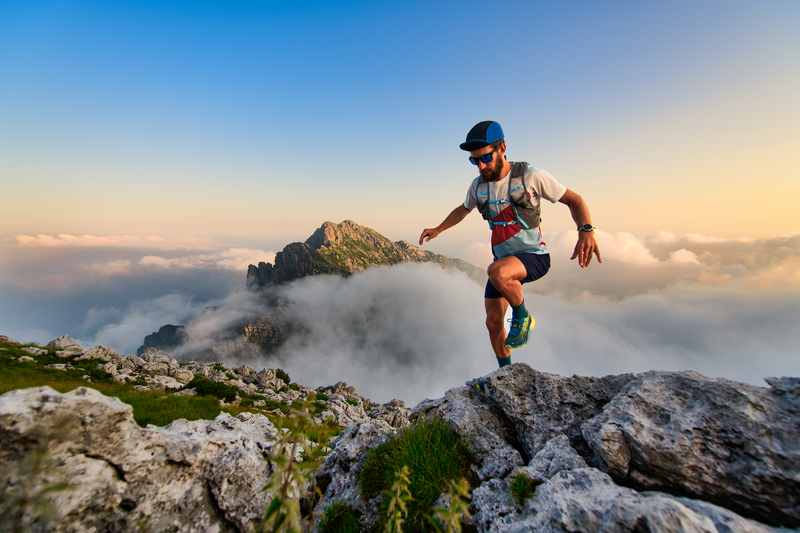
95% of researchers rate our articles as excellent or good
Learn more about the work of our research integrity team to safeguard the quality of each article we publish.
Find out more
REVIEW article
Front. Cell. Infect. Microbiol. , 12 July 2022
Sec. Parasite and Host
Volume 12 - 2022 | https://doi.org/10.3389/fcimb.2022.852902
This article is part of the Research Topic Leishmania Genome Variability: Impacts on Parasite Evolution, Parasitism and Leishmaniases Control View all 8 articles
Multiple genes and proteins have been identified as differentially expressed in the stages of the Leishmania life cycle. The differentiation processes are implicated in specific transcriptional and proteomic adjustments driven by gene expression regulation mechanisms. Leishmania parasites lack gene-specific transcriptional control, and gene expression regulation mostly depends on posttranscriptional mechanisms. Due to the lack of transcriptional regulation, criticism regarding the relevance of transcript quantification as a possible and efficient prediction of protein levels is recurrent in studies that use transcriptomic information. The advent of high-throughput technologies has improved the analysis of genomes, transcriptomes and proteomes for different organisms under several conditions. Nevertheless, defining the correlation between transcriptional and proteomic profiles requires arduous and expensive work and remains a challenge in Leishmania. In this review, we analyze transcriptomic and proteomic data for several Leishmania species in two different stages of the parasite life cycle: metacyclogenesis and amastigogenesis (amastigote differentiation). We found a correlation between mRNA and protein levels of 60.9% and 69.8% for metacyclogenesis and amastigogenesis, respectively; showing that majority mRNA and protein levels increase or decrease concomitantly. Among the analyzed genes that did not present correlation indicate that transcriptomic data should be carefully interpreted as protein expression. We also discuss possible explanations and mechanisms involved for this lack of correlation.
The central dogma of biology, as described by Francis Crick (Crick, 1970), provided a solid comprehension of the genetic flow followed by most cells, despite its limitations. The idea that genetic information flows from DNA to RNA molecules via transcription and from RNA to proteins via translation is still being vastly explored in molecular biology practices (Li and Xie, 2011; Liu et al., 2018; Schneider-Poetsch and Yoshida, 2018). The latest advances in genomics, transcriptomics and proteomics have enabled assessing levels of gene and protein expression in cells under different conditions (Kim et al., 2010; Zhang et al., 2014; Segundo-Val and Sanz-Lozano, 2016; Aslam et al., 2017; Hung and Weng, 2017). Considering that several mechanisms are involved in transcription and translation regulation, inferences can be made to extrapolate one type of data to predict the other, even though there is no trivial relationship between levels of transcripts and proteins (Jansen et al., 2002; Greenbaum et al., 2003; De Sousa Abreu et al., 2009; Buccitelli and Selbach, 2020).
In most eukaryotes, known regulatory mechanisms present monocistronic mRNAs with transcription regulated by individual promoters, enhancers and transcription factors (Cramer, 2019) resulting in highly regulated RNA production. Some of these mechanisms, however, are not present in trypanosomatids such as Leishmania, which exclusively utilizes posttranscriptional regulation (Martínez-Calvillo et al., 2004; Ivens et al., 2005; Clayton, 2016; De Pablos et al., 2016; Clayton, 2019). The absence of gene-specific transcription regulation raises questions about the relevance of quantifying mRNA in these organisms: How could the quantification of mRNA provide insight on the orchestration of the phenotype in different scenarios? What could be the relevance of measuring mRNA levels in these organisms? How is mRNA information relevant for overall expression analysis?
Leishmania is a protozoan parasite and causative agent of leishmaniases, a group of diseases characterized by cutaneous, mucocutaneous or visceral lesions. Leishmaniases is caused by at least 20 species of the Leishmania genus, affecting approximately 0.7 to 1 million people every year in nearly 100 endemic countries (Burza et al., 2018). According to the World Health Organization (WHO), leishmaniases are emerging tropical neglected diseases for which new treatments should be prioritized (Burza et al., 2018).
The parasite presents two different life forms: the promastigote and the amastigote (Figure 1). Drastic changes in pH, temperature and nutrient availability are related to differentiation (Gupta et al., 2001); these changes challenge the parasite’s ability to adapt under a lack of regulation at the transcriptional level. The promastigote forms present a motile elongated body with an apparent flagellum, live and multiply within the digestive tract of sandflies (25°C and pH ~7.0) in a microenvironment containing insect gut nutrients, digestive enzymes associated with its microbiota and saliva components (Kamhawi, 2006; Dostálová and Volf, 2012). In this environment, the promastigote undergoes a series of morphological changes that culminate in differentiation into metacyclic forms, which are the infectious and nonreplicative stage. This process includes the differentiation of procyclic promastigotes into nectomonad promastigotes, leptomonad promastigotes and metacyclic promastigotes (Sacks and Perkins, 1984; Bates and Tetley, 1993; Serafim et al., 2012). Metacyclic promastigotes are defined as highly infective, rapidly swimming, nonproliferative and present a long flagellum that allows motility to infect a mammalian host (Sacks and Perkins, 1984). Metacyclic promastigotes infect the host during the sandfly blood meal and differentiate into amastigotes once inside host cells. Additionally, new insight into parasite development have been described and have led to a revised Leishmania life cycle (Bates, 2018). Furthermore, metacyclic promastigote forms are able to dedifferentiate in the sandfly, enhancing parasite population growth through a second blood meal and providing a greater disease transmission potential (Serafim et al., 2018).
Figure 1 Leishmania life cycle in mammalian and insect hosts. The differentiation processes involve drastic changes in pH, temperature, and nutrient availability, challenging the parasite’s ability to orchestrate its gene expression accordingly. Metacyclogenesis is essentially represented in the right portion of the figure, comprising the differentiation from procyclic into nectomonad and then into leptomonad and metacyclic forms inside the sandfly digestive tract. Amastigogenesis is represented in the left portion of the figure, comprising the differentiation from metacyclic promastigote to amastigote forms inside the mammalian host macrophage. N, Macrophage Nucleus; PV, Parasitophorous Vacuole.
Once inside mammalian host cells, metacyclic promastigotes differentiate into amastigotes. The amastigote stage of Leishmania displays a rounded cell body with a nonapparent flagellum (Howells and Gardener, 1972; Gardener et al., 1973; Hentzer and Kobayasi, 1977). These life forms live and multiply inside the host phagocytic compartment (36°C and pH ~5.0).
The advent of high-throughput technologies has improved the analysis of genomes, transcriptomes and proteomes; however, establishing a correlation among these profiles is still a challenge. Most studies focus individually on mRNA or protein expression, as performing both can be time-consuming, expensive and demands trained personnel. Despite that, it is worth noting that general trends in groups of genes observed in transcriptomes or proteomes are not predictive of individual gene or protein expression. Therefore, the present review aims to discuss the correlation between mRNA and protein levels in the Leishmania life cycle, mainly with regard to metacyclogenesis and amastigote differentiation.
To understand the extent of Leishmania mRNA and protein studies, we conducted a simple search in PubMed using the keywords “mRNA”, “transcriptome”, “transcriptomic”, “protein”, “proteome”, “proteomic”, “leishmania” and the Boolean operator “AND”. Considering that transcriptomic is defined as a methodology to study transcriptome which is a set of mRNAs; and it is complementary to proteomic that is defined as a methodology to study proteome which is a set of proteins, we encompassed the number of publications up to December 2021 using these terminologies (Figure 2).
Figure 2 Graph showing the number of publications retrieved in PubMed using the terms “mRNA AND leishmania” (light blue bars), “protein AND leishmania” (red bars), “transcriptome AND leishmania” (dark blue bars), “proteome AND leishmania” (pink bars), “transcriptomic AND leishmania” (gray bars), and “proteomic AND leishmania” (purple bars). The searches were conducted up to December 2021.
A significant difference between the Boolean pairs was identified: 1,095 publications for “mRNA AND leishmania” versus 13,032 publications for “protein AND leishmania”, indicating an order of magnitude in favor of protein researches. The advent of new sequencing technologies starting in early 2000´s has generated studies on a large scale and the discrepancy decreased significantly, showing 213 publications for “transcriptome AND leishmania” versus 474 publications for “proteome AND leishmania”, and 136 publications for “transcriptomic AND leishmania” versus 137 publications for “proteomic AND leishmania” (Figure 2). Based on these findings, the question that we are approaching in this review remains open: how relevant is to quantify mRNA or protein, individually, to provide a phenotype pattern?
Protein-coding genes in the Leishmania genome are organized into long polycistronic units containing multiple open reading frames, as observed in chromosome 1 (containing 29 and 50 genes in each of the two polycistronic units) and chromosome 3 (containing 69 and 30 genes in each of the two polycistronic units) (Myler et al., 1999; Martínez-Calvillo et al., 2004). These units can be up to 100,000 bases long and have no introns. (Ivens et al., 2005; Clayton, 2016). The transcription of these units generates long precursor mRNAs that are processed cotranscriptionally, generating mature mRNAs corresponding to each gene originally encoded on the polycistronic unit (Liang et al., 2003; Martínez-Calvillo et al., 2004). Then, mRNAs are trans-spliced with the addition of a spliced leader (SL) molecule to the 5’ end of each mRNA (Clayton, 2016). The polyadenylation process occurs simultaneously, as the long polycistronic precursor is divided into smaller mature mRNAs encoding single genes to be translated (Michaeli et al., 1993; Liang et al., 2003; Michaeli, 2011; Preußer et al., 2012; Clayton, 2016). SL addition and polyadenylation enhance molecular stability and avoid degradation (Agabian, 1990), which can occur in the nucleus by RNase activity (Clayton, 2019). In this context, competition between mRNA degradation and processing is relevant to the levels of mature mRNAs in the cell (Fadda et al., 2014). Next, individual mature mRNAs are exported from the nucleus (Bühlmann et al., 2015). In the cytosol, the binding of eukaryotic translational initiation factors recruits ribosomes and initiates translation (Clayton, 2019) (Figure 3). Knockout of different translation initiation factors in Leishmania impacts the cells’ whole proteome, affecting parasite morphology and infectivity (Tupperwar et al., 2019; Baron et al., 2020; Shrivastava et al., 2021). Posttranscriptional and posttranslational controls, including mRNA stability, translation initiation and protein folding are mechanisms of gene expression regulation in Leishmania, indicating how complex the process from gene to protein can be (Clayton and Shapira, 2007; Haile and Papadopoulou, 2007; Stiles et al., 2016).
Figure 3 Main aspects of polycistronic and monocistronic gene expression. (A) Monocistronic coding regions are common to most higher eukaryotes. With this genome organization, protein-coding genes are organized within intronic portions that are nonprotein-coding regions. Transcription is regulated by promoters and enhancers; thus, transcription is individually controlled. Processing of precursor mRNA involves the removal of introns, 5’ cap addition and polyadenylation, generating the mature mRNA molecule that is translated after export from the nucleus. (B) The polycistronic organization of coding genes differentiates Leishmania gene expression. The absence of transcriptional control is shown, with several genes encoded in the same polycistronic coding region being transcribed together. Another difference is the existence of multicopy genes in tandem arrays. All these characteristics point to uncontrolled transcription. Processing of precursor mRNA involves trans-splicing (addition of the SL molecule), polyadenylation and RNAse activity to eliminate mRNAs that will not be translated.
In contrast, gene expression in higher eukaryotes is monocistronic, with coding regions individualized by the presence of gene transcription promoters and enhancers. Transcription factors activate different transcriptional programs, modulating the cellular response to environmental cues. Premature RNA processing in higher eukaryotes involves the removal of intronic regions after the addition of the 5’ cap, followed by poly-A tail synthesis (Figure 3), and these processes are generally coupled (Maniatis and Reed, 2002).
Recently, new mechanisms of regulation have been described to play important roles in Leishmania gene expression (Nandan et al., 2017; Azizi and Papadopoulou, 2020). This fact highlights that Leishmania possesses tightly tuned regulation of its gene expression, though the regulatory elements themselves are still largely uncharacterized (Fernandes et al., 2019). Among these, noncoding RNAs (ncRNAs) and RNA-binding proteins (RBPs) may contribute to the mRNA-protein level discrepancies observed in Leishmania.
ncRNAs vary in size and have many different mechanisms of action. In association with other molecules, ncRNAs control transcription, translation and RNA degradation (Mattick, 2001; Hombach and Kretz, 2016; Fernandes et al., 2019). Some L. major and L. donovani untranslated regions (UTRs) have been identified as origins of ncRNAs (Castro et al., 2017). Thousands of ncRNAs of different classes were also identified in the L. braziliensis genome and confirmed by RNA-seq analysis, suggesting that they are real transcripts (Torres et al., 2017; Ruy et al., 2019). Additionally, RNA-seq data have revealed a ncRNA among the top five differentially expressed transcripts in comparison of two L. amazonensis lineages, indicating an important role in the modulation of a specific metabolic pathway, such as arginase activity (Aoki et al., 2017). Developmentally regulated ncRNAs specific to the amastigote life stage of L. donovani present similar transcription characteristics to known protein-coding mRNAs (Dumas et al., 2006).
RBPs also play an important role in regulating translation in trypanosomatids and have been described as important regulatory components in Leishmania (Terrão et al., 2017; Ruy et al., 2019). RBPs interact with mRNAs, representing another posttranscriptional mechanism that regulates gene expression. The association of RBPs with methyltransferases suggests the role of these proteins in regulating arginine methylation, a known posttranslational modification of proteins (Ferreira et al., 2014). The relationship between RBPs and methyltransferases also impact on the virulence and protein stability of Leishmania (Ferreira et al., 2020). There is an evidence of a potential protective effect of RBPs in L. mexicana, accumulating in the nucleus in response to actinomycin D treatment (Názer and Sánchez, 2011).
The regulatory elements described in this session have not been fully explored and described in Leishmania yet, probably due to the complexity of their mechanisms and interactions, and also the lack of sufficient genome annotations. However, characterization of these elements might improve knowledge of the biology of the parasite, genomic organization, and regulation processes, providing potential new biomarkers and/or drug targets.
The Leishmania genome appears to be constitutively expressed at the transcriptional level (Leifso et al., 2007; Alcolea et al., 2019), presenting a small percentage of differentially expressed mRNAs between life stages (Holzer et al., 2006; Cohen-Freue et al., 2007; Saxena et al., 2007; Aoki et al., 2017). A previous comparative analysis of proteomes and transcriptomes of L. infantum during amastigogenesis revealed that although expression trends were comparable, fold changes usually did not correlate (McNicoll et al., 2006). To better understand the extent to which mRNA and protein data are connected in Leishmania, we reviewed published studies describing mRNA and protein expression during Leishmania development to find correlations focusing on selected genes and proteins related to differentiation of parasite forms during the life cycle.
Metacyclogenesis is essentially the process of differentiation from procyclic to metacyclic promastigotes. Throughout this process, procyclic promastigotes differentiate into nectomonad and leptomonad promastigote stages, finally becoming metacyclic promastigotes (Sacks and Perkins, 1984; Bates and Tetley, 1993; Sacks and Kamhawi, 2001; Serafim et al., 2012). At the proteomic level, metacyclic promastigotes present a reduction in the abundance of proteins involved in protein synthesis and an increase in proteins involved in cell motility (Mojtahedi et al., 2008; Amiri-Dashatan et al., 2020b). Additionally, transcriptomic analysis of metacyclogenesis has demonstrated that each promastigote life stage has exclusive differentially expressed transcripts associated with them (Inbar et al., 2017; Coutinho-Abreu et al., 2020).
Overall, the specific conditions that trigger metacyclogenesis have not been fully disclosed. In nature, this process occurs within the midgut of the sandfly and involves stress induction and a lack of nutrients (Bates and Tetley, 1993; Kamhawi, 2006; Bates, 2008). Furthermore, the absence of purines seems to play a role in triggering metacyclogenesis (Serafim et al., 2012). Binding to the midgut of the insect is an essential process for promastigote development and this binding capability is strictly stage specific: it is observed in the leptomonad and nectomonad uninfective promastigote forms but it is not common in the metacyclic stage (Pimenta et al., 1992; Wilson et al., 2010). Surface glycoconjugate lipophosphoglycan (LPG) has been described as responsible for host midgut binding in L. major (Pimenta et al., 1992) and it is also hypothesized to be the molecule responsible for midgut binding in other Leishmania species (Sacks and Kamhawi, 2001). Detachment and release from the host midgut occurs as the LPG molecule becomes elongated and modified by transferase enzymes (McConville et al., 1992; Novozhilova and Bovin, 2010). The observed stage-specific ability to bind to the host midgut found in different Leishmania species (Pimenta et al., 1992; Wilson et al., 2010) is a finding that might indicate that the expression pattern of mRNAs and proteins involved in LPG synthesis is accordingly regulated.
Since the host midgut microenvironment plays an important role in metacyclogenesis, questions on the reliability of cultured promastigotes as a model, compared to sandfly-derived promastigotes, have emerged (Alcolea et al., 2016). The insect microenvironment contains several digestive enzymes and a microbiota. Some stage-specific molecules have been identified to play a role in protecting the parasite from proteolytic activity found within the insect midgut (Sacks and Kamhawi, 2001). Other molecules present in the saliva of the insect are also crucial to the development of infection in the mammal and are capable of suppressing the host immune response and determining the fate of the infection (Katz et al., 2000; Andrade et al., 2007). Transcriptomic comparisons among sandfly and culture-derived metacyclic promastigotes have revealed an overall transcriptional similarity, with specific differences in transcripts associated with nutrient stress (such as amino acid transport, fatty acid biosynthesis, catabolism of ketone bodies, and protein recycling via autophagy) that appear upregulated in cultured metacyclic promastigotes (Inbar et al., 2017).
To assess correlations between mRNA and protein levels in metacyclogenesis, we analyzed data from 6 different studies, 3 of which contained transcriptomic data (Almeida et al., 2004; Dillon et al., 2015; Inbar et al., 2017) and 3 of which contained proteomic data (Mojtahedi et al., 2008; Amiri-Dashatan et al., 2020a; Amiri-Dashatan et al., 2020b) from L. major and L. tropica, differentiating from procyclic to metacyclic promastigotes. To standardize our comparisons, we used the L. major Friedlin genome as a reference for gene IDs. Even though there are obvious differences in transcriptomic and proteomic profile between these two Leishmania species, the authors decided to use gene IDs of L. major since it is the most complete genomic database among Leishmania species. In cases in which the reference genome was another, we obtained syntenic orthologs of the L. major Friedlin genome using TryTrip database (www.tritrypdb.org). It is also worth noting that our search for studies upon which to base the present work retrieved a significantly smaller number of proteomic and transcriptomic studies of Leishmania metacyclogenesis in comparison to those focusing on amastigote differentiation. All analyzed data was statistically verified by each individual study then, the authors of this review did not interfere with or perform own statistical analysis of the data used. It is important to take into consideration that different studies may have presented different statistical thresholds to determine the own differentially expressed genes and proteins. Because of this limitation, the results observed here should be interpreted as proof-of-concept, since they were obtained from an extensive literature review and manual ad-hoc curation. The analysis was performed using a simple search tool in a table containing raw data from every analyzed study to find correlation between protein and mRNA trends. There is a consideration that some genes in the Leishmania genome can present the same coding region but differ in UTRs (Rastrojo et al., 2019). This aspect of the Leishmania genome organization is very complex and not discussed within the analyzed studies that were based solely on coding regions.
Table 1 shows differentially expressed mRNAs and proteins according to transcriptomic and proteomic studies comparing procyclic and metacyclic promastigotes. For most genes, correlations between protein and mRNA levels were detected, whereas some genes show opposite trends in comparisons. We analyzed genes within the following biological groups: stress response, mitochondria, gene expression, energy metabolism and cell signaling. Hypothetical proteins and other biological functions were also considered (Table 1). The genes were grouped to facilitate the analysis. Gene Ontology was originally characterized in the respective study (Almeida et al., 2004; Mojtahedi et al., 2008; Dillon et al., 2015; Inbar et al., 2017; Amiri-Dashatan et al., 2020a; Amiri-Dashatan et al., 2020b).
Table 1 Common genes found in independent transcriptomic and proteomic data obtained from metacyclogenesis (procyclic vs. metacyclicpromastigotes) differentiation.
For the stress response, two peroxidases (LmjF.26.0800 and LmjF.15.1040) appeared to be upregulated at both the mRNA and protein levels in metacyclic promastigotes in comparison to procyclic promastigote forms. This may represent an adaptation of the parasite during metacyclogenesis, preparing to infect mammalian cells. A putative gene (LmjF.12.1130 - NADH/flavin oxidoreductase) and a heat shock protein (LmjF.33.2390 - TRAP1/HSP75) did not present correlation between protein and mRNA levels, with both showing a decrease in transcript levels in metacyclic promastigotes and an increase in protein levels (Table 1).
During metacyclogenesis, correlation between mRNA and protein levels was observed for genes encoding for mitochondrial proteins: two cytochrome c oxidase subunits (LmjF.26.1710 and LmjF.25.1130) appeared downregulated in metacyclic promastigotes compared to procyclic promastigotes (Table 1).
The most expressive finding for metacyclogenesis was for gene expression-related genes. Of the 11 related genes found in our analysis, 8 (over 72%) were found to consistently be downregulated (both mRNA and proteins) in metacyclic promastigotes. This category included elongation factors (LmjF.18.0740, LmjF.36.0180, LmjF.34.0840), RNA helicases (LmjF.21.1552, LmjF.35.3100), histone H3 (LmjF.10.0870), guanosine monophosphate (GMP) reductase (LmjF.17.0725) and the protein transport protein SEC13 (LmjF.32.0050). This downregulation in gene expression-related proteins is in accordance with the previously demonstrated reduction in transcription and translation in metacyclic promastigotes (Kloehn et al., 2015).
Proteins involved in cell energy metabolism, such as phosphoglycerate kinase (PGKC) (LmjF.20.0100) and pyruvate kinase (LmjF.35.0030), were increased in both mRNA and protein levels during metacyclogenesis (Table 1). On the other hand, ATP-dependent 6-phosphofructokinase (PFK) (LmjF.29.2510), a main regulator of glycolysis (Mor et al., 2011), showed decreased levels during metacyclogenesis in L. tropica (Table 1), possibly indicating a reduction in glycolytic activity. These data, with reduced levels of cytochrome c oxidase subunits V and VII, corroborate the hypothesis that cell respiration is affected upon metacyclogenesis. Additionally, greater energy consumption has been shown to occur in procyclic promastigotes of L. mexicana than in metacyclic promastigotes due to a higher replication rate (Costa et al., 2011).
Interestingly, glutamate dehydrogenase (GDH) (LmjF.28.2910) shows opposite trends in different Leishmania species In L. major, both mRNA and protein levels of GDH increased in metacyclics in comparison to procyclic promastigotes. In L. tropica, however, there was a decrease in GDH (LmjF.28.2910) during metacyclogenesis at both protein and mRNA levels. Nonetheless, another GDH (LmjF.15.1010) presented no correlation when we compared L. major and L. tropica data, with decreased mRNA levels but increased protein levels (Table 1).
A putative lipophosphoglycan biosynthetic protein 3 (LPG3) is reportedly downregulated in metacyclic forms of L. tropica and L. major (Inbar et al., 2017; Amiri-Dashatan et al., 2020a). LPG is a protein responsible for insect midgut binding and also acts as a virulence factor in mammalian hosts. This supports the idea that regulation of LPG expression is stage-specific, as is the ability of Leishmania to bind to the host midgut. (Pimenta et al., 1992; Sacks and Kamhawi, 2001; Wilson et al., 2010). Metacyclic promastigotes, however, present upregulation of transferase mRNAs, in accordance with the previous identification of elongated LPG in this specific phase (Rowton et al., 1995). Adding to this hypothesis, mRNA for the glycosyltransferase gene, which is involved in elongation and modification of LPG, appears to be upregulated in nectomonads (Coutinho-Abreu et al., 2020). Other transferases (galactosyl and mannosyl-transferases) are also upregulated in nectomonad and metacyclic promastigotes, indicating that these stages of metacyclogenesis detach from the midgut, in accordance with what is known about the parasite life cycle.
Overall, of 46 common genes found in our independent analysis of transcriptomic and proteomic data, more than 60.9% (28 genes) presented a positive correlation between mRNA and protein levels in metacyclogenesis; the other 39.1% (18 genes) exhibited contrary trends (Table 1). Based on these findings, the data obtained from each individual study supports the hypothesis that transcriptional differences exist between procyclic and metacyclic forms in the Leishmania life cycle, even though the genome is mostly constitutively expressed.
Amastigote differentiation or amastigogenesis is the process by which metacyclic promastigotes differentiate into amastigotes inside phagocytic cells. Amastigotes live and replicate inside mammalian host cells in the compartment called parasitophorous vacuoles (PVs) or phagolysosomes (Figure 1), which is the most drastic change in environmental conditions faced by the parasite in its life cycle, involving pH, temperature, and nutrient availability changes, as previously described (Gupta et al., 2001). This environmental alteration causes some observable consequences on parasite gene expression, even though the vast majority of genes appear to be constitutively expressed (Cohen-Freue et al., 2007). An overall reduction in RNA content is observed in amastigotes compared to promastigotes (Shapira et al., 1988). Indeed, most differences in mRNA abundance are observed when comparing the amastigote and procyclic promastigote stages (Inbar et al., 2017). A study showed that RNA abundance seems to play an important role in the early stages of amastigogenesis, but later in the process, posttranscriptional and translational mechanisms act to regulate gene expression (Lahav et al., 2011). The same study found that approximately 20-30% of genes presented a correlation between mRNA and protein levels during differentiation, most of which were up/downregulated in the early stages of differentiation (Lahav et al., 2011).
Amastigogenesis can be induced in vitro for Leishmania species by subjecting promastigotes to environmental conditions that mimic the inside of the mammalian host cell (37°C, pH ~ 5.0) for over 5 h (Barak et al., 2005; Zilberstein, 2020). In this context, temperature seems to play an important role in altering the gene expression and morphology of the parasite, inducing differentiation (Zilberstein and Shapira, 1994; Kramer et al., 2008; Alcolea et al., 2010b; Zilberstein, 2020). Although axenic amastigotes have been proven to be good models for amastigote studies, they lack complex host signaling and downstream effects (Saar et al., 1998; Gupta et al., 2001; Barak et al., 2005). RNA expression studies have revealed transcriptional differences between axenic amastigotes and intracellular amastigotes related to metabolic processes, surface proteins, intracellular transport and response to oxidative stress (Rochette et al., 2009).
A remarkable change in amastigote morphology is the flagellum structure. The paraflagellar rod structure is known to compose the flagellum in kinetoplastids during specific flagellated life stages; its absence has been observed in amastigotes (Portman and Gull, 2010). Accordingly, several studies have identified upregulation of paraflagellar rod protein 1 and 2 mRNAs (PRF1 and PRF2) in promastigotes of approximately 10- to 15-fold higher than in amastigotes (Moore et al., 1996; Mishra et al., 2003). PRF genes encode a component of the paraflagellar rod.
Another change involves the transport of sugars in promastigote and amastigote metabolism. It is known that the main carbon source for promastigotes is sugar and amino acids but that amastigotes mainly utilize amino acids and fatty acids (Krassner, 1969; Krassner and Flory, 1972; Hart and Coombs, 1982; Kuile and Opperdoes, 1992; Opperdoes and Coombs, 2007). A transcriptomic analysis confirmed that levels of different sugar and amino acid transporter genes are upregulated in procyclic promastigotes in comparison to amastigotes (Inbar et al., 2017). In metacyclogenesis, the mRNA levels of these transporters further increased from procyclic promastigotes to neptomonads, reaching their peak in the metacyclic stage (Inbar et al., 2017). This is strong evidence that observable metabolic changes during the Leishmania life cycle can be detected at the transcriptomic level.
Moreover, there are similarities between the transcriptional profile of metacyclic promastigotes and amastigotes (for example, in comparison to procyclics, metacyclic promastigotes present an upregulation of amastin-like proteins - known to be characteristic of the amastigote phase of Leishmania), suggesting that metacyclogenesis is a process that “prepares” the parasite for infection (Inbar et al., 2017).
To assess correlations between mRNA and protein levels in amastigogenesis, we analyzed data from 8 articles involving five Leishmania species: 5 contained transcriptomic data (Almeida et al., 2004; Holzer et al., 2006; Leifso et al., 2007; Saxena et al., 2007; Alcolea et al., 2010a) and 3 proteomic data (Walker et al., 2006; Leifso et al., 2007; Brotherton et al., 2010). These studies obtained Leishmania amastigotes in different ways: recovered from BALB/c mice lesions (Almeida et al., 2004; Holzer et al., 2006, and Leifso et al., 2007), macrophage lysis (Alcolea et al., 2010a) and in vitro cultivation of axenic amastigotes (Walker et al., 2006; Saxena et al., 2007 and Brotherton et al., 2010). The analysis was performed in a similar manner as previously described for metacyclogenesis.
In these works, differentially expressed mRNAs and proteins were compared and correlations were established (Table 2). Each gene listed in Table 2 was identified as differentially expressed in the analyzed proteomic or transcriptomic studies comparing promastigote to amastigote differentiation. Following the same trend observed for promastigotes, a positive correlation between mRNA and protein levels was found for most genes in Table 2 although some genes presented opposite levels. We analyzed genes within the following biological groups: stress response, gene expression, energy metabolism, cell signaling and proliferation. Hypothetical proteins and other biological functions were also considered (Table 2).
Table 2 Common genes found in independent transcriptomic and proteomic data obtained from amastigogenesis (promastigote vs. amastigote) differentiation.
Stress response proteins appeared upregulated in amastigotes compared to promastigotes in response to the drastic environmental changes that trigger differentiation and the hostile acidic PV environment. mRNA and protein levels of stress response proteins, such as HSP70 (LmjF.28.2780) and Chaperone protein DNAJ homolog – JDP7 (LmjF.32.1940), presented a positive correlation and were consistently upregulated in amastigotes. On the other hand, superoxide dismutase (LmjF.32.1820) showed opposite mRNA and protein levels, with a decrease in mRNA but an increase in protein. It is interesting to note that some HSPs (LmjF.28.2780 and LmjF.33.0312) presented correlations but that others (LmjF.28.2781 and LmjF.36.2030) did not. Stress inducible protein 1 (STI1) transcripts are heat inducible in L. major promastigotes, with increased transcript levels when subjected to an increased temperature (Webb et al., 1997). Our analysis revealed a decrease in both the mRNA and protein content of an STI1 homolog in amastigotes of L. mexicana and L. donovani (mRNA data) and L. infantum (protein data).
Gene expression-related genes appeared mostly downregulated, with mRNA and protein levels of 10 out of 14 genes being consistently downregulated in amastigotes, suggesting that gene expression is reduced in this life stage. In this category, we observed a polyA-binding protein (LmjF.35.5040), an RNA upstream-binding protein (UBP2) (LmjF.25.0490), and several histones. Histone H4 was the most notable observation, with 6 different genes being downregulated at both the mRNA and protein levels in amastigotes (LmjF.02.0020, LmjF.06.0010, LmjF.31.3180, LmjF.35.1310, LmjF.36.0020 and LmjF.25.2450). Reduced mRNA and protein levels histone 2B (LmjF.19.0030) also was detected in amastigotes, but histone 3 (LmjF.10.0970) mRNA and protein levels did not correlate.
For energy metabolism-related proteins, fructose 1,6-bisphosphate aldolase (LmjF.36.1260) and putative 3-ketoacyl-CoA thiolase-like protein (LmjF.31.1630) were the only proteins, of 6 in total, that were upregulated in amastigotes. These are coincidently the only two that did not present mRNA and protein level correlations, as their mRNAs were downregulated. For all 4 other proteins (LmjF.23.0690, LmjF.21.1770, LmjF.30.2970, LmjF.14.1160), both mRNA and protein levels were reduced upon amastigogenesis. The consistent downregulation of glycolytic enzymes (LmjF.30.2970 - glyceraldehyde 3-phosphate dehydrogenase and LmjF.14.1160 – Enolase) is in accordance with what has been described for L. mexicana amastigotes, utilizing less glucose in relation to promastigotes (Hart and Coombs, 1982).
It is also possible to observe some classical developmentally regulated genes, such as several GP63 and a few paraflagellar rod protein genes (PFR2). Both the protein and mRNA levels of these genes decrease during amastigote differentiation, in accordance with what is known about the parasite life cycle, as discussed previously.
One interesting trend unveiled by Table 2 is that of the 30 genes that presented mRNA and protein correlations, 86% (26 genes) showed decreasing expression in amastigotes. This is consistent with what has been reported about a decrease in mRNA and protein content in amastigotes (Shapira et al., 1988). Of the only 4 correlating genes in the table with increased expression during amastigogenesis, 3 are related to the stress response, and the other is a hypothetical protein.
Overall, of 43 common genes found in our analysis of independent transcriptomic and proteomic data, 30 (69.8%) presented positive correlations between mRNA and protein levels during amastigote differentiation; opposite trends in mRNA and protein levels were found for the other 13 genes (30.2%). Once again, this supports the idea that mRNA and protein levels can correlate in the Leishmania life cycle. This finding of over 69% correlation between mRNA and protein trends in amastigote differentiation is larger than the 53% previously observed in combined proteomic and transcriptomic studies of L. infantum differentiation (McNicoll et al., 2006). Our analysis, however, incorporated a larger number of Leishmania species and several independent studies, which might explain the difference in the percentage of mRNA/protein correlations.
It is important to point that this review was based on an extensive literature review and manual ad-hoc curation. No statistical analysis was performed with the data from all the analyzed studies, proposing this reading as a proof-of-concept. Our analyses were obtained from over 4,000 genes in transcriptomic data and over 400 proteins in proteomic data during metacyclogenesis and amastigonesis in Leishmania. Interestingly, only 46 and 43 genes were commonly differentially expressed in metacyclogenesis and amastigogenesis, respectively (Figure 4). Among these, 28 and 30 genes presented correlation between mRNA and protein levels in metacyclogenesis and amastigonesis, respectively (Figure 4). The considered genes that did not present correlation corroborated the idea that it is not always possible to accurately predict protein levels based solely on levels of mRNA, mostly due to extensive posttranscriptional mechanisms regulating gene expression. In particular case of our analysis the lack of correlation might be related to the different Leishmania species being compared that may interfere in establishing such correlations.
Figure 4 Differentially expressed genes and proteins observed in independent transcriptomic and proteomic studies of Leishmania life cycle. Both Venn Diagrams on the top portion of the figure describe the total number of DEPs and DEGs found in all the analyzed studies that was based this review. Further, among the commonly differentially expressed genes, we indicate the amount of genes that presented correlation between mRNA and protein levels in metacyclogenesis and amastigogenesis. It is worth noting that these results should be read as proof-of-concept, since no statistical analysis was performed to compare the analyzed studies.
The idea that the Leishmania genome is constitutively expressed at the transcriptional level does not necessarily mean that protein levels cannot follow the same trend of the corresponding mRNA to be expressed. Here, we showed that the mRNA and protein levels of several genes increase or decrease concomitantly during metacyclogenesis and amastigogenesis. These differences in mRNA and protein levels might also be used in epidemiological practice and/or research as stage-specific markers to identify, isolate and recognize specific life forms in the Leishmania life cycle.
To date, little research establishing the correlation of mRNA and protein levels in Leishmania has been performed due to the complexity of data handling. The increase in proteomic and transcriptomic data associated with detailed comparative analysis will certainly enrich the understanding of gene expression regulation in trypanosomatids, providing new ways to use molecular biological data in the control and treatment of the disease. Another approaches that may be helpful in obtaining new answers for the Leishmania gene expression questions could be performed through half-lives measurement (Archer et al., 2008) and polysome profiling (Bifeld et al., 2018; Karamysheva et al., 2018). This technique may provide important information on the correlation between mRNA and protein levels in Leishmania, by exclusively analyzing mRNAs that are in fact being translated.
LC, JA, and LF-W contributed to the conception of the review and manuscript revision. LC made the first draft, performed analysis, and designed figures and tables. JA and LF-W added new information to the original manuscript. All authors contributed to the article and approved the submitted version.
The work was supported by São Paulo Research Foundation (FAPESP) - grants #2017/23696-1 (LC), #2021/04422-3 (JA) and #2018/23512-0 (LF-W).
The authors declare that the research was conducted in the absence of any commercial or financial relationships that could be construed as a potential conflict of interest.
The handling editor LT declared a shared affiliation with the authors at the time of review.
All claims expressed in this article are solely those of the authors and do not necessarily represent those of their affiliated organizations, or those of the publisher, the editors and the reviewers. Any product that may be evaluated in this article, or claim that may be made by its manufacturer, is not guaranteed or endorsed by the publisher.
Agabian, N. (1990). Trans Splicing of Nuclear Pre-mRNAs. Cell 61, 1157–1160. doi: 10.1016/0092-8674(90)90674-4
Alcolea, P. J., Alonso, A., Domínguez, M., Parro, V., Jiménez, M., Molina, R., et al. (2016). Influence of the Microenvironment in the Transcriptome of Leishmania Infantum Promastigotes: Sand Fly Versus Culture. PloS Neglect. Trop. Dis. 10:1–23. doi: 10.1371/journal.pntd.0004693
Alcolea, P. J., Alonso, A., Gómez, M. J., Moreno, I., Domínguez, M., Parro, V., et al. (2010a). Transcriptomics Throughout the Life Cycle of Leishmania Infantum: High Down-Regulation Rate in the Amastigote Stage. Int. J. Parasitol. 40, 1497–1516. doi: 10.1016/j.ijpara.2010.05.013
Alcolea, P. J., Alonso, A., Gómez, M. J., Sánchez-Gorostiaga, A., Moreno-Paz, M., González-Pastor, E., et al. (2010b). Temperature Increase Prevails Over Acidification in Gene Expression Modulation of Amastigote Differentiation in Leishmania Infantum. BMC Genomics 11:1–24. doi: 10.1186/1471-2164-11-31
Alcolea, P. J., Alonso, A., Molina, R., Jiménez, M., Myler, P. J., Larraga, V. (2019). Functional Genomics in Sand Fly–Derived Leishmania Promastigotes. PloS Neglect. Trop. Dis. 13:1–30. doi: 10.1371/journal.pntd.0007288
Almeida, R., Gilmartin, B. J., McCann, S. H., Norrish, A., Ivens, A. C., Lawson, D., et al. (2004). Expression Profiling of the Leishmania Life Cycle: cDNA Arrays Identify Developmentally Regulated Genes Present But Not Annotated in the Genome. Mol. Biochem. Parasitol. 136, 87–100. doi: 10.1016/j.molbiopara.2004.03.004
Amiri-Dashatan, N., Rezaei-Tavirani, M., Ahmadi, N. (2020a). A Quantitative Proteomic and Bioinformatics Analysis of Proteins in Metacyclogenesis of Leishmania Tropica. Acta Tropica. 202, 105227. doi: 10.1016/j.actatropica.2019.105227
Amiri-Dashatan, N., Rezaei-Tavirani, M., Zali, H., Koushki, M., Ahmadi, N. (2020b). Quantitative Proteomic Analysis Reveals Differentially Expressed Proteins in Leishmania Major Metacyclogenesis. Microb. Pathogen. 149, 104557. doi: 10.1016/j.micpath.2020.104557
Andrade, B. B., De Oliveira, C. I., Brodskyn, C. I., Barral, A., Barral-Netto, M. (2007). Role of Sand Fly Saliva in Human and Experimental Leishmaniasis: Current Insights. Scand. J. Immunol. 66, 122–127. doi: 10.1111/J.1365-3083.2007.01964.X
Aoki, J. I., Muxel, S. M., Zampieri, R. A., Laranjeira-Silva, M. F., Müller, K. E., Nerland, A. H., et al. (2017). RNA-Seq Transcriptional Profiling of Leishmania Amazonensis Reveals an Arginase-Dependent Gene Expression Regulation. PloS Neglected. Trop. Dis. 11:1–22. doi: 10.1371/journal.pntd.0006026
Archer, S., Queiroz, R., Stewart, M., Clayton, C. (2008). Trypanosomes as a Model to Investigate mRNA Decay Pathways. Methods Enzymol. 448, 359–377. doi: 10.1016/S0076-6879(08)02618-9
Aslam, B., Basit, M., Nisar, M. A., Khurshid, M., Rasool, M. H. (2017). Proteomics: Technologies and Their Applications. J. Chromatogr. Sci. 55, 182–196. doi: 10.1093/chromsci/bmw167
Azizi, H., Papadopoulou, B. (2020). In Vivo Tethering System to Isolate RNA-Binding Proteins Regulating mRNA Decay in Leishmania. Methods Mol. Biol. 2116, 325–338. doi: 10.1007/978-1-0716-0294-2_20
Barak, E., Amin-Spector, S., Gerliak, E., Goyard, S., Holland, N., Zilberstein, D. (2005). Differentiation of Leishmania Donovani in Host-Free System: Analysis of Signal Perception and Response. Mol. Biochem. Parasitol. 141, 99–108. doi: 10.1016/j.molbiopara.2005.02.004
Baron, N., Tupperwar, N., Davidov, G., Zarivach, R., Shapira, M. (2020). Distinct Features of the Leishmania Cap-Binding Protein LeishIF4E2 Revealed by CRISPR-Cas9 Mediated Heterozygous Deletion. bioRxiv 15, e0008352. doi: 10.1101/2020.05.13.093914
Bates, P. A. (2008). Leishmania Sand Fly Interaction: Progress and Challenges. Curr. Opin. Microbiol. 11, 340–344. doi: 10.1016/j.mib.2008.06.003
Bates, P. A. (2018). Revising Leishmania’s Life Cycle. Nat. Microbiol. 3, 529–530, 53. doi: 10.1038/s41564-018-0154-2
Bates, P. A., Tetley, L. (1993). Leishmania Mexicana: Induction of Metacyclogenesis by Cultivation of Promastigotes at Acidic pH. Exp. Parasitol. 76, 412–423. doi: 10.1006/expr.1993.1050
Bifeld, E., Lorenzen, S., Bartsch, K., Vasquez, J.-J., Siegel, T. N., Clos, J. (2018). Ribosome Profiling Reveals HSP90 Inhibitor Effects on Stage-Specific Protein Synthesis in Leishmania Donovani. mSystems 3:1–18. doi: 10.1128/msystems.00214-18
Brotherton, M. C., Racine, G., Foucher, A. L., Drummelsmith, J., Papadopoulou, B., Ouellette, M. (2010). Analysis of Stage-Specific Expression of Basic Proteins in Leishmania Infantum. J. Proteome Res. 9, 3842–3853. doi: 10.1021/pr100048m
Buccitelli, C., Selbach, M. (2020). mRNAs, Proteins and the Emerging Principles of Gene Expression Control. Nat. Rev. Genet. 21, 630–644. doi: 10.1038/s41576-020-0258-4
Bühlmann, M., Walrad, P., Rico, E., Ivens, A., Capewell, P., Naguleswaran, A., et al. (2015). NMD3 Regulates Both mRNA and rRNA Nuclear Export in African Trypanosomes via an XPOI-Linked Pathway. Nucleic Acids Res. 43, 4491–4504. doi: 10.1093/nar/gkv330
Burza, S., Croft, S. L., Boelaert, M. (2018). Leishmaniasis. Lancet 392, 951–970. doi: 10.1016/S0140-6736(18)31204-2
Castro, F. F., Ruy, P. C., Zeviani, K. N., Santos, R. F., Toledo, J. S., Cruz, A. K. (2017). Evidence of Putative Non-Coding RNAs From Leishmania Untranslated Regions. Mol. Biochem. parasitol. 214, 69–74. doi: 10.1016/j.molbiopara.2017.04.002
Clayton, C. (2016). Gene Expression in Kinetoplastids. Curr. Opin. Microbiol. 32, 46–51. doi: 10.1016/j.mib.2016.04.018
Clayton, C. (2019). Regulation of Gene Expression in Trypanosomatids: Living With Polycistronic Transcription. Open Biol. 9:1–24. doi: 10.1098/rsob.190072
Clayton, C., Shapira, M. (2007). Post-Transcriptional Regulation of Gene Expression in Trypanosomes and Leishmanias. Mol. Biochem. Parasitol. 156, 93–101. doi: 10.1016/j.molbiopara.2007.07.007
Cohen-Freue, G., Holzer, T. R., Forney, J. D., McMaster, W. R. (2007). Global Gene Expression in Leishmania. Int. J. Parasitol. 37, 1077–1086. doi: 10.1016/j.ijpara.2007.04.011
Costa, T. L., Ribeiro-Dias, F., Oliveira, M. A. P., Bezerra, J. C. B., Vinaud, M. C. (2011). Energetic Metabolism of Axenic Promastigotes of Leishmania (Viannia) Braziliensis. Exp. Parasitol. 128, 438–443. doi: 10.1016/j.exppara.2011.05.018
Coutinho-Abreu, I. V., Serafim, T. D., Meneses, C., Kamhawi, S., Oliveira, F., Valenzuela, J. G. (2020). Distinct Gene Expression Patterns in Vector-Residing Leishmania Infantum Identify Parasite Stage-Enriched Markers. PloS Neglect. Trop. Dis. 14:1–23. doi: 10.1371/journal.pntd.0008014
Cramer, P. (2019). Organization and Regulation of Gene Transcription. Nature 573, 45–54. doi: 10.1038/s41586-019-1517-4
De Pablos, L. M., Ferreira, T. R., Walrad, P. B. (2016). Developmental Differentiation in Leishmania Lifecycle Progression: Post-Transcriptional Control Conducts the Orchestra. Curr. Opin. Microbiol. 34, 82–89. doi: 10.1016/j.mib.2016.08.004
De Sousa Abreu, R., Penalva, L. O., Marcotte, E. M., Vogel, C. (2009). Global Signatures of Protein and mRNA Expression Levels. Mol. Biosyst. 5, 1512–1526. doi: 10.1039/b908315d
Dillon, L. A. L., Okrah, K., Hughitt, K. V., Suresh, R., Li, Y., Fernandes, M. C., et al. (2015). Transcriptomic Profiling of Gene Expression and RNA Processing During Leishmania Major Differentiation. Nucleic Acids Res. 43, 6799–6813. doi: 10.1093/nar/gkv656
Dostálová, A., Volf, P. (2012). Leishmania Development in Sand Flies: Parasite-Vector Interactions Overview. Parasit. Vectors 5, 1–12, 1 5. doi: 10.1186/1756-3305-5-276
Dumas, C., Chow, C., Müller, M., Papadopoulou, B. (2006). A Novel Class of Developmentally Regulated Noncoding RNAs in Leishmania. Eukaryotic Cell 5, 2033–2046. doi: 10.1128/ec.00147-06
Fadda, A., Ryten, M., Droll, D., Rojas, F., Färber, V., Haanstra, J. R., et al. (2014). Transcriptome-Wide Analysis of Trypanosome mRNA Decay Reveals Complex Degradation Kinetics and Suggests a Role for Co-Transcriptional Degradation in Determining mRNA Levels. Mol. Microbiol. 94, 307–326. doi: 10.1111/mmi.12764
Fernandes, J. C. R., Acuña, S. M., Aoki, J. I., Floeter-Winter, L. M., Muxel, S. M. (2019). Long Non-Coding RNAs in the Regulation of Gene Expression: Physiology and Disease. Non-coding. RNA 5:1–25. doi: 10.3390/ncrna5010017
Ferreira, T. R., Alves-Ferreira, E. V. C., Defina, T. P. A., Walrad, P., Papadopoulou, B., Cruz, A. K. (2014). Altered Expression of an RBP-Associated Arginine Methyltransferase 7 in Leishmania Major Affects Parasite Infection. Mol. Microbiol. 94, 1085–1102. doi: 10.1111/mmi.12819
Ferreira, T. R., Dowle, A. A., Parry, E., Alves-Ferreira, E. V. C., Hogg, K., Kolokousi, F., et al. (2020). PRMT7 Regulates RNA-Binding Capacity and Protein Stability in Leishmania Parasites. Nucleic Acids Res. 48, 5511–5526. doi: 10.1093/nar/gkaa211
Gardener, P. J., Howells, R. E., Moleneux, H. (1973). Ultrastructure of Leishmania Amastigotes. Trans. R. Soc. Trop. Med. Hyg. 67, 23–23. doi: 10.1016/0035-9203(73)90287-3
Greenbaum, D., Colangelo, C., Williams, K., Gerstein, M. (2003). Comparing Protein Abundance and mRNA Expression Levels on a Genomic Scale. Genome Biol. 4, 117. doi: 10.1186/gb-2003-4-9-117
Gupta, N., Goyal, N., Rastogi, A. K. (2001). In Vitro Cultivation and Characterization of Axenic Amastigotes of Leishmania. Trends Parasitol. 17(3), 150–153. doi: 10.1016/S1471-4922(00)01811-0
Haile, S., Papadopoulou, B. (2007). Developmental Regulation of Gene Expression in Trypanosomatid Parasitic Protozoa. Curr. Opin. Microbiol. 10, 569–577. doi: 10.1016/J.MIB.2007.10.001
Hart, D. T., Coombs, G. H. (1982). Leishmania Mexicana: Energy Metabolism of Amastigotes and Promastigotes. Exp. Parasitol. 54, 397–409. doi: 10.1016/0014-4894(82)90049-2
Hentzer, B., Kobayasi, T. (1977). The Ultrastructure of Leishmania Tropica in Skin Lesions. Acta Patholog. Microbiol. Scand. Section. B Microbiol. 85, 153–160. doi: 10.1111/J.1699-0463.1977.TB01689.X
Holzer, T. R., McMaster, W. R., Forney, J. D. (2006). Expression Profiling by Whole-Genome Interspecies Microarray Hybridization Reveals Differential Gene Expression in Procyclic Promastigotes, Lesion-Derived Amastigotes, and Axenic Amastigotes in Leishmania Mexicana. Mol. Biochem. Parasitol. 146, 198–218. doi: 10.1016/j.molbiopara.2005.12.009
Hombach, S., Kretz, M. (2016). Non-Coding RNAs: Classification, Biology and Functioning. Adv. Exp. Med. Biol. 937, 3–17. doi: 10.1007/978-3-319-42059-2_1
Howells, R. E., Gardener, P. J. (1972). Preliminary Electron Microscope Observations on the Amastigote Forms of Trypanosoma Cruzi and Leishmania Donovani. Trans. R. Soc. Trop. Med. Hyg. 66, 336. doi: 10.1016/0035-9203(72)90214-3
Hung, J.-H., Weng, Z. (2017). Analysis of Microarray and RNA-Seq Expression Profiling Data. Cold Spring Harbor Protoc. 2017, 191–196. doi: 10.1101/pdb.top093104
Inbar, E., Hughitt, V. K., Dillon, L. A. L., Ghosh, K., El-Sayed, N. M., Sacks, D. L. (2017). The Transcriptome of Leishmania Major Developmental Stages in Their Natural Sand Fly Vector. mBio 8:1–18. doi: 10.1128/mBio.00029-17
Ivens, A. C., Peacock, C. S., Worthey, E. A., Murphy, L., Aggarwal, G., Berriman, M., et al. (2005). The Genome of the Kinetoplastid Parasite, Leishmania Major. Science 309, 436–442. doi: 10.1126/science.1112680
Jansen, R., Greenbaum, D., Gerstein, M. (2002). Relating Whole-Genome Expression Data With Protein-Protein Interactions. Genome Res. 12, 37–46. doi: 10.1101/gr.205602
Kamhawi, S. (2006). Phlebotomine Sand Flies and Leishmania Parasites: Friends or Foes? Trends Parasitol. 22, 439–445. doi: 10.1016/j.pt.2006.06.012
Karamysheva, Z. N., Tikhonova, E. B., Grozdanov, P. N., Huffman, J. C., Baca, K. R., Karamyshev, A., et al. (2018). Polysome Profiling in Leishmania, Human Cells and Mouse Testis. J. Visualized. Experiments : JoVE 34:1–12.. doi: 10.3791/57600
Katz, O., Waitumbi, J. N., Zer, R., Warburg, A. (2000). Adenosine, AMP, and Protein Phosphatase Activity in Sandfly Saliva. Am. J. Trop. Med. Hyg. 62, 145–150. doi: 10.4269/AJTMH.2000.62.145
Kim, K., Zakharkin, S. O., Allison, D. B. (2010). Expectations, Validity, and Reality in Gene Expression Profiling. J. Clin. Epidemiol. 63, 950–959. doi: 10.1016/J.JCLINEPI.2010.02.018
Kloehn, J., Saunders, E. C., O’Callaghan, S., Dagley, M. J., McConville, M. J. (2015). Characterization of Metabolically Quiescent Leishmania Parasites in Murine Lesions Using Heavy Water Labeling. PloS Pathog. 11:1–19. doi: 10.1371/journal.ppat.1004683
Kramer, S., Queiroz, R., Ellis, L., Webb, H., Hoheisel, J. D., Clayton, C., et al. (2008). Heat Shock Causes a Decrease in Polysomes and the Appearance of Stress Granules in Trypanosomes Independently of Eif2α Phosphorylation at Thr169. J. Cell Sci. 121, 3002–3014. doi: 10.1242/jcs.031823
Krassner, S. M. (1969). Proline Metabolism in Leishmania Tarentolae. Exp. Parasitol. 24, 348–363. doi: 10.1016/0014-4894(69)90173-8
Krassner, S. M., Flory, B. (1972). Proline Metabolism in Leishmania Donovani Promastigotes. J. Protozool. 19, 682–685. doi: 10.1111/j.1550-7408.1972.tb03560.x
Kuile, B. H. T., Opperdoes, F. R. (1992). A Chemostat Study on Proline Uptake and Metabolism of Leishmania Donovani. J. Protozool. 39, 555–558. doi: 10.1111/j.1550-7408.1992.tb04850.x
Lahav, T., Sivam, D., Volpin, H., Ronen, M., Tsigankov, P., Green, A., et al. (2011). Multiple Levels of Gene Regulation Mediate Differentiation of the Intracellular Pathogen Leishmania. FASEB J. 25, 515–525. doi: 10.1096/fj.10-157529
Leifso, K., Cohen-Freue, G., Dogra, N., Murray, A., McMaster, W. R. (2007). Genomic and Proteomic Expression Analysis of Leishmania Promastigote and Amastigote Life Stages: The Leishmania Genome Is Constitutively Expressed. Mol. Biochem. Parasitol. 152, 35–46. doi: 10.1016/j.molbiopara.2006.11.009
Liang, X. H., Haritan, A., Uliel, S., Michaeli, S. (2003). Trans and Cis Splicing in Trypanosomatids: Mechanism, Factors, and Regulation. Eukaryotic Cell 2, 830–840. doi: 10.1128/EC.2.5.830-840.2003
Liu, C. C., Jewett, M. C., Chin, J. W., Voigt, C. A. (2018). Toward an Orthogonal Central Dogma. Nat. Chem. Biol. 14, 103. doi: 10.1038/NCHEMBIO.2554
Li, G.-W., Xie, X. S. (2011). Central Dogma at the Single-Molecule Level in Living Cells. Nature 475, 308. doi: 10.1038/nature10315
Maniatis, T., Reed, R. (2002). An Extensive Network of Coupling Among Gene Expression Machines. Nat. 2002 416:6880 416, 499–506. doi: 10.1038/416499a
Martínez-Calvillo, S., Nguyen, D., Stuart, K., Myler, P. J. (2004). Transcription Initiation and Termination on Leishmania Major Chromosome 3. Eukaryotic Cell 3, 506–517. doi: 10.1128/EC.3.2.506-517.2004
Mattick, J. S. (2001). Non-Coding RNAs: The Architects of Eukaryotic Complexity. EMBO Rep. 2, 986–991. doi: 10.1093/embo-reports/kve230
McConville, M. J., Turco, S. J., Ferguson, M. A. J., Sacks, D. L. (1992). Developmental Modification of Lipophosphoglycan During the Differentiation of Leishmania Major Promastigotes to an Infectious Stage. EMBO J. 11, 3593–3600. doi: 10.1002/j.1460-2075.1992.tb05443.x
McNicoll, F., Drummelsmith, J., Müller, M., Madore, É., Boilard, N., Ouellette, M., et al. (2006). A Combined Proteomic and Transcriptomic Approach to the Study of Stage Differentiation in Leishmania Infantum. Proteomics 6, 3567–3581. doi: 10.1002/pmic.200500853
Michaeli, S. (2011). Trans-Splicing in Trypanosomes: Machinery and Its Impact on the Parasite Transcriptome. Future Microbiol. 6, 459–474. doi: 10.2217/fmb.11.20
Michaeli, S., Agami, R., Shapira, M. (1993). Leishmania Mexicana Amazonensis: Effect of Heat Shock on the Spliced Leader RNA and Its Ribonucleoprotein Particle SL RNP. Exp. Parasitol. 76, 59–67. doi: 10.1006/expr.1993.1007
Mishra, K. K., Holzer, T. R., Moore, L. L., LeBowitz, J. H. (2003). A Negative Regulatory Element Controls mRNA Abundance of the Leishmania Mexicana Paraflagellar Rod Gene PFR2. Eukaryotic Cell 2, 1009–1017. doi: 10.1128/EC.2.5.1009-1017.2003
Mojtahedi, Z., Clos, J., Kamali-Sarvestani, E. (2008). Leishmania Major: Identification of Developmentally Regulated Proteins in Procyclic and Metacyclic Promastigotes. Exp. Parasitol. 119, 422–429. doi: 10.1016/j.exppara.2008.04.008
Moore, L. L., Santrich, C., LeBowitz, J. H. (1996). Stage-Specific Expression of the Leishmania Mexicana Paraflagellar Rod Protein PFR-2. Mol. Biochem. Parasitol. 80, 125–135. doi: 10.1016/0166-6851(96)02688-6
Mor, I., Cheung, E. C., Vousden, K. H. (2011). Control of Glycolysis Through Regulation of PFK1: Old Friends and Recent Additions. Cold Spring Harbor Symp. Quant. Biol. 76, 211–216. doi: 10.1101/sqb.2011.76.010868
Myler, P. J., Audleman, L., Devos, T., Hixson, G., Kiser, P., Lemley, C., et al. (1999). Leishmania Major Friedlin Chromosome 1 has an Unusual Distribution of Protein-Coding Genes. Proc. Natl. Acad. Sci. U.S.A. 96, 2902–2906. doi: 10.1073/PNAS.96.6.2902
Nandan, D., Thomas, S. A., Nguyen, A., Moon, K.-M., Foster, L. J., Reiner, N. E. (2017). Comprehensive Identification of mRNA-Binding Proteins of Leishmania Donovani by Interactome Capture. PloS One 12, e0170068. doi: 10.1371/journal.pone.0170068
Názer, E., Sánchez, D. O. (2011). Nucleolar Accumulation of RNA Binding Proteins Induced by ActinomycinD Is Functional in Trypanosoma Cruzi and Leishmania Mexicana But Not in T. Brucei. PloS One 6, e24184. doi: 10.1371/journal.pone.0024184
Novozhilova, N. M., Bovin, N. V. (2010). Structure, Functions, and Biosynthesis of Glycoconjugates of Leishmania Spp. Cell Surface. Biochem. (Moscow). 75, 686–694. doi: 10.1134/S0006297910060027
Opperdoes, F. R., Coombs, G. H. (2007). Metabolism of Leishmania: Proven and Predicted. Trends Parasitol. 23, 149–158. doi: 10.1016/j.pt.2007.02.004
Pimenta, P. F. P., Turco, S. J., McConville, M. J., Lawyer, P. G., Perkins, P. V., Sacks, D. L. (1992). Stage-Specific Adhesion of Leishmania Promastigotes to the Sandfly Midgut. Science 256, 1812–1815. doi: 10.1126/science.1615326
Portman, N., Gull, K. (2010). The Paraflagellar Rod of Kinetoplastid Parasites: From Structure to Components and Function. Int. J. Parasitol. 40, 135–148. doi: 10.1016/j.ijpara.2009.10.005
Preußer, C., Jaé, N., Bindereif, A. (2012). MRNA Splicing in Trypanosomes. Int. J. Med. Microbiol. 302, 221–224. doi: 10.1016/j.ijmm.2012.07.004
Rastrojo, A., Corvo, L., Lombraña, R., Solana, J. C., Aguado, B., Requena, J. M. (2019). Analysis by RNA-Seq of Transcriptomic Changes Elicited by Heat Shock in Leishmania Major. Sci. Rep. 9:1–18. doi: 10.1038/s41598-019-43354-9
Rochette, A., Raymond, F., Corbeil, J., Ouellette, M., Papadopoulou, B. (2009). Whole-Genome Comparative RNA Expression Profiling of Axenic and Intracellular Amastigote Forms of Leishmania Infantum. Mol. Biochem. Parasitol. 165, 32–47. doi: 10.1016/j.molbiopara.2008.12.012
Rowton, E., Pimenta, P. F. P., Brodin, T. N., Modi, G. B., Sacks, D. L. (1995). Changes in Lipophosphoglycan and Gene Expression Associated With the Development of Leishmania Major in Phlebotomus Papatasi. Parasitology 111, 275–287. doi: 10.1017/S003118200008183X
Ruy, P. D. C., Monteiro-Teles, N. M., Magalhães, R. D. M., Freitas-Castro, F., Dias, L., Defina, T. P. A., et al. (2019). Comparative Transcriptomics in Leishmania Braziliensis: Disclosing Differential Gene Expression of Coding and Putative Noncoding RNAs Across Developmental Stages. RNA Biol. 16, 639–660. doi: 10.1080/15476286.2019.1574161
Saar, Y., Ransford, A., Waldman, E., Mazareb, S., Amin-Spector, S., Plumblee, J., et al. (1998). Characterization of Developmentally-Regulated Activities in Axenic Amastigotes of Leishmania Donovani. Mol. Biochem. Parasitol. 95, 9–20. doi: 10.1016/S0166-6851(98)00062-0
Sacks, D., Kamhawi, S. (2001). Molecular Aspects of Parasite-Vector and Vector-Host Interactions in Leishmaniasis. Annu. Rev. Microbiol. 55, 453–483. doi: 10.1146/annurev.micro.55.1.453
Sacks, D. L., Perkins, P. V. (1984). Identification of an Infective Stage of Leishmania Promastigotes. Science 223, 1417–1419. doi: 10.1126/science.6701528
Saxena, A., Lahav, T., Holland, N., Aggarwal, G., Anupama, A., Huang, Y., et al. (2007). Analysis of the Leishmania Donovani Transcriptome Reveals an Ordered Progression of Transient and Permanent Changes in Gene Expression During Differentiation. Mol. Biochem. Parasitol. 152, 53–65. doi: 10.1016/j.molbiopara.2006.11.011
Schneider-Poetsch, T., Yoshida, M. (2018). Along the Central Dogma—Controlling Gene Expression With Small Molecules. Annual Rev. of Biochem. 87, 391–420. doi: 10.1146/annurev-biochem-060614-033923
Segundo-Val, I. S., Sanz-Lozano, C. S. (2016). Introduction to the Gene Expression Analysis. Methods. Mol Biol. 1434, 29–43. doi: 10.1007/978-1-4939-3652-6_3
Serafim, T. D., Coutinho-Abreu, I. V., Oliveira, F., Meneses, C., Kamhawi, S., Valenzuela, J. G. (2018). Sequential Blood Meals Promote Leishmania Replication and Reverse Metacyclogenesis Augmenting Vector Infectivity. Nat. Microbiol. 3, 548–555, 5 3. doi: 10.1038/s41564-018-0125-7
Serafim, T. D., Figueiredo, A. B., Costa, P. A. C., Marques-da-Silva, E. A., Gonçalves, R., de Moura, S. A. L., et al. (2012). Leishmania Metacyclogenesis Is Promoted in the Absence of Purines. PloS Neglect. Trop. Dis. 6, e1833. doi: 10.1371/journal.pntd.0001833
Shapira, M., McEwen, J. G., Jaffe, C. L. (1988). Temperature Effects on Molecular Processes Which Lead to Stage Differentiation in Leishmania. EMBO J. 7, 2895. doi: 10.1002/j.1460-2075.1988.tb03147.x
Shrivastava, R., Tupperwar, N., Schwartz, B., Baron, N., Shapira, M. (2021). Leishif4e-5 is a Promastigote-Specific Cap-Binding Protein in Leishmania. Int. J. Mol. Sci. 22, 22. doi: 10.3390/ijms22083979
Stiles, J. K., Hicock, P. I., Shah, P. H., Meade, J. C. (2016). Genomic Organization, Transcription, Splicing and Gene Regulation in Leishmania. Annals of Tropical Med. Parasitol. 93, 781–807. doi: 10.1080/00034983.1999.11813485
Terrão, M. C., de Vasconcelos, E. J. R., Defina, T. A., Myler, P. J., Cruz, A. K. (2017). Disclosing 3’ UTR Cis-Elements and Putative Partners Involved in Gene Expression Regulation in Leishmania Spp. PloS One 12:1–18. doi: 10.1371/journal.pone.0183401
Torres, F., Arias-Carrasco, R., Caris-Maldonado, J. C., Barral, A., Maracaja-Coutinho, V., De Queiroz, A. T. L. (2017). LeishDB: A Database of Coding Gene Annotation and Non-Coding RNAs in Leishmania Braziliensis. Database 2017, 1–11. doi: 10.1093/database/bax047
Tupperwar, N., Shrivastava, R., Shapira, M. (2019). LeishIF4E1 Deletion Affects the Promastigote Proteome, Morphology, and Infectivity. mSphere 4:1–20. doi: 10.1128/msphere.00625-19
Walker, J., Vasquez, J.-J., Gomez, M. A., Drummelsmith, J., Burchmore, R., Girard, I., et al. (2006). Identification of Developmentally-Regulated Proteins in Leishmania Panamensis by Proteome Profiling of Promastigotes and Axenic Amastigotes. Mol. Biochem. Parasitol. 147, 64–73. doi: 10.1016/j.molbiopara.2006.01.008
Webb, J. R., Campos-Neto, A., Skeiky, Y. A. W., Reed, S. G. (1997). Molecular Characterization of the Heat-Inducible LmSTI1 Protein of Leishmania Major. Mol. Biochem. Parasitol. 89, 179–193. doi: 10.1016/S0166-6851(97)00115-1
Wilson, R., Bates, M. D., Dostalova, A., Jecna, L., Dillon, R. J., Volf, P., et al. (2010). Stage-Specific Adhesion of Leishmania Promastigotes to Sand Fly Midguts Assessed Using an Improved Comparative Binding Assay. PloS Neglect. Trop. Dis. 4:1–9. doi: 10.1371/journal.pntd.0000816
Zhang, Z., Wu, S., Stenoien, D. L., Paša-Tolić, L. (2014). High-Throughput Proteomics. Annu. Rev. Anal. Chem. (Palo. Alto. Calif.) 7, 427–454. doi: 10.1146/annurev-anchem-071213-020216
Zilberstein, D. (2020). “In Vitro Culture for Differentiation Simulation of Leishmania Spp,” in Trypanosomatids: Methods and Protocols. Eds. Michels, P. A. M., Ginger, M. L., Zilberstein, D. (New York, NY: Springer US), 39–47. doi: 10.1007/978-1-0716-0294-2_3
Keywords: metacyclogenesis, amastigote differentiation, life cycle, gene expression, transcriptome, proteome
Citation: Cortazzo da Silva L, Aoki JI and Floeter-Winter LM (2022) Finding Correlations Between mRNA and Protein Levels in Leishmania Development: Is There a Discrepancy? Front. Cell. Infect. Microbiol. 12:852902. doi: 10.3389/fcimb.2022.852902
Received: 12 January 2022; Accepted: 02 May 2022;
Published: 12 July 2022.
Edited by:
Luiz Tosi, University of São Paulo, BrazilReviewed by:
Jose M. Requena, Autonomous University of Madrid, SpainCopyright © 2022 Cortazzo da Silva, Aoki and Floeter-Winter. This is an open-access article distributed under the terms of the Creative Commons Attribution License (CC BY). The use, distribution or reproduction in other forums is permitted, provided the original author(s) and the copyright owner(s) are credited and that the original publication in this journal is cited, in accordance with accepted academic practice. No use, distribution or reproduction is permitted which does not comply with these terms.
*Correspondence: Lucile Maria Floeter-Winter, bHVjaWxlQGliLnVzcC5icg==
Disclaimer: All claims expressed in this article are solely those of the authors and do not necessarily represent those of their affiliated organizations, or those of the publisher, the editors and the reviewers. Any product that may be evaluated in this article or claim that may be made by its manufacturer is not guaranteed or endorsed by the publisher.
Research integrity at Frontiers
Learn more about the work of our research integrity team to safeguard the quality of each article we publish.