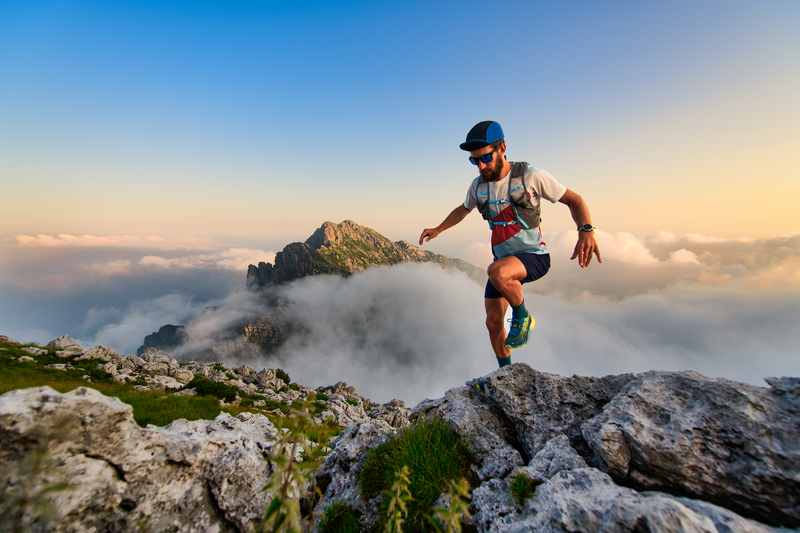
94% of researchers rate our articles as excellent or good
Learn more about the work of our research integrity team to safeguard the quality of each article we publish.
Find out more
REVIEW article
Front. Cell. Infect. Microbiol. , 09 March 2022
Sec. Microbiome in Health and Disease
Volume 12 - 2022 | https://doi.org/10.3389/fcimb.2022.854879
This article is part of the Research Topic Interaction between Traditional Chinese Medicine and Gut Microbiota View all 43 articles
Non-alcoholic fatty liver disease (NAFLD) remains a common disease with a significant health and economic burden worldwide. The gut microbiota (GM) and bile acids (BAs), which play important roles in the gut-liver axis, have been confirmed to jointly participate in the development of NAFLD. GM not only regulate bile acids’ synthesis, transport, and reabsorption by regulating other metabolites (such as trimetlyl amine oxide, butyrate), but also regulate dehydrogenation, dehydroxylation and desulfurization of bile acids. Meanwhile, disordered bile acids influence the gut microbiota mainly through promoting the bacterial death and lowering the microbial diversity. Although weight loss and lifestyle changes are effective in the treatment of NAFLD, the acceptability and compliance of patients are poor. Recently, increasing natural plants and their active ingredients have been proved to alleviate NAFLD by modulating the joint action of gut microbiota and bile acids, and considered to be promising potential candidates. In this review, we discuss the efficacy of natural plants in treating NAFLD in the context of their regulation of the complex interplay between the gut microbiota and bile acids, the crosstalk of which has been shown to significantly promote the progression of NAFLD. Herein, we summarize the prior work on this topic and further suggest future research directions in the field.
Non-alcoholic fatty liver disease (NAFLD), characterized by excessive lipid deposition in hepatocytes, has become the most common chronic liver disease worldwide. Clinically, the spectrum of histopathology ranges from simple steatosis (NAFL) and non-alcoholic steatohepatitis (NASH) to liver cirrhosis (Friedman et al., 2018b; Powell et al., 2021). Currently, the global incidence of NAFLD has greatly increased to an estimated prevalence of 25% among adults (NCD Risk Factor Collaboration, 2017; Hales et al., 2018; Zhou et al., 2019a). Until now, the best characterization of the mechanisms underlying NAFLD is provided in the “two hit” theory proposed by Day in 1998; this theory suggests that on the basis of hepatic lipid accumulation caused by developed insulin resistance, the “two hit” from other factors is required to trigger the pathological procession of NAFLD (Day and James, 1998; Friedman et al., 2018b). However, the exact mechanism underlying metabolic disorders in NAFLD remains unclear. The “gut-liver axis,” first proposed by Marshall in 1998, is essential in the regulation of systemic metabolism, gut hormone release, and the immune response (Marshall, 1998). The gut microbiota (GM) and bile acids (BAs), which play important roles in the gut-liver axis, have been confirmed to jointly participate in the development of NAFLD (Chavez-Talavera et al., 2017; Kolodziejczyk et al., 2019; Aron-Wisnewsky et al., 2020).
The GM is defined as a complex and dynamic microbial ecosystem in the gut, composed of symbiotic bacteria, archaea, fungi, and viruses, (Zmora et al., 2019). Several studies have shown that the GM and its metabolites could be used as key signaling factors to regulate the host’s glucose and lipid metabolism, insulin resistance, immunity, and inflammation in NAFLD (Aron-Wisnewsky et al., 2020). BAs, a metabolite of GM, are a class of amphiphilic molecules synthesized from cholesterol (Li and Chiang, 2014). Significant evidence has suggested that BAs could participate in NAFLD by regulating glucose and lipid metabolism and energy homeostasis (Arab et al., 2017). Meanwhile, the crosstalk between the GM and BAs can significantly promote the progression of NAFLD (Jia et al., 2018; Winston and Theriot, 2020; Agus et al., 2021). Thus, the interplay between GM and BAs may be a promising target for the prevention and treatment of NAFLD.
Natural plants, including plants and active ingredients, have been proven as effective treatments of NAFLD (Liu et al., 2017; Leng et al., 2020; Xin et al., 2021). The therapeutic effects of natural plants have attracted increasing attention. Furthermore, the potential regulatory effects of natural plants on intestinal dysbacteriosis and BA metabolism have been reported (Meng et al., 2018; Leng et al., 2020). Thus, this review aimed to discuss the efficacy of natural plants, which act by regulating the interplay between GM and BAs, against NAFLD.
The human GM is rich in various species, including those of Firmicutes, Bacteroides, Proteobacteria, and Actinomycetes in the gut (Eckburg et al., 2005). Early evidence linking gut dysbiosis and NAFLD has shown that the abundance of gram-negative bacteria increases, whereas that of gram-positive bacteria decreases during the course of NAFLD, indicating that microbial populations are altered in NAFLD patients (Ley et al., 2006). A large number of metabolites, including BAs, lipopolysaccharides, short-chain fatty acids (SCFAs), and inflammatory factors, are produced following host–microorganism interactions. The content of BAs, especially secondary BAs, increases significantly in the serum of hosts with NAFLD (Jiao et al., 2018). Meanwhile, the interplay between GM and BAs has been shown to maintain host homeostasis in NAFLD (Jiao et al., 2021). When colonizing germ-free mice with the feces of wild type or humans, the total BA content was obviously reduced (Sayin et al., 2013).
The GM regulates the synthesis, transport, and reabsorption of BAs via regulation of metabolites and the farnesoid X receptor (FXR). Moreover, the GM can also modify primary BAs to secondary BAs with enzymes such as bile salt hydrolase (BSH) through dehydrogenation, dehydroxylation, and desulfurization. All of this research has been clarified and discussed below, with the mechanisms represented in Figure 1.
Figure 1 Microbial regulation of Bile Acids in NAFLD. ①: Microbial modification of primary BAs to secondary BAs. ②: Microbial regulation of BAs synthesis. ③: Microbial regulation of BAs transport, and reabsorption. (BESP, bile salt export pump; MRP2, multidrug resistance protien 2; BAs, bile acids; BSH, bile salt hydrolase; β-MCA, β-murocholic acid; CA, Cholic acid; CDCA, Chenodeoxycholic acid; CYP7A1, cytochrome P450 family 27 subfamily A member 1; CYP7B1, cytochrome P450 family 7 subfamily B member 1; DCA, deoxycholic acid; FXR, farnesoid X receptor; FFA, free fatty acid; IBABP, ileal bile acid binding protein; LCA, lithocholic acid; NAFLD, non-alcoholic fatty liver disease; SHP, small heterodimer partner; SREBP-1, sterol regulatory element binding protein-1; TβMCA, tauro-β-muricholic acid; TC, total cholesterol; TMAO, trimethylamine oxide; UDCA, ursodeoxycholic).
The synthesis of BAs is complex, including reaction steps catalyzed by more than a dozen enzymes. BAs are synthesized in pericentral hepatocytes and can be fulfilled via two different pathways. Specifically, the classical pathway, which accounts for 75% of BA synthesis, is initiated by the 7a-hydroxylation of cholesterol catalyzed by cytochrome P450 family 27 subfamily A member 1 (CYP7A1). In a case-control study, trimethylamine oxide (TMAO), the metabolites from GM, significantly increased BA synthesis by upregulating hepatic CYP7A1 mRNA levels in patients with NAFLD (Tan et al., 2019). Correspondingly, the alternative pathway is initiated by the 27-hydroxycholesterol of cholesterol catalyzed by CYP27A1 and further hydroxylated by cytochrome P450 family 7 subfamily B member 1 (CYP7B1) in the liver. This pathway accounts for approximately 9–25% of BA synthesis. After antibiotic treatment, hepatic CYP7B1 was upregulated in high-fat diet (HFD)-fed hamsters, contributing to a more hydrophilic BA profile with increased tauro-β-muricholic acid (TβMCA) (Sun et al., 2019a). Therefore, the GM regulates the expression of several enzymes in the BA synthesis pathway to promote NAFLD. Furthermore, the alternative pathway predominantly generates Chenodeoxycholic acid (CDCA), whereas the classical pathway generates both CDCA and Cholic acid (CA), which are called primary BAs (Chiang, 2013). Rodents also produce α-murocholic acid (α-MCA) and β-murocholic acid (β-MCA) as primary BAs. Sheng confirmed that butyrate, the metabolite of GM, significantly decreased the content of β-MCA by activating hepatic FXR-small heterodimer partner (SHP) signaling in HFD-fed mice (Sheng et al., 2017). However, the regulation of β-MCA by GM may be attributed to unknown enzymes.
Independent of the synthetic route, the carboxylic acid group from CA/CDCA conjugated the peroxisomes of glycine or taurine to form conjugated BAs (G/TCA and G/TCDCA, respectively) in humans (Jia et al., 2018). This conjugation with taurine alone occurred to form TCA/TCDCA in rodents. Subsequently, conjugated BAs are actively transported into bile via the bile salt export pump (BSEP) and may be stored in the gall-bladder until being released into the duodenum after ingestion of a meal. Approximately 95% of BAs are reabsorbed from the intestine, predominantly as conjugated BAs in the distal ileum, by the apical sodium-dependent bile acid transporter (ASBT), and recirculate via the portal vein to the liver, from where they are secreted again (Ticho et al., 2019). Treatment with FXR-stimulatory Bacteroides dorei-derived metabolites could strongly induce ileal bile acid binding protein (IBABP) and organic solute transporter (OST) α, thereby improving the obesity phenotype, including body weight gain, liver damage, and lipid metabolism in HFD-fed mice (Zhang et al., 2015). Overall, GM can regulate the synthesis, transport, and reabsorption of BAs via its metabolites or FXR in the progression of NAFLD.
In addition, GM participates in the biotransformation of BAs via the catalysis of microbial enzymes, thereby changing the composition of BA pools in NAFLD. Hydrolysis mediated by BSH transforms conjugated BAs into unconjugated BAs. BSH, an intracellular enzyme encoded by the bsh gene (Song et al., 2019), has been extensively identified in gastrointestinal microorganisms, including Lactobacillus, Bifidobacterium, Enterococcus, Clostridium, and Bacteroides (Ridlon et al., 2006). Different intestinal bacteria are associated with different BSH activities. The levels of taurine and glycine-metabolizing bacteria that express BSH enzymes were enhanced in the gut of patients with NAFLD, significantly increasing the production of secondary BAs (Jiao et al., 2018).
Next, unconjugated BAs were further transformed into deoxycholic acid (DCA) and lithocholic acid (LCA) via 7α-dehydroxylation or ursodeoxycholic (UDCA) by 7-alpha-hydroxysteroid dehydrogenase (HSDH). Sydro et al. indicated a clear association between the 7α-dehydrogenase related to Bacteroides and Lactobacillus, and the primary conjugated BA composition in patients with NASH (Sydor et al., 2020). Recently, Yu et al. analyzed the associations between GM and the concentrations of primary and secondary BAs in fecal samples of children with NAFLD. Further, a reduction in Eubacterium and Ruminococcaceae bacteria, which express BSH and 7α-dehydroxylation, was significantly positively correlated with the level of fecal LCA (Yu et al., 2021).
Furthermore, the main conclusions of the previous studies are consistent with those observed in rodents. In HFD-fed mice, higher abundance levels of the genus Lactobacillus and increased BSH activity resulted in decreased levels of TβMCA, a substrate of BSH, and a potent FXR antagonist (Gonzalez et al., 2016). Another study also confirmed that an 8 week HFD significantly increased abundance levels of Extibactermuris, related to 7α-dehydroxylation, metabolizing CA to DCA in mice (Streidl et al., 2021). Similarly, Bacteroides expressing HSDH and 7-alpha HSDH were enriched to further modify unconjugated BAs into UDCA in the gut microbiome of HFD-fed rats (Jiao et al., 2018).
Despite this, the interplay between the GM and BAs is not a one-way street. BAs can further influence the structure and function of the GM. After administration of obeticholic acid (OCA), the levels of gram-positive bacteria increased in humans, whereas that of Firmicutes bacteria increased in mice (Friedman et al., 2018a). Sevelamer, a BA sequestrant, reversed this increase in Lactobacillus and decreased the levels of Desulfovibrio in HFD-fed mice (Takahashi et al., 2020). Other promising studies have shown that BAs exert direct and indirect effects on the GM (Figure 2).
Figure 2 BAs influence GM in NAFLD. (BAs, bile acids; CA, Cholic acid; CDCA, Chenodeoxycholic acid; C3GnT, Core 3 O-glycan; DCA, deoxycholic acid; FXR, farnesoid X receptor; Muc2, mucin 2; NAFLD, non-alcoholic fatty liver disease; G-β-MCA, glycine-β-muricholic acid; TUDCA, tauro-ursodeoxycholic).
The direct action of BAs on GM has been shown to promote cell death via membrane regulation. In the intestinal lumen, BAs exert direct antimicrobial activity based on their detergent properties to shape the GM (Schubert et al., 2017). Free bile acids (FBAs), including CA, DCA, and CDCA, dissipated the transmembrane electrical potential (DeltaPsi). In the human intestine, the populations of Lactobacillus and Bifidobacterium are controlled in part by the accumulation of CA. As this accumulation must occur during bacterial growth in the intestine, growth inhibition may be associated with the accumulation of FBAs. Taking these observations together, although FBAs disturb the membrane integrity, leading to leakage of proton and other cellular components, they also reduced the internal pH levels of bacteria with rapid and stepwise kinetics and, at certain concentrations, dissipated DeltapH. High concentrations of BAs can decrease the internal pH of Lactobacilli and Bifidobacteria, and reduce the production of ATP, ultimately inducing bacterial death (Kurdi et al., 2006).
In addition to exerting strong direct effect, the indirect effects by which BAs regulate GM through signaling factors also have significant consequences. Basic experiments have confirmed the role of FXR in regulating the structure of intestinal bacteria (Inagaki et al., 2006). Zhang et al. confirmed that oral G-β-MCA administration altered the gut microbial community structure, notably reducing the ratio of Firmicutes to Bacteroidetes in NASH mice. This revealed that metabolic improvement was dependent on intestinal FXR (Zhang et al., 2016). In addition, TUDCA has been shown to increase the Bacteroidetes/Firmicutes ratio and the abundance of Faecalibacterium and Akkermansia in mice with NAFLD. The growth of bacterial genera might be related to the upregulation of mucin 2 (Muc2) and Core 3 O-glycan (C3GnT), which provide a substrate for the growth of intestinal bacteria (Wang et al., 2018).
Although weight loss and lifestyle changes are effective in the treatment of NAFLD (Vilar-Gomez et al., 2015; Khoo et al., 2017), the acceptability and compliance of patients are poor. Currently, the use of synthetic drugs for the treatment of NAFLD remains limited. Despite the numerous options for the tested pharmaceuticals, none of them are satisfactory in treating NAFLD. However, increasing evidence has indicated that natural plants efficiently exhibit anti-NAFLD properties, with lower toxicity and side effects, and may be useful in regulating the joint action of GM and BAs (Liu et al., 2017). Therefore, we summarized the new studies on Natural plants that have indicated that they exert anti-NAFLD properties by modulating the interplay between GM and BAs. All these are clarified and discussed below, with the mechanisms presented in Tables 1, 2.
Table 2 Active ingredients in natural plants regulate the interplay between GM and BAs in the treatment of NAFLD.
Tea, processed from the leaves of Camellia sinensis, is one of the most widely consumed beverages worldwide. This drink is characterized by high levels of an antioxidant that has been shown to prevent different types of metabolic syndromes, cardiovascular diseases, obesity, and type 2 diabetes (Bag et al., 2022). Huang et al. indicated that consumption of Pu-erh tea significantly increased the content of fecal conjugated BAs by decreasing the BSH activity, which was linked to a reduction in the levels of Lactobacillus, Bacillus, Streptococcus, and Lactococcus, resulting in the inhibition of intestinal FXR-FGF15 activation. The excretion of BAs was enhanced in the feces as the expression of CYP27A1 and CYP7B1 increased in the liver, thereby reducing hepatic lipid deposition in HFD-fed mice (Huang et al., 2019).
Huangjinya black tea is a natural photosensitive tea containing abundant free amino acids. This drink has been proven to ameliorate hepatic steatosis by reducing hepatic triglyceride (TG), free fatty acid (FFA), and lipid accumulation in HFD-fed mice. Furthermore, Huangjinya black tea consumption could also significantly reverse GM disruption and increase the ratio of primary/secondary BAs (Xu et al., 2020).
Whole grains contain the endosperm, germ, and bran of the original seeds, in contrast to refined grains, in which the germ and bran are removed during the milling process (Aune et al., 2016). The whole grain, rich in dietary fiber, is considered to have a beneficial effect on the risk reduction of NAFLD (Berna and Romero-Gomez, 2020). Sun et al. confirmed that oats and tartary buckwheat-based food (OF) reduced the hepatic total cholesterol (TC) and TG levels in HFD-fed hamsters. Furthermore, it can change the overall structure of the gut microbiota. Specifically, the relative abundance of Erysipelotrichaceae, Ruminococcaceae, and Lachnospiraceae decreased, whereas that of Eubacteriaceae increased. Meanwhile, the excretion of fecal BAs increased and the concentration of SCFAs (acetic acid, propionic acid, butyric acid, and total short-chain fatty acids) increased significantly after OF intervention (Sun et al., 2019b).
Another study revealed that wheat gluten consumption resulted in higher fecal BA excretion, thereby reducing the hepatic TC and TG levels in HCD-diet hamsters. Moreover, the enhanced fecal BAs may be related to an increase in hepatic CYP7A1 levels. Furthermore, it has been shown that the relative abundance of Firmicutes and Erysipelas decreased, whereas that of Bacteroides, Bacteroides, and Rumenococcus increased (Liang et al., 2019).
Legumes are defined as the pods or fruits of plants belonging to the botanical families Leguminosae or Favaceae, which includes soybeans, peanuts, green/dry beans, and lentils (McCrory et al., 2010). These seeds are a good source of protein, fiber, B vitamins, minerals, and polyphenols, and are considered as low-glycemic-index foods (Rebello et al., 2014). Populations with high legume consumption (peas, beans, lentils) have a low risk of cancer and chronic degenerative diseases (Campos-Vega et al., 2013).
Micioni et al. found that lentils significantly reduced serum TC and LDL levels in HCD-fed rats. Furthermore, the contents of fecal BAs were enhanced, resulting in an increased excretion of BA in feces. In addition, the abundance of Bifidobacteria and the level of butyric acid markedly increased after intervention with lentils (Micioni Di Bonaventura et al., 2017). Inagaki et al. demonstrated the effect of pea pods on decreasing serum TC and TG levels in HS-fed SD rats. Meanwhile, the upregulation of fecal BAs and the abundance of bifidobacteria were observed significantly (Inagaki et al., 2016).
Plant oil, rich in dietary omega-3 long-chain polyunsaturated fatty acids (LCPUFA), comprises important biomolecules that have been shown to regulate hepatic lipid metabolism (Manson et al., 2019; Maattanen et al., 2020). For example, wild melon seed oil (CO) supplementation reduced the body weight, liver weight, and hepatic TC and enhanced the excretion of fecal BAs, upregulating the gene expression of hepatic CYP7A1 in HCD-fed hamsters. Meanwhile, CO intervention favorably altered the relative abundances of Eubacteriaceae, Clostridiales_vadinBB60_group, Ruminococcaceae, Streptococcaceae, and Desulfovibrionaceae at the family level (Hao et al., 2020).
In another study, oral consumption of sacha inchi oil alleviated hepatic fat accumulation in HFD-fed rats. Furthermore, the imbalance of the GM was reversed, embodied by decreasing abundances of Enterobacteriaceae and Escherichia and increasing ratios of Roseburia, Turicibacter, and Butyrivibrio. Meanwhile, the content of CA, GCA, TCDCA, and TCA significantly decreased after sacha inchi intervention (Li et al., 2020).
Grapes are one of the most highly consumed fruits worldwide. In addition to being a rich source of vitamins and fiber, the skin and seeds of grapes contain abundant polyphenols, specifically proanthocyanidins. The proanthocyanidin-rich grape seed extract is beneficial against many diseases, including inflammation, cardiovascular disease, hypertension, diabetes, cancer, peptic ulcers, and microbial infections.
Han et al. indicated that grape extract (GE) decreased liver weight, accompanied by a decline in adipocyte size and hepatic fatty deposits in HFD-fed mice. Additionally, GE restores dysbiosis of the GM by augmenting the observed species, enhancing the Firmicutes-to-Bacteroidetes ratio, and increasing the abundance of the Bifidobacterium, Akkermansia, and Clostridia genera. The restoration of Akkermansia, Clostridium, and Bifidobacterium was negatively correlated with the concentrations of TαMCA, TβMCA, and TCA, but positively correlated with DCA (Han et al., 2020).
Scutellaria baicalensis Georgi. (Lamiaceae) is a plant of the genus Lamiaceae, whose root is commonly used as a medicine. This medicinal plant is widely distributed in China, Russia, Mongolia, North Korea, and Japan. To date, over 40 compounds have been isolated and identified from Scutellaria baicalensis, including flavonoids, terpenoids, volatile oils, and polysaccharides. The compounds and extracts exhibit a wide range of pharmacological activities, including effects on the immune system, liver protection, antioxidant effects, and other pharmacological effects (Zhao et al., 2019).
Zhao et al. found that Radix scutellariae water extract (WESB) improved hepatic steatosis, inflammation, and ballooning of hepatocytes in HFD-fed rats. Of note, the abundance of Lactobacillus, which exhibits significant negative correlations with certain fecal-conjugated BAs (TCDCA, GUDCA, and TUDCA), was significantly decreased in the feces of HFD-fed rats after treatment with WESB. Moreover, this effect might be related to the activation of hepatic CYP7A1 and the inhibition of FXR expression in the intestine rather than in the liver (Zhao et al., 2021).
Gl, commonly referred to as “Lingzhi” in Chins, is one of the best-known medicinal mushrooms, and has been used in herbal medicines worldwide for more than two thousand years. In recent decades, Gl-related biological and pharmacological research has focused on the bioactive compounds extracted from its fruiting bodies, including polysaccharides, triterpenoids, sterols, proteins, and peptides, which comprise constituents with numerous biological activities such as antioxidant, anti-inflammatory, immunomodulatory, hypoglycemic, and hypolipidemic activities (Lu et al., 2020).
Several studies have shown that the consumption of low and high doses of Gl extracts almost entirely prevented the accumulation of lipids in the liver of high-cholesterol diet-fed mice. Analyses of hepatic cholesterol and triglyceride levels confirmed this histological analysis. These effects were associated with a significant increase in the excretion of fecal bile acids and an increase in CYP7A1 gene expression in the liver. Furthermore, the relative abundance of Lactobacillus significantly increased after intervention with Gl (Meneses et al., 2016).
Sandra et al. showed that Mexican Gl extracts prevent hepatic fatty acid synthesis and accumulation through the downregulation of genes involved in lipogenesis. Furthermore, the Gl extract activated one of the major BA synthesis pathways via CYP7B1-mediated hydroxylation of cholesterol. In addition to gene modulation, the administration of Gl extracts also modulates the composition of the gut microbiota, triggering an increase in abundance of the Lactobacillaceae family and the genus Lactobacillus (Romero-Cordoba et al., 2020).
Grifola frondosa (Dicks.) Gray is a widely consumed edible and medicinal fungus. Ancient books record that it can boost qi and fortify the spleen, moisten the lungs, and protect the liver. Over the past three decades, GF polysaccharides have been shown to possess various promising bioactivities, including antitumor, immunomodulation, anti-oxidation, and anti-hyperglycemia, and can also effectively act on the skin and hematopoietic stem cells (He et al., 2017).
Pan et al. found that the levels of serum ALT/AST and hepatic lipid accumulation were significantly decreased in GF-treated HFD-fed rats. Furthermore, GF consumption significantly enhanced the excretion of BAs in the cecum. In addition, a higher abundance of Butyricimonas was observed in the GF group (Pan et al., 2018).
Previously, we reviewed the efficacies and modes of action of plants regulating the interplay between GM and BAs in NAFLD. It is important to note that many of the active ingredients of these plants can exert the same medicinal effects as the source plant itself. Therefore, from the perspective of the chemical structure, we divided these active ingredients into polysaccharides, oligosaccharides, polyphenols, ketones, and other active ingredients.
Polysaccharides, which are natural macromolecules composed of monosaccharides, are among the most important members of the biopolymer family. To date, more than 300 natural polysaccharide compounds have been identified and are ubiquitously present in plants, animals, and microorganisms, where they engage in a variety of physiological functions. Several pharmacological and clinical studies have shown that plant polysaccharides have multiple functions, such as immune regulation, anti-inflammatory, antiviral, and hypoglycemic (Kouakou et al., 2013; Chen and Huang, 2018).
Guar gum, a naturally occurring polymer, is a galactomannan obtained from the ground endosperm of Cyamopsis tetragonolobus or Cyamopsis psoraloides. Pectin is a heteropolysaccharide abundant in the cell wall of plants and is obtained mainly from fruits (citrus and apple). In HFD-fed rats, guar gum and pectin significantly decreased hepatic TG and TC levels. Furthermore, guar gum increased the cecal amounts of CA, CDCA, and UDCA, as well as α-, β-, and ω-MCA to a greater extent, whereas that of DCA and HDCA were reduced. In contrast, differences in BA composition between pectin groups were less obvious, but the cecal levels of α- and ω-MCA were higher in rats. Meanwhile, these two fibers decreased the cecal abundance of Oscillospira and an unclassified genus in Ruminococcaceae, while increasing that of an unclassified family in RF32 (Ghaffarzadegan et al., 2016).
G. frondosa polysaccharides (GFP), derived from the plant Grifola frondosa, exerts various biological activities, such as antitumor, hypoglycemic, antioxidant, and immune regulatory activities. Li et al. found that GFP markedly alleviated hepatic lipid accumulation and steatosis in HFD-fed rats. In addition, the excretion of fecal BAs was also promoted by significantly increasing the mRNA expression of CYP7A1 and BSEP after oral administration of GFP. Meanwhile, GFP supplementation significantly increased the proportion of Helicobater, Intestinimonas, Barnesiella, Defluviitalea, Ruminococcus, Flavonifractor, and Paraprevotella, but decreased the relative abundances of Clostridium-XVIII, Butyricicoccus, and Turicibacter (Li et al., 2019).
The microalgae Chlorella pyrenoidosa is considered a valuable and nutritious microalga, which contains a variety of polysaccharides, carotene, chlorophylls, and polyunsaturated fatty acids in abundance. The pharmacological effects of Chlorella phytochemicals include anti-inflammatory, antitumor, and antioxidant activities. Moreover, CPP is regarded as an effective hypolipidemic agent. Wan et al. demonstrated that CPP could effectively inhibit hepatic lipid accumulation in HFD-fed rats. In addition, CPP accelerates the metabolism of total cecal BAs, while increasing the relative abundance of lactobacilli in the intestines (Wan et al., 2020).
Oligosaccharides are saccharide polymers containing a small number of monosaccharides, which are obtained by direct extraction from natural sources, hydrolysis of polysaccharides, or enzymatic and chemical synthesis from disaccharides; they play an important role in preventing obesity and other metabolic diseases (McCranie and Bachmann, 2014).
Hu et al. showed that administration of citrus pectin oligosaccharides (POS) markedly reduced liver weight and hepatic TC in HF-fed mice. In addition, the relative abundances of specific bacterial groups in the feces and the concentrations of their metabolites were higher after POS intervention. Furthermore, we observed significant correlations among Bifidobacterium, Lactobacillus, and Bacteroides and fecal BAs, as well as hepatic CYP7A1 reductase (Hu et al., 2019).
Polyphenols are a large and heterogeneous group of phytochemicals, including multiple sub-classes such as flavonoids, stilbenes, phenolic acids, and lignans (Manach et al., 2005), which have shown promise in the management of many diseases due to their antioxidant, anti-inflammatory, anti-fibrotic, and metabolic regulation functions. Several hundred different polyphenols are found in plant-based foods, including tea, coffee, legumes, cereals, plant-derived beverages, and chocolate (Khan et al., 2008).
Huang et al. indicated that HFD-fed mice receiving the polyphenol theabrownin exhibited significant decreases in hepatic TG and TC levels. Additionally, the abundance of BSH-producing Lactobacillus, Bacillus, Streptococcus, and Lactococcus genera decreased, as did the BSH activity in mice treated with theabrownin. Conjugated BAs inhibit intestinal FGF15/FGF19-FGFR4 signaling, coupled with increased activation of hepatic FXR-SHP signaling in the liver, thereby increasing CYP7B1 levels to promote BA synthesis (Huang et al., 2019).
Xie et al. revealed that Kudingcha dicaffeoylquinic acid (diCQA), made from Kudingcha, decreased the hepatic and adipose tissue masses in HFD-fed mice. It can also induce functional differences in microbial communities consisting of several metabolic pathways, including primary bile acid biosynthesis and secondary bile acid biosynthesis. Furthermore, the relative abundances of Bifidobacterium and Akkermansia were shown to increase after treatment with diCQAs (Xie et al., 2019).
Naito et al. confirmed that EGCG significantly inhibited the HFD-induced increase in histological fatty deposits and TG accumulation in the liver, and improved intestinal dysbiosis. Meanwhile, inhibition of 7a-dehydroxylation, associated with abundance of the Akkermansia and Parabacteroides genera, was shown to decrease the conversion of CA to TCA in the intestine (Naito et al., 2020).
He et al. found that after pure citrus total flavonoid (PTFC) intervention, the degree of fatty changes, infiltration of inflammatory cells, and ballooning of hepatocytes in the liver of HFD-fed mice were significantly reduced. Furthermore, while the relative abundances of Bacteroides and Artemisiaceae increased, the content of toxic BAs (TDCA, DCA, TCA, CA) and the ratio of secondary BAs/primary Bas were enhanced. This revealed that PTFC could alleviate NAFLD by co-regulating GM and BA metabolism (He et al., 2021).
Polyketides consist of a large group of natural biomolecules that are normally produced by bacteria, fungi, and plants. These molecules are clinically important because of their anti-cancer, anti-microbial, anti-oxidant, and anti-inflammatory properties (Xu et al., 2021). Monascus pigments (MPs) from red yeast rice significantly ameliorate lipid metabolism disorders by inhibiting hepatic lipid accumulation and steatosis in HFD-fed rats. Furthermore, the excretion of fecal BAs was also promoted by oral administration of MPs. Meanwhile, some beneficial GM (such as Oscillibacter sp., Ruminococcus albus, and Clostridium sp.) were found to be negatively correlated with serum and hepatic lipid indicators (Zhou et al., 2019b).
Alkaloids, as a class of natural ingredients derived from traditional Chinese medicines, have previously been shown to inhibit proliferation, metastasis, and angiogenesis; change cell morphology; promote apoptosis and autophagy; and trigger cell cycle arrest (Liu et al., 2019).
Rhizoma coptidis (RC) alkaloids reduced body weight gain, serum TG, and hepatic lipid accumulation in HFHC-fed mice. Furthermore, RC alkaloid feeding significantly increased the abundance of Sporobacter termitidis, Alcaligenes faecalis, and Akkermansia muciniphila in the gut of mice, whereas that of Escherichia coli, Desulfovibrio C21_c20, and Parabacteroides distasonis was suppressed. The synthesis of bile acids was increased by the upregulation of CYP7A1 (He et al., 2016).
Triterpenoids (TTP) are widely distributed in higher plants and are of interest because of their structural diversity and broad range of bioactivities. TTP possesses antioxidant, metabolic-regulating, immunomodulatory, and anti-inflammatory activities, suggesting its application as an alternative therapy in some chronic diseases (Ren and Kinghorn, 2019).
2α-OH-protopanaxandiol (GP2), a metabolite of gypenosides in vivo, has been shown to protect mice from HFD-induced obesity and improve glucose tolerance. Moreover, GP2 treatment inhibited the activity of BSH and decreased the abundance of species of the genera Brucella and Desulfovibrio from Proteobacteria and the genera norank_f_Bacteroidales_S24-7_group from Bacteroidetes, thereby increasing the content of TβMCA in the intestine. TβMCA induced GLP-1 production and secretion by reducing the transcriptional activity of nuclear FXR, thereby ameliorating metabolic syndrome (Xie et al., 2020).
In this review, we discussed and summarized the new findings regarding the effects of natural plants on the regulation of interplay between GM and BA to improve NAFLD. We systemically discussed the joint action between the GM and BA in the pathogenesis of NAFLD. These components show a strong interrelationship. The GM regulates BA synthesis, transport, and reabsorption via expression of metabolites and FXR, while also promoting the conversion of primary BAs to secondary BAs via enzyme activity. Conversely, BAs can directly inhibit the growth of intestinal flora by acting on bacterial membranes. In addition, it can indirectly affect the structure and growth of intestinal bacteria. In addition, we comprehensively reviewed the management of joint action between GM and BAs in NAFLD, in terms of the evidence regarding Natural Plants. These Natural Plants could act as reference for further drug research and discovery to treat NAFLD.
In recent years, a number of studies have shown the potential of food and herb plants as treatments for diet-induced NAFLD. We summarized that the main ingredients in plant foods, which worked effectively, might be attributed to dietary fiber, plant protein, and LCPUFA. Meanwhile, frequent changes in the GM mainly include alterations in the abundance of Bacteroides, Bifidobacterium, Parabacter, Prevotella, and Clostridium after intervention with plant foods. However, the changes in BAs modulated by the plant food profile did not show regularity. In addition, plant herbs, such as Scutellaria baicalensis, Rhizoma coptidis, Ganoderma lucidum, and Grifola frondosa, can also significantly improve the disturbance of GM and BA spectra caused in NAFLD, most of which are anti-NAFLD drugs commonly used in clinical settings. Furthermore, the abundance of Escherichia coli, Bacteroides, Lactobacillus, and Monascus changed frequently after drug treatment. Notably, most drugs improved NAFLD by acting on hepatic CYP7A1, indicating that the BA synthesis pathway accounts for a large proportion of treatment with plant herbs. From the perspective of pathogenesis, both foods and drugs improved glucose/lipid metabolism, insulin resistance, and inflammation in NAFLD. Interestingly, alterations in lipid metabolism were the most obvious, indicating the importance of this pathway.
In addition, active ingredients, including polysaccharides, oligosaccharides, polyphenols, alkaloids, and triterpenoids, can effectively regulate joint action in NAFLD. In the intestine of NAFLD hosts, the active substances mainly regulate the relative abundance of Deferrobacterium, Akkermania, Parabacter, Bifidobacterium, Clostridium, Bacteroides, and Desulfovibrio. Meanwhile, the synthesis pathway of hepatic BAs and modification of primary BAs were significantly modulated after intervention with active ingredients. Also, hepatic lipid metabolism plays a major role in the effect on active ingredients against NAFLD.
However, some limitations in this field remain. Although natural plants have been shown to regulate the interplay of GM and BAs in NAFLD, most studies have not fully clarified the regulatory effect on the interaction between GM and Bas, except for theanbrownin, suggesting that the potential mechanisms of many natural plants need to be further explored. In addition, it remains unclear whether some natural plants can be used as clinical drugs or dietary supplements. Meanwhile, heterogeneity between NAFLD patients should be considered in the clinical setting. Furthermore, the stability and safety of the natural plants need to be confirmed both in vivo and in vitro. Nevertheless, more in-depth research should be conducted to explore the potential mechanism of the interaction between GM and BAs to better reveal the therapeutic effect of natural plants on NAFLD.
QF conceived this study. QS was responsible for organizing the literature and drawing chemical formulas. QS, XX, and ZA were in charge of consulting the literature. QS wrote the manuscript and drew the figures. QF and YH supervised the study and gave final approval of the version to be published. All authors critically participated in the discussion and commented on the manuscript.
This work was supported by the Key projects of national natural fund of China (No. 81830119 to YH), the National Natural Science Foundation of China (No. 82174186 to YH; No. 82174040 to QF), the Shanghai Key Laboratory of Traditional Chinese Clinical Medicine (No. 20DZ2272200), and the Innovative Projects of Shanghai University of Traditional Chinese Medicine (No. Y2021081 to QS).
The authors declare that the research was conducted in the absence of any commercial or financial relationships that could be construed as a potential conflict of interest.
All claims expressed in this article are solely those of the authors and do not necessarily represent those of their affiliated organizations, or those of the publisher, the editors and the reviewers. Any product that may be evaluated in this article, or claim that may be made by its manufacturer, is not guaranteed or endorsed by the publisher.
α-MCA, α-murocholic acid; ALT, alanine aminotransferase; adenosine; AST, serum aspartate aminotransferase; ASBT, apical sodium-dependent bile acid transporter; BAs, bile acids; BSH, bile salt hydrolase; β-MCA, β-murocholic acid; CA, Cholic acid; CDCA, Chenodeoxycholic acid; CYP7A1, cytochrome P450 family 27 subfamily A member 1; CYP7B1, cytochrome P450 family 7 subfamily B member 1; DCA, deoxycholic acid; FXR, farnesoid X receptor; FFA, free fatty acid; GM, gut microbiota; HDL-C, high-density lipoprotein cholesterol; HFD, high-fat diet; HFHC, high-fat high-calorie; IBABP, ileal bile acid binding protein; LCA, lithocholic acid; LDL-C, low-density lipoprotein cholesterol; NAFL, non-alcoholic fatty liver; NAFLD, non-alcoholic fatty liver disease; NAS, NAFLD activity score; NASH, non-alcoholic steatohepatitis; OCA, obeticholic acid; OST, organic solute transporter;SCFAs, short-chain fatty acids; SHP, small heterodimer partner; SREBP-1, sterol regulatory element binding protein-1; TβMCA, tauro-β-muricholic acid; TC, total cholesterol; TG, triglyceride; TMAO, trimethylamine oxide; UDCA, ursodeoxycholic.
Agus, A., Clement, K., Sokol, H. (2021). Gut Microbiota-Derived Metabolites as Central Regulators in Metabolic Disorders. Gut 70 (6), 1174–1182. doi: 10.1136/gutjnl-2020-323071
Arab, J. P., Karpen, S. J., Dawson, P. A., Arrese, M., Trauner, M. (2017). Bile Acids and Nonalcoholic Fatty Liver Disease: Molecular Insights and Therapeutic Perspectives. Hepatology 65 (1), 350–362. doi: 10.1002/hep.28709
Aron-Wisnewsky, J., Vigliotti, C., Witjes, J., Le, P., Holleboom, A. G., Verheij, J., et al. (2020). Gut Microbiota and Human NAFLD: Disentangling Microbial Signatures From Metabolic Disorders. Nat. Rev. Gastroenterol. Hepatol. 17 (5), 279–297. doi: 10.1038/s41575-020-0269-9
Aune, D., Keum, N., Giovannucci, E., Fadnes, L. T., Boffetta, P., Greenwood, D. C., et al. (2016). Whole Grain Consumption and Risk of Cardiovascular Disease, Cancer, and All Cause and Cause Specific Mortality: Systematic Review and Dose-Response Meta-Analysis of Prospective Studies. BMJ 353, i2716. doi: 10.1136/bmj.i2716
Bag, S., Mondal, A., Majumder, A., Banik, A. (2022). Tea and its Phytochemicals: Hidden Health Benefits & Modulation of Signaling Cascade by Phytochemicals. Food Chem. 371, 131098. doi: 10.1016/j.foodchem.2021.131098
Berna, G., Romero-Gomez, M. (2020). The Role of Nutrition in non-Alcoholic Fatty Liver Disease: Pathophysiology and Management. Liver. Int. 40 Suppl;1, 102–108. doi: 10.1111/liv.14360
Campos-Vega, R., Oomah, B. D., Loarca-Pina, G., Vergara-Castaneda, H. A. (2013). Common Beans and Their Non-Digestible Fraction: Cancer Inhibitory Activity-An Overview. Foods 2 (3), 374–392. doi: 10.3390/foods2030374
Chavez-Talavera, O., Tailleux, A., Lefebvre, P., Staels, B. (2017). Bile Acid Control of Metabolism and Inflammation in Obesity, Type 2 Diabetes, Dyslipidemia, and Nonalcoholic Fatty Liver Disease. Gastroenterology 152 (7), 1679–1694 e1673. doi: 10.1053/j.gastro.2017.01.055
Chen, L., Huang, G. (2018). Antitumor Activity of Polysaccharides: An Overview. Curr. Drug Targets 19 (1), 89–96. doi: 10.2174/1389450118666170704143018
Chiang, J. Y. (2013). Bile Acid Metabolism and Signaling. Compr. Physiol. 3 (3), 1191–1212. doi: 10.1002/cphy.c120023
Day, C. P., James, O. F. (1998). Steatohepatitis: A Tale of Two "Hits"? Gastroenterology 114 (4), 842–845. doi: 10.1016/s0016-5085(98)70599-2
Eckburg, P. B., Bik, E. M., Bernstein, C. N., Purdom, E., Dethlefsen, L., Sargent, M., et al. (2005). Diversity of the Human Intestinal Microbial Flora. Science 308 (5728), 1635–1638. doi: 10.1126/science.1110591
Friedman, E. S., Li, Y., Shen, T. D., Jiang, J., Chau, L., Adorini, L., et al. (2018a). FXR-Dependent Modulation of the Human Small Intestinal Microbiome by the Bile Acid Derivative Obeticholic Acid. Gastroenterology 155 (6), 1741–1752.e1745. doi: 10.1053/j.gastro.2018.08.022
Friedman, S. L., Neuschwander-Tetri, B. A., Rinella, M., Sanyal, A. J. (2018b). Mechanisms of NAFLD Development and Therapeutic Strategies. Nat. Med. 24 (7), 908–922. doi: 10.1038/s41591-018-0104-9
Ghaffarzadegan, T., Marungruang, N., Fak, F., Nyman, M. (2016). Molecular Properties of Guar Gum and Pectin Modify Cecal Bile Acids, Microbiota, and Plasma Lipopolysaccharide-Binding Protein in Rats. PloS One 11 (6), e0157427. doi: 10.1371/journal.pone.0157427
Gonzalez, F. J., Jiang, C., Patterson, A. D. (2016). An Intestinal Microbiota-Farnesoid X Receptor Axis Modulates Metabolic Disease. Gastroenterology 151 (5), 845–859. doi: 10.1053/j.gastro.2016.08.057
Hales, C. M., Fryar, C. D., Carroll, M. D., Freedman, D. S., Ogden, C. L. (2018). Trends in Obesity and Severe Obesity Prevalence in US Youth and Adults by Sex and Age 2007-2008 to 2015-2016. JAMA 319 (16), 1723–1725. doi: 10.1001/jama.2018.3060
Han, X., Guo, J., Yin, M., Liu, Y., You, Y., Zhan, J., et al. (2020). Grape Extract Activates Brown Adipose Tissue Through Pathway Involving the Regulation of Gut Microbiota and Bile Acid. Mol. Nutr. Food Res. 64 (10), e2000149. doi: 10.1002/mnfr.202000149
Hao, W., Zhu, H., Chen, J., Kwek, E., He, Z., Liu, J., et al. (2020). Wild Melon Seed Oil Reduces Plasma Cholesterol and Modulates Gut Microbiota in Hypercholesterolemic Hamsters. J. Agric. Food Chem. 68 (7), 2071–2081. doi: 10.1021/acs.jafc.9b07302
He, K., Hu, Y., Ma, H., Zou, Z., Xiao, Y., Yang, Y., et al. (2016). Rhizoma Coptidis Alkaloids Alleviate Hyperlipidemia in B6 Mice by Modulating Gut Microbiota and Bile Acid Pathways. Biochim. Biophys. Acta 1862 (9), 1696–1709. doi: 10.1016/j.bbadis.2016.06.006
He, B., Jiang, J., Shi, Z., Wu, L., Yan, J., Chen, Z., et al. (2021). Pure Total Flavonoids From Citrus Attenuate non-Alcoholic Steatohepatitis via Regulating the Gut Microbiota and Bile Acid Metabolism in Mice. BioMed. Pharmacother. 135, 111183. doi: 10.1016/j.biopha.2020.111183
He, X., Wang, X., Fang, J., Chang, Y., Ning, N., Guo, H., et al. (2017). Polysaccharides in Grifola Frondosa Mushroom and Their Health Promoting Properties: A Review. Int. J. Biol. Macromol. 101, 910–921. doi: 10.1016/j.ijbiomac.2017.03.177
Huang, F., Zheng, X., Ma, X., Jiang, R., Zhou, W., Zhou, S., et al. (2019). Theabrownin From Pu-Erh Tea Attenuates Hypercholesterolemia via Modulation of Gut Microbiota and Bile Acid Metabolism. Nat. Commun. 10 (1), 4971. doi: 10.1038/s41467-019-12896-x
Hu, H., Zhang, S., Liu, F., Zhang, P., Muhammad, Z., Pan, S. (2019). Role of the Gut Microbiota and Their Metabolites in Modulating the Cholesterol-Lowering Effects of Citrus Pectin Oligosaccharides in C57BL/6 Mice. J. Agric. Food Chem. 67 (43), 11922–11930. doi: 10.1021/acs.jafc.9b03731
Inagaki, T., Moschetta, A., Lee, Y. K., Peng, L., Zhao, G., Downes, M., et al. (2006). Regulation of Antibacterial Defense in the Small Intestine by the Nuclear Bile Acid Receptor. Proc. Natl. Acad. Sci. U. S. A. 103 (10), 3920–3925. doi: 10.1073/pnas.0509592103
Inagaki, K., Nishimura, Y., Iwata, E., Manabe, S., Goto, M., Ogura, Y., et al. (2016). Hypolipidemic Effect of the Autoclaved Extract Prepared From Pea (Pisum Sativum L.) Pods In Vivo and In Vitro. J. Nutr. Sci. Vitaminol. (Tokyo) 62 (5), 322–329. doi: 10.3177/jnsv.62.322
Jiao, N., Baker, S. S., Chapa-Rodriguez, A., Liu, W., Nugent, C. A., Tsompana, M., et al. (2018). Suppressed Hepatic Bile Acid Signalling Despite Elevated Production of Primary and Secondary Bile Acids in NAFLD. Gut 67 (10), 1881–1891. doi: 10.1136/gutjnl-2017-314307
Jiao, N., Loomba, R., Yang, Z. H., Wu, D., Fang, S., Bettencourt, R., et al. (2021). Alterations in Bile Acid Metabolizing Gut Microbiota and Specific Bile Acid Genes as a Precision Medicine to Subclassify NAFLD. Physiol. Genomics 53 (8), 336–348. doi: 10.1152/physiolgenomics.00011.2021
Jia, W., Xie, G., Jia, W. (2018). Bile Acid-Microbiota Crosstalk in Gastrointestinal Inflammation and Carcinogenesis. Nat. Rev. Gastroenterol. Hepatol. 15 (2), 111–128. doi: 10.1038/nrgastro.2017.119
Khan, N., Afaq, F., Mukhtar, H. (2008). Cancer Chemoprevention Through Dietary Antioxidants: Progress and Promise. Antioxid. Redox Signal. 10 (3), 475–510. doi: 10.1089/ars.2007.1740
Khoo, J., Hsiang, J., Taneja, R., Law, N. M., Ang, T. L. (2017). Comparative Effects of Liraglutide 3 Mg vs Structured Lifestyle Modification on Body Weight, Liver Fat and Liver Function in Obese Patients With Non-Alcoholic Fatty Liver Disease: A Pilot Randomized Trial. Diabetes Obes. Metab. 19 (12), 1814–1817. doi: 10.1111/dom.13007
Kolodziejczyk, A. A., Zheng, D., Shibolet, O., Elinav, E. (2019). The Role of the Microbiome in NAFLD and NASH. EMBO Mol. Med. 11 (2), e9302. doi: 10.15252/emmm.201809302
Kouakou, K., Schepetkin, I. A., Yapi, A., Kirpotina, L. N., Jutila, M. A., Quinn, M. T. (2013). Immunomodulatory Activity of Polysaccharides Isolated From Alchornea Cordifolia. J. Ethnopharmacol. 146 (1), 232–242. doi: 10.1016/j.jep.2012.12.037
Kurdi, P., Kawanishi, K., Mizutani, K., Yokota, A. (2006). Mechanism of Growth Inhibition by Free Bile Acids in Lactobacilli and Bifidobacteria. J. Bacteriol. 188 (5), 1979–1986. doi: 10.1128/JB.188.5.1979-1986.2006
Leng, J., Huang, F., Hai, Y., Tian, H., Liu, W., Fang, Y., et al. (2020). Amelioration of non-Alcoholic Steatohepatitis by Qushi Huayu Decoction Is Associated With Inhibition of the Intestinal Mitogen-Activated Protein Kinase Pathway. Phytomedicine 66, 153135. doi: 10.1016/j.phymed.2019.153135
Ley, R. E., Turnbaugh, P. J., Klein, S., Gordon, J. I. (2006). Microbial Ecology: Human Gut Microbes Associated With Obesity. Nature 444 (7122), 1022–1023. doi: 10.1038/4441022a
Liang, T. T., Tong, L. T., Geng, D. H., Wang, L. L., Zhou, X. R., Pu, H. Y., et al. (2019). Wheat Gluten Regulates Cholesterol Metabolism by Modulating Gut Microbiota in Hamsters With Hyperlipidemia. J. Oleo. Sci. 68 (9), 909–922. doi: 10.5650/jos.ess18257
Li, T., Chiang, J. Y. (2014). Bile Acid Signaling in Metabolic Disease and Drug Therapy. Pharmacol. Rev. 66 (4), 948–983. doi: 10.1124/pr.113.008201
Li, L., Guo, W. L., Zhang, W., Xu, J. X., Qian, M., Bai, W. D., et al. (2019). Grifola Frondosa Polysaccharides Ameliorate Lipid Metabolic Disorders and Gut Microbiota Dysbiosis in High-Fat Diet Fed Rats. Food Funct. 10 (5), 2560–2572. doi: 10.1039/c9fo00075e
Li, P., Huang, J., Xiao, N., Cai, X., Yang, Y., Deng, J., et al. (2020). Sacha Inchi Oil Alleviates Gut Microbiota Dysbiosis and Improves Hepatic Lipid Dysmetabolism in High-Fat Diet-Fed Rats. Food Funct. 11 (7), 5827–5841. doi: 10.1039/d0fo01178a
Liu, C., Yang, S., Wang, K., Bao, X., Liu, Y., Zhou, S., et al. (2019). Alkaloids From Traditional Chinese Medicine Against Hepatocellular Carcinoma. BioMed. Pharmacother. 120, 109543. doi: 10.1016/j.biopha.2019.109543
Liu, Q., Zhu, L., Cheng, C., Hu, Y. Y., Feng, Q. (2017). Natural Active Compounds From Plant Food and Chinese Herbal Medicine for Nonalcoholic Fatty Liver Disease. Curr. Pharm. Des. 23 (34), 5136–5162. doi: 10.2174/1381612823666170918120643
Lu, J., He, R., Sun, P., Zhang, F., Linhardt, R. J., Zhang, A. (2020). Molecular Mechanisms of Bioactive Polysaccharides From Ganoderma Lucidum (Lingzhi), a Review. Int. J. Biol. Macromol. 150, 765–774. doi: 10.1016/j.ijbiomac.2020.02.035
Maattanen, P., Lurz, E., Botts, S. R., Wu, R. Y., Robinson, S. C., Yeung, C. W., et al. (2020). Plant- and Fish-Derived N-3 PUFAs Suppress Citrobacter Rodentium-Induced Colonic Inflammation. Mol. Nutr. Food Res. 64 (6), e1900873. doi: 10.1002/mnfr.201900873
Manach, C., Williamson, G., Morand, C., Scalbert, A., Remesy, C. (2005). Bioavailability and Bioefficacy of Polyphenols in Humans. I. Review of 97 Bioavailability Studies. Am. J. Clin. Nutr. 81 (1 Suppl), 230S–242S. doi: 10.1093/ajcn/81.1.230S
Manson, J. E., Cook, N. R., Lee, I. M., Christen, W., Bassuk, S. S., Mora, S., et al. (2019). Marine N-3 Fatty Acids and Prevention of Cardiovascular Disease and Cancer. N. Engl. J. Med. 380 (1), 23–32. doi: 10.1056/NEJMoa1811403
Marshall, J. C. (1998). The Gut as a Potential Trigger of Exercise-Induced Inflammatory Responses. Can. J. Physiol. Pharmacol. 76 (5), 479–484. doi: 10.1139/cjpp-76-5-479
McCranie, E. K., Bachmann, B. O. (2014). Bioactive Oligosaccharide Natural Products. Nat. Prod. Rep. 31 (8), 1026–1042. doi: 10.1039/c3np70128j
McCrory, M. A., Hamaker, B. R., Lovejoy, J. C., Eichelsdoerfer, P. E. (2010). Pulse Consumption, Satiety, and Weight Management. Adv. Nutr. 1 (1), 17–30. doi: 10.3945/an.110.1006
Meneses, M. E., Martinez-Carrera, D., Torres, N., Sanchez-Tapia, M., Aguilar-Lopez, M., Morales, P., et al. (2016). Hypocholesterolemic Properties and Prebiotic Effects of Mexican Ganoderma Lucidum in C57BL/6 Mice. PloS One 11 (7), e0159631. doi: 10.1371/journal.pone.0159631
Meng, X., Li, S., Li, Y., Gan, R. Y., Li, H. B. (2018). Gut Microbiota's Relationship With Liver Disease and Role in Hepatoprotection by Dietary Natural Products and Probiotics. Nutrients 10 (10), 1457. doi: 10.3390/nu10101457
Micioni Di Bonaventura, M. V., Cecchini, C., Vila-Donat, P., Caprioli, G., Cifani, C., Coman, M. M., et al. (2017). Evaluation of the Hypocholesterolemic Effect and Prebiotic Activity of a Lentil (Lens Culinaris Medik) Extract. Mol. Nutr. Food Res. 61 (11), 1700430. doi: 10.1002/mnfr.201700403
Naito, Y., Ushiroda, C., Mizushima, K., Inoue, R., Yasukawa, Z., Abe, A., et al. (2020). Epigallocatechin-3-Gallate (EGCG) Attenuates non-Alcoholic Fatty Liver Disease via Modulating the Interaction Between Gut Microbiota and Bile Acids. J. Clin. Biochem. Nutr. 67 (1), 2–9. doi: 10.3164/jcbn.20-39
NCD Risk Factor Collaboration (2017). Worldwide Trends in Body-Mass Index, Underweight, Overweight, and Obesity From 1975 to 2016: A Pooled Analysis of 2416 Population-Based Measurement Studies in 128.9 Million Children, Adolescents, and Adults. Lancet 390 (10113), 2627–2642. doi: 10.1016/S0140-6736(17)32129-3
Pan, Y. Y., Zeng, F., Guo, W. L., Li, T. T., Jia, R. B., Huang, Z. R., et al. (2018). Effect of Grifola Frondosa 95% Ethanol Extract on Lipid Metabolism and Gut Microbiota Composition in High-Fat Diet-Fed Rats. Food Funct. 9 (12), 6268–6278. doi: 10.1039/c8fo01116h
Powell, E. E., Wong, V. W., Rinella, M. (2021). Non-Alcoholic Fatty Liver Disease. Lancet 397 (10290), 2212–2224. doi: 10.1016/S0140-6736(20)32511-3
Rebello, C. J., Greenway, F. L., Finley, J. W. (2014). A Review of the Nutritional Value of Legumes and Their Effects on Obesity and its Related Co-Morbidities. Obes. Rev. 15 (5), 392–407. doi: 10.1111/obr.12144
Ren, Y., Kinghorn, A. D. (2019). Natural Product Triterpenoids and Their Semi-Synthetic Derivatives With Potential Anticancer Activity. Planta. Med. 85 (11-12), 802–814. doi: 10.1055/a-0832-2383
Ridlon, J. M., Kang, D. J., Hylemon, P. B. (2006). Bile Salt Biotransformations by Human Intestinal Bacteria. J. Lipid Res. 47 (2), 241–259. doi: 10.1194/jlr.R500013-JLR200
Romero-Cordoba, S. L., Salido-Guadarrama, I., Meneses, M. E., Cosentino, G., Iorio, M. V., Tagliabue, E., et al. (2020). Mexican Ganoderma Lucidum Extracts Decrease Lipogenesis Modulating Transcriptional Metabolic Networks and Gut Microbiota in C57BL/6 Mice Fed With a High-Cholesterol Diet. Nutrients 13 (1), 38. doi: 10.3390/nu13010038
Sayin, S. I., Wahlstrom, A., Felin, J., Jantti, S., Marschall, H. U., Bamberg, K., et al. (2013). Gut Microbiota Regulates Bile Acid Metabolism by Reducing the Levels of Tauro-Beta-Muricholic Acid, a Naturally Occurring FXR Antagonist. Cell Metab. 17 (2), 225–235. doi: 10.1016/j.cmet.2013.01.003
Schubert, K., Olde Damink, S. W. M., von Bergen, M., Schaap, F. G. (2017). Interactions Between Bile Salts, Gut Microbiota, and Hepatic Innate Immunity. Immunol. Rev. 279 (1), 23–35. doi: 10.1111/imr.12579
Sheng, L., Jena, P. K., Hu, Y., Liu, H. X., Nagar, N., Kalanetra, K. M., et al. (2017). Hepatic Inflammation Caused by Dysregulated Bile Acid Synthesis is Reversible by Butyrate Supplementation. J. Pathol. 243 (4), 431–441. doi: 10.1002/path.4983
Song, Z., Cai, Y., Lao, X., Wang, X., Lin, X., Cui, Y., et al. (2019). Taxonomic Profiling and Populational Patterns of Bacterial Bile Salt Hydrolase (BSH) Genes Based on Worldwide Human Gut Microbiome. Microbiome 7 (1), 9. doi: 10.1186/s40168-019-0628-3
Streidl, T., Karkossa, I., Segura Munoz, R. R., Eberl, C., Zaufel, A., Plagge, J., et al. (2021). The Gut Bacterium Extibacter Muris Produces Secondary Bile Acids and Influences Liver Physiology in Gnotobiotic Mice. Gut. Microbes 13 (1), 1–21. doi: 10.1080/19490976.2020.1854008
Sun, L., Pang, Y., Wang, X., Wu, Q., Liu, H., Liu, B., et al. (2019a). Ablation of Gut Microbiota Alleviates Obesity-Induced Hepatic Steatosis and Glucose Intolerance by Modulating Bile Acid Metabolism in Hamsters. Acta Pharm. Sin. B. 9 (4), 702–710. doi: 10.1016/j.apsb.2019.02.004
Sun, N. X., Tong, L. T., Liang, T. T., Wang, L. L., Liu, L. Y., Zhou, X. R., et al. (2019b). Effect of Oat and Tartary Buckwheat - Based Food on Cholesterol - Lowering and Gut Microbiota in Hypercholesterolemic Hamsters. J. Oleo. Sci. 68 (3), 251–259. doi: 10.5650/jos.ess18221
Sydor, S., Best, J., Messerschmidt, I., Manka, P., Vilchez-Vargas, R., Brodesser, S., et al. (2020). Altered Microbiota Diversity and Bile Acid Signaling in Cirrhotic and Noncirrhotic NASH-HCC. Clin. Transl. Gastroenterol. 11 (3), e00131. doi: 10.14309/ctg.0000000000000131
Takahashi, S., Luo, Y., Ranjit, S., Xie, C., Libby, A. E., Orlicky, D. J., et al. (2020). Bile Acid Sequestration Reverses Liver Injury and Prevents Progression of Nonalcoholic Steatohepatitis in Western Diet-Fed Mice. J. Biol. Chem. 295 (14), 4733–4747. doi: 10.1074/jbc.RA119.011913
Tan, X., Liu, Y., Long, J., Chen, S., Liao, G., Wu, S., et al. (2019). Trimethylamine N-Oxide Aggravates Liver Steatosis through Modulation of Bile Acid Metabolism and Inhibition of Farnesoid X Receptor Signaling in Nonalcoholic Fatty Liver Disease. Mol. Nutr. Food Res. 63 (17), e1900257. doi: 10.1002/mnfr.201900257
Ticho, A. L., Malhotra, P., Dudeja, P. K., Gill, R. K., Alrefai, W. A. (2019). Intestinal Absorption of Bile Acids in Health and Disease. Compr. Physiol. 10 (1), 21–56. doi: 10.1002/cphy.c190007
Vilar-Gomez, E., Martinez-Perez, Y., Calzadilla-Bertot, L., Torres-Gonzalez, A., Gra-Oramas, B., Gonzalez-Fabian, L., et al. (2015). Weight Loss Through Lifestyle Modification Significantly Reduces Features of Nonalcoholic Steatohepatitis. Gastroenterology 149 (2), 367–378.e365; quiz e314-365. doi: 10.1053/j.gastro.2015.04.005
Wan, X. Z., Ai, C., Chen, Y. H., Gao, X. X., Zhong, R. T., Liu, B., et al. (2020). Physicochemical Characterization of a Polysaccharide From Green Microalga Chlorella Pyrenoidosa and Its Hypolipidemic Activity via Gut Microbiota Regulation in Rats. J. Agric. Food Chem. 68 (5), 1186–1197. doi: 10.1021/acs.jafc.9b06282
Wang, W., Zhao, J., Gui, W., Sun, D., Dai, H., Xiao, L., et al. (2018). Tauroursodeoxycholic Acid Inhibits Intestinal Inflammation and Barrier Disruption in Mice With Non-Alcoholic Fatty Liver Disease. Br. J. Pharmacol. 175 (3), 469–484. doi: 10.1111/bph.14095
Winston, J. A., Theriot, C. M. (2020). Diversification of Host Bile Acids by Members of the Gut Microbiota. Gut. Microbes 11 (2), 158–171. doi: 10.1080/19490976.2019.1674124
Xie, M., Chen, G., Wan, P., Dai, Z., Zeng, X., Sun, Y. (2019). Effects of Dicaffeoylquinic Acids From Ilex Kudingcha on Lipid Metabolism and Intestinal Microbiota in High-Fat-Diet-Fed Mice. J. Agric. Food Chem. 67 (1), 171–183. doi: 10.1021/acs.jafc.8b05444
Xie, Z., Jiang, H., Liu, W., Zhang, X., Chen, D., Sun, S., et al. (2020). The Triterpenoid Sapogenin (2alpha-OH-Protopanoxadiol) Ameliorates Metabolic Syndrome via the Intestinal FXR/GLP-1 Axis Through Gut Microbiota Remodelling. Cell Death Dis. 11 (9), 770. doi: 10.1038/s41419-020-02974-0
Xin, X., Cheng, C., Bei-Yu, C., Hong-Shan, L., Hua-Jie, T., Xin, W., et al. (2021). Caffeine and EGCG Alleviate High-Trans Fatty Acid and High-Carbohydrate Diet-Induced NASH in Mice: Commonality and Specificity. Front. Nutr. 8, 784354. doi: 10.3389/fnut.2021.784354
Xu, J., Li, M., Zhang, Y., Chu, S., Huo, Y., Zhao, J., et al. (2020). Huangjinya Black Tea Alleviates Obesity and Insulin Resistance via Modulating Fecal Metabolome in High-Fat Diet-Fed Mice. Mol. Nutr. Food Res. 64 (22), e2000353. doi: 10.1002/mnfr.202000353
Xu, X., Qu, R., Wu, W., Jiang, C., Shao, D., Shi, J. (2021). Applications of Microbial Co-Cultures in Polyketides Production. J. Appl. Microbiol. 130 (4), 1023–1034. doi: 10.1111/jam.14845
Yu, J., Zhang, H., Chen, L., Ruan, Y., Chen, Y., Liu, Q. (2021). Disease-Associated Gut Microbiota Reduces the Profile of Secondary Bile Acids in Pediatric Nonalcoholic Fatty Liver Disease. Front. Cell Infect. Microbiol. 11, 698852. doi: 10.3389/fcimb.2021.698852
Zhang, X., Osaka, T., Tsuneda, S. (2015). Bacterial Metabolites Directly Modulate Farnesoid X Receptor Activity. Nutr. Metab. (Lond). 12, 48. doi: 10.1186/s12986-015-0045-y
Zhang, L., Xie, C., Nichols, R. G., Chan, S. H., Jiang, C., Hao, R., et al. (2016). Farnesoid X Receptor Signaling Shapes the Gut Microbiota and Controls Hepatic Lipid Metabolism. mSystems 1 (5), e00070-16. doi: 10.1128/mSystems.00070-16
Zhao, L., Ma, P., Peng, Y., Wang, M., Peng, C., Zhang, Y., et al. (2021). Amelioration of Hyperglycaemia and Hyperlipidaemia by Adjusting the Interplay Between Gut Microbiota and Bile Acid Metabolism: Radix Scutellariae as a Case. Phytomedicine 83, 153477. doi: 10.1016/j.phymed.2021.153477
Zhao, T., Tang, H., Xie, L., Zheng, Y., Ma, Z., Sun, Q., et al. (2019). Scutellaria Baicalensis Georgi. (Lamiaceae): A Review of its Traditional Uses, Botany, Phytochemistry, Pharmacology and Toxicology. J. Pharm. Pharmacol. 71 (9), 1353–1369. doi: 10.1111/jphp.13129
Zhou, W., Guo, R., Guo, W., Hong, J., Li, L., Ni, L., et al. (2019b). Monascus Yellow, Red and Orange Pigments From Red Yeast Rice Ameliorate Lipid Metabolic Disorders and Gut Microbiota Dysbiosis in Wistar Rats Fed on a High-Fat Diet. Food Funct. 10 (2), 1073–1084. doi: 10.1039/c8fo02192a
Zhou, F., Zhou, J., Wang, W., Zhang, X. J., Ji, Y. X., Zhang, P., et al. (2019a). Unexpected Rapid Increase in the Burden of NAFLD in China From 2008 to 2018: A Systematic Review and Meta-Analysis. Hepatology 70 (4), 1119–1133. doi: 10.1002/hep.30702
Keywords: natural plants, active ingredients, non-alcoholic fatty liver disease, gut microbiota, bile acids
Citation: Sun Q, Xin X, An Z, Hu Y and Feng Q (2022) Therapeutic Potential of Natural Plants Against Non-Alcoholic Fatty Liver Disease: Targeting the Interplay Between Gut Microbiota and Bile Acids. Front. Cell. Infect. Microbiol. 12:854879. doi: 10.3389/fcimb.2022.854879
Received: 14 January 2022; Accepted: 16 February 2022;
Published: 09 March 2022.
Edited by:
Xiaolin Tong, China Academy of Chinese Medical Sciences, ChinaReviewed by:
Lixin Zhu, The Sixth Affiliated Hospital of Sun Yat-sen University, ChinaCopyright © 2022 Sun, Xin, An, Hu and Feng. This is an open-access article distributed under the terms of the Creative Commons Attribution License (CC BY). The use, distribution or reproduction in other forums is permitted, provided the original author(s) and the copyright owner(s) are credited and that the original publication in this journal is cited, in accordance with accepted academic practice. No use, distribution or reproduction is permitted which does not comply with these terms.
*Correspondence: YiYang Hu, eXlodWxpdmVyQDE2My5jb20=; Qin Feng, ZmVuZ3FpbkBzaHV0Y20uZWR1LmNu
Disclaimer: All claims expressed in this article are solely those of the authors and do not necessarily represent those of their affiliated organizations, or those of the publisher, the editors and the reviewers. Any product that may be evaluated in this article or claim that may be made by its manufacturer is not guaranteed or endorsed by the publisher.
Research integrity at Frontiers
Learn more about the work of our research integrity team to safeguard the quality of each article we publish.