- Department of Biology, Niagara University, Niagara Falls, NY, United States
Candida sp. are among the most common fungal commensals found in the human microbiome. Although Candida can be found residing harmlessly on the surface of the skin and mucosal membranes, these opportunistic fungi have the potential to cause superficial skin, nail, and mucus membrane infections as well as life threatening systemic infections. Severity of infection is dependent on both fungal and host factors including the immune status of the host. Virulence factors associated with Candida sp. pathogenicity include adhesin proteins, degradative enzymes, phenotypic switching, and morphogenesis. A central transcriptional regulator of morphogenesis, the transcription factor Efg1 was first characterized in Candida albicans in 1997. Since then, EFG1 has been referenced in the Candida literature over three thousand times, with the number of citations growing daily. Arguably one of the most well studied genes in Candida albicans, EFG1 has been referenced in nearly all contexts of Candida biology from the development of novel therapeutics to white opaque switching, hyphae morphology to immunology. In the review that follows we will synthesize the research that has been performed on this extensively studied transcription factor and highlight several important unanswered questions.
Introduction
As an obligate human commensal, Candida albicans must be able to readily adapt to the niches present in the human host, as well as transition to a pathogenic state under a specific set of environmental cues. Transcriptional programs coordinate the morphology, nutrient absorption, cell wall components, growth/reproduction, and metabolic patterns (among many other traits) employed in response to the combination of environmental cues in a given niche (Rodriguez et al., 2020). These transcriptional patterns are regulated by networks of transcription factors, with a given transcription factor often having both overlapping and distinct functions from the other transcription factors within the network. Efg1 is a transcription factor that has been implicated in several different transcription factor regulatory networks including white/opaque switching, cell morphology, and biofilm formation, all integral to the ability of C. albicans to exist as either a commensal or transition to a pathogenic state (Lassak et al., 2011; Nobile et al., 2012; Hernday et al., 2013; Rodriguez et al., 2020; Witchley et al., 2021). As such, understanding the function of EFG1 is vital to understanding the transcriptional programs that govern virulence in C. albicans. This review begins with the structure, binding, and activation of Efg1, then discusses the role of EFG1 in filamentation, biofilm formation, white/gray/opaque switching, gut colonization, and virulence. For each of these components there is an emphasis on how EFG1 expression and Efg1 activity are regulated, the transcripts that Efg1 binds, and the interacting protein partners that make up the complex transcription factor regulatory networks that contribute to the success of C. albicans as both a commensal and a pathogen.
Efg1 Structure and DNA Binding Activity
Efg1 is a member of the basic helix-loop-helix (bHLH) transcription factor family. bHLH transcription factors are found throughout the eukaryotic kingdom and are characterized by the presence of a DNA-binding basic region and a helix-loop-helix motif (Jones, 2004). Efg1 belongs to a subcategory of bHLH transcription factors in fungi called APSES (Asm1, Phd1, Sok2, Efg1 and StuA) family transcriptional regulators. APSES proteins can act as either transcriptional activators or repressors and have been shown to regulate several fungal cellular processes including sporulation, mating, aspects of metabolism, and morphology. The ASPES region of the protein contains a highly conserved region of approximately 100 amino acids, the central domain of which forms the bHLH structure (Zhao et al., 2015). Based on targeted deletion analysis in C. albicans, this ASPES structure is required for normal yeast morphology, hyphal induction, opaque to white switching, chlamydospore formation, binding to MCB motifs, repressor activity, and for maintaining Efg1 protein levels in the cell (Noffz et al., 2008). Outside of the ASPES region the N- and C- terminal regions also have specific roles in contributing to chlamydospore formation, opaque to white switching, hyphae formation in Lee’s medium, repressor activity and Czf1 binding (Noffz et al., 2008). The effects observed in mutations to regions outside of the DNA binding region may be due to changes in either protein interaction regions and/or phosphorylation sites. Yeast two-hybrid studies have shown that Efg1 is capable of interacting with several proteins including Czf1, Flo8, and members of the NuA4 histone acetyltransferase complex (Giusani et al., 2002; Cao et al., 2006; Lu et al., 2008; Noffz et al., 2008). These same studies demonstrated that the typical protein-protein interaction site, the APSES structure, is not required for Efg1 protein-protein interactions with Czf1 or Flo8 (Cao et al., 2006; Noffz et al., 2008). Instead Efg1 protein-protein interactions are likely mediated by prion-like domains (PrLDs) found in the N- and C- terminal regions of Efg1 (Frazer et al., 2020). PrLDs have been identified in several transcription factors known to form a transcriptional regulatory network with Efg1, this list includes Czf1 which has been demonstrated to physically interact with Efg1 (Giusani et al., 2002; Frazer et al., 2020). These PrLDs allow for Efg1 and other transcription factors containing PrLDs to undergo liquid-liquid phase separation into larger transcription factor regulatory complexes similar to the super-enhancers found in mammalian systems (Frazer et al., 2020). The ASPES region is also typically associated with homodimer formation in other ASPES transcription factors. Yeast-two hybrid assays of Efg1 suggested Efg1 does not form a homodimer, nor does Efg1 form a heterodimer with the closely related Efh1 transcription factor (Doedt et al., 2004). However phase separation experiments demonstrated that purified Efg1 protein is able to form droplets in a manner dependent on both the N- and C- terminal PrLDs, suggesting that Efg1 is able to undergo liquid-liquid phase separation to form larger condensates of Efg1 complexes with itself (Frazer et al., 2020). It is possible that attachment of LexA to Efg1 in previous yeast-two hybrid experiments may have disrupted the ability of the Efg1 PrLD regions to form multivalent functional assemblies of Efg1.
Several studies have attempted to identify the DNA target sequence of Efg1. Initial studies characterized Efg1 as capable of binding the E Box motif (CANNTG) using an E. coli expression system (Leng et al., 2001). Efg1 has also been shown to bind MluI (MCB) in a yeast one-hybrid assay (Noffz et al., 2008). Chromatin immunoprecipitation microarray (ChIP-chip) binding experiments at 30°C in YPD yielded a consensus element of (TATGCATA) with an observed transformation in the binding sequence following hyphae induction in serum at 37°C (Lassak et al., 2011). Subsequent ChIP-chip experiments of Efg1 under biofilm formation conditions and during standard mid-log phase growth generated cis-regulatory sequence motifs of (RTGCATRW) and (TGCAT) respectively (Nobile et al., 2012; Hernday et al., 2013). Recruitment of Efg1 to alternative DNA binding sites under different conditions is likely the result of changes in Efg1 phosphorylation status and/or interactions with other transcription factors (Lassak et al., 2011). In vitro studies using recombinant Efg1 eliminate the potential for interactions with other DNA binding proteins to modulate the consensus element of Efg1. Use of recombinant Efg1 and the MITOMI 2.0 technique which uses an 8-mer DNA sequence library to screen for the binding affinity identified an Efg1 DNA binding motif of CATGCGY (Hernday et al., 2013).
Efg1 has been observed to be heavily phosphorylated under yeast growth conditions, with the phosphorylation status of Efg1 changing under both hyphal induction and hypoxic conditions (Lassak et al., 2011; Saputo et al., 2014; Desai et al., 2015). Studies using epistasis modeling and phosphomimetic mutations point to residues T208 and T181 in Efg1 as predicted phosphorylation sites for PKA and Cdc28-Hgc1, respectively (note that residues T208 and T181 in assembly 22 SC5314 were previously reported as T206 and T179) (Bockmühl and Ernst, 2001; Bockmühl et al., 2001; Wang et al., 2009). However, changes in the phosphorylation status of Efg1 in a PKA deletion mutant were not detected by western blot of Efg1 under hyphal induction conditions with 10% serum (Lassak et al., 2011). Additionally, phosphoproteomic studies have failed to identify these phosphorylation sites when filamentation was induced with Lee’s medium or GlcNac. Instead, phosphoproteomic studies identified S355, S357, T512, and S518 as Efg1 phosphorylation sites (Willger et al., 2015; Cao et al., 2017; Min et al., 2021). Further investigation into the differences in phosphorylation patterns under different conditions and the potential inconsistency between studies using epistasis and phosphomimetic mutations versus phosphoproteomic techniques are necessary.
Role of Efg1 in Filamentation and Morphogenesis
As part of the ASPES family of transcription factors, Efg1 is highly homologous to both Sok2 and Phd1, transcription factors which have roles in pseudohyphal growth in Saccharomyces cerevisiae (Stoldt et al., 1997). Phd1 is a positive regulator of pseudohyphae formation, binding to the promoter of FLO11 which is required for the cell-to cell-adhesion that facilitates invasive pseudohyphal growth (Pan and Heitman, 2000; Cullen and Sprague, 2012). Sok2 acts as a negative regulator of pseudohyphae formation repressing expression of both PHD1 and FLO11 (Pan and Heitman, 2000; Cullen and Sprague, 2012; Mayhew and Mitra, 2014). Both Sok2 and Phd1 function downstream of the cAMP/PKA pathway in Saccharomyces cerevisiae (Cullen and Sprague, 2012; Raithatha et al., 2012). EFG1 was initially characterized by Joachim Ernst’s group in 1997 through a Saccharomyces cerevisiae screen for C. albicans genes that promoted pseudohyphal morphogenesis (Stoldt et al., 1997). In this study high levels of Efg1 in S. cerevisiae produced robust pseudohyphae, while overexpression of EFG1 in C. albicans caused increased pseudohyphal growth. Conversely, repression of EFG1 in C. albicans produced elongated cells resembling the opaque cell type. As such, these experiments were the first to link EFG1 to not only cell morphology but also white/opaque switching (Stoldt et al., 1997).
There are a wide variety of conditions that induce filamentation in C. albicans including factors that mimic the interstitial fluid environment such as serum (Taschdjian et al., 1960), 5% CO2 (Klengel et al., 2005), a neutral pH (Buffo et al., 1984), incubation at 37°C (Shapiro et al., 2009), and growth in tissue culture media (Sudbery, 2011). Factors that mimic the phagolysosome such as hydrogen peroxide and nitric oxide have also been shown to induce hyphal formation (Lo et al., 1997; Nasution et al., 2008; Uwamahoro et al., 2014; Koch et al., 2018). Other conditions and media that cause hyphal growth include the presence of N-acetylglucosamine (GlcNac) (Simonetti et al., 1974), growth within an embedded matrix (Brown Jr. et al., 1999), nutrient deprivation (Lu et al., 2011), nitrogen starvation (Lee et al., 2014), bacterial peptidoglycan (Xu et al., 2008) as well as Lee’s medium (Lee et al., 1975) and Spider medium among others (Liu et al., 1994). Although there are shared transcriptional patterns associated with hyphae formation under various conditions, different environmental cues trigger different signaling cascades to induce filamentation (Sudbery, 2011; Noble et al., 2017). Efg1 has been shown to both positively and negatively impact hyphae formation depending on the environmental cues and subsequent signaling cascades. Figure 1 provides an overview of the environmental conditions and signaling cascades that result in Efg1-mediated induction of hyphae-associated gene expression.
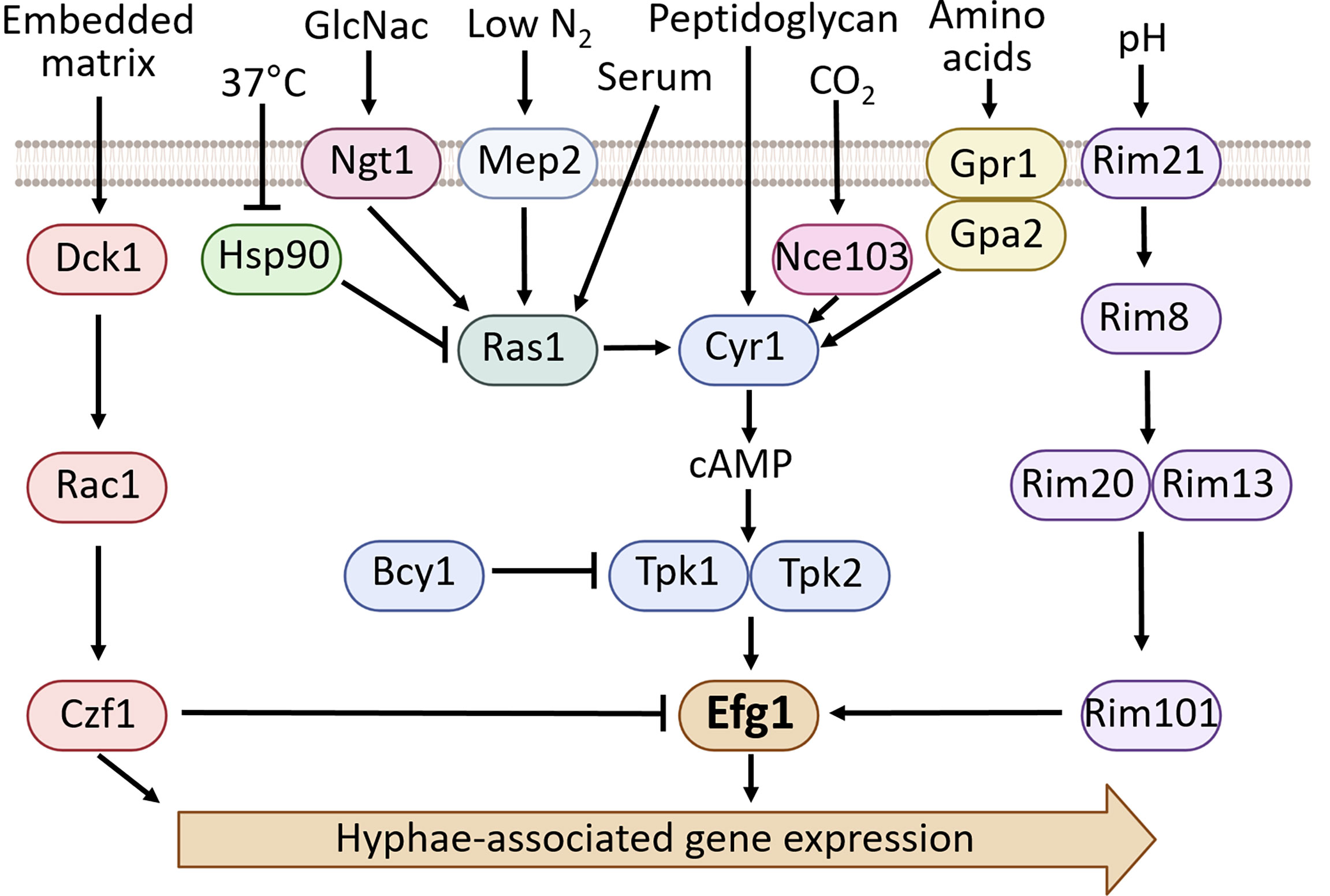
Figure 1 An overview of the environmental cues and factors that regulate hyphae formation in C. albicans, focusing on the signaling pathways involving Efg1 (Sudbery, 2011; Noble et al., 2017; Chen et al., 2020).
The cAMP/PKA pathway is a significant signal transduction pathway for filamentation in C. albicans (Figure 1). In response to environmental cues such as low nitrogen, serum, CO2 or growth in Lee’s media/Spider media there is activation of Cyr1. Cyr1 is an adenylate cyclase which converts ATP to cAMP. The cAMP then binds to Bcy1, the regulatory subunit of the PKA complex (protein kinase A) (Giacometti et al., 2012; Ding et al., 2017). The interaction between cAMP and Bcy1 produces a conformational change that causes the dissociation of Bcy1 from the PKA catalytic subunits Tpk1 and Tpk2. Tpk1 and Tpk2 then phosphorylate target proteins, including Efg1. Epistasis analysis using overexpression of Efg1 in a TPK2 deletion mutant restored filamentation on Spider media, while overexpression of Tpk2 in an EFG1 deletion mutant did not restore filamentation under the same conditions (Sonneborn et al., 2000). Furthermore, mutation of Efg1 residue T208 (previously reported as T206) to a glutamic acid that mimics phosphorylation produces robust filamentation on Spider media, and also rescues filamentation in a TPK2 deletion mutant (Bockmühl and Ernst, 2001). These results suggest that Efg1 functions downstream of Tpk2. Upon activation by the cAMP/PKA pathway, Efg1 binds to the promoter regions of hyphae associated genes, including several genes that encode for transcriptional regulators such as EED1, TEC1 and CRZ1. Efg1 also binds to the EFG1 transcript itself. (Lassak et al., 2011). In addition to binding transcripts directly, Efg1 has been shown to work in combination with Flo8 to regulate gene expression during the transition to hyphal growth in serum (Cao et al., 2006).
Efg1 has been observed within the nuclei of yeast cells under normal growth conditions and also upon hyphal induction. Following hyphal induction both the expression of EFG1 and nuclear levels of Efg1 drop dramatically suggesting Efg1 may be important for the initial transition to hyphae but not continued hyphal growth in serum and Spider media (Tebarth et al., 2003; Bharucha et al., 2011; Saputo et al., 2014). Immediately following the addition of serum to induce filamentation, Efg1 is found bound to the EFG1 promoter, and expression of EFG1 declines dramatically. As such, Efg1 is likely acting as a negative autoregulator of its own expression under these conditions. (Lassak et al., 2011). This downregulation of EFG1 is required for continued hyphal formation as overexpression of EFG1 in the presence of serum prevented the formation of hyphae and instead produced yeast and pseudohyphae cells (Tebarth et al., 2003). It is important to note that EFG1 expression levels demonstrate considerable cell-to-cell variability in standard YPD media (and in the host), therefore the changes in expression patterns that occur following a transition to hyphal induction conditions are representative of what is occurring at the population level (Pierce and Kumamoto, 2012).
In C. albicans pH is a strong regulator of morphology with a low pH environment restricting growth of C. albicans to yeast cell types while a neutral or alkaline pH is permissive to filamentation. Rim101 has been characterized as the primary regulator of pH dependent cellular responses by promoting expression of alkaline-associated genes while repressing acidity-associated genes under neutral pH conditions. Deletion of RIM101 prevents hyphae formation under neutral pH conditions (Davis et al., 2000). Conversely, mutations that result in constitutive activation of Rim101 allow for filamentation at low pH. Deletion of EFG1 in dominant active RIM101 strains suppresses the overactive filamentation phenotype at low pH. This observation suggests that Efg1 functions downstream of Rim101 to promote filamentation in a pH dependent manner (Barkani et al., 2000).
In contrast to its widely described role as a positive regulator of filamentation, Efg1 also acts as a suppressor of filamentation under embedded and hypoxic conditions, as deletion of EFG1 under these conditions causes an increase in filamentation (Giusani et al., 2002; Doedt et al., 2004; Mulhern et al., 2006). The increase in filamentation observed in efg1Δ/efg1Δ mutants under hypoxic conditions occurs at temperatures <37°C suggesting the repressive effects of Efg1 are temperature dependent (Setiadi et al., 2006). Czf1 is also involved in the filamentation pathway under hypoxic conditions with deletion of CZF1 decreasing filamentation in an embedded matrix (Brown Jr. et al., 1999). Epistasis-based genetic models suggest that Czf1 may act to relieve Efg1 suppression of filamentation. Evidence to support this hypothesis can be found in the fact that Czf1 binds Efg1 in a yeast two-hybrid screen (Giusani et al., 2002). Additionally, changes in CZF1 expression (overexpression or deletion) did not alter the hyperfilamentation phenotype of the efg1Δ/efg1Δ mutant in embedded conditions. This would be expected in a model where the effect of Czf1 on filamentation is dependent on suppression of Efg1 (Giusani et al., 2002). EFG1 has also been implicated in the formation of chlamydospores under embedded conditions. These large, round cells can be found attached to suspensor cells and can be induced under specific conditions that include nutrient deprivation and low temperature combined with embedded conditions. Deletion of EFG1 has also been shown to prevent chlamydospore formation despite the efg1Δ/efg1Δ mutant being hyperfilamentous under these conditions (Sonneborn et al., 1999b).
Under normoxic conditions expression of Efg1 is suppressed through interactions with Ifu5 (Rastogi et al., 2020) Following the transition to a hypoxic environment suppression of Efg1 by Ifu5 decreases, and as a result Efg1 expression increases. Under these hypoxic conditions Efg1 downregulates CEK1 and CPH1, components of the CEK1 MAP (Mitogen Activated Protein) kinase cascade required for filamentation (Desai et al., 2015). Together with Bcr1, Efg1 and Bcr1 work together to repress filamentation under hypoxic conditions with elevated CO2 levels (Desai et al., 2015). In addition to repressing filamentation under hypoxic conditions, Efg1 is also responsible for the induction of hypoxia-responsive genes as well as metabolic reprogramming under hypoxic conditions through changes in the expression of genes involved in unsaturated fatty acid metabolism (Setiadi et al., 2006). It is possible that the temperature and hypoxic dependent effects of Efg1 to repress filamentation may act to promote colonization of C. albicans within specific niche microenvironments of the host, however this hypothesis is in conflict with other evidence on the role of Efg1 in gut commensalism discussed below (Desai et al., 2015).
Many of the conditions used for examining filamentation in vitro mimic host conditions in an effort to identify and examine factors that influence in vivo filamentation. A comparison of transcriptomic profiles for multiple solid and liquid filamentation has identified some common filamentation response genes including ALS3, HWP1, DCK1, IHD1, and RBT1, however the transcriptomic profiles for filamentation differ dramatically under different filamentation inducing conditions (Azadmanesh et al., 2017). Additionally, as mentioned previously Efg1 has disparate roles in filamentation under different in vitro conditions. As a result in vitro filamentation may not be an accurate predictor of in vivo filamentation, depending on the type of filamentation inducing conditions used. Despite these caveats it does appear as though media that mimics the host environment, particularly RPMI solid media, is a more accurate predictor of virulence within the host than other forms of media tested (Azadmanesh et al., 2017). A study by Wakade et al. examined the differences between filamentation in vitro and in vivo for several genes associated with filamentation including EFG1 across multiple clinical isolates. Comparison of the filamentation of the efg1Δ/efg1Δ mutant strain to the parental strain in RPMI + 10% serum demonstrated a decrease in filamentation in the efg1Δ/efg1Δ mutant across five of the six clinical isolates tested (the sixth strain, P75010, has significantly reduced filamentation compared to the other C. albicans clinical isolates and therefore the reduction in filamentation observed in the efg1Δ/efg1Δ mutant compared to the parental strain was not statistically significant). Similarly, intravital imagining of efg1Δ/efg1Δ mutants compared to the parental strains in a mouse ear model revealed a statistically significant decrease in filamentation in the efg1Δ/efg1Δ mutants among all clinical isolates tested (Wakade et al., 2021). These results suggest that EFG1 does appear to be a central regulator of filamentation in vivo across multiple clinical isolates.
Efg1 Is a Central Regulator of the Biofilm Transcription Factor Regulatory Network
In addition to being an important regulator of filamentation, Efg1 is one of the six core transcription factors that regulate biofilm formation in C. albicans (Nobile et al., 2012; Glazier et al., 2017). Biofilms are complex highly structured communities of fungi and/or bacteria. C. albicans biofilms are composed of yeast, pseudohyphae and hyphal cells surrounded in an extracellular matrix. Biofilm formation requires several steps that begin with the adhesion of yeast cells to a surface. Following adherence the yeast cells begin to proliferate producing both pseudohyphal and hyphal cells. The increase in cell density allows for the biofilm to develop 3D architecture, aided by the projections of hyphal cells and the synthesis of polysaccharide extracellular matrix. Once the mature biofilm has developed, yeast cells are dispersed to seed new sites of infection (Blankenship and Mitchell, 2006; Nobile and Johnson, 2015). The biofilm transcription factor regulatory network is composed of BRG1, NDT80, ROB1, TEC1, BCR1, and EFG1 (Nobile et al., 2012; Nobile and Johnson, 2015). Transcription factors within this regulatory network control (either directly or indirectly) crucial aspects of biofilm architecture such as the production of adhesion proteins and extracellular matrix material, as well as the morphological transitions between cell types including hyphae formation, dispersal cells, and persister cells.
Given the critical role of Efg1 in filamentation, the biofilm formation defects in the efg1Δ/efg1Δ mutant are often attributed to the inability to form hyphae, as hyphae are required for the architectural stability of the biofilm. However, there is evidence to suggest that biofilm formation is not entirely dependent on filamentation. Deletion of both CPH1 and EFG1 results in a mutant that is unable to form hyphae yet still has some ability to bind to abiotic surfaces and form a biofilm entirely of yeast cells under specific environmental conditions (García-Sánchez et al., 2004). Similarly, overexpression of ALS3 in the efg1Δ/efg1Δ mutant allows for the formation of yeast only biofilms on catheter material in vitro, suggesting that adhesion defects may prevent biofilm formation in the efg1Δ/efg1Δ deletion strain. Additional evidence that adhesion is required for biofilm formation can be found in the BCR1 deletion mutant which has downregulation of several adhesin factors including ALS1, ALS3 and HWP1, causing a biofilm formation defect despite being able to form hyphae (Nobile et al., 2006).
The adhesion of C. albicans to surfaces and the attachment of cells to one another requires several adhesins that are downstream targets of Efg1 including the adhesin proteins ALS1, ECE1 and HWP1 (Braun and Johnson, 2000; Fu et al., 2002; Nobile et al., 2012). Efg1 has also been shown to regulate both ALS3 and EAP1 under serum conditions (Leng et al., 2001; Li and Palecek, 2003; Argimón et al., 2007). Additionally, as mentioned previously, overexpression of ALS3 in the efg1Δ/efg1Δ strain rescues biofilm formation on catheter material in vitro (Zhao et al., 2006). However, Efg1 was not observed to bind ALS3 nor EAP1 in a chromatin immunoprecipitation assay of biofilms formed with Spider media at 37°C (Nobile et al., 2012), despite both ALS3 and EAP1 being required for biofilm formation (Zhao et al., 2006; Li et al., 2007). It is possible that Efg1 binds to the promoter regions of EAP1 and ALS3 under specific environmental cues. Alternatively, the effects of Efg1 on EAP1 and ALS3 may be through indirect interactions with other target proteins. Addition evidence that contradicts a role for EFG1 in adhesion can be found in flow cells assays measuring the adhesion of mutants grown in YPD to a silicone substrate (Finkel et al., 2012). In this assay, the efg1Δ/efg1Δ mutant has a mild, but not statistically significant decrease in adhesion as compared to wild type. These experiments were performed in YPD so it is possible that under different media conditions (including those that elicit biofilm formation) EFG1 may have a greater impact on the adhesion process (Finkel et al., 2012).
Table S1 includes a list of transcripts with twofold change in expression in the efg1Δ/efg1Δ mutant compared to wild type under biofilm conditions that also have been identified as having promoter regions that Efg1 binds to (Nobile et al., 2012). These represent transcripts in which Efg1 is likely directly regulating the expression of. Key components of biofilm formation including ECE1, BRG1, ALS1, NDT80, BCR1 and TEC1 are all downregulated in the absence of EFG1, likely contributing the decrease in biofilm formation observed in the efg1Δ/efg1Δ mutant (Nobile et al., 2012). At least a portion of the phenotype that is produced by a decrease/loss of EFG1 is likely dependent on the activity of Tec1 downstream of Efg1, as overexpression of TEC1 is able to rescue biofilm formation in an efg1Δ/EFG1 heterozygous deletion strain (Glazier et al., 2017).
The biofilm transcription factor regulatory network has been extensively studied in the SC5314 background (Fox and Nobile, 2012; Nobile et al., 2012; Fox et al., 2015; Nobile and Johnson, 2015; Glazier et al., 2017; Mancera et al., 2021). These studies have characterized a fragile network in vitro whereby deletion of a single transcription factor within the network disrupts biofilm formation (Glazier and Krysan, 2018). However recent work comparing the biofilm transcription factor regulatory network in SC5314 to other clinical isolates of Candida albicans has revealed dramatic differences in regulatory circuits that govern biofilm formation between strains (Huang et al., 2019). All of the clinical isolates studied had decreased biofilm formation upon deletion of EFG1, providing support for EFG1 as an essential regulator of biofilm formation in all C. albicans strains. Consistent with what is observed in SC5314, deletion of EFG1 in the 4 clinical isolates tested also resulted in decreased expression of ALS1 and BRG1. However deletion of EFG1 decreased expression of ECE1 in only three of the four clinical isolate strains studied. Similarly, only two of the four clinical isolate strains had a decrease in TEC1 expression when EFG1 was deleted (Huang et al., 2019). Therefore although Efg1 is a central regulator of biofilm formation across all isolates tested, is appears to regulate different subsets of the transcripts required for biofilm formation among the various clinical isolates.
In this context, the fragility of the biofilm network observed in SC5314 raises an interesting evolutionary question around how such a fragile transcription factor regulatory network could have evolved. The SC5314 biofilm transcription factor regulatory network has a lower than expected number of genes with close homologs in other Candida species (Mancera et al., 2021). This observation combined with the lower than expected frequency of Ndt80 and Efg1 consensus elements in other closely related species suggests the C. albicans biofilm regulatory network may be a more recent evolutionary adaptation. Additionally, ChIP-chip analysis of the transcription factor binding sites to target genes promoters has identified larger than expected promoter regions, which may facilitate relatively quick incorporation of new target genes into the regulatory network (Nobile et al., 2012; Mancera et al., 2021). Based on these observations it is possible that some of the disparities between the different clinical isolates are due to the incorporation of targets into the biofilm transcription factor regulatory network that do not directly contribute to biofilm adaptation. These target genes are then maintained within in a given clinical isolate due to neutral (non-adaptive) selection. However this hypothesis does not explain why the core transcription factor regulatory networks between the clinical isolates also seems fundamentally altered. The variability in biofilm regulatory circuitry in clinical isolates reinforces the need to study additional C. albicans strains to determine the commonalities between the regulatory circuitry patterns that govern biofilm formation across multiple isolates. This variability also highlights the dynamic capacity for C. albicans to maintain robust biofilm formation using different transcription factor network architecture in different strains (Huang et al., 2019).
Efg1 Is Required for Transition to, and Maintenance of, the White State
In addition to yeast cells, pseudohyphae and hyphal morphology, C. albicans is also able to transition while in the yeast confirmation between white, gray, and opaque phenotypes. Characterized by their colony appearance, the white, gray, and opaque states have specific cellular morphology, metabolic programing, cell wall traits, as well as effects on both virulence and commensalism. The transcription factor regulatory network that controls white/gray/opaque phenotype switching includes Efg1, Wor1, Wor2, Wor3, Ahr1 and Czf1 (Hernday et al., 2013). Efg1 is highly expressed in the white cell state. Overexpression of EFG1 causes opaque state cells to transition to the white state (Sonneborn et al., 1999a). Conversely, in opaque cells, EFG1 is expressed at low levels. Deletion of EFG1 produces cells that form an intermediate gray state in a/a, α/α cell types as well as some a/α clinical isolates (Sonneborn et al., 1999a; Srikantha et al., 2000; Zordan et al., 2007; Xie et al., 2013; Liang et al., 2019; Park et al., 2019; Park et al., 2020). Once in the gray state, efg1Δ/efg1Δ mutants in a/a and α/α MLT strains have a high rate of transition to the opaque state. Hemizygosity of EFG1, which is often seen in clinical isolates, produces cell types that can switch to the intermediate gray state and then undergo additional conversion to the opaque state depending on environmental conditions and selection pressures (Liang et al., 2019).
In white cells, the increased expression of Efg1 allows for Efg1 to repress expression of WOR1, the master regulator of the opaque state, thereby maintaining the cells in the white state. The repression of WOR1 by Efg1 is direct, as Efg1 binds to the WOR1 promoter in white cells (Hernday et al., 2013). Brg1 has also been identified as binding to the WOR1 promoter under biofilm conditions (Nobile et al., 2012). Evidence that deletion of either Brg1 or Efg1 in a heterozygous MTL a/α strain allows for white to opaque switching suggests that Brg1 may also repress WOR1 expression in conjunction with Efg1, however further research is necessary (Xie et al., 2013). Efg1 also acts with Ahr1 (Zcf37) to repress WOR2 (Zordan et al., 2007; Sriram et al., 2009; Wang et al., 2011). WOR2 is required for maintenance of the opaque state, with WOR1 and WOR2 forming a positive feedback loop promoting the opaque cell type (Zordan et al., 2007; Sriram et al., 2009). Ahr1 binds to the promoter of EFG1 and WOR2, therefore in addition to Efg1 acting in concert with Ahr1 to downregulate WOR2, Ahr1 may also regulate EFG1 expression (Hernday et al., 2013). In summary Efg1 represses WOR1 both directly by binding to the promoter of WOR1 and indirectly through repression of WOR2.
In the opaque state EFG1 expression is downregulated by both Czf1 and Wor1. Czf1 represses EFG1 expression in opaque cells, which in turn results in increased expression of WOR2, facilitating the transition to the opaque state (Sriram et al., 2009; Hernday et al., 2013). Wor1 also binds to the promoter of EFG1 acting as a repressor of EFG1 expression (Zordan et al., 2007; Pujol et al., 2016). A transcriptomic analysis comparing white and opaque cell types identified a shortening of the 5’UTR (untranslated region) of EFG1 during the opaque state with the transcription start site near the Wor1 binding site. This data suggests that Wor1 may direct changes in the transcription start site producing a shorter 5’ UTR of EFG1 while cells are in the opaque state (Tuch et al., 2010). 5’UTR modifications are known to influence ribosomal recruitment and therefore translation. It is possible that the shorter 5’ UTR of EFG1 during the opaque state may modify post-translational regulation of the EFG1 transcript. In particular, the shorter 5’UTR may result in changes in ribosomal recruitment that decrease translation of Efg1. Table S1 includes microarray results comparing efg1Δ/efg1Δ mutant and wild type transcriptomic profiles in both white and opaque states (Hernday et al., 2013). The data has been filtered to only include genes with Efg1 bound to the promoter region that also demonstrate a twofold or greater change in gene expression when EFG1 is deleted.
Efg1 Has Opposing Functions in Commensalism and Invasive Infection
A similar dynamic between Efg1 and Wor1 observed in white/gray/opaque switching is also found in the regulation of commensalism within the gut. In the mouse gastrointestinal (GI) tract Wor1 acts as a driver for the GUT phenotype, facilitating commensalism within the gastrointestinal tract, with deletion of WOR1 causing decreased fitness in a commensalism model (Pande et al., 2013). Conversely, Efg1 inhibits commensalism with the efg1Δ/efg1Δ mutant displaying a gray phenotype (similar to the GUT phenotype) and increased commensalism, outcompeting wild type cells in a mouse model of gastrointestinal candidiasis (Pierce and Kumamoto, 2012; Pande et al., 2013; Cottier and Hall, 2020). Calling card-seq, a method by which transcription factor binding sites can be mapped using transposons in vivo during GI colonization, show that Czf1 binds EFG1 (Witchley et al., 2021). Additionally gut colonization of the double deletion mutant efg1Δ/efg1Δ and czf1Δ/czf1Δ produces the same phenotype as efg1Δ/efg1Δ alone. These results suggest the effect of Czf1 on commensalism is EFG1 dependent, supporting a similar transcription factor regulatory network to what is seen in white/gray/opaque switching where Czf1 regulates EFG1 expression (Witchley et al., 2021).
It is speculated that Efg1 likely inhibits commensalism within the gastrointestinal tract through multiple mechanisms. The first method is by inhibiting Wor1, which as mentioned previously, is the central regulator of the GUT phenotype and required for commensalism. Efg1 also represses expression of other transcripts required for gut colonization including CHT2 which encodes a GPI-linked chitinase (Witchley et al., 2021). The third mechanism is through upregulating hyphal specific genes, particularly SAP6 which encodes a pro-inflammatory aspartyl protease. Sap6 has been hypothesized to trigger a localized immune response that restricts invasive hyphal growth in the GI tract (Pietrella et al., 2010; Witchley et al., 2019; Witchley et al., 2021). Similar dynamics are seen when other transcription factors that promote hyphal formation are deleted, including BRG1, ROB1, TEC1, and UME6. Deletion of any one of these transcription factors produced mutants with increased commensalism as compared to wild type. It is worth noting that hyphae can still be found in parts of the gut, particularly the large intestine and cecum, where several drivers of filamentation, N-acetylglucosamine, hypoxia, and high levels of CO2 likely promote hyphae formation (Witchley et al., 2019).
Evidence for a multifaceted role of Efg1 in commensalism is supported by the GI fitness of the efg1Δ/efg1Δ wor1Δ/wor1Δ double mutant which displays an intermediate GI fitness between efg1Δ/efg1Δ and wor1Δ/wor1Δ single deletion mutants. In this double deletion mutant the loss of Wor1, the driver of the GUT phenotype, decreases commensalism. Yet the mutant presumably is still has higher levels of CHT2 and lower levels of the hypha specific transcripts such as SAP6, similar to the efg1Δ/efg1Δ mutant. The combination of factors likely results in the intermediate GI fitness (Witchley et al., 2021). A list of genes differentially regulated between wild type and the efg1Δ/efg1Δ during gut commensalism can be found in Table S1 (Witchley et al., 2021). Transcripts were filtered by a twofold change in expression compared to wild type, and the ability of Efg1 to bind the promoter region of the gene.
There is conflicting evidence for the role of Efg1 in oropharyngeal candidiasis. In a gnotobiotic pig model of oropharyngeal candidiasis efg1Δ/efg1Δ mutants are able to form hyphae (Riggle et al., 1999). Similarly, deletion of EFG1 produces a variable fungal burden in an oropharyngeal candidiasis mouse model with the efg1Δ/efg1Δ mutants still able to form hyphae in vivo (Solis et al., 2022). However in another oropharyngeal candidiasis mouse model deletion of EFG1 decreased fungal burden with the efg1Δ/efg1Δ mutant strain forming yeast and short pseudohyphae (Park et al., 2005). It is possible that the differences in morphology and virulence may be attributable to differences in the host model (male CD-1 compared to female BALB/c mice) and/or strain (SN background compared to CAI). Future studies are required to determine whether the variability in the role of EFG1 in OPC virulence is due to diversification of the transcription factor regulatory network that governs OPC, as seen in the biofilm regulatory network which includes EFG1 (Huang et al., 2019), or whether the differences observed are due to nuances in the experimental techniques and/or models.
Much of the work done on interactions between the host immune system and C. albicans has focused on the cph1Δ/chp1Δ efg1Δ/efg1Δ yeast locked strain, and it is therefore hard to deduce the role of Efg1 versus Cph1. However EFG1 is required for the transition to hyphae in both macrophages and neutrophils (Korting et al., 2003; McKenzie et al., 2010; Wellington et al., 2012). Deletion of EFG1 results in cells that are unable to trigger macrophage lysis as measured by LDH release (Wellington et al., 2012). Studies on macrophage activation following engulfment of efg1Δ/efg1Δ mutants have shown that these mutants are unable to activate caspase 1, trigger the ROS response, and have macrophage responses with lower levels of IL-1β, TNF-α, IL-4, and IL-23 cytokine production. Changes in immunogenicity of the efg1Δ/efg1Δ mutants compared to wild type is likely due to a combination of changes on the surface of the cell including changes in cell wall architecture (Wellington et al., 2012; Zavrel et al., 2012).
Lower levels of EFG1 results in a fitness advantage within the gut when compared to cells with higher levels of EFG1 expression (Pierce and Kumamoto, 2012; Pande et al., 2013). It is perhaps not surprising then that examination of clinical isolates has identified several strains that were naturally hemizygous for EFG1 (Liang et al., 2019). Passaging through the mouse GI tract results in decreased expression of EFG1 with clinical isolates that are hemizygous for EFG1 further transitioning into an EFG1 null state, supporting the GI fitness advantage conferred by loss of EFG1 expression (Liang et al., 2019). Similarly, evolution studies using SC5314 passage through the GI tract of mice treated with antibiotics produced mutants homozygous for de novo non-synonymous mutations in EFG1, again suggesting a selective pressure whereby loss of EFG1 increases fitness in the gastrointestinal tract (Tso et al., 2018). Although decreasing EFG1 expression produces a fitness advantage within the gastrointestinal tract, EFG1 is required for invasive disease with efg1Δ/efg1Δ mutants being avirulent in a tail vein injection mouse model (Lo et al., 1997; Pierce and Kumamoto, 2012; Pande et al., 2013). EFG1 expression levels are also dependent on the immune status of the host, with EFG1 expression decreasing in the gastrointestinal tract in an immunocompromised mouse model (Pierce and Kumamoto, 2012). Taken together, these observations suggest EFG1 expression is dynamic, highly variable, and that control of Efg1 levels is essential to the survival of C. albicans as both a pathogen and commensal. It is likely that within the host different populations of C. albicans express varying levels of EFG1 in response to environmental factors present within the niche site, and that the selection pressures within these sites select for mutations that results in changes to EFG1 expression which confer increased fitness under those conditions. This natural genetic diversity of C. albicans within a single host has been proposed to allow rapid adaptation in response to changes in the host environment (Sitterlé et al., 2019).
Efg1 Has Both Shared and Distinct Targets Under Different Environmental Conditions
Efg1 has been observed to have different consensus elements and different targets depending on environmental conditions, likely as the result of changes in phosphorylation patterns and/or interactions with other transcription factors. These differences in targets allow for Efg1 to function as a regulator of the very different morphological programs required for of gut commensalism, filamentation, biofilm formation, and the white state. By compiling the information on Efg1 binding to targets along with transcriptomic changes in EFG1 mutants we can compare the functionally relevant Efg1 targets differentially regulated in gut commensalism, biofilm formation, and transition between white and opaque states (Figure 2) (Nobile et al., 2012; Hernday et al., 2013; Witchley et al., 2021). Targets were included if they exhibit both Efg1 binding to the promoter regions and a twofold change in gene expression when EFG1 was deleted. The core set of target genes that are shared under all three conditions include RFG1, BAS1, PHO84, CUP9, ADAEC, AAF1, TYE7, and EFG1. Of the share target genes, RFG1, BAS1, CUP9, and TYE7 all encode transcription factors, consistent with the previously describe roles of Efg1 in multiple transcription factor regulatory networks (Nobile et al., 2012; Hernday et al., 2013; Rodriguez et al., 2020). Efg1, as previously mentioned, has been showed to bind its own promoter region and appears to be autoregulatory in all three conditions. The overlap in targets is highest between biofilm formation and commensalism, which may reflect similar roles of Efg1 in hyphal morphogenesis. While the overlap in targets between commensalism and the white/opaque state may reflect similarities in the efg1Δ/efg1Δ mutants to both GUT and gray/opaque phenotypes.
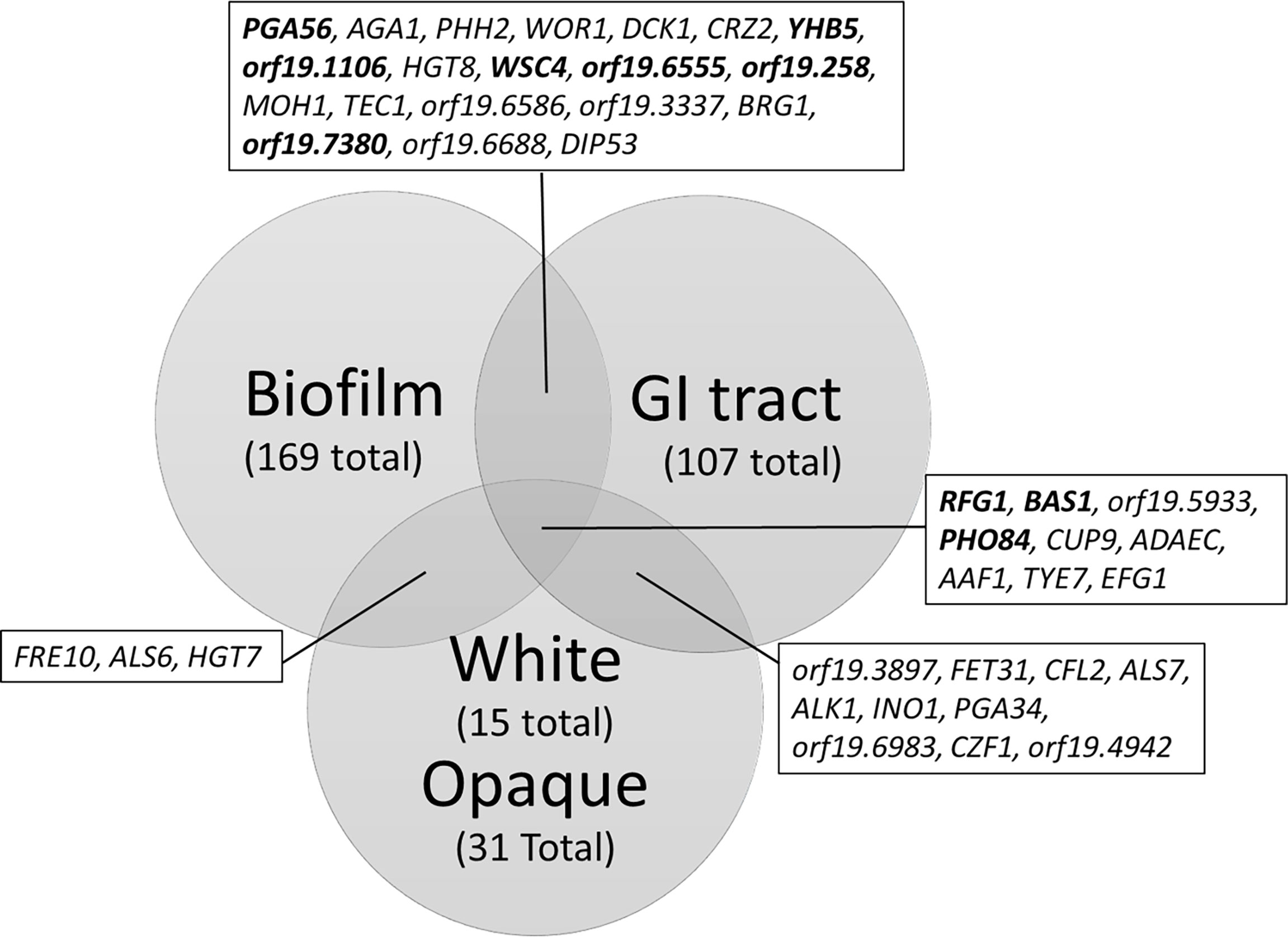
Figure 2 Venn Diagram of Efg1 targets during biofilm formation, gut commensalism, and either the white or opaque state. Transcriptomic studies comparing the efg1Δ/Δ deletion mutant and wild type strains under the conditions listed were used to determine transcripts with twofold changes in expression upon deletion of EFG1. Transcripts were then filtered by whether Efg1 is able to bind to the promoter regions of the encoding gene to create a list of Efg1 targets with differential expression upon deletion of EFG1. Bold identifies transcripts that are differentially regulated (upregulated in one condition, downregulate in another). Data adapted from (Nobile et al., 2012; Hernday et al., 2013; Witchley et al., 2021).
Conclusions
In the roughly 25 years since EFG1 was first characterized in Candida albicans, EFG1 has been explored in nearly all contents of C. albicans biology. Despite its popularity, or perhaps because of its popularity, several key questions remain with regard to Efg1 regulation and activity. One key question is relates to the activation and phosphorylation of Efg1 and whether differences in the consensus elements are governed by changes in phosphorylation status of Efg1, context specific interactions with other protein partners, or both. Another relatively unexplored aspect of EFG1 is the post-transcriptional regulatory processes that control EFG1 expression. Finally, given that EFG1 expression within the host and Efg1 function in the regulatory network for biofilm formation is variable between clinical isolates, can we better understand the content of the changes in EFG1 expression/function between strains to gain a more comprehensive understanding of C. albicans pathogenesis as a whole?
Author Contributions
The author confirms being the sole contributor of this work and has approved it for publication.
Funding
ACIS funding through Niagara University.
Conflict of Interest
The author declares that the research was conducted in the absence of any commercial or financial relationships that could be construed as a potential conflict of interest.
Publisher’s Note
All claims expressed in this article are solely those of the authors and do not necessarily represent those of their affiliated organizations, or those of the publisher, the editors and the reviewers. Any product that may be evaluated in this article, or claim that may be made by its manufacturer, is not guaranteed or endorsed by the publisher.
Supplementary Material
The Supplementary Material for this article can be found online at: https://www.frontiersin.org/articles/10.3389/fcimb.2022.855229/full#supplementary-material
Supplementary Table 1 | Efg1 targets with two fold change upon deletion of EFG1. (A) Biofilm- Changes in RNA expression between the efg1Δ/Δ deletion mutant and wild type under biofilm formation conditions (Spider medium at 37°C in 6-well polystyrene plates) determined by RNA sequencing. Efg1 binding sites determined by chromatin immunoprecipitation tiling microarray (ChIP-chip) using Efg1-myc (Nobile et al., 2012). (B) White/opaque state- changes in RNA expression between the efg1Δ/Δ deletion mutant and wild type using gene expression microarray of cells in mid-log phase in synthetic complete media, Efg1 binding sites determined by ChIP-chip using Efg1 specific polyclonal antibodies (Hernday et al., 2013). (C) Gastrointestinal tract- changes in RNA expression between the efg1Δ/Δ deletion mutant and wild type in a commensalism mouse model within the gastrointestinal tract measured by RNA seq. Efg1 binding sites determined using Calling Card-seq with Efg1 fused to PiggyBac transposase (Witchley et al., 2021).
References
Argimón, S., Wishart, J. A., Leng, R., Macaskill, S., Mavor, A., Alexandris, T., et al. (2007). Developmental Regulation of an Adhesin Gene During Cellular Morphogenesis in the Fungal Pathogen Candida Albicans. Eukaryot. Cell 6 (4), 682–692. doi: 10.1128/EC.00340-06
Azadmanesh, J., Gowen, A. M., Creger, P. E., Schafer, N. D., Blankenship, J. R. (2017). Filamentation Involves Two Overlapping, But Distinct, Programs of Filamentation in the Pathogenic Fungus Candida Albicans. G3 Genes Genomes Genet. 7 (11), 3797–3808. doi: 10.1534/g3.117.300224
Barkani, A. E., Kurzai, O., Fonzi, W. A., Ramon, A., Porta, A., Frosch, M., et al. (2000). Dominant Active Alleles of RIM101 (PRR2) Bypass the PH Restriction on Filamentation of Candida Albicans. Mol. Cell. Biol. 20 (13), 4635–4647. doi: 10.1128/MCB.20.13.4635-4647.2000
Bharucha, N., Chabrier-Roselló, Y., Xu, T., Johnson, C., Sobczynski, S., Song, Q., et al. (2011). A Large-Scale Complex Haploinsufficiency-Based Genetic Interaction Screen in Candida Albicans: Analysis of the RAM Network During Morphogenesis. PloS Genet. 7 (4), e1002058. doi: 10.1371/journal.pgen.1002058
Blankenship, J. R., Mitchell, A. P. (2006). How to Build a Biofilm: A Fungal Perspective. Curr. Opin. Microbiol. 9 (6), 588–594. doi: 10.1016/j.mib.2006.10.003
Bockmühl, D. P., Ernst, J. F. (2001). A Potential Phosphorylation Site for an A-Type Kinase in the Efg1 Regulator Protein Contributes to Hyphal Morphogenesis of Candida Albicans. Genetics 157 (4), 1523–1530. doi: 10.1093/genetics/157.4.1523
Bockmühl, D. P., Krishnamurthy, S., Gerads, M., Sonneborn, A., Ernst, J. F. (2001). Distinct and Redundant Roles of the Two Protein Kinase A Isoforms Tpk1p and Tpk2p in Morphogenesis and Growth of Candida Albicans. Mol. Microbiol. 42 (5), 1243–1257. doi: 10.1046/j.1365-2958.2001.02688.x
Braun, B. R., Johnson, A. D. (2000). TUP1, CPH1 and EFG1 Make Independent Contributions to Filamentation in Candida Albicans. Genetics 155 (1), 57–67. doi: 10.1093/genetics/155.1.57
Brown, D. H., Jr., Giusani, A. D., Chen, X., Kumamoto, C. A. (1999). Filamentous Growth of Candida Albicans in Response to Physical Environmental Cues and Its Regulation by the Unique CZF1 Gene. Mol. Microbiol. 34 (4), 651–662. doi: 10.1046/j.1365-2958.1999.01619.x
Buffo, J., Herman, M. A., Soll, D. R. (1984). A Characterization of pH-Regulated Dimorphism in Candida Albicans. Mycopathologia 85 (1–2), 21–30. doi: 10.1007/BF00436698
Cao, F., Lane, S., Pala Raniga, P., Lu, Y., Zhou, Z., Ramon, K., et al. (2006). The Flo8 Transcription Factor Is Essential for Hyphal Development and Virulence in Candida Albicans. Mol. Biol. Cell 17 (1), 295–307. doi: 10.1091/mbc.e05-06-0502
Cao, C., Wu, M., Bing, J., Tao, L., Ding, X., Liu, X., et al. (2017). Global Regulatory Roles of the cAMP/PKA Pathway Revealed by Phenotypic, Transcriptomic and Phosphoproteomic Analyses in a Null Mutant of the PKA Catalytic Subunit in Candida Albicans. Mol. Microbiol. 105 (1), 46–64. doi: 10.1111/mmi.13681
Chen, H., Zhou, X., Ren, B., Cheng, L. (2020). The Regulation of Hyphae Growth in Candida Albicans. Virulence 11 (1), 337–348. doi: 10.1080/21505594.2020.1748930
Cottier, F., Hall, R. A. (2020). Face/Off: The Interchangeable Side of Candida Albicans. Front. Cell. Infect. Microbiol. 9. doi: 10.3389/fcimb.2019.00471
Cullen, P. J., Sprague, G. F. (2012). The Regulation of Filamentous Growth in Yeast. Genetics 190 (1), 23–49. doi: 10.1534/genetics.111.127456
Davis, D., Bryce Wilson, R., Mitchell, A. P. (2000). RIM101-Dependent and -Independent Pathways Govern pH Responses in Candida Albicans. Mol. Cell. Biol. 20 (3), 971–978. doi: 10.1128/MCB.20.3.971-978.2000
Desai, P. R., van Wijlick, L., Kurtz, D., Juchimiuk, M., Ernst, J. F. (2015). Hypoxia and Temperature Regulated Morphogenesis in Candida Albicans. PloS Genet. 11 (8), e1005447. doi: 10.1371/journal.pgen.1005447
Ding, X., Cao, C., Zheng, Q., Huang, G. (2017). The Regulatory Subunit of Protein Kinase A (Bcy1) in Candida Albicans Plays Critical Roles in Filamentation and White-Opaque Switching But Is Not Essential for Cell Growth. Front. Microbiol. 7. doi: 10.3389/fmicb.2016.02127
Doedt, T., Krishnamurthy, S., Bockmühl, D. P., Tebarth, B., Stempel, C., Russell, C. L., et al. (2004). APSES Proteins Regulate Morphogenesis and Metabolism in Candida Albicans. Mol. Biol. Cell 15 (7), 3167–3180. doi: 10.1091/mbc.e03-11-0782
Finkel, J. S., Xu, W., Huang, D., Hill, E. M., Desai, J. V., Woolford, C. A., et al. (2012). Portrait of Candida Albicans Adherence Regulators. PloS Pathog. 8 (2), e1002525. doi: 10.1371/journal.ppat.1002525
Fox, E. P., Bui, C. K., Nett, J. E., Hartooni, N., Mui, M. C., Andes, D. R., et al. (2015). An Expanded Regulatory Network Temporally Controls Candida Albicans Biofilm Formation. Mol. Microbiol. 96 (6), 1226–1239. doi: 10.1111/mmi.13002
Fox, E. P., Nobile, C. J. (2012). A Sticky Situation. Transcription 3 (6), 315–322. doi: 10.4161/trns.22281
Frazer, C., Staples, M. I., Kim, Y., Hirakawa, M., Dowell, M. A., Johnson, N. V., et al. (2020). Epigenetic Cell Fate in Candida Albicans Is Controlled by Transcription Factor Condensates Acting at Super-Enhancer-Like Elements. Nat. Microbiol. 5 (11), 1374–1389. doi: 10.1038/s41564-020-0760-7
Fu, Y., Ibrahim, A. S., Sheppard, D. C., Chen, Y.-C., French, S. W., Cutler, J. E., et al. (2002). Candida Albicans Als1p: An Adhesin That Is a Downstream Effector of the EFG1 Filamentation Pathway. Mol. Microbiol. 44 (1), 61–725. doi: 10.1046/j.1365-2958.2002.02873.x
García-Sánchez, S., Aubert, S., Iraqui, I., Janbon, G., Ghigo, J.-M., d’Enfert, C. (2004). Candida Albicans Biofilms: A Developmental State Associated With Specific and Stable Gene Expression Patterns. Eukaryot. Cell 3 (2), 536–545. doi: 10.1128/EC.3.2.536-545.2004
Giacometti, R., Kronberg, F., Biondi, R. M., Hernández, A. I., Passeron, S. (2012). Cross Regulation Between Candida Albicans Catalytic and Regulatory Subunits of Protein Kinase A. Fungal Genet. Biol. 49 (1), 74–85. doi: 10.1016/j.fgb.2011.12.001
Giusani, A. D., Vinces, M., Kumamoto, C. A. (2002). Invasive Filamentous Growth of Candida Albicans Is Promoted by Czf1p-Dependent Relief of Efg1p-Mediated Repression. Genetics 160 (4), 1749–1753. doi: 10.1093/genetics/160.4.1749
Glazier, V. E., Krysan, D. J. (2018). Transcription Factor Network Efficiency in the Regulation of Candida Albicans Biofilms: It Is a Small World. Curr. Genet. 64 (4), 883–888. doi: 10.1007/s00294-018-0804-1
Glazier, V. E., Murante, T., Murante, D., Koselny, K., Liu, Y., Kim, D., et al. (2017). Genetic Analysis of the Candida Albicans Biofilm Transcription Factor Network Using Simple and Complex Haploinsufficiency. PloS Genet. 13 (8), e1006948. doi: 10.1371/journal.pgen.1006948
Hernday, A. D., Lohse, M. B., Fordyce, P. M., Nobile, C. J., DeRisi, J. L., Johnson, A. D. (2013). Structure of the Transcriptional Network Controlling White-Opaque Switching in Candida Albicans. Mol. Microbiol. 90 (1), 22–35. doi: 10.1111/mmi.12329
Huang, M. Y., Woolford, C. A., May, G., McManus, C. J., Mitchell, A. P. (2019). Circuit Diversification in a Biofilm Regulatory Network. PloS Pathog. 15 (5), e1007787. doi: 10.1371/journal.ppat.1007787
Jones, S. (2004). An Overview of the Basic Helix-Loop-Helix Proteins. Genome Biol. 5 (6), 226. doi: 10.1186/gb-2004-5-6-226
Klengel, T., Liang, W.-J., Chaloupka, J., Ruoff, C., Schröppel, K., Naglik, J. R., et al. (2005). Fungal Adenylyl Cyclase Integrates CO2 Sensing With cAMP Signaling and Virulence. Curr. Biol. 15 (22), 2021–2026. doi: 10.1016/j.cub.2005.10.040
Koch, B., Barugahare, A. A., Lo, T. L., Huang, C., Schittenhelm, R. B., Powell, D. R., et al. (2018). A Metabolic Checkpoint for the Yeast-To-Hyphae Developmental Switch Regulated by Endogenous Nitric Oxide Signaling. Cell Rep. 25 (8), 2244–2258.e7. doi: 10.1016/j.celrep.2018.10.080
Korting, H. C., Hube, B., Oberbauer, S., Januschke, E., Hamm, G., Albrecht, A., et al. (2003). Reduced Expression of the Hyphal-Independent Candida Albicans Proteinase Genes SAP1 and SAP3 in the Efg1 Mutant Is Associated With Attenuated Virulence During Infection of Oral Epithelium. J. Med. Microbiol. 52 (8), 623–325. doi: 10.1099/jmm.0.05125-0
Lassak, T., Schneider, E., Bussmann, M., Kurtz, D., Manak, J. R., Srikantha, T., et al. (2011). Target Specificity of the Candida Albicans Efg1 Regulator. Mol. Microbiol. 82 (3), 602–618. doi: 10.1111/j.1365-2958.2011.07837.x
Lee, K. L., Buckley, H. R., Campbell, C. C. (1975). An Amino Acid Liquid Synthetic Medium for the Development of Mycelial and Yeast Forms of Candida Albicans. Sabouraudia 13 (2), 148–153. doi: 10.1080/00362177585190271
Lee, S.-H., Chung, S.-C., Shin, J., Oh, K.-B. (2014). GST2 Is Required for Nitrogen Starvation-Induced Filamentous Growth in Candida Albicans. J. Microbiol. Biotechnol. 24 (9), 1207–1215. doi: 10.4014/jmb.1405.05012
Leng, P., Lee, P. R., Wu, H., Brown, A. J. P. (2001). Efg1, a Morphogenetic Regulator in Candida Albicans, Is a Sequence-Specific DNA Binding Protein. J. Bacteriol. 183 (13), 4090–4093. doi: 10.1128/JB.183.13.4090-4093.2001
Liang, S.-H., Anderson, M. Z., Hirakawa, M. P., Wang, J. M., Frazer, C., Alaalm, L. M., et al. (2019). Hemizygosity Enables a Mutational Transition Governing Fungal Virulence and Commensalism. Cell Host Microbe 25 (3), 418–431.e6. doi: 10.1016/j.chom.2019.01.005
Li, F., Palecek, S. P. (2003). EAP1, a Candida Albicans Gene Involved in Binding Human Epithelial Cells. Eukaryot. Cell 2 (6), 1266–1273. doi: 10.1128/EC.2.6.1266-1273.2003
Li, F., Svarovsky, M. J., Karlsson, A. J., Wagner, J. P., Marchillo, K., Oshel, P., et al. (2007). Eap1p, an Adhesin That Mediates Candida Albicans Biofilm Formation In Vitro and In Vivo. Eukaryot. Cell 6 (6), 931–939. doi: 10.1128/EC.00049-07
Liu, H., Köhler, J., Fink, G. R. (1994). Suppression of Hyphal Formation in Candida Albicans by Mutation of a STE12 Homolog. Science 266 (5191), 1723–1726. doi: 10.1126/science.7992058
Lo, H.-J., Köhler, J. R., DiDomenico, B., Loebenberg, D., Cacciapuoti, A., Fink, G. R. (1997). Nonfilamentous C. Albicans Mutants Are Avirulent. Cell 90 (5), 939–949. doi: 10.1016/S0092-8674(00)80358-X
Lu, Y., Su, C., Mao, X., Pala Raniga, P., Liu, H., Chen, J. (2008). Efg1-Mediated Recruitment of NuA4 to Promoters Is Required for Hypha-Specific Swi/Snf Binding and Activation in Candida Albicans. Mol. Biol. Cell 19 (10), 4260–4272. doi: 10.1091/mbc.E08-02-0173
Lu, Y., Su, C., Wang, A., Liu, H. (2011). Hyphal Development in Candida Albicans Requires Two Temporally Linked Changes in Promoter Chromatin for Initiation and Maintenance. PloS Biol. 9 (7), e1001105. doi: 10.1371/journal.pbio.1001105
Mancera, E., Nocedal, I., Hammel, S., Gulati, M., Mitchell, K. F., Andes, D. R., et al. (2021). Evolution of the Complex Transcription Network Controlling Biofilm Formation in Candida Species. ELife 10 (April), e64682. doi: 10.7554/eLife.64682
Mayhew, D., Mitra, R. D. (2014). Transcription Factor Regulation and Chromosome Dynamics During Pseudohyphal Growth. Mol. Biol. Cell 25 (17), 2669–2676. doi: 10.1091/mbc.E14-04-0871
McKenzie, C. G. J., Koser, U., Lewis, L. E., Bain, J. M., Mora-Montes, H. M., Barker, R. N., et al. (2010). Contribution of Candida Albicans Cell Wall Components to Recognition by and Escape From Murine Macrophages. Infect. Immun 78 (4), 1650–1658. doi: 10.1128/IAI.00001-10
Min, K., Jannace, T. F., Si, H., Veeramah, K. R., Haley, J. D., Konopka, J. B. (2021). Integrative Multi-Omics Profiling Reveals cAMP-Independent Mechanisms Regulating Hyphal Morphogenesis in Candida Albicans. PloS Pathog. 17 (8), e1009861. doi: 10.1371/journal.ppat.1009861
Mulhern, S. M., Logue, M. E., Butler, G. (2006). Candida Albicans Transcription Factor Ace2 Regulates Metabolism and Is Required for Filamentation in Hypoxic Conditions. Eukaryot. Cell 5 (12), 2001–2013. doi: 10.1128/EC.00155-06
Nasution, O., Srinivasa, K., Kim, M., Kim, Y.-J., Kim, W., Jeong, W., et al. (2008). Hydrogen Peroxide Induces Hyphal Differentiation in Candida Albicans. Eukaryot. Cell 7 (11), 2008–2011. doi: 10.1128/EC.00105-08
Nobile, C. J., Andes, D. R., Nett, J. E., Smith, F. J., Jr., Yue, F., Phan, Q.-T., et al. (2006). Critical Role of Bcr1-Dependent Adhesins in C. Albicans Biofilm Formation In Vitro and In Vivo. PloS Pathog. 2 (7), e63. doi: 10.1371/journal.ppat.0020063
Nobile, C. J., Fox, E. P., Nett, J. E., Sorrells, T. R., Mitrovich, Q. M., Hernday, A. D., et al. (2012). A Recently Evolved Transcriptional Network Controls Biofilm Development in Candida Albicans. Cell 148 (1–2), 126–138. doi: 10.1016/j.cell.2011.10.048
Nobile, C. J., Johnson, A. D. (2015). Candida Albicans Biofilms and Human Disease. Annu. Rev. Microbiol. 69, 71–92. doi: 10.1146/annurev-micro-091014-104330
Noble, S. M., Gianetti, B. A., Witchley, J. N. (2017). Candida Albicans Cell-Type Switching and Functional Plasticity in the Mammalian Host. Nat. Rev. Microbiol. 15 (2), 96–108. doi: 10.1038/nrmicro.2016.157
Noffz, C. S., Liedschulte, V., Lengeler, K., Ernst, J. F. (2008). Functional Mapping of the Candida Albicans Efg1 Regulator. Eukaryot. Cell 7 (5), 881–893. doi: 10.1128/EC.00033-08
Pande, K., Chen, C., Noble, S. M. (2013). Passage Through the Mammalian Gut Triggers a Phenotypic Switch That Promotes Candida Albicans Commensalism. Nat. Genet. 45 (9), 1088–1091. doi: 10.1038/ng.2710
Pan, X., Heitman, J. (2000). Sok2 Regulates Yeast Pseudohyphal Differentiation via a Transcription Factor Cascade That Regulates Cell-Cell Adhesion. Mol. Cell. Biol. 20 (22), 8364–8372. doi: 10.1128/MCB.20.22.8364-8372.2000
Park, Y. N., Conway, K., Conway, T. P., Daniels, K. J., Soll, D. R. (2019). Roles of the Transcription Factors Sfl2 and Efg1 in White-Opaque Switching in a/α Strains of Candida Albicans. MSphere 4 (2), e00703-18. doi: 10.1128/mSphere.00703-18
Park, Y.-N., Conway, K., Pujol, C., Daniels, K. J., Soll, D. R. (2020). EFG1 Mutations, Phenotypic Switching, and Colonization by Clinical a/α Strains of Candida Albicans. MSphere 5 (1), e00795–19. doi: 10.1128/mSphere.00795-19
Park, H., Myers, C. L., Sheppard, D. C., Phan, Q. T., Sanchez, A. A., Edwards, J. E., et al. (2005). Role of the Fungal Ras-Protein Kinase A Pathway in Governing Epithelial Cell Interactions During Oropharyngeal Candidiasis. Cell. Microbiol. 7 (4), 499–510. doi: 10.1111/j.1462-5822.2004.00476.x
Pierce, J. V., Kumamoto, C. A. (2012). Variation in Candida Albicans EFG1 Expression Enables Host-Dependent Changes in Colonizing Fungal Populations. MBio 3 (4), e00117–12. doi: 10.1128/mBio.00117-12
Pietrella, D., Rachini, A., Pandey, N., Schild, L., Netea, M., Bistoni, F., et al. (2010). The Inflammatory Response Induced by Aspartic Proteases of Candida Albicans Is Independent of Proteolytic Activity. Infect. Immun. 78 (11), 4754–4762. doi: 10.1128/IAI.00789-10
Pujol, C., Srikantha, T., Park, Y.-N., Daniels, K. J., Soll, D. R. (2016). Binding Sites in the EFG1 Promoter for Transcription Factors in a Proposed Regulatory Network: A Functional Analysis in the White and Opaque Phases of Candida Albicans. G3: Genes Genomes Genet. 6 (6), 1725–1737. doi: 10.1534/g3.116.029785
Raithatha, S., Su, T.-C., Lourenco, P., Goto, S., Sadowski, I. (2012). Cdk8 Regulates Stability of the Transcription Factor Phd1 To Control Pseudohyphal Differentiation of Saccharomyces Cerevisiae. Mol. Cell. Biol. 32 (3), 664–674. doi: 10.1128/MCB.05420-11
Rastogi, S. K., van Wijlick, L., Ror, S., Lee, K. K., Román, E., Agarwal, P., et al. (2020). Ifu5, a WW Domain-Containing Protein Interacts With Efg1 to Achieve Coordination of Normoxic and Hypoxic Functions to Influence Pathogenicity Traits in Candida Albicans. Cell. Microbiol. 22 (2), e13140. doi: 10.1111/cmi.13140
Riggle, P. J., Andrutis, K. A., Chen, X., Tzipori, S. R., Kumamoto, C. A. (1999). Invasive Lesions Containing Filamentous Forms Produced by a Candida Albicans Mutant That Is Defective in Filamentous Growth in Culture. Infect. Immun. 67 (7), 3649–3652. doi: 10.1128/IAI.67.7.3649-3652.1999
Rodriguez, D. L., Quail, M. M., Hernday, A. D., Nobile, C. J. (2020). Transcriptional Circuits Regulating Developmental Processes in Candida Albicans. Front. Cell. Infect. Microbiol. 10. doi: 10.3389/fcimb.2020.605711
Saputo, S., Kumar, A., Krysan, D. J. (2014). Efg1 Directly Regulates ACE2 Expression To Mediate Cross Talk Between the cAMP/PKA and RAM Pathways During Candida Albicans Morphogenesis. Eukaryot. Cell 13 (9), 1169–1180. doi: 10.1128/EC.00148-14
Setiadi, E. R., Doedt, T., Cottier, F., Noffz, C., Ernst, J. F. (2006). Transcriptional Response of Candida Albicans to Hypoxia: Linkage of Oxygen Sensing and Efg1p-Regulatory Networks. J. Mol. Biol. 361 (3), 399–411. doi: 10.1016/j.jmb.2006.06.040
Shapiro, R. S., Uppuluri, P., Zaas, A. K., Collins, C., Senn, H., Perfect, J. R., et al. (2009). Hsp90 Orchestrates Temperature-Dependent Candida Albicans Morphogenesis via Ras1-PKA Signaling. Curr. Biol.: CB 19 (8), 621–629. doi: 10.1016/j.cub.2009.03.017
Simonetti, N., Strippoli, V., Cassone, A. (1974). Yeast-Mycelial Conversion Induced by N-Acetyl-D-Glucosamine in Candida Albicans. Nature 250 (464), 344–346. doi: 10.1038/250344A0
Sitterlé, E., Maufrais, C., Sertour, N., Palayret, M., d’Enfert, C., Bougnoux, M.-E. (2019). Within-Host Genomic Diversity of Candida Albicans in Healthy Carriers. Sci. Rep. 9 (1), 2563. doi: 10.1038/s41598-019-38768-4
Solis, N. V., Wakade, R. S., Glazier, V. E., Ollinger, T. L., Wellington, M., Mitchell, A. P., et al. (2022). Systematic Genetic Interaction Analysis Identifies a Transcription Factor Circuit Required for Oropharyngeal Candidiasis. MBio 13 (1), e0344721. doi: 10.1128/mbio.03447-21
Sonneborn, A., Bockmühl, D. P., Ernst, J. F. (1999b). Chlamydospore Formation in Candida Albicans Requires the Efg1p Morphogenetic Regulator. Infect. Immun 67 (10), 5514–5517. doi: 10.1128/IAI.67.10.5514-5517.1999
Sonneborn, A., Bockmühl, D. P., Gerads, M., Kurpanek, K., Sanglard, D., Ernst, J. F. (2000). Protein Kinase A Encoded by TPK2 Regulates Dimorphism of Candida Albicans. Mol. Microbiol. 35 (2), 386–396. doi: 10.1046/j.1365-2958.2000.01705.x
Sonneborn, A., Tebarth, B., Ernst, J. F. (1999a). Control of White-Opaque Phenotypic Switching in Candida Albicans by the Efg1p Morphogenetic Regulator. Infect. Immun. 67 (9), 4655–4660. doi: 10.1128/IAI.67.9.4655-4660.1999
Srikantha, T., Tsai, L. K., Daniels, K., Soll, D. R. (2000). EFG1 Null Mutants of Candida Albicans Switch But Cannot Express the Complete Phenotype of White-Phase Budding Cells. J. Bacteriol. 182 (6), 1580–1915. doi: 10.1128/JB.182.6.1580-1591.2000
Sriram, K., Soliman, S., Fages, F. (2009). Dynamics of the Interlocked Positive Feedback Loops Explaining the Robust Epigenetic Switching in Candida Albicans. J. Theor. Biol. 258 (1), 71–88. doi: 10.1016/j.jtbi.2009.01.008
Stoldt, V. R., Sonneborn, A., Leuker, C. E., Ernst, J. F. (1997). Efg1p, an Essential Regulator of Morphogenesis of the Human Pathogen Candida Albicans, Is a Member of a Conserved Class of BHLH Proteins Regulating Morphogenetic Processes in Fungi. EMBO J. 16 (8), 1982–1991. doi: 10.1093/emboj/16.8.1982
Sudbery, P. E. (2011). Growth of Candida Albicans Hyphae. Nat. Rev. Microbiol. 9 (10), 737–748. doi: 10.1038/nrmicro2636
Taschdjian, C. L., Burchall, J. J., Kozinn, P. J. (1960). Rapid Identification of Candida Albicans by Filamentation on Serum and Serum Substitutes. A.M.A. J. Dis. Children 99 (February), 212–215. doi: 10.1001/archpedi.1960.02070030214011
Tebarth, B., Doedt, T., Krishnamurthy, S., Weide, M., Monterola, F., Dominguez, A., et al. (2003). Adaptation of the Efg1p Morphogenetic Pathway in Candida Albicans by Negative Autoregulation and PKA-Dependent Repression of the EFG1 Gene. J. Mol. Biol. 329 (5), 949–962. doi: 10.1016/S0022-2836(03)00505-9
Tso, G. H. W., Antonio Reales-Calderon, J., Shin Min Tan, A., Sem, X. H., Thi Thu Le, G., Guan Tan, T., et al. (2018). Experimental Evolution of a Fungal Pathogen Into a Gut Symbiont. Science 362 (6414), 589–595. doi: 10.1126/science.aat0537
Tuch, B. B., Mitrovich, Q. M., Homann, O. R., Hernday, A. D., Monighetti, C. K., de la Vega, F. M., et al. (2010). The Transcriptomes of Two Heritable Cell Types Illuminate the Circuit Governing Their Differentiation. PloS Genet. 6 (8), e1001070. doi: 10.1371/journal.pgen.1001070
Uwamahoro, N., Verma-Gaur, J., Shen, H.-H., Qu, Y., Lewis, R., Lu, J., et al. (2014). The Pathogen Candida Albicans Hijacks Pyroptosis for Escape From Macrophages. MBio March 5 (2), e00003–14. doi: 10.1128/mBio.00003-14
Wakade, R. S., Huang, M., Mitchell, A. P., Wellington, M., Krysan, D. J. (2021). Intravital Imaging of Candida Albicans Identifies Differential In Vitro and In Vivo Filamentation Phenotypes for Transcription Factor Deletion Mutants. MSphere 6 (3), e00436–e00421. doi: 10.1128/mSphere.00436-21
Wang, A., Pala Raniga, P., Lane, S., Lu, Y., Liu, H. (2009). Hyphal Chain Formation in Candida Albicans: Cdc28-Hgc1 Phosphorylation of Efg1 Represses Cell Separation Genes. Mol. Cell. Biol 29 (16), 4406–4416. doi: 10.1128/MCB.01502-08
Wang, H., Song, W., Huang, G., Zhou, Z., Ding, Y., Chen, J. (2011). Candida Albicans Zcf37, a Zinc Finger Protein, Is Required for Stabilization of the White State. FEBS Lett. 585 (5), 797–802. doi: 10.1016/j.febslet.2011.02.005
Wellington, M., Koselny, K., Krysan, D. J. (2012). Candida Albicans Morphogenesis Is Not Required for Macrophage Interleukin 1β Production. MBio 4 (1), e00433-12. doi: 10.1128/mBio.00433-12
Willger, S. D., Liu, Z., Olarte, R. A., Adamo, M. E., Stajich, J. E., Myers, L. C., et al. (2015). Analysis of the Candida Albicans Phosphoproteome. Eukaryot. Cell 14 (5), 474–485 doi: 10.1128/EC.00011-15
Witchley, J. N., Basso, P., Brimacombe, C. A., Abon, N. V., Noble, S. M. (2021). Recording of DNA-Binding Events Reveals the Importance of a Repurposed Candida Albicans Regulatory Network for Gut Commensalism. Cell Host Microbe 29 (6), 1002–1013.e9. doi: 10.1016/j.chom.2021.03.019
Witchley, J. N., Penumetcha, P., Abon, N. V., Woolford, C. A., Mitchell, A. P., Noble, S. M. (2019). Candida Albicans Morphogenesis Programs Control the Balance Between Gut Commensalism and Invasive Infection. Cell Host Microbe 25 (3), 432–443.e6. doi: 10.1016/j.chom.2019.02.008
Xie, J., Tao, L., Nobile, C. J., Tong, Y., Guan, G., Sun, Y., et al. (2013). White-Opaque Switching in Natural MTLa/α Isolates of Candida Albicans: Evolutionary Implications for Roles in Host Adaptation, Pathogenesis, and Sex. PloS Biol. 11 (3), e1001525. doi: 10.1371/journal.pbio.1001525
Xu, X.-L., Teck Ho Lee, R., Fang, H.-M., Wang, Y.-M., Li, R., Zou, H., et al. (2008). Bacterial Peptidoglycan Triggers Candida Albicans Hyphal Growth by Directly Activating the Adenylyl Cyclase Cyr1p. Cell Host Microbe 4 (1), 28–39. doi: 10.1016//j.chom.2008.05.014
Zavrel, M., Majer, O., Kuchler, K., Rupp, S. (2012). Transcription Factor Efg1 Shows a Haploinsufficiency Phenotype in Modulating the Cell Wall Architecture and Immunogenicity of Candida Albicans. Eukaryot. Cell 11 (2), 129–140. doi: 10.1128/EC.05206-11
Zhao, X., Daniels, K. J., Oh, S.-H., Green, C. B., Yeater, K. M., Soll, D. R., et al. (2006). Candida Albicans Als3p Is Required for Wild-Type Biofilm Formation on Silicone Elastomer Surfaces. Microbiol. (Read. England) 152 (Pt 8), 2287–2299. doi: 10.1099/mic.0.28959-0
Zhao, Y., Su, H., Zhou, J., Feng, H., Zhang, K.-Q., Yang, J. (2015). The APSES Family Proteins in Fungi: Characterizations, Evolution and Functions. Fungal Genet. Biol.: FG B 81 (August), 271–280. doi: 10.1016/j.fgb.2014.12.003
Keywords: EFG1, Candida albicans, transcription factor regulatory network, biofilm, virulence, transcription factor, fungal pathogen
Citation: Glazier VE (2022) EFG1, Everyone’s Favorite Gene in Candida albicans: A Comprehensive Literature Review. Front. Cell. Infect. Microbiol. 12:855229. doi: 10.3389/fcimb.2022.855229
Received: 14 January 2022; Accepted: 24 February 2022;
Published: 22 March 2022.
Edited by:
Clarissa J. Nobile, University of California, Merced, United StatesReviewed by:
Richard J. Bennett, Brown University, United StatesMatthew Lohse, University of California, San Francisco, United States
Copyright © 2022 Glazier. This is an open-access article distributed under the terms of the Creative Commons Attribution License (CC BY). The use, distribution or reproduction in other forums is permitted, provided the original author(s) and the copyright owner(s) are credited and that the original publication in this journal is cited, in accordance with accepted academic practice. No use, distribution or reproduction is permitted which does not comply with these terms.
*Correspondence: Virginia E. Glazier, dmdsYXppZXJAbmlhZ2FyYS5lZHU=