.884001
Moseley, J. B., Mayeux, A., Paoletti, A., Nurse, P. (2009). A Spatial Gradient Coordinates Cell Size and Mitotic Entry in Fission Yeast. Nature 459, 857–860. doi: 10.1038/nature08074
Mulder, N. J., Apweiler, R., Attwood, T. K., Bairoch, A., Bateman, A., Binns, D., et al. (2005). InterPro, Progress and Status in 2005. Nucleic Acids Res. 33, D201–D205. doi: 10.1093/nar/gki106
Noor, N. M., Zakaria, L. (2018). Identification and Characterization of Colletotrichum Spp. Associated With Chili Anthracnose in Peninsular Malaysia. Eur. J. Plant Pathol. 151, 961–973. doi: 10.1007/s10658-018-1431-x
Park, G., Xue, C., Zhao, X., Kim, Y., Orbach, M., Xu, J.-R. (2006). Multiple Upstream Signals Converge on the Adaptor Protein Mst50 in Magnaporthe Grisea. Plant Cell 18, 2822–2835. doi: 10.1105/tpc.105.038422
Pham, C. L., Rey, A., Lo, V., Soulès, M., Ren, Q., Meisl, G., et al. (2016). Self-Assembly of MPG1, a Hydrophobin Protein From the Rice Blast Fungus That Forms Functional Amyloid Coatings, Occurs by a Surface-Driven Mechanism. Sci. Rep. 6, 1–16. doi: 10.1038/srep25288
Saito, H., Posas, F. (2012). Response to Hyperosmotic Stress. Genetics 192, 289–318. doi: 10.1534/genetics.112.140863
Sambrook, J., Fritsch, E. F., Maniatis, T. (1989). Molecular cloning: A Laboratory Manual (New York: Cold Spring Harbor Laboratory Press).
Shin, J.-H., Han, J.-H., Park, H.-H., Fu, T., Kim, K. S. (2019). Optimization of Polyethylene Glycol-Mediated Transformation of the Pepper Anthracnose Pathogen Colletotrichum Scovillei to Develop an Applied Genomics Approach. Plant Pathol. J. 35, 575. doi: 10.5423/PPJ.OA.06.2019.0171
Takano, Y., Kikuchi, T., Kubo, Y., Hamer, J. E., Mise, K., Furusawa, I. (2000). The Colletotrichum Lagenarium MAP Kinase Gene CMK1 Regulates Diverse Aspects of Fungal Pathogenesis. Mol. Plant-Microbe Interact. 13, 374–383. doi: 10.1094/MPMI.2000.13.4.374
Talbot, N. J. (1995). Having a Blast: Exploring the Pathogenicity of Magnaporthe Grisea. Trends Microbiol. 3, 9–16. doi: 10.1016/S0966-842X(00)88862-9
Talbot, N. J., Ebbole, D. J., Hamer, J. E. (1993). Identification and Characterization of MPG1, a Gene Involved in Pathogenicity From the Rice Blast Fungus Magnaporthe Grisea. Plant Cell 5, 1575–1590. doi: 10.1105/tpc.5.11.1575
Tamura, K., Stecher, G., Peterson, D., Filipski, A., Kumar, S. (2013). MEGA6: Molecular Evolutionary Genetics Analysis Version 6.0. Mol. Biol. Evol. 30, 2725–2729. doi: 10.1093/molbev/mst197
Tatebayashi, K., Yamamoto, K., Tanaka, K., Tomida, T., Maruoka, T., Kasukawa, E., et al. (2006). Adaptor Functions of Cdc42, Ste50, and Sho1 in the Yeast Osmoregulatory HOG MAPK Pathway. EMBO J. 25, 3033–3044. doi: 10.1038/sj.emboj.7601192
Thines, E., Weber, R. W., Talbot, N. J. (2000). MAP Kinase and Protein Kinase A–dependent Mobilization of Triacylglycerol and Glycogen During Appressorium Turgor Generation by Magnaporthe Grisea. Plant Cell 12, 1703–1718. doi: 10.1105/tpc.12.9.1703
Thompson, J. D., Higgins, D. G., Gibson, T. J. (1994). CLUSTAL W: Improving the Sensitivity of Progressive Multiple Sequence Alignment Through Sequence Weighting, Position-Specific Gap Penalties and Weight Matrix Choice. Nucleic Acids Res. 22, 4673–4680. doi: 10.1093/nar/22.22.4673
Van Wuytswinkel, O., Reiser, V., Siderius, M., Kelders, M., Ammerer, G., Ruis, H., et al. (2000). Response of Saccharomyces Cerevisiae to Severe Osmotic Stress: Evidence for a Novel Activation Mechanism of the HOG MAP Kinase Pathway. Mol. Microbiol. 37, 382–397. doi: 10.1046/j.1365-2958.2000.02002.x
Wang, C., Zhang, S., Hou, R., Zhao, Z., Zheng, Q., Xu, Q., et al. (2011). Functional Analysis of the Kinome of the Wheat Scab Fungus Fusarium Graminearum. PloS Pathog. 7, e1002460. doi: 10.1371/journal.ppat.1002460
Wessels, J., De Vries, O., Asgeirsdottir, S., Springer, J. (1991). The Thn Mutation of Schizophyllum Commune, Which Suppresses Formation of Aerial Hyphae, Affects Expression of the Sc3 Hydrophobin Gene. Microbiology 137, 2439–2445. doi: 10.1099/00221287-137-10-2439
Wilson, R. A., Talbot, N. J. (2009). Under Pressure: Investigating the Biology of Plant Infection by Magnaporthe Oryzae. Nat. Rev. Microbiol. 7, 185–195. doi: 10.1038/nrmicro2032
Wu, C., Jansen, G., Zhang, J., Thomas, D. Y., Whiteway, M. (2006). Adaptor Protein Ste50p Links the Ste11p MEKK to the HOG Pathway Through Plasma Membrane Association. Genes Dev. 20, 734–746. doi: 10.1101/gad.1375706
Xu, J.-R., Hamer, J. E. (1996). MAP Kinase and cAMP Signaling Regulate Infection Structure Formation and Pathogenic Growth in the Rice Blast Fungus Magnaporthe Grisea. Genes Dev.1 10 (21), 2696–2706. doi: 10.1101/gad.10.21.2696
Yi, M., Chi, M.-H., Khang, C. H., Park, S.-Y., Kang, S., Valent, B., et al. (2009). The ER Chaperone LHS1 is Involved in Asexual Development and Rice Infection by the Blast Fungus Magnaporthe Oryzae. Plant Cell 21, 681–695. doi: 10.1105/tpc.107.055988
Yu, J.-H., Hamari, Z., Han, K.-H., Seo, J.-A., Reyes-Domínguez, Y., Scazzocchio, C. (2004). Double-Joint PCR: A PCR-Based Molecular Tool for Gene Manipulations in Filamentous Fungi. Fungal Genet. Biol. 41, 973–981. doi: 10.1016/j.fgb.2004.08.001
Zhao, X., Mehrabi, R., Xu, J. R. (2007). Mitogen-Activated Protein Kinase Pathways and Fungal Pathogenesis. Eukaryot. Cell 6, 1701–1714. doi: 10.1128/EC.00216-07
Zhao, W., Wang, T., Chen, Q., Chi, Y., Swe, T., Qi, R. (2016). First Report of Colletotrichum Scovillei Causing Anthracnose Fruit Rot on Pepper in Anhui Province, China. Plant Dis. 100, 2168–2168. doi: 10.1094/PDIS-04-16-0443-PDN
Keywords: cell division, DYRK, Colletotrichum scovillei, pepper anthracnose, Pom1
Citation: Shin J-H, Kim H-Y, Fu T, Lee K-H and Kim KS (2022) CsPOM1, a DYRK Family Kinase, Plays Diverse Roles in Fungal Development, Virulence, and Stress Tolerance in the Anthracnose Pathogen Colletotrichum scovillei. Front. Cell. Infect. Microbiol. 12:861915. doi: 10.3389/fcimb.2022.861915
Received: 25 January 2022; Accepted: 23 March 2022;
Published: 26 April 2022.
Edited by:
Yong-Sun Bahn, Yonsei University, South KoreaReviewed by:
Ci Fu, University of Toronto, CanadaHee-Soo Park, Kyungpook National University, South Korea
Copyright © 2022 Shin, Kim, Fu, Lee and Kim. This is an open-access article distributed under the terms of the Creative Commons Attribution License (CC BY). The use, distribution or reproduction in other forums is permitted, provided the original author(s) and the copyright owner(s) are credited and that the original publication in this journal is cited, in accordance with accepted academic practice. No use, distribution or reproduction is permitted which does not comply with these terms.
*Correspondence: Kyoung Su Kim, kims@kangwon.ac.kr