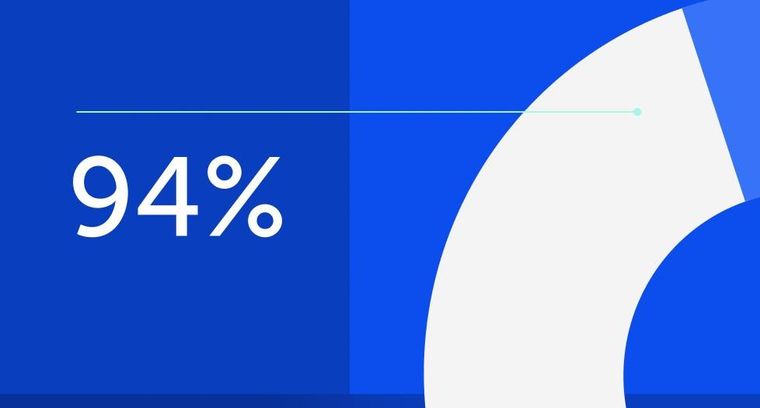
94% of researchers rate our articles as excellent or good
Learn more about the work of our research integrity team to safeguard the quality of each article we publish.
Find out more
MINI REVIEW article
Front. Cell. Infect. Microbiol., 07 March 2022
Sec. Molecular Bacterial Pathogenesis
Volume 12 - 2022 | https://doi.org/10.3389/fcimb.2022.861899
This article is part of the Research TopicObligate Intracellular Bacteria: Evasion and Adaptative Tactics Shaping the Host-Pathogen InterfaceView all 11 articles
Lateral gene transfer (LGT) facilitates many processes in bacterial ecology and pathogenesis, especially regarding pathogen evolution and the spread of antibiotic resistance across species. The obligate intracellular chlamydiae, which cause a range of diseases in humans and animals, were historically thought to be highly deficient in this process. However, research over the past few decades has demonstrated that this was not the case. The first reports of homologous recombination in the Chlamydiaceae family were published in the early 1990s. Later, the advent of whole-genome sequencing uncovered clear evidence for LGT in the evolution of the Chlamydiaceae, although the acquisition of tetracycline resistance in Chlamydia (C.) suis is the only recent instance of interphylum LGT. In contrast, genome and in vitro studies have shown that intraspecies DNA exchange occurs frequently and can even cross species barriers between closely related chlamydiae, such as between C. trachomatis, C. muridarum, and C. suis. Additionally, whole-genome analysis led to the identification of various DNA repair and recombination systems in C. trachomatis, but the exact machinery of DNA uptake and homologous recombination in the chlamydiae has yet to be fully elucidated. Here, we reviewed the current state of knowledge concerning LGT in Chlamydia by focusing on the effect of homologous recombination on the chlamydial genome, the recombination machinery, and its potential as a genetic tool for Chlamydia.
The gram-negative Chlamydiaceae family consists of several pathogenic species that cause diseases ranging from pneumonia to sexually transmitted infections (STI) in humans, livestock, pets, and wildlife. In humans, Chlamydia (C.) trachomatis is the cause of chronic eye infections leading to blindness (trachoma), and STI, while C. pneumoniae induces community-acquired pneumonia. C. psittaci is a zoonotic pathogen primarily detected in birds causing flu-like symptoms to life-threatening pneumonia in humans. C. abortus is the cause of ovine enzootic abortion (OEA) in sheep and goats and may also induce miscarriage in women. In contrast, C. suis, another chlamydial species with zoonotic potential, is found in the eyes and intestinal tract of pigs, often remaining asymptomatic (Dean et al., 2013; De Puysseleyr et al., 2014; De Puysseleyr et al., 2017; Sachse and Borel, 2020; Jordan et al., 2020). Although the chlamydial obligate intracellular life cycle is reflected by extensive streamlining and reduction of the genome (Palmer, 2002; Toft and Andersson, 2010), the Chlamydiaceae possess a number of genes involved in DNA uptake, recombination, and repair (Stephens et al., 1998; LaBrie et al., 2019) enabling intra- and interspecies lateral gene transfer (Suchland et al., 2009; Somboonna et al., 2011; Joseph and Read, 2012; Joseph et al., 2015; Marti et al., 2017).
Lateral, or horizontal, gene transfer (LGT) involves transfer of genetic material (DNA) from one cell to another and subsequent integration into the genome of the recipient cell. In bacteria, DNA transfer is primarily facilitated by transduction (bacteriophage infection), conjugation/mobilization/conduction (plasmid transfer), and transformation (uptake of naked DNA). DNA integration is then directed by homologous or non-homologous recombination (Redfield, 2001).
Here, we will review the current state of knowledge regarding lateral gene transfer (LGT) in the Chlamydiaceae by focusing on i) the impact of recombination on the Chlamydiaceae genome, ii) the homologous recombination machinery of the Chlamydiaceae, and iii) homologous recombination as a potential genetic tool.
The first reports providing evidence for intrastrain recombination within C. trachomatis were published in the 1990s and were based on gene-specific sequence analysis of ompA, which encodes the major outer membrane protein (MOMP) (Lampe et al., 1993; Brunham et al., 1994; Hayes et al., 1994; Hayes et al., 1995). Whole-genome analysis of laboratory and clinical strains later revealed that recombination events occurred across the entire genome during the evolution of C. trachomatis (Jeffrey et al., 2010; Joseph et al., 2011; Harris et al., 2012), as well as other chlamydial species such as C. pneumoniae, C. psittaci, and C. suis (Read et al., 2013; Roulis et al., 2015; Joseph et al., 2016; Seth-Smith et al., 2017b). Interestingly, investigation of C. abortus revealed no sign of recombination in currently circulating strains (Joseph et al., 2015; Seth-Smith et al., 2017a).
Whole-genome analyses further identified regions of high genomic diversity and, in parallel, regions with apparently higher rates of recombination. In C. trachomatis and C. pneumoniae; these included ompA (Hayes et al., 1994), the polymorphic membrane protein-encoding genes (pmps) (Jordan et al., 2001; Rocha et al., 2002; Brunelle and Sensabaugh, 2006), incA, and the translocated actin-recruiting phosphoprotein-encoding gene tarp (Joseph et al., 2012; Joseph and Read, 2012; Roulis et al., 2015), as well as the plasticity zone (PZ) (Jeffrey et al., 2010). Tarp is an important effector protein involved in the re-structuring of the host cytoskeleton (Tolchard et al., 2018). The PZ encodes for a range of different genes that are hypothesized to have important functions in the pathogenicity of the chlamydiae and may be a site of increased susceptibility for DNA uptake, genetic variation, and functional gene loss (Read et al., 2000; Thomson et al., 2008; Rajaram et al., 2015).
In the evolution and diversification of the Chlamydiaceae family, widespread gene rearrangement and translocation were identified between C. pneumoniae and C. trachomatis (Tillier and Collins, 2000). Furthermore, LGT events were detected both within and among the four major strain clusters of C. trachomatis, namely, the lymphogranuloma venereum (LGV), the trachoma, and two urogenital (T1, T2) clusters (Hadfield et al., 2017). Some studies have shown that this could have a clinical impact in terms of virulence and epidemiology (Somboonna et al., 2011; Andersson et al., 2016; Hadfield et al., 2017; Borges et al., 2019). Moreover, the effect of recombination can vary greatly between the four above-mentioned lineages of C. trachomatis, with the ocular strains being less affected than the urogenital lineages and the clonal LGV lineage having undergone no significant re-combination (Hadfield et al., 2017; Seth-Smith et al., 2021).
Overall, current data suggest that C. psittaci, C. pneumoniae, and C. suis have undergone higher rates of recombination than the entirety of the four C. trachomatis lineages. However, direct comparison between studies remains difficult due to the varying number of available genomes per species and because of the different approaches used to calculate r/m and other statistics that aim to quantify the recombination rate of a population (Read et al., 2013; Joseph et al., 2015; Roulis et al., 2015; Joseph et al., 2016; Hadfield et al., 2017; Seth-Smith et al., 2017b).
Additionally, one study proposed that ribosomal binding sites and tRNA may be associated with recombinant breakpoints (Gomes et al., 2006). However, these findings have yet to be confirmed by in vitro studies. So far, in vitro studies dealing with LGT following co-infection found little evidence for specific patterns, regions, or sites of recombination (Jeffrey et al., 2013; Marti et al., 2021), although there are notable differences between interspecies and intraspecies crosses, with intraspecies crosses generally leading to a higher proportion of donor DNA in the recombinant strains (Suchland et al., 2019). Moreover, the same study found that the replication termination is a target for interspecies recombination.
One very interesting chlamydial species in the context of LGT is C. suis. It is the only chlamydial species to have naturally obtained a resistance gene, tetA(C), which encodes a tetracycline efflux pump. This resistance allele and its genetic content were integrated as a genomic island (Tet-island) into the C. suis chromosome in an invasion-like gene (inv), probably during a transposition event directed by the transposase-encoding insertion sequence IScs605, although the exact mode of transmission and integration could not be replicated in an in vitro model involving C. suis (Dugan et al., 2004; Dugan et al., 2007). This Tet-island is the only evidence for recent acquisition of foreign DNA from other bacteria in Chlamydia spp. It shares high nucleotide identity with a pRAS3-type plasmid from the fish pathogen Aeromonas salmonicida ssp. salmonicida (Massicotte et al., 2019). It has been hypothesized that the plasmid was transferred via feeding of pigs with fish meal (Sandoz and Rockey, 2010) and was selected for with the use of tetracycline as a growth promoter in pig production facilities (Dugan et al., 2004). The use of tetracycline in pigs as prophylactic and therapeutic treatment has been shown to increase the rate of C. suis strains positive for tetA(C) (Borel et al., 2012; Borel et al., 2016; Wanninger et al., 2016), and whole-genome analysis and in vitro studies have indicated that intraspecies spread of tetA(C) is the result of homologous recombination (Joseph et al., 2016; Marti et al., 2017; Marti et al., 2021).
It is concerning that the tetA(C) marker can readily and stably integrate into C. trachomatis and C. muridarum strains in vitro (Suchland et al., 2009), leading to the possibility that these strains could acquire tetracycline resistance in clinical settings. This possibility was strengthened when C. suis was detected and isolated from the eyes, feces, and pharynges of veterinarians, pig farmers, and abattoir workers, although tetracycline-resistant C. suis strains have yet to be isolated from human samples (Dean et al., 2013; De Puysseleyr et al., 2014; De Puysseleyr et al., 2017). Because ocular C. trachomatis infection (inclusion conjunctivitis) through autoinoculation with genital C. trachomatis strains D-K has been reported (Haller-Schober and El-Shabrawi, 2002), the possibility of Tet-island transmission from C. suis to C. trachomatis cannot be excluded.
One remarkable finding that has emerged during natural LGT in Chlamydia is the difference between cross-species vs. intraspecies genetic transfer occurrence. We envision a model where LGT within the inclusion is very common, to the extent that clonal C. trachomatis recombines regularly in inclusions that form following infection with a single EB. The selective driver for such common genetic exchange is currently unclear but would be consistent with the principles of Muller’s Ratchet, where it is hypothesized that random mutation in haploid organisms would lead to fully degraded genomes in the absence of LGT (Joseph et al., 2011; Takeuchi et al., 2014). Other selective drivers, such as the Hill–Robertson effect, where the overall responsiveness to selection is reduced in finite populations, may also play a role (Joseph et al., 2011). Therefore, we propose that the reason for such common intraspecies LGT is to regenerate or maintain wild-type genomes in an intracellular environment that otherwise might be considered stressful and mutagenic (MacLean et al., 2013; Maharjan and Ferenci, 2017).
Homologous recombination allows inter- and intragenomic exchange of DNA and therefore plays a crucial role in genetic diversification and DNA repair (Rocha et al., 2005). In bacteria, homologous recombination consists of two major pathways, the RecBCD (Figure 1A) and the RecFOR pathway (Figure 1B), both of which facilitate DNA exchange between a com-plementary sequence and single-strand DNA (ssDNA) using the RecA protein (Rocha et al., 2005).
Figure 1 Homologous recombination in gram-negative bacteria. (A) The RecBCD pathway is activated following a double-strand break that causes the RecBCD complex to bind on both ends and degrade DNA from the 3′ to 5′ end until one of the complexes encounters a Chi site. RecBCD then degrades the DNA from to 5′ to 3′ end while RecA (green) can bind to the 3′ extension. Next, the RecA-covered single-strand DNA invades a homologous sequence (synapsis formation) and RuvABC (with or without RecG) is used to resolve the Holliday junction, exchanging DNA via recombination. (B) In the RecFOR pathway, a single-strand break is first unwound with helicase RecQ and degraded with RecJ, while single-stranded binding protein (SSB) attaches to the exposed strand. This is followed by RecFOR promoting the replacement of SSB with RecA followed by the same process as described in the RecBCD pathway. Proteins that were analyzed in detail regarding its function and activity in Chlamydia are labeled in blue; protein/sites that are unknown or inexistent in Chlamydia are labeled in red. The figure was modified from Rocha et al. (2005), Figure 1, and Snyder et al. (2013), Figures 10.2, 10.3, and 10.4.
In Chlamydia, whole-genome sequencing revealed that the genome contains various genes from the recombination and DNA repair machinery (Stephens et al., 1998; Azuma et al., 2006). However, only few studies have investigated the function and exact mechanism of the chlamydial homologous re-combination machinery. The first chlamydial recombination-associated protein to be analyzed was RecA in C. trachomatis, which was found to have moderate recombinational activity and possessed low efficiency after DNA damage by UV radiation compared to other bacteria (Hintz et al., 1995; Zhang et al., 1995). Chlamydial RecJ has a similar function as that of other gram-negative bacteria, namely, exonuclease activity in RecBCD-independent and conjugational recombination (Hsia and Bavoil, 1996; Rocha et al., 2005). It is expected that the RecBCD and RecFOR pathways of Chlamydia work similarly to that of E. coli, including formation and resolution of Holliday junctions due to the presence of ruv genes (Bastidas and Valdivia, 2016).
Some Chlamydia-specific particularities and open questions remain. For example, the histone-like protein Hc1 is involved in the condensation of the chlamydial nucleoid and inhibits RecA activity. Interestingly, however, Hc1 only inhibits its repair and not its recombinational activity (Ennis et al., 2000). For the RecBCD pathway, the exact identity of Chlamydia-specific Chi sites is unknown, as indicated in Figure 1 (Gomes et al., 2006).
Overall, it appears that the recombination machinery of the Chlamydiaceae family is complete, which underlines the importance of homologous recombination for a bacterial species that has undergone significant gene reduction (Palmer, 2002). However, more studies are necessary to confirm current assumptions that are only based on genomic data. With increasing options to genetically modify the chlamydiae (Valdivia and Bastidas, 2018), these investigations have become a possibility. First advances have already been made in recent years by the creation of knockout mutants in which genes involved in LGT are inactivated (Kokes et al., 2015; LaBrie et al., 2019; Wang et al., 2019). Currently available knockout mutants concerning LGT involving DNA uptake and ho-mologous recombination are listed in Table 1.
Genetic manipulation is an indispensable tool to understanding the biology of eukaryotic and prokaryotic cells. In the Chlamydia research field, tools for genetic modification have only recently been developed. The currently available methods have been reviewed in detail (Bastidas and Valdivia, 2016); therefore, we will only discuss genetic engineering in the context of homologous recombination.
The first report of successful, albeit transient, transformation of Chlamydia was published in the 1990s (Tam et al., 1994). Fifteen years later, a study could stably introduce kasugamycin and spectinomycin resistance into C. psittaci by introducing a pUC derivative, which carried the ribosomal RNA (rrn) region of C. psittaci with resistance-inducing point mutations, into the wild type using electroporation (Binet and Maurelli, 2009). Shuttle vectors comprising an E. coli vector and the chlamydial plasmid later allowed stable and reproducible transformation of Chlamydia (Wang et al., 2011), overhauling the field of Chlamydia genetics.
One of the remaining challenges of genetic manipulation of Chlamydia spp. is the inability of the pathogen to maintain plasmids with replication systems that do not include the native chlamydial plasmid. There has been significant progress in this field when a very recent report used a recombinant construct based on a broad-spectrum plasmid from Bordetella pertussis (pBBR1 MCS4) to transform a C. trachomatis L2 strain. This plasmid, pBVR1, contained C. trachomatis genomic sequences that allowed integration of the element into the C. trachomatis chromosome. This construct was maintained as both an episome and an integrated element in transformed strains. It is expected that further work with the pBBR1 vector system will perhaps allow the maintaining of non-chlamydial-plasmid-based genetic elements in transformed strains (Garvin et al., 2021).
Additionally, co-culture models were established as an alternative method to genetically modify the chlamydiae by co-infecting cells with two C. trachomatis strains, each carrying resistance-conferring mutations to either ofloxacin, lincomycin, trimethoprim, or rifampicin, and selecting for double-resistant recombinants. These studies detected recombination frequencies of 10-4 to 10-3 and further proposed that LGT likely played an important part in chlamydial evolution (DeMars et al., 2007; DeMars and Weinfurter, 2008). Similar protocols further demonstrated that interspecies transfer of the Tet-island from C. suis to C. trachomatis and C. muridarum, but not the more distantly related C. caviae, is possible. While C. muridarum obtained an approximately 100 kb-long sequence (the Tet-island and surrounding genes) as the result of a homologous recombination-mediated crossover event, co-infection of C. suis and C. trachomatis produced a mosaic strain with three instead of two rrn operons (Suchland et al., 2009). Interspecies transfer of the Tet-island via homologous recombination has been shown for C. trachomatis (Jeffrey et al., 2013) and C. suis, both in the presence (Marti et al., 2021) and absence (Marti et al., 2017) of double selection. Interestingly, comparison of in vitro-generated recombinant strains with clinical strains demonstrated that there are statistically more breakpoints in in vitro C. trachomatis strains compared to clinical strains, especially in the resistance-conferring genes rpoB (rifamycin group) and gyrA (ofloxacin) (Srinivasan et al., 2012).
The principle of co-infection and selection for recombinants has since become crucial in genetic engineering of the Chlamydia. For example, it has been used as a mapping tool in forward genetics, either by chemical mutagenesis and subsequent selection of recombinants using resistance markers (Nguyen and Valdivia, 2013; Nguyen and Valdivia, 2014) or by employing markerless recombination approaches (Brothwell et al., 2016). Moreover, suicide vectors that allow gene deletion following homologous recombination have been successfully constructed and used (Mueller et al., 2016; McKuen et al., 2017; Mueller et al., 2017). Finally, the principle of interspecies LGT has been exploited to create a hybrid strain library of C. trachomatis/C. muridarum crosses: a tet-resistant C. trachomatis strain was crossed in vitro with C. muridarum strains mutated by the plasmid-based Himar transposition system that randomly integrated a chloramphenicol marker into the genome (Suchland et al., 2019; Wang et al., 2019). This method was then used to produce PZ chimeras where the C. muridarum PZ replaced that of C. trachomatis, which demonstrated that the C. muridarum-specific large putative cytotoxins are not responsible for cytopathic and cytotoxic effects. This switch-out method further led to the detection of an inclusion protein, CT147, and CTL0402, which plays a role in the inclusion integrity (Dimond et al., 2021). A back-crossing strategy, which is a technique that can be used to effect functional complementation of mutants, was then used to restore both the wild-type genotype and phenotype.
Research over the past two decades has identified a paradox with regard to the genetic exchange and transformation within chlamydiae. First, decades of effort have demonstrated that Chlamydia spp. are very challenging to transform genetically, and even now the use of genetic systems remains difficult. This challenge is further exemplified by the near absence of LGT by members of the chlamydiae from bacteria across species. In contrast, some chlamydiae, notably C. trachomatis, undergo regular intraspecies LGT between different isolates.
The rarity of interphylum LGT events is contrasted with the presence of abundant LGT machinery retained in the chlamydial genome, even despite the considerable gene reduction following adaptation to its intracellular life cycle (Toft and Andersson, 2010). As described in this review, the recombination machinery of Chlamydia is complete, although some questions remain, and of the three major known forms of DNA uptake, two have been described. Specifically, while the Chlamydiaceae family does not possess a known conjugation machinery (Greub et al., 2004), transformation and transduction are possible. For example, one recent study showed that CT336 in C. trachomatis, a protein with limited sequence similarity to the Bacillus ComEC protein, plays an important role in DNA uptake via transformation (LaBrie et al., 2019). However, the same study noted that other important genes involved in the uptake of free dsDNA, namely, homologs of PilQ, ComEA, and DprA, are absent, which led to the conclusion that natural transformation in the Chlamydiaceae is different from that of other gram-negative bacteria, similar to Helicobacter pylori (LaBrie et al., 2019). Furthermore, chlamydiaphages (chlamydial bacteriophages) in the Chla-mydiamicrovirus genus have been described in various chlamydial species such as C. psittaci, C. abortus, C. felis, C. caviae, C. pecorum, and C. pneumoniae, but not in the more distantly related C. suis, C. muridarum, and C. trachomatis (Pawlikowska-Warych et al., 2015; Bastidas and Valdivia, 2016). Effort to use these phages to facilitate genetic in-troduction has not yet been successful, but perhaps future research will identify ways to use transduction as a tool of genetic exchange in Chlamydia.
In conclusion, despite significant progress in our un-derstanding of LGT in Chlamydia, many open questions remain. For example, most in vitro studies concerning LGT and homologous recombination have been conducted with C. suis, C. muridarum, and C. trachomatis. While it is possible to induce competence in C. psittaci, C. felis, and C. pneumoniae (Shima et al., 2018; Shima et al., 2020), we know very little about their DNA uptake system and if it is similar to that of C. trachomatis, C. muridarum, and C. suis. There may be a different mechanism for these species, as there appears to be a barrier of recombination between C. suis, C. trachomatis, and C. caviae (Suchland et al., 2009). Equipped with new genetic tools and a more extensive knowledge of LGT in the Chlamydiaceae family, we can tackle these challenging questions and further explore the biology of these complex bacteria.
HM, RS, and DR substantially contributed to the conception and design of the manuscript and reviewed the literature. All authors drafted and/or critically revised the manuscript, finally approved the version to be published, and agreed to be accountable for all aspects of the work.
This work was funded in part by the National MD-Ph.D. scholarship program organized by the Swiss Academy of Medical Sciences (SAMW), sponsored by the Swiss National Science Foundation (SNSF; Grant No. 323530_177579, awarded to HM from September 2017 to August 2020).
The authors declare that the research was conducted in the absence of any commercial or financial relationships that could be construed as a potential conflict of interest.
All claims expressed in this article are solely those of the authors and do not necessarily represent those of their affiliated organizations, or those of the publisher, the editors and the reviewers. Any product that may be evaluated in this article, or claim that may be made by its manufacturer, is not guaranteed or endorsed by the publisher.
We are grateful to Addison DeBoer for a careful reading of the manuscript.
Andersson, P., Harris, S. R., Smith, H. M. B. S., Hadfield, J., O’Neill, C., Cutcliffe, L. T., et al. (2016). Chlamydia Trachomatis From Australian Aboriginal People With Trachoma Are Polyphyletic Composed of Multiple Distinctive Lineages. Nat. Commun. 7, 10688. doi: 10.1038/ncomms10688
Azuma, Y., Hirakawa, H., Yamashita, A., Cai, Y., Rahman, M. A., Suzuki, H., et al. (2006). Genome Sequence of the Cat Pathogen, Chlamydophila Felis. DNA Res. 13, 15–23. doi: 10.1093/dnares/dsi027
Bastidas, R. J., Valdivia, R. H. (2016). Emancipating Chlamydia: Advances in the Genetic Manipulation of a Recalcitrant Intracellular Pathogen. Microbiol. Mol. Biol. Rev. 80, 411–427. doi: 10.1128/mmbr.00071-15
Binet, R., Maurelli, A. T. (2009). Transformation and Isolation of Allelic Exchange Mutants of Chlamydia Psittaci Using Recombinant DNA Introduced by Electroporation. Proc. Natl. Acad. Sci. 106, 292–297. doi: 10.1073/pnas.0806768106
Borel, N., Leonard, C., Slade, J., Schoborg, R. V. (2016). Chlamydial Antibiotic Resistance and Treatment Failure in Veterinary and Human Medicine. Curr. Clin. Microbiol. Rep. 3, 10–18. doi: 10.1007/s40588-016-0028-4
Borel, N., Regenscheit, N., Di Francesco, A., Donati, M., Markov, J., Masserey, Y., et al. (2012). Selection for Tetracycline-Resistant Chlamydia Suis in Treated Pigs. Vet. Microbiol. 156, 143–146. doi: 10.1016/j.vetmic.2011.10.011
Borges, V., Cordeiro, D., Salas, A. I., Lodhia, Z., Correia, C., Isidro, J., et al. (2019). Chlamydia Trachomatis: When the Virulence-Associated Genome Backbone Imports a Prevalence-Associated Major Antigen Signature. Microb. Genomics 5, e000313. doi: 10.1099/mgen.0.000313
Brothwell, J. A., Muramatsu, M. K., Toh, E., Rockey, D. D., Putman, T. E., Barta, M. L., et al. (2016). Interrogating Genes That Mediate Chlamydia Trachomatis Survival in Cell Culture Using Conditional Mutants and Recombination. J. Bacteriol. 198, 2131–2139. doi: 10.1128/JB.00161-16
Brunelle, B. W., Sensabaugh, G. F. (2006). The ompA Gene in Chlamydia Trachomatis Differs in Phylogeny and Rate of Evolution From Other Regions of the Genome. Infect. Immun. 74, 578–585. doi: 10.1128/IAI.74.1.578-585.2006
Brunham, R., Yang, C., Maclean, I., Kimani, J., Maitha, G., Plummer, F. (1994). Chlamydia Trachomatis From Individuals in a Sexually Transmitted Disease Core Group Exhibit Frequent Sequence Variation in the Major Outer Membrane Protein (Omp1) Gene. J. Clin. Invest 94, 458–463. doi: 10.1172/JCI117347
Dean, D., Rothschild, J., Ruettger, A., Kandel, R. P., Sachse, K. (2013). Zoonotic Chlamydiaceae Species Associated With Trachoma, Nepal. Emerg. Infect. Dis. 19, 1948–1955. doi: 10.3201/eid1912.130656
DeMars, R., Weinfurter, J. (2008). Interstrain Gene Transfer in Chlamydia Trachomatis In Vitro: Mechanism and Significance. J. Bacteriol. 190, 1605–1614. doi: 10.1128/JB.01592-07
DeMars, R., Weinfurter, J., Guex, E., Lin, J., Potucek, Y. (2007). Lateral Gene Transfer In Vitro in the Intracellular Pathogen Chlamydia Trachomatis. J. Bacteriol. 189, 991–1003. doi: 10.1128/JB.00845-06
De Puysseleyr, L., De Puysseleyr, K., Braeckman, L., Morré, S. A., Cox, E., Vanrompay, D. (2017). Assessment of Chlamydia Suis Infection in Pig Farmers. Transbound Emerg. Dis. 64, 826–833. doi: 10.1111/tbed.12446
De Puysseleyr, K., De Puysseleyr, L., Dhondt, H., Geens, T., Braeckman, L., Morré, S. A., et al. (2014). Evaluation of the Presence and Zoonotic Transmission of Chlamydia Suis in a Pig Slaughterhouse. BMC Infect. Dis. 14, 560. doi: 10.1186/s12879-014-0560-x
Dimond, Z. E., Suchland, R. J., Baid, S., LaBrie, S. D., Soules, K. R., Stanley, J., et al. (2021). Inter-Species Lateral Gene Transfer Focused on the Chlamydia Plasticity Zone Identifies Loci Associated With Immediate Cytotoxicity and Inclusion Stability. Mol. Microbiol 116, 1433–48. doi: 10.1111/mmi.14832
Dugan, J., Andersen, A. A., Rockey, D. D. (2007). Functional Characterization of IScs605, an Insertion Element Carried by Tetracycline-Resistant Chlamydia Suis. Microbiology 153, 71–79. doi: 10.1099/mic.0.29253-0
Dugan, J., Rockey, D. D., Jones, L. (2004). And Andersen, aTetracycline Resistance in Chlamydia Suis Mediated by Genomic Islands Inserted Into the Chlamydial Inv-Like Gene. A Antimicrob. Agents Chemother. 48, 3989–3995. doi: 10.1128/AAC.48.10.3989-3995.2004
Ennis, D. G., Woodgate, R., Shi, M. (2000). Selective Inhibition of RecA Functions by the Hc1 Nucleoid Condensation Protein From Chlamydia Trachomatis. FEMS Microbiol. Lett. 182, 279–283. doi: 10.1111/j.1574-6968.2000.tb08908.x
Garvin, L., Vande Voorde, R., Dickinson, M., Carrell, S., Hybiske, K., Rockey, D. (2021). A Broad-Spectrum Cloning Vector That Exists as Both an Integrated Element and a Free Plasmid in Chlamydia Trachomatis. PloS One 16, e0261088. doi: 10.1371/journal.pone.0261088
Gomes, J. P., Bruno, W. J., Nunes, A., Santos, N., Florindo, C., Borrego, M. J., et al. (2006). Evolution of Chlamydia Trachomatis Diversity Occurs by Widespread Interstrain Recombination Involving Hotspots. Genome Res. 17, 50–60. doi: 10.1101/gr.5674706
Greub, G., Collyn, F., Guy, L., Roten, C.-A. (2004). A Genomic Island Present Along the Bacterial Chromosome of the Parachlamydiaceae UWE25, an Obligate Amoebal Endosymbiont, Encodes a Potentially Functional F-Like Conjugative DNA Transfer System. BMC Microbiol. 4, 48. doi: 10.1186/1471-2180-4-48
Hadfield, J., Harris, S. R., Seth-Smith, H. M. B., Parmar, S., Andersson, P., Giffard, P. M., et al. (2017). Comprehensive Global Genome Dynamics of Chlamydia Trachomatis Show Ancient Diversification Followed by Contemporary Mixing and Recent Lineage Expansion. Genome Res. 27, 1220–1229. doi: 10.1101/gr.212647.116
Haller-Schober, E. M., El-Shabrawi, Y. (2002). Chlamydial Conjunctivitis (in Adults), Uveitis, and Reactive Arthritis, Including SARA. Sexually Acquired Reactive Arthritis. Best Pract. Res. Clin. Obstet Gynaecol. 16, 815–828. doi: 10.1053/beog.2002.0320
Harris, S. R., Clarke, I. N., Seth-Smith, H. M. B., Solomon, A. W., Cutcliffe, L. T., Marsh, P., et al. (2012). Whole-Genome Analysis of Diverse Chlamydia Trachomatis Strains Identifies Phylogenetic Relationships Masked by Current Clinical Typing. Nat. Genet. 44, 413–419. doi: 10.1038/ng.2214
Hayes, L. J., Pecharatana, S., Bailey, R. L., Hampton, T. J., Pickett, M. A., Mabey, D. C. W., et al. (1995). Extent and Kinetics of Genetic Change in the Omp1 Gene of Chlamydia Trachomatis in Two Villages With Endemic Trachoma. J. Infect. Dis. 172, 268–272. doi: 10.1093/infdis/172.1.268
Hayes, L. J., Yearsley, P., Treharne, J. D., Ballard, R. A., Fehler, G. H., Ward, M. E. (1994). Evidence for Naturally Occurring Recombination in the Gene Encoding the Major Outer Membrane Protein of Lymphogranuloma Venereum Isolates of Chlamydia Trachomatis. Infect. Immun. 62, 5659–5663. doi: 10.1128/iai.62.12.5659-5663.1994
Hintz, N. J., Ennis, D. G., Liu, W. F., Larsen, S. H. (1995). The recA Gene of Chlamydia Trachomatis : Cloning, Sequence, and Characterization in Escherichia Coli. FEMS Microbiol. Lett. 127, 175–179. doi: 10.1111/j.1574-6968.1995.tb07470.x
Hsia, R. C., Bavoil, P. M. (1996). Homologs of Escherichia Coli Recj, gltX and of a Putative “Early” Gene of Avian Chlamydia Psittaci Are Located Upstream of the “Late” Omp2 Locus of Chlamydia Psittaci Strain Guinea Pig Inclusion Conjunctivitis. Gene 176, 163–169. doi: 10.1016/0378-1119(96)00240-5
Jeffrey, B. M., Suchland, R. J., Eriksen, S. G., Sandoz, K. M., Rockey, D. D. (2013). Genomic and Phenotypic Characterization of In Vitro-Generated Chlamydia Trachomatis Recombinants. BMC Microbiol. 13, 142. doi: 10.1186/1471-2180-13-142
Jeffrey, B. M., Suchland, R. J., Quinn, K. L., Davidson, J. R., Stamm, W. E., Rockey, D. D. (2010). Genome Sequencing of Recent Clinical Chlamydia Trachomatis Strains Identifies Loci Associated With Tissue Tropism and Regions of Apparent Recombination. Infect. Immun. 78, 2544–2553. doi: 10.1128/IAI.01324-09
Jordan, I. K., Makarova, K. S., Wolf, Y. I., Koonin, E. V. (2001). Gene Conversions in Genes Encoding Outer-Membrane Proteins in H. Pylori and C. Pneumoniae. Trends Genet. 17, 7–10. doi: 10.1016/S0168-9525(00)02151-X
Jordan, S., Nelson, D., Geisler, W. (2020). “Chlamydia Trachomatis Infections,” in Chlamydia Biology: From Genome to Disease. Eds. Tan, M., Hegeman, J. H., Sütterlin, C. (Norfolk: Caister Academic Press), 1–30. doi: 10.21775/9781912530281.01
Joseph, S. J., Didelot, X., Gandhi, K., Dean, D., Read, T. D. (2011). Interplay of Recombination and Selection in the Genomes of Chlamydia Trachomatis. Biol. Direct 6, 28. doi: 10.1186/1745-6150-6-28
Joseph, S. J., Didelot, X., Rothschild, J., de Vries, H. J. C., Morré, S. A., Read, T. D., et al. (2012). Population Genomics of Chlamydia Trachomatis: Insights on Drift, Selection, Recombination, and Population Structure. Mol. Biol. Evol. 29, 3933–3946. doi: 10.1093/molbev/mss198
Joseph, S. J., Marti, H., Didelot, X., Castillo-Ramirez, S., Read, T. D., Dean, D. (2015). Chlamydiaceae Genomics Reveals Interspecies Admixture and the Recent Evolution of Chlamydia Abortus Infecting Lower Mammalian Species and Humans. Genome Biol. Evol. 7, 3070–3084. doi: 10.1093/gbe/evv201
Joseph, S. J., Marti, H., Didelot, X., Read, T. D., Dean, D. (2016). Tetracycline Selective Pressure and Homologous Recombination Shape the Evolution of Chlamydia Suis : A Recently Identified Zoonotic Pathogen. Genome Biol. Evol. 8, 2613–2623. doi: 10.1093/gbe/evw182
Joseph, S. J., Read, T. D. (2012). Genome-Wide Recombination in Chlamydia Trachomatis. Nat. Genet. 44, 364–366. doi: 10.1038/ng.2225
Kokes, M., Dunn, J. D., Granek, J. A., Nguyen, B. D., Barker, J. R., Valdivia, R. H., et al. (2015). Integrating Chemical Mutagenesis and Whole-Genome Sequencing as a Platform for Forward and Reverse Genetic Analysis of Chlamydia. Cell Host Microbe 17, 716–725. doi: 10.1016/j.chom.2015.03.014
LaBrie, S. D., Dimond, Z. E., Harrison, K. S., Baid, S., Wickstrum, J., Suchland, R. J., et al. (2019). Transposon Mutagenesis in Chlamydia Trachomatis Identifies CT339 as a ComEC Homolog Important for DNA Uptake and Lateral Gene Transfer. MBio 10, e01343–19. doi: 10.1128/mBio.01343-19
Lampe, M. F., Suchland, R. J., Stamm, W. E. (1993). Nucleotide Sequence of the Variable Domains Within the Major Outer Membrane Protein Gene From Serovariants of Chlamydia Trachomatis. Infect. Immun. 61, 213–219. doi: 10.1128/iai.61.1.213-219.1993
MacLean, R. C., Torres-Barceló, C., Moxon, R. (2013). Evaluating Evolutionary Models of Stress-Induced Mutagenesis in Bacteria. Nat. Rev. Genet. 14, 221–227. doi: 10.1038/nrg3415
Maharjan, R. P., Ferenci, T. (2017). A Shifting Mutational Landscape in 6 Nutritional States: Stress-Induced Mutagenesis as a Series of Distinct Stress Input–Mutation Output Relationships. PloS Biol. 15, e2001477. doi: 10.1371/journal.pbio.2001477
Marti, H., Bommana, S., Read, T. D., Pesch, T., Prähauser, B., Dean, D., et al. (2021). Generation of Tetracycline and Rifamycin Resistant Chlamydia Suis Recombinants. Front. Microbiol. 12, 630293. doi: 10.3389/fmicb.2021.630293
Marti, H., Kim, H., Joseph, S. J., Dojiri, S., Read, T. D., Dean, D. (2017). Tet(C) Gene Transfer Between Chlamydia Suis Strains Occurs by Homologous Recombination After Co-Infection: Implications for Spread of Tetracycline-Resistance Among Chlamydiaceae. Front. Microbiol. 8, 156. doi: 10.3389/fmicb.2017.00156
Massicotte, M.-A., Vincent, A. T., Schneider, A., Paquet, V. E., Frenette, M., Charette, S. J. (2019). One Aeromonas Salmonicida Subsp. Salmonicida Isolate With a Pasa5 Variant Bearing Antibiotic Resistance and a Pras3 Variant Making a Link With a Swine Pathogen. Sci. Total Environ. 690, 313–320. doi: 10.1016/j.scitotenv.2019.06.456
McKuen, M. J., Mueller, K. E., Bae, Y. S., Fields, K. A. (2017). Fluorescence-Reported Allelic Exchange Mutagenesis Reveals a Role for Chlamydia Trachomatis TmeA in Invasion That Is Independent of Host AHNAK. Infect. Immun. 85, e00640–17. doi: 10.1128/IAI.00640-17
Mueller, K. E., Wolf, K., Fields, K. A. (2016). Gene Deletion by Fluorescence-Reported Allelic Exchange Mutagenesis in Chlamydia Trachomatis. MBio 7, e01817–15. doi: 10.1128/mBio.01817-15
Mueller, K. E., Wolf, K., Fields, K. A. (2017). Chlamydia Trachomatis Transformation and Allelic Exchange Mutagenesis. Curr. Protoc. Microbiol. 45, 11A.3.1–11A.3.15. doi: 10.1002/cpmc.31
Nguyen, B. D., Valdivia, R. H. (2013). Forward Genetic Approaches in Chlamydia Trachomatis. J. Vis. Exp. 50636. doi: 10.3791/50636
Nguyen, B., Valdivia, R. (2014). A Chemical Mutagenesis Approach to Identify Virulence Determinants in the Obligate Intracellular Pathogen Chlamydia Trachomatis. Methods Mol. Biol. 1197, 347–358. doi: 10.1007/978-1-4939-1261-2_20
Palmer, G. H. (2002). The Highest Priority: What Microbial Genomes Are Telling Us About Immunity. Vet. Immunol. Immunopathol. 85, 1–8. doi: 10.1016/S0165-2427(01)00415-9
Pawlikowska-Warych, M., Śliwa-Dominiak, J., Deptuła, W. (2015). Chlamydial Plasmids and Bacteriophages. Acta Biochim. Pol. 62, 1–6. doi: 10.18388/abp.2014_764
Rajaram, K., Giebel, A. M., Toh, E., Hu, S., Newman, J. H., Morrison, S. G., et al. (2015). Mutational Analysis of the Chlamydia Muridarum Plasticity Zone. Infect. Immun. 83, 2870–2881. doi: 10.1128/IAI.00106-15
Read, T. D., Brunham, R. C., Shen, C., Gill, S. R., Heidelberg, J. F., White, O., et al. (2000). Genome Sequences of Chlamydia Trachomatis MoPn and Chlamydia Pneumoniae AR39. Nucleic Acids Res. 28, 1397–1406. doi: 10.1093/nar/28.6.1397
Read, T. D., Joseph, S. J., Didelot, X., Liang, B., Patel, L., Dean, D. (2013). Comparative Analysis of Chlamydia Psittaci Genomes Reveals the Recent Emergence of a Pathogenic Lineage With a Broad Host Range. MBio 4, e00604–12-e00604-12. doi: 10.1128/mBio.00604-12
Rocha, E. P. C., Cornet, E., Michel, B. (2005). Comparative and Evolutionary Analysis of the Bacterial Homologous Recombination Systems. PloS Genet. 1, 0247–0259. doi: 10.1371/journal.pgen.0010015
Rocha, E. P. C., Pradillon, O., Bui, H., Sayada, C., Denamur, E. (2002). A New Family of Highly Variable Proteins in the Chlamydophila Pneumoniae Genome. Nucleic Acids Res. 30, 4351–4360. doi: 10.1093/nar/gkf571
Roulis, E., Bachmann, N. L., Myers, G. S. A., Huston, W., Summersgill, J., Hudson, A., et al. (2015). Comparative Genomic Analysis of Human Chlamydia Pneumoniae Isolates From Respiratory, Brain and Cardiac Tissues. Genomics 106, 373–383. doi: 10.1016/j.ygeno.2015.09.008
Sachse, K., Borel, N. (2020). “Recent Advances in Epidemiology, Pathology and Immunology of Veterinary Chlamydiae,” in Chlamydia Biology: From Genome to Disease. Eds. Tan, M., Hegeman, J. H., Sütterlin, C. (Norfolk: Caister Academic Press), 403–428. doi: 10.21775/9781912530281.17
Sandoz, K. M., Rockey, D. D. (2010). Antibiotic Resistance in Chlamydiae. Future Microbiol. 5, 1427–1442. doi: 10.2217/fmb.10.96
Seth-Smith, B., Bénard, A., Bruisten, S. M., Versteeg, B., Herrmann, B., Kok, J., et al. (2021). Ongoing Evolution of Chlamydia Trachomatis Lymphogranuloma Venereum: Exploring the Genomic Diversity of Circulating Strains. Microb. Genomics 7, 599. doi: 10.1099/mgen.0.000599
Seth-Smith, H. M. B., Busó, L. S., Livingstone, M., Sait, M., Harris, S. R., Aitchison, K. D., et al. (2017a). European Chlamydia Abortus Livestock Isolate Genomes Reveal Unusual Stability and Limited Diversity, Reflected in Geographical Signatures. BMC Genomics 18, 344. doi: 10.1186/s12864-017-3657-y
Seth-Smith, H. M. B., Wanninger, S., Bachmann, N., Marti, H., Qi, W., Donati, M., et al. (2017b). The Chlamydia Suis Genome Exhibits High Levels of Diversity, Plasticity, and Mobile Antibiotic Resistance: Comparative Genomics of a Recent Livestock Cohort Shows Influence of Treatment Regimes. Genome Biol. Evol. 9, 750–760. doi: 10.1093/gbe/evx043
Shima, K., Wanker, M., Skilton, R. J., Cutcliffe, L. T., Schnee, C., Kohl, T. A., et al. (2018). The Genetic Transformation of Chlamydia pneumoniae. mSphere 3. doi: 10.1128/mSphere.00412-18
Shima, K., Weber, M. M., Schnee, C., Sachse, K., Käding, N., Klinger, M., et al. (2020). Development of a Plasmid Shuttle Vector System for Genetic Manipulation of Chlamydia Psittaci. mSphere 5, e00787–20. doi: 10.1128/mSphere.00787-20
Snyder, L., Peters, J. E., Henkin, T., Champness, W., Snyder, L. (2013). Molecular Genetics of Bacteria. 4th (Washington DC, USA: American Society for Microbiology (ASM).
Somboonna, N., Wan, R., Ojcius, D. M., Pettengill, M. A., Joseph, S. J., Chang, A., et al. (2011). Hypervirulent Chlamydia Trachomatis Clinical Strain Is a Recombinant Between Lymphogranuloma Venereum (L2) and D Lineages. MBio 2, e00045–11. doi: 10.1128/mBio.00045-11
Srinivasan, T., Bruno, W. J., Wan, R., Yen, A., Duong, J., Dean, D. (2012). In Vitro Recombinants of Antibiotic-Resistant Chlamydia Trachomatis Strains Have Statistically More Breakpoints Than Clinical Recombinants for the Same Sequenced Loci and Exhibit Selection at Unexpected Loci. J. Bacteriol. 194, 617–626. doi: 10.1128/JB.06268-11
Stephens, R. S., Kalman, S., Lammel, C., Fan, J., Marathe, R., Aravind, L., et al. (1998). Genome Sequence of an Obligate Intracellular Pathogen of Humans: Chlamydia Trachomatis. Sci. (80-) 282, 754–759. doi: 10.1126/science.282.5389.754
Suchland, R. J., Carrell, S. J., Wang, Y., Hybiske, K., Kim, D. B., Dimond, Z. E., et al. (2019). Chromosomal Recombination Targets in Chlamydia Interspecies Lateral Gene Transfer. J. Bacteriol. 201, e00365–19. doi: 10.1128/JB.00365-19
Suchland, R. J., Sandoz, K. M., Jeffrey, B. M., Stamm, W. E., Rockey, D. D. (2009). Horizontal Transfer of Tetracycline Resistance Among Chlamydia Spp. In Vitro. Antimicrob. Agents Chemother. 53, 4604–4611. doi: 10.1128/AAC.00477-09
Takeuchi, N., Kaneko, K., Koonin, E. V. (2014). Horizontal Gene Transfer can Rescue Prokaryotes From Muller’s Ratchet: Benefit of DNA From Dead Cells and Population Subdivision. G3 Genes Genomes Genet. 4, 325–339. doi: 10.1534/G3.113.009845/-/DC1
Tam, J. E., Davis, C. H., Wyrick, P. B. (1994). Expression of Recombinant DNA Introduced Into Chlamydia Trachomatis by Electroporation. Can. J. Microbiol. 40, 583–591. doi: 10.1139/m94-093
Thomson, N. R., Holden, M. T. G., Carder, C., Lennard, N., Lockey, S. J., Marsh, P., et al. (2008). Chlamydia Trachomatis: Genome Sequence Analysis of Lymphogranuloma Venereum Isolates. Genome Res. 18, 161–171. doi: 10.1101/gr.7020108
Tillier, E. R. M., Collins, R. A. (2000). Genome Rearrangement by Replication-Directed Translocation. Nat. Genet. 26, 195–197. doi: 10.1038/79918
Toft, C., Andersson, S. G. E. (2010). Evolutionary Microbial Genomics: Insights Into Bacterial Host Adaptation. Nat. Rev. Genet. 11, 465–475. doi: 10.1038/nrg2798
Tolchard, J., Walpole, S. J., Miles, A. J., Maytum, R., Eaglen, L. A., Hackstadt, T., et al. (2018). The Intrinsically Disordered Tarp Protein From Chlamydia Binds Actin With a Partially Preformed Helix. Sci. Rep. 8, 1960. doi: 10.1038/s41598-018-20290-8
Valdivia, R. H., Bastidas, R. J. (2018). The Expanding Molecular Genetics Tool Kit in Chlamydia. J. Bacteriol. 200, e00590–18. doi: 10.1128/JB.00590-18
Wang, Y., Kahane, S., Cutcliffe, L. T., Skilton, R. J., Lambden, P. R., Clarke, I. N. (2011). Development of a Transformation System for Chlamydia Trachomatis: Restoration of Glycogen Biosynthesis by Acquisition of a Plasmid Shuttle Vector. PloS Pathog. 7, e1002258. doi: 10.1371/journal.ppat.1002258
Wang, Y., LaBrie, S. D., Carrell, S. J., Suchland, R. J., Dimond, Z. E., Kwong, F., et al. (2019). Development of Transposon Mutagenesis for Chlamydia Muridarum. J. Bacteriol. 201, e1002258. doi: 10.1128/JB.00366-19
Wanninger, S., Donati, M., Di Francesco, A., Hässig, M., Hoffmann, K., Seth-Smith, H. M. B., et al. (2016). Selective Pressure Promotes Tetracycline Resistance of Chlamydia Suis in Fattening Pigs. PloS One 11, e0166917. doi: 10.1371/journal.pone.0166917
Keywords: horizontal gene transfer, homologous recombination, Chlamydiaceae, RecBCD, RecFOR, co-infection, membrane proteins, DNA uptake
Citation: Marti H, Suchland RJ and Rockey DD (2022) The Impact of Lateral Gene Transfer in Chlamydia. Front. Cell. Infect. Microbiol. 12:861899. doi: 10.3389/fcimb.2022.861899
Received: 25 January 2022; Accepted: 07 February 2022;
Published: 07 March 2022.
Edited by:
Luís Jaime Mota, NOVA School of Science and Technology, PortugalReviewed by:
Sandeep J. Joseph, Centers for Disease Control and Prevention (CDC), United StatesCopyright © 2022 Marti, Suchland and Rockey. This is an open-access article distributed under the terms of the Creative Commons Attribution License (CC BY). The use, distribution or reproduction in other forums is permitted, provided the original author(s) and the copyright owner(s) are credited and that the original publication in this journal is cited, in accordance with accepted academic practice. No use, distribution or reproduction is permitted which does not comply with these terms.
*Correspondence: Hanna Marti, aGFubmEubWFydGlAdXpoLmNo
Disclaimer: All claims expressed in this article are solely those of the authors and do not necessarily represent those of their affiliated organizations, or those of the publisher, the editors and the reviewers. Any product that may be evaluated in this article or claim that may be made by its manufacturer is not guaranteed or endorsed by the publisher.
Research integrity at Frontiers
Learn more about the work of our research integrity team to safeguard the quality of each article we publish.