- 1Blue Genomics SpA, Puerto Varas, Chile
- 2Laboratorio de Diagnóstico y Biotecnología, R & D Department, ADL Diagnostic Chile, Puerto Montt, Chile
Bacterial cell envelopes play a critical role in host-pathogen interactions. Macromolecular components of these structures have been closely linked to the virulence of pathogens. Piscirickettsia salmonis is a relevant salmonid pathogen with a worldwide distribution. This bacterium is the etiological agent of piscirickettsiosis, a septicemic disease that causes a high economic burden, especially for the Chilean salmon farming industry. Although P. salmonis has been discovered long ago, its pathogenicity and virulence mechanisms are not completely understood. In this work, we present a genetic approach for producing in-frame deletion mutants on genes related to the biosynthesis of membrane-associated polysaccharides. We provide a detailed in vitro phenotype description of knock-out mutants on wzx and wcaJ genes, which encode predicted lipopolysaccharide (LPS) flippase and undecaprenyl-phosphate glucose phosphotransferase enzymes, respectively. We exhibit evidence that the wzx mutant strain carries a defect in the probably most external LPS moiety, while the wcaJ mutant proved to be highly susceptible to the bactericidal action of serum but retained the ability of biofilm production. Beyond that, we demonstrate that the deletion of wzx, but not wcaJ, impairs the virulence of P. salmonis in an intraperitoneally infected Atlantic salmon, Salmo salar, model of piscirickettsiosis. Our findings support a role for LPS in the virulence of P. salmonis during the onset of piscirickettsiosis.
Introduction
Piscirickettsia salmonis is a Gram-negative bacterium of the class Gammaproteobacteria and an intracellular facultative microorganism that causes piscirickettsiosis, a deadly and highly contagious disease of reared salmonids (Fryer and Hedrick, 2003). Although the pathogen is globally disseminated, virulent strains are particularly prevalent in the south of Chile where frequent outbreaks occur that often need to be controlled by antimicrobial therapy (Rozas and Enriquez, 2014). Piscirickettsiosis is also a big concern for salmon producers from an economic point of view: the annual costs of mortality, vaccines and antibiotic treatments may add up to 700 million dollars, according to the Technological Institute of Salmon, Intesal (https://www.intesal.cl/). Due to its environmental dimension, the required amount of antibiotics is also a regulatory and trading issue. Therefore, addressing this sanitary threat is a priority for several stakeholders in the Chilean industry.
In recent years, it has become evident that P. salmonis shares some features found in other, better-known pathogens. In the context of its genetic relationship to Legionella, a functional effector export machinery, including type 4 secretion systems or T4SS, was found to be expressed in vitro and required to survive in vivo (Gomez et al., 2013; Mancilla et al., 2018). In this regard, adhesins such as T4SS pilin have been demonstrated to occur in vitro (Sánchez et al., 2018). Flagellar proteins have been detected within P. salmonis cells, but motility has not been described (Carril et al., 2016). P. salmonis seems to require iron, since the bacterium expresses siderophores, and salmonids respond to the infection using iron-deprivation as a defense mechanism (Pulgar et al., 2015; Almarza et al., 2016; Calquin et al., 2017). More recently, gene expression studies have accounted for the expression of putative virulence factors carried by cryptic plasmids, as well as several chromosomal and plasmid-borne antibiotic resistance markers (Sandoval et al., 2016; Saavedra et al., 2018; Ortiz-Severin et al., 2020). Altogether, it becomes clear that the intracellular phase of the P. salmonis life cycle requires adaptive traits for survival within macrophages. Bactericidal mechanisms, for instance, maybe overcome by interference with intracellular vesicle traffic (Perez-Stuardo et al., 2019; Zuniga et al., 2020). The ability of biofilm formation has also been explored, and the transition from planktonic growth to cells embedded in an exopolysaccharide (EPS) matrix has been postulated as an adaptive life form needed for survival under stress conditions in the marine environment (Marshall et al., 2012; Albornoz et al., 2017).
Gram-negative bacteria share a common cell envelope structure, with a peptidoglycan polymer interspaced between a double lipid bilayer. The outer membrane is mainly composed of an amphipathic chemical species called lipopolysaccharide (LPS), a complex macromolecule containing three canonical moieties: lipid A is anchored to the membrane and connects to the outermost motif known as O-antigen or O-chain via the core oligosaccharide (Samuel and Reeves, 2003). The bacterial membrane can also be covered by a matrix of polysaccharide nature derived from the export of EPS. If this polysaccharide remains attached to the surface, it is referred to as capsule (Reid and Szymanski, 2010). Otherwise, this polymer may also be enzymatically released and contribute to biofilm formation (Donot et al., 2012). In bacterial pathogens, it is well established that both polysaccharides LPS and EPS are critical for the maintenance of cell integrity, adhesion, bacteriophage receptors, resilience to host defense mechanisms, recognition by the host immune system, and virulence (Moradali and Rehm, 2020). Attempts to gain insight into the structure and function of LPS and EPS have been reported (Vadovic et al., 2007; Marshall et al., 2012; Vinogradov et al., 2013), but the role of these biopolymers in the pathogenicity of P. salmonis remains unclear.
Since our understanding of the pathogen’s biology and virulence mechanisms will benefit the development of effective disease prevention and management strategies, the goal of this study was to shed light on the role of cell envelope polysaccharides in the virulence of P. salmonis. We provide genetic and functional evidence on the identification of a gene cluster encoding biosynthetic functions for both LPS and EPS within the genome of P. salmonis. To further support our hypothesis, we generated P. salmonis mutants defective in LPS or EPS using a genetic approach designed to overcome unwanted side effects and to facilitate the study of their virulent behavior in vitro and in vivo.
Material and Methods
Bacterial Strains, Plasmids, and Culture Conditions
Bacterial strains and plasmids used throughout this study are listed in Table 1. P. salmonis strains were cultivated in P. salmonis broth (PSB) or on its solid equivalent PSA at 18°C (Henriquez et al., 2016). E. coli strains were propagated in Luria-Bertani broth (LB, Difco) or on tryptic soy agar plates (TSA, Difco) at 37°C. Plasmids were introduced into E. coli strains by transformation and into P. salmonis strains through mating with the donor strain E. coli S17-1λpir (Simon et al., 1983). Where necessary, antibiotics (Sigma) were used at the following concentrations: kanamycin at 50 μg/ml and polymyxin B at 10 μg/ml. Any potentially infectious residue was inactivated by autoclaving before disposal according to the biosafety protocols of ADL Diagnostic Chile. Plasmids pCR2.1 TOPO (Invitrogen) and pJQK (Quandt and Hynes, 1993) were manipulated according to standard cloning procedures.
Sequence Analysis
The P. salmonis PM15972A1 genome sequence was retrieved from the GenBank database (accession n° CP012413). The annotation was examined with CLC Genomics Workbench software (Qiagen), looking for genes encoding functions related to the biosynthesis of precursors of LPS and EPS. Sequence alignments were accomplished with BLAST. For comparative purposes, sequences from identified gene clusters were compared with those annotated in the KEGG database (https://www.genome.jp/kegg).
Construction of In-Frame Deletion Mutant Strains
All primers used in this study are listed in Table 2. The sequences of the wzx and wcaJ genes of P. salmonis PM15972A1 were obtained from the NCBI (National Center for Biotechnology Information, http://www.ncbi.nlm.nih.gov/) (Bohle et al., 2014). P. salmonis wzx or wcaJ in-frame deletion mutants were engineered by overlapping PCR following a protocol previously described for Brucella (Conde-Alvarez et al., 2006). Briefly, two DNA fragments obtained with the primer pairs wzx_F1/wzx_R2 and wzx_F3/wzx_R4 were ligated by PCR extension, and the resulting fragment was cloned into pCR2.1-TOPO supported by the TOP10 E. coli strain (Figure S1). Subcloning into the pJQK plasmid was achieved by restriction digestion of the corresponding pCR2.1 TOPO derivative and transformation into the S17-1λpir E. coli donor strain. The resulting pJQK derivative was introduced into P. salmonis by conjugation. Transconjugants carrying the pJQwzx plasmid integrated into the chromosome by a single crossover event were then selected on PSA containing kanamycin and polymyxin B. Allelic exchange between the chromosomal gene and the mutagenized plasmid copy was achieved by a second crossover event and was counter-selected on PSA containing 5% sucrose (Figure S2). An identical procedure but different primers were used for the construction of the pJQwcaJ mutator plasmid and the corresponding P. salmonis ΔwcaJ mutant strain. The presence of the respective mutated genes in each strain was confirmed by the analysis of PCR amplicons generated with primers F1-R4. Mutated genes were also sequenced by Sanger chemistry to confirm the interchange with the wild-type allele in the resulting mutant clones. Bacterial strains were stored in glycerol stocks at -70°C.
Growth Kinetics, Bacterial Counts, and Self-Agglutination Tests
100 µL aliquots of deep-frozen P. salmonis wild-type and mutant strains were streaked onto PSA plates and incubated for 2 days at 18°C. By this time, a homogeneous bacterial lawn had formed and we proceeded to suspend a loopful of bacteria in 1 mL of buffered saline solution (BSS: 8.5 g/L NaCl, 2.0 g/L K2HPO4, 2.0 g/L KH2PO4, pH 6.4), transferred them onto new PSA plates, and incubated as mentioned above. Bacterial suspensions (OD600 = 1.0) were prepared by mixing bacteria with 5 mL of cold BSS in a conical tube kept at 10°C. For growth kinetics, 96-well microplates were inoculated with 150 µL/well of 1:100 bacterial suspensions in PSB and incubated during 70 h. Hourly reads were realized with an EPOCH2 spectrophotometer (Biotek, USA). By contrast, self-agglutination tests lasted 8 h, but used bacterial suspensions prepared in PSB that were held in plastic cuvettes at 18°C and pH 7.2. Reads were collected each hour with a densitometer (Ultrospec 10, GE LifeSciences). In order to determine bacterial counts, aliquots of log10 dilutions in PSB were seeded on PSA plates in triplicates and incubated at 18°C for one week. Acriflavine tests were carried out using fresh bacterial suspension in BSS mixed with identical volumes (10 µL) of 0.1% acriflavine on a glass slide.
Bactericidal Action of Salmo salar Naïve Serum
The complement killing activity of naïve serum was evaluated following a previously described protocol (Saavedra et al., 2017). Atlantic salmon (Salmo salar) smolts with an average body weight of 96 g were caudally punctured, and naïve serum was obtained after blood clotting for 2 h at 4°C and centrifugation at 2,000 × g for 5 min. Samples from 5 fish were pooled. Bacterial inoculum was set at ~107 colony forming units (cfu)/mL and was treated with undiluted fish serum for 3 h at 18°C. Heat-inactivated (44°C, 20 min) serum aliquots were used as negative controls.
LPS Purification and Analysis
LPS was extracted from P. salmonis wild-type and mutants following a previously described method (Yi and Hackett, 2000). 15 mL of a fresh culture with OD600 = 1.9 were harvested, which corresponded to roughly 10 mg of freeze-dried bacteria. LPS was isolated with a commercial RNA isolation reagent (Trizol) and subsequently resuspended in 200 µL of PBS. Discontinuous SDS-PAGE electrophoresis was run on 15% and 20% polyacrylamide stacking/solving gels. Gels were silver-stained according to a well-known protocol (Hitchcock and Brown, 1983). Alternatively, LPS was extracted without organic solvent following a protocol described before (Renzi et al., 2016). Briefly, 1 mL of bacterial suspension in PBS, adjusted to OD600 = 1.0, was pelleted by centrifugation and subsequently lysed with Laemmli buffer. Samples were boiled and treated twice with proteinase K (50 µg/mL final protease concentration, overnight at 37°C). Crude protein samples and LPS prepared following the recommendations by Renzi and colleagues were used for independent Western blot assays. These samples were eletrophoresed in 15% polyacrylamide gels. Nitrocellulose membranes were blotted according to a conventional protocol, using semi-dry transfer equipment (Bio-Rad) for 35 min at 20 V. These blots were incubated with polyclonal serum extracted from a rabbit that had been immunized with heat-inactivated P. salmonis PM15972A1 cells. Signals were then revealed with an HRP-conjugated secondary antibody and a suitable chemical substrate.
Biofilm Formation Assessment
Biofilm formation assays were performed following a previously described method (Pal et al., 2019), with few modifications. Briefly, the wells of a sterile 24-well polystyrene microtiter plate were inoculated with 1 mL of P. salmonis wild-type and mutant strain suspensions adjusted in PSB to obtain ~7.0 × 106 cfu/well. Sterile PBS was used as a negative control. Plates were incubated at 18°C for 30 days under static conditions. Then, wells were washed twice with BSS to remove the planktonic cells and stained with 0.1% crystal violet (CV) for 15 min. Excess CV was removed by renewed washing with BSS, and bound dye was solubilized in 1 mL of 33% acetic acid. Solubilized CV was subsequently transferred to a new microplate and its absorbance was spectrophotometrically determined at 620 nm. This same measurement was done on negative-control wells containing bacteria-free medium in order to account for non-specific and abiotic factors. Total biofilm formation was calculated by subtracting the average OD620 value of the stained control wells from the OD620 value of the stained biofilm (Naves et al., 2008). Calculated values were finally normalized to those obtained for the P. salmonis PM15972A1 positive control.
RNA Purification and Gene Expression Analysis
So as to evaluate possible differences in gene expression triggered by the treatment with P. salmonis wild-type and mutant strains, RNA extraction and cDNA synthesis were performed as described by Mancilla et al. (2018). RNA was purified using the EZNA Total RNA Kit I (Omega Bio-Tek, Norcross, GA, USA). After DNAse I digestion, purified RNA was stored at -70°C. Reverse transcription was performed using the M-MLV RT Kit (Promega, USA) and synthesized cDNA was stored at -20°C. Gene expression was analyzed by qPCR using the Maxima SYBR® Green qPCR Master Mix (Thermo Scientific) in a StepOne PCR machine (Applied Biosystems, Waltham, MA, USA), whereby each reaction was performed using 250 nM of primers (Table 2) and 1.0 µL of 1:2 diluted cDNA as a template. The thermal profile included an initial step of 10 min at 95°C, followed by 40 cycles of 15 s at 95°C and 1 min at 60°C. The fold change of gene expression was calculated according to the 2-ΔΔCt method (Pfaffl, 2001), using the expression of the elongation factor 1α (efl1α) as a normalizer and the expression of all markers under control conditions as a calibrator.
Cell Infection Assays
SHK-1 cells (passage 72) were cultured without antibiotics in Leibovitz’s L-15 medium supplemented with 10% fetal bovine serum (FBS) at 20°C in 75-cm2 flasks. Cells were seeded into 24-well plates at a concentration of ~1.0 × 105 cell/well, incubated for 24 h at 20°C and then infected with the selected P. salmonis strain at a multiplicity of infection (MOI) = 10. Purified nucleic acid samples were collected from the cell culture at 5 and 10 days post infection (dpi). Cell damage induced by P. salmonis in SHK-1 cell monolayers was determined by the release of the cytosolic enzyme lactate dehydrogenase (LDH) into the medium using a commercial Cytotoxicity LDH Detection Kit (Thermo Scientific). LDH activity levels were analyzed in 50 µL aliquots of cell-free supernatant obtained from each well. Additionally, the supernatant of cells lysed with L-15 medium containing 1% Triton X-100 was used as a total lysis control (maximum LDH liberation) and the supernatant of uninfected cells was used as a negative control (basal LDH liberation). Optical densities measured at 490 nm and 680 provided the basis for calculations as recommended by the manufacturer of the detection kit.
The in vitro infectivity of P. salmonis was assessed using an endpoint dilution assay (TCID50) as described early, with few modifications (House et al., 1999). CHSE-214 cells were cultured in 96-well flat-bottom microplates in antibiotic-free L-15 Medium supplemented with 10% FBS and incubated at 20°C until they reached confluence (~5.0 × 104 cells/well). As inoculum, bacterial suspensions (~5.0 × 107 cfu/mL) were prepared in L-15 supplemented with 2% FBS, and log10 dilutions were made up to the 10th dilution. Monolayers were infected (8 wells/dilution), incubated for 15 days at 18°C, and then visually inspected for the presence of cytopathic effects. TCID50 were calculated according to the Spearman–Kärber algorithm (Hierholzer and Killington, 1996).
In Vivo Evaluation
Atlantic salmon smolts were confirmed as “pathogen free” using RT-PCR routine diagnostic methods for IPNV, ISAV, PRV, P. salmonis and Renibacterium salmoninarum. After their transfer to the experimental center, fish were tagged using Passive Integrated Transponder tags (PIT tags). The animals were allowed to acclimatize to seawater for 21 days and fed ad libitum; they were maintained at 14 ± 1°C, with a water change rate of 1.2 - 1.5/hour, pH of 7.0 - 8.0, a photoperiod of 24 light hours, and a specific feeding rate of 1.5 - 2%. Prior to any handling and marking, fish were anesthetized with benzocaine. Finally, euthanasia was performed using an overdose of anesthetic. All efforts were made to provide best growth conditions and minimize suffering.
The in vivo challenge was carried out on 420 fish with an approximate weight of 90 - 100 g. These fish were uniformly allocated to three 0.5 m3 tanks, yielding initial densities of 25 - 30 kg/m³ and 140 fish/tank. 35 fish of each tank comprised a single treatment group that received 0.1 mL of bacterial suspension (either P. salmonis wild-type, P. salmonis Δwzx, or P. salmonis ΔwcaJ) or vehicle (BSS). Fresh bacterial suspensions (OD600 = 0.7) were used to prepare the inocula that were administered to the fish via intraperitoneal injection. In parallel, a sample of each inoculum was utilized to perform bacterial counts at the time of injection.
Fish were monitored daily for 30 days, and mortalities were removed from the tanks. As for tanks 1 and 2, mortalities were recorded and subjected to necropsy. Additionally, PCR of internal organ samples were done to assess for the presence of P. salmonis. The third tank was intervened on days 5, 12 and 20 post infection, removing three live fish/group to collect head kidney tissue samples for gene expression analysis.
Statistical Analysis
Data obtained from the bactericidal serum effect studies were tested for normal distribution using the Shapiro–Wilk test and were subsequently analyzed with the non-parametric Mann–Whitney rank-sum test. For biofilm quantification and cytotoxicity assays, the mean and standard deviation values were compared with the Kruskal-Wallis test and Dunn’s multiple comparison test as a post-hoc analysis (p < 0.05). These same tests were applied to the mean and standard deviation values of the fold change of gene expression, which are given in terms of relative expression. PCR efficiencies were determined by linear regression analysis of sample data using LinReg-PCR. With regard to in vivo testing, the percentages of cumulative mortalities were analyzed using the Kaplan-Meier method, and the differences were evaluated using the log-rank test performed with GraphPad Prism v6.0.
Results
Identification of Genes Encoding Functions Related to Polysaccharide Biosynthesis
BLAST searches and annotation analyses allowed for the identification of a ~18 kb cluster carrying 15 genes putatively involved in the biosynthesis of LPS (Figure 1). Those genes presumably encode enzymes involved in furnishing (glycosyltransferases), O-chain transport across the membrane, and precursor biosynthesis (Table 3). An O-antigen flippase (wzx, KW89_2967, 462 amino acids), which catalyzes the transport of the O-antigen unit across the inner membrane, was predicted. We also found genes encoding proteins possibly participating in the polymerization of the O-antigen (wzy, KW89_2964; wzz, KW89_2972). Surprisingly, homologs of the waaL O-antigen ligase, which facilitates the transference of polymerized O-antigen to the core-lipid A, could not be identified. A putative undecaprenyl-phosphate glucose phosphotransferase or wcaJ (KW89_2959, 466 amino acids) was found to be located in that same cluster, but upstream of wzx and in close proximity to another gene encoding an S-layer protein. The enzyme encoded by wcaJ is allegedly responsible for the initiation of the synthesis of colanic acid, which is part of the EPS and the capsule of the Enterobacteriaceae family members. In detail, undecaprenyl-phosphate glucose phosphotransferase accounts for the transfer of glucose-1-phosphate onto the carrier lipid undecaprenyl phosphate. Interestingly, a second cluster of ~8.5 kb containing genes for the biosynthesis of a capsular polysaccharide was found (Figure S3 and Table S1). Some genes of this cluster show similarities with orthologs in Francisella philomiragia and F. noatunensis subsp. orientalis.

Figure 1 P. salmonis PM15972 LPS gene cluster. Genetic organization of the ~18 kb cluster containing genes putatively involved in the biosynthesis of LPS and EPS. Mutagenesis gene targets wzx and wcaJ are marked in black. For a detailed description of gene functions, see Table 3.
According to the literature, a knock-out mutation of wzx or wcaJ would carry a detrimental effect on the biosynthesis of LPS and EPS, respectively. Therefore, altered virulence phenotypes of the corresponding mutants could be expected if those structures play a role in the pathogenesis of piscirickettsiosis.
Mutagenesis of wzx and wcaJ Genes
Since the target genes are likely to be part of operon units, the mutagenesis approach was engineered to yield in-frame deletion mutants. Mutator plasmids were designed using the pJQK genetic tool, a suicide plasmid already tested in P. salmonis (Mancilla et al., 2018). We have chosen a strategy similar to that used in other bacterial models, replacing the wild-type gene by a short, afunctional copy of the same gene. This way, we could avoid polar effects and remove antibiotic resistance markers (Figure 2A and Figure S4). The interchanged alleles remaining after the mutagenesis process were detected and confirmed by conventional PCR (Figure 2B). It shall be mentioned that the mutant strains have lost their kanamycin resistance markers and displayed minimal inhibitory concentration (MIC) values at control, wild-type levels.
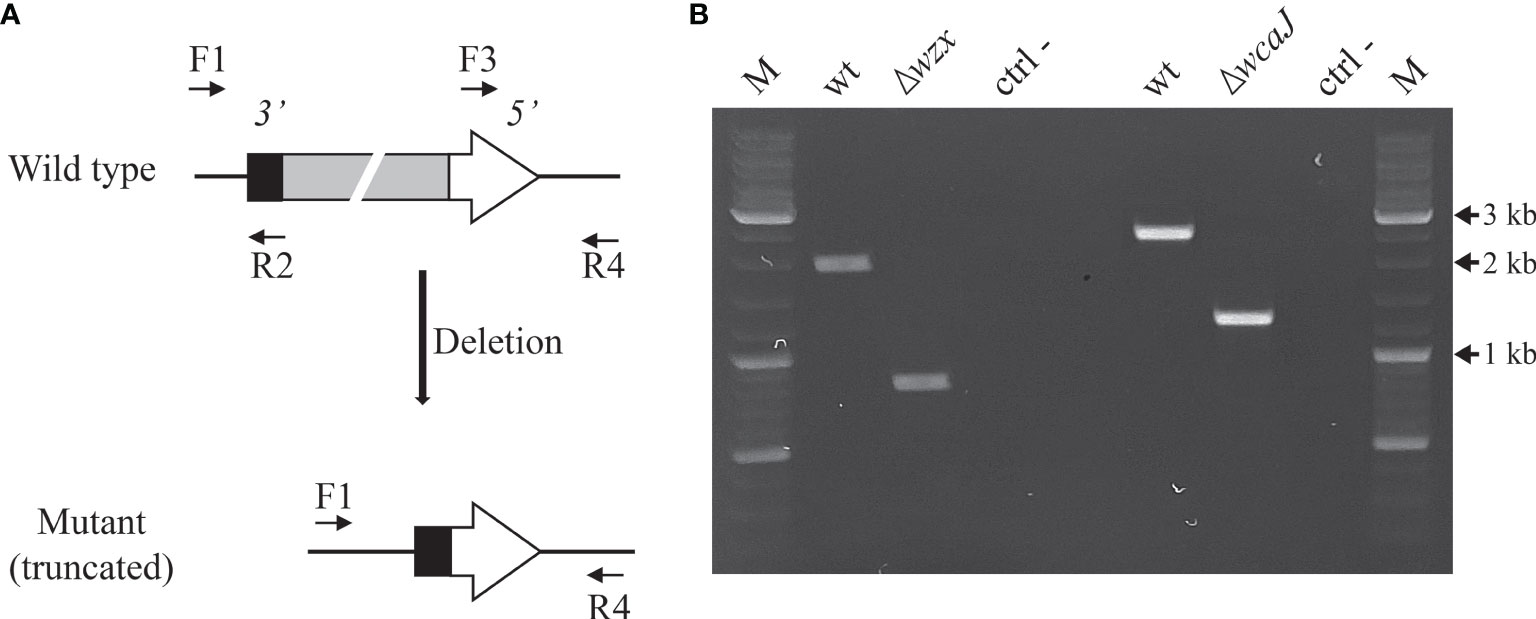
Figure 2 Genotypic characterization of Δwzx and ΔwcaJ P. salmonis mutant strains. (A) Position of primer pairs to accomplish PCR overlap and expected engineered mutant allele. (B) PCR analysis of wild-type and mutant strains using flanking primers F1 and R4 for each gene. ctrl-, non-template control. M,10 kb DNA ladder.
P. salmonis Mutants Display Particular In Vitro Phenotypes
Cell envelope mutants frequently present colony phenotypes that are easily distinguished on agar plates. However, isolated colonies from the P. salmonis mutants described in this study looked macroscopically identical to the wild-type strain (not shown). Growth kinetics in liquid medium showed no differences between the mutants and the parent strain (Figure S5). Considering prior reports on the self-agglutination ability of mutants with cell envelope defects, we studied our P. salmonis strains in this regard. On the one hand, we measured the optical density of bacterial suspensions in liquid medium, and we conducted acriflavine tests. In liquid medium, mutant strains displayed a higher self-agglutination capacity than their parent strain, and this characteristic was particularly pronounced in ΔwcaJ (Figure 3A). On the contrary, the acriflavine test was positive only for the Δwzx strain, where cell clumps became evident (Figure 3B). Since LPS is the main target of some antibiotics, such as polymyxin B, we assessed the mutant strains’ MIC to this antibiotic. Unexpectedly, similar MIC values were determined for the parent and mutant phenotypes (8 - 16 µg/mL).
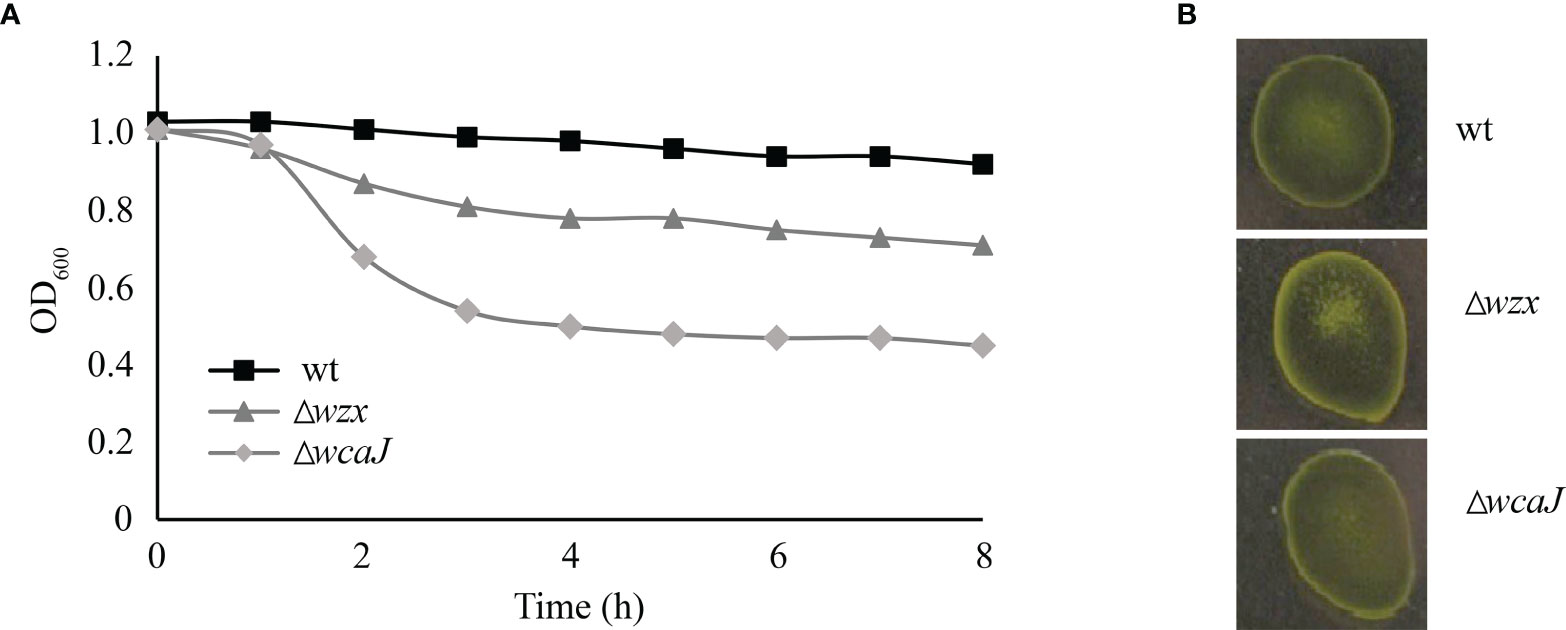
Figure 3 Self-agglutination assessment in P. salmonis strains. (A) Kinetic OD measurement of wild-type and mutant bacterial suspensions. (B) Acriflavine slide test.
In a Δwzx genetic background, it is expected to find O-chain-deficient LPS species with a lower molecular mass when compared with the parent strain. That feature can be explored by electrophoretic profiling, so we examined the profiles of LPS samples from the parent and mutant strains in a 15% conventional acrylamide gel. Consistent with previous reports (Kuzyk et al., 1996; Vadovic et al., 2007), we visualized a single-band profile with an apparent molecular mass of 11 kDa, but did not find any differences among tested strains (not shown). Upon increasing the resolution of polyacrylamide gels, we observed subtly augmented electrophoretic mobility for the Δwzx LPS fraction, when compared with the parent and ΔwcaJ mutant strains (Figure 4A). This pattern resembled the canonical core-lipid A profile. In a next step, we used crude protein samples that would be expected to carry LPS, performed Western blots and finally incubated with immunoreactive rabbit serum against P. salmonis wild-type. While samples corresponding to the parent and ΔwcaJ mutant strains yielded similar profiles, the Δwzx mutant lacked a band (Figure 4B). An identical pattern was observed on a Western blot of LPS samples extracted without organic solvent and extensively treated with proteinase K (Figure 4C).
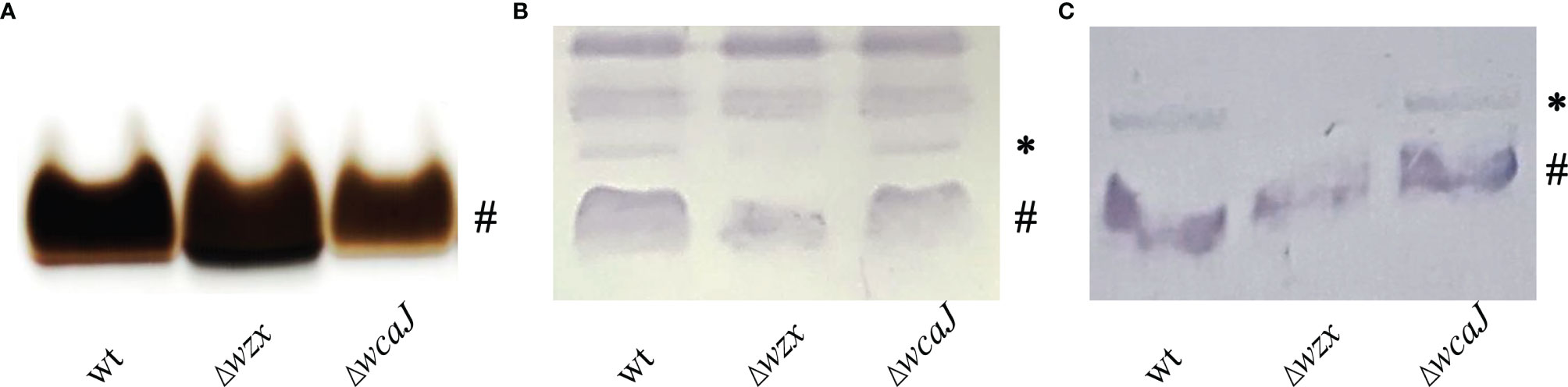
Figure 4 LPS characterization. (A) Silver-stained LPS fractions from each strain extracted with organic solvent. (B) Western blots of crude protein samples and (C) LPS extracted without organic solvent. Blots were incubated with rabbit polyclonal serum against P. salmonis wild-type strain. (#) refers to core-lipid A species, while (*) indicates the core-lipid A plus O-antigen, which is absent in Δwzx mutant.
Some bacterial pathogens are able to resist the killing activity of naïve serum. While this applies to P. salmonis, the resistance of this pathogen seems to depend on its genetic background (Saavedra et al., 2017). In this regard, we found the parent and the Δwzx mutant strains to display partial susceptibility to salmon naïve serum. The ΔwcaJ strain, however, proved to be completely susceptible to the serum treatment and was unable to survive (Figure 5).
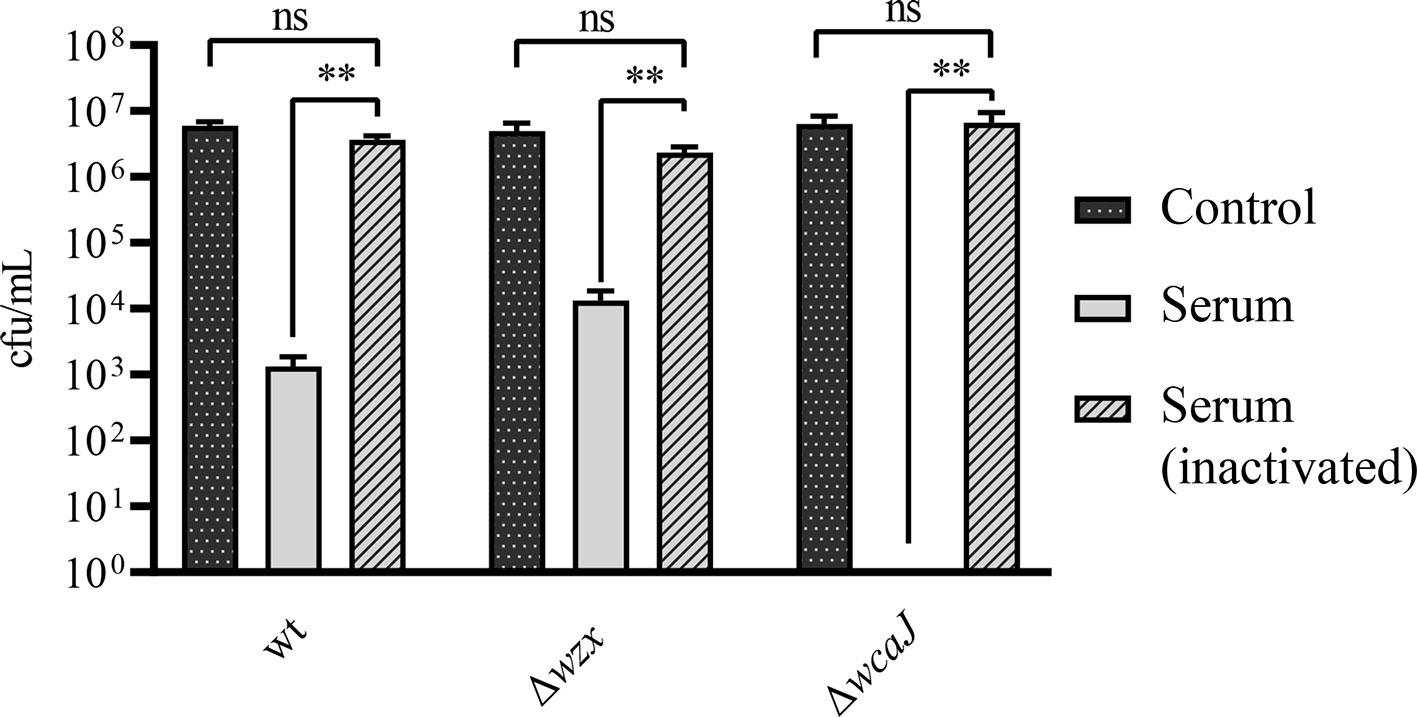
Figure 5 Bactericidal action of naïve serum. Survival is denoted as colony counts recovered after serum treatment. Control corresponds to bacterial suspension without treatment. Differences were statistically significant (**p < 0.01). No significant differences are denoted by "ns"
Available experimental evidence does not unequivocally confirm the role of the wcaJ gene in the biosynthesis of EPS. While a knock-out mutation of this gene in the fish pathogen Edwarsiella tarda caused an impairment of biofilm production (Yu et al., 2015), enhanced biofilm formation was described for Klebsiella pneumoniae carrying the same mutation (Pal et al., 2019). As for our P. salmonis strains, the evaluation of biofilm production after 30 days of incubation showed that the disruption of wcaJ is associated with enhanced biofilm formation. The corresponding mutant strain formed up to 50% more biofilm than the wild-type strain. In contrast, no differences were observed between the Δwzx and wild-type strains (Figure 6).
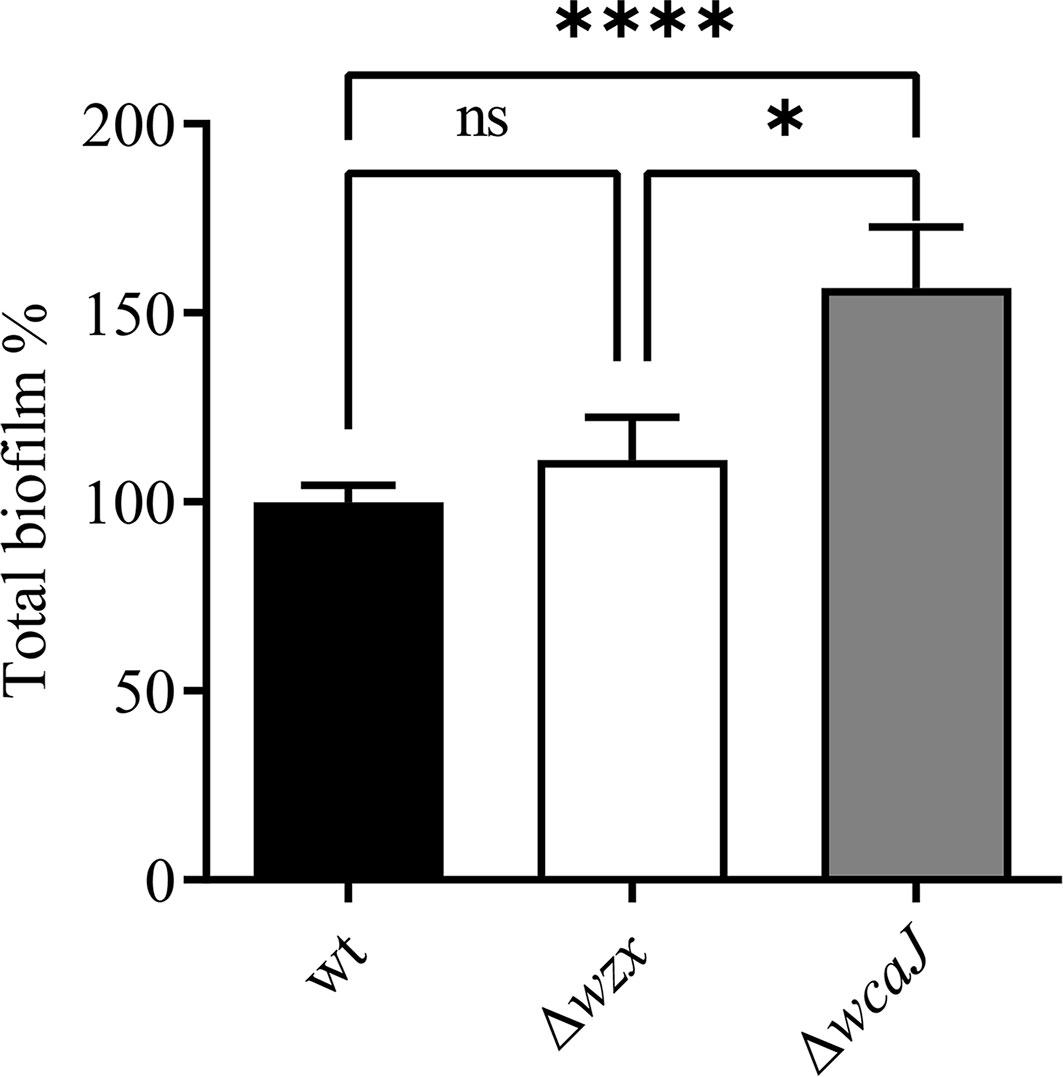
Figure 6 Biofilm formation assay. Ability of biofilm formation expressed as percentage of crystal violet staining using the wild-type strain as reference. Enhanced ability of biofilm production by the ΔwcaJ strain was statistically significant (*p < 0.05 and ****p < 0.001). No significant differences (ns) were found between Δwzx and its parent strain.
Mutants Are Distinguishable in LDH Cytotoxicity Assays
The CHSE-214 cell line has been described as susceptible to infection with P. salmonis. Hence, we hypothesized that using comparable amounts of bacteria to perform TCID50 studies would reveal potential differences in the virulence of mutant strains. We thus conducted the assays at a similar MOI. Notwithstanding, titers reached by the mutants and the parent strain were in the same order of magnitude and ranged from 6.0 - 6.7 × 105 TCID50/ml. In a second, independent experiment, calculated titers were somewhat higher, but still largely identical across P. salmonis strains, ranging from 8.0 - 9.3 × 105 TCID50/ml. In order to consolidate these findings, we decided to further assess the virulence of P. salmonis mutants in SHK-1 cells. The direct observation of cytopathic effects is less reliable in SHK-1 cells, which is why we opted for the quantification of LDH release and activity as an alternative, more sensitive measure of the in vitro virulence of P. salmonis strains in this cell line. To this end, monolayers of SHK-1 cells were infected at a lower MOI and incubated at 18°C for 10 days. In this model, both mutants exhibited lowered cytotoxicity when compared with their parent strain (Figure 7). The Δwzx mutant was the most attenuated one.
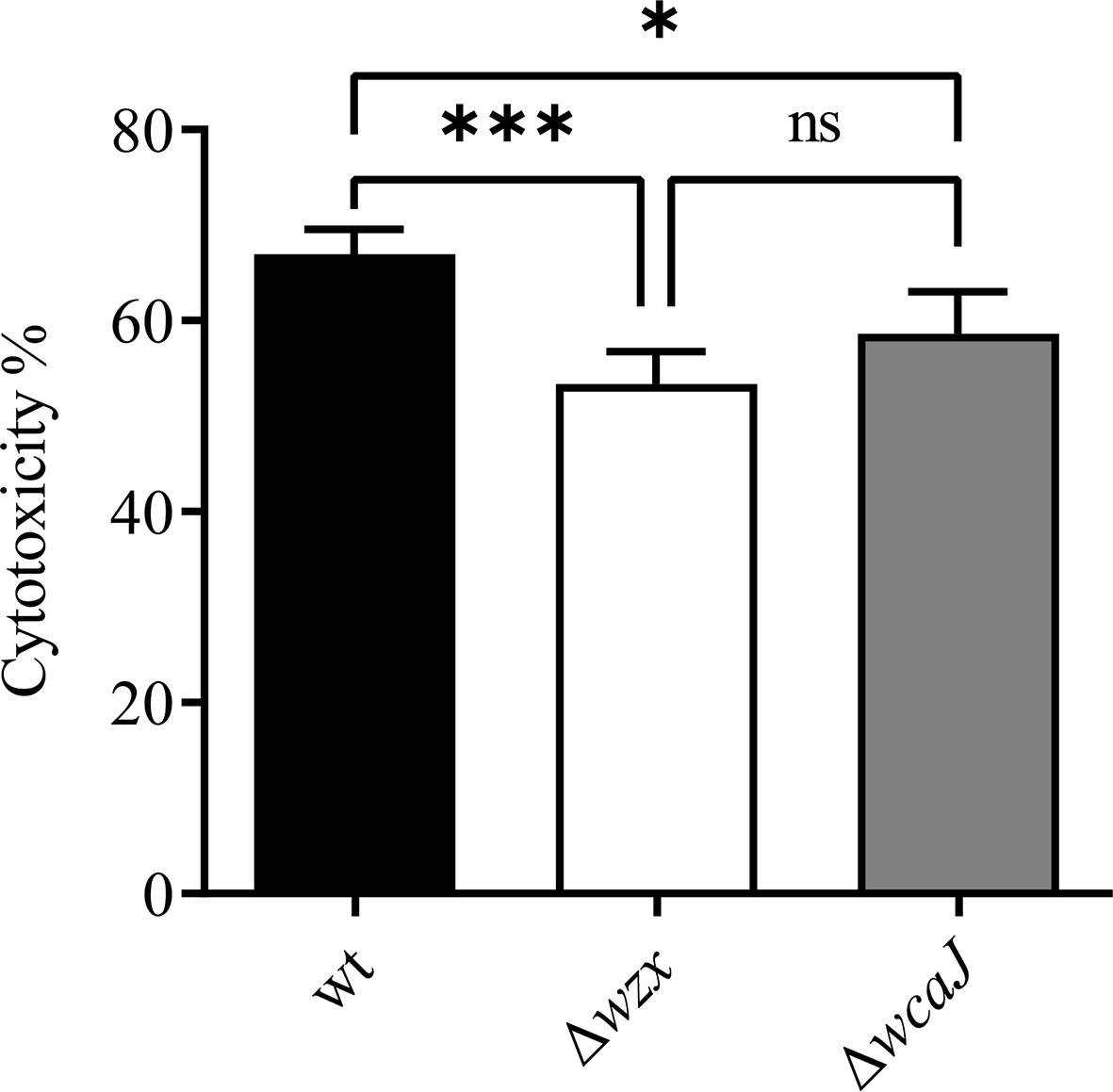
Figure 7 Cytotoxicity assay. LDH release by SHK-1 cells expressed as percentage of enzymatic activity using the wild-type as reference. Differences were statistically significant (*p < 0.05 and ***p < 0.005). No significant differences are denoted by "ns".
Mutants Trigger a Differential Proinflammatory Response In Vitro and In Vivo
Pathogen mutants with cell envelope defects tend to induce differential patterns of gene expression of immune response markers in host cells. We tested this hypothesis for P. salmonis strains by determining the expression profiles of a proinflammatory cytokine gene panel in SHK-1 cells, sampled at 5 or 10 dpi. All strains were able to stimulate a proinflammatory response, but clear differences became evident (Figure 8A). The Δwzx strain upregulated the expression of il1β and downregulated the expression of tnfα at 5 dpi. At 10 dpi, tnfα and ifnγ were downregulated. Interestingly, cells infected with the ΔwcaJ strain showed a notable upregulation of tnfα at both points in time (>20-fold change), while il1β was found to be upregulated at 5 dpi but dropped below reference values at 10 dpi. As for the expression of ifnγ after infection with the ΔwcaJ strain, a clear downregulation was registered at 5 dpi. Gene expression was also examined in samples derived from the in vivo assay. An upregulation of the expression of il1β and tnfα was recorded at 5 dpi in fish infected with all strains tested (Figure 8B). Consistent with the pattern observed in vitro, a downregulation of ifnγ expression was observed at 5 dpi in the group infected with the ΔwcaJ strain. Interestingly, at 12 dpi, expression profiles of all markers tended to level off at reference levels, with the exception of the ifnγ transcript. The expression pattern for this cytokine gene was characterized by persistent downregulation in fish treated with the ΔwcaJ strain. Extensive mortality was registered in groups infected with the parent and ΔwcaJ strains; only fish from the Δwzx-challenged group survived up to 20 dpi. Therefore, no comparison of gene expression could be made at this sampling point in time. We highlight the reliability of our qPCR data, which it can be deduced from the normalizer behavior across samples (Figure S6).
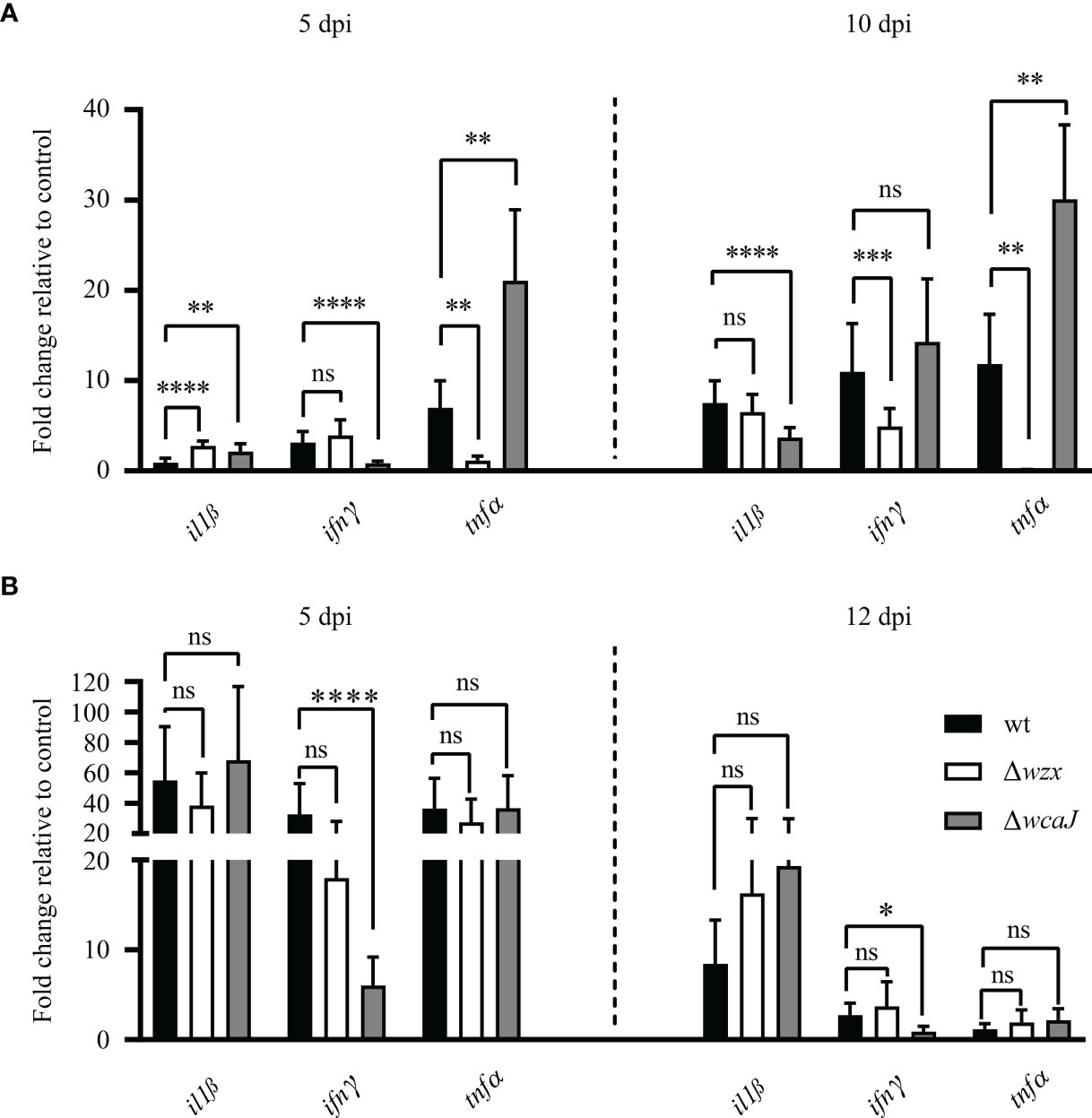
Figure 8 Proinflammatory marker gene expression after bacterial infection. Relative gene expression of cytokine genes il1ß, ifnγ and tnfα as observed in the (A) in vitro SHK-1 cell model of P. salmonis infection and (B) in intraperitoneally challenged Atlantic salmon smolts. Differences were statistically significant (*p < 0.05, **p < 0.01, ***p < 0.005 and ****p < 0.001). No significant differences are denoted by "ns".
Δwzx Mutant Presents Lower Virulence in S. salar
The in vivo assay was carried out infecting S. salar smolt cohorts with similar amounts of P. salmonis strains via intraperitoneal injection. According to our experience with the challenge strain, the horizontal transmission of P. salmonis PM15972 is negligible in the period set for the experiment, and any mortality occurring within that time frame may be interpreted as an effect of the injected material. The target concentration of the inoculum was set at 1.0 × 107 cfu/fish (Mancilla et al., 2018). Precisely, individual fish were inoculated with 3.0 × 107 and 2.6 × 107 cfu of the parent strain and Δwzx mutant, respectively, while the ΔwcaJ treatment group received 1.3 × 106 cfu/fish. As shown in Figure 9, the group injected with the parent strain accumulated almost 100% mortality within a few days. Treatment with the ΔwcaJ mutant induced a similar mortality curve, although it was slightly displaced in time. Fish infected with P. salmonis Δwzx had a significantly higher probability of survival in both tanks. Necropsies conducted on dead fish revealed pathognomonic signs of piscirickettsiosis in internal organs, regardless of the challenge strain (not shown).
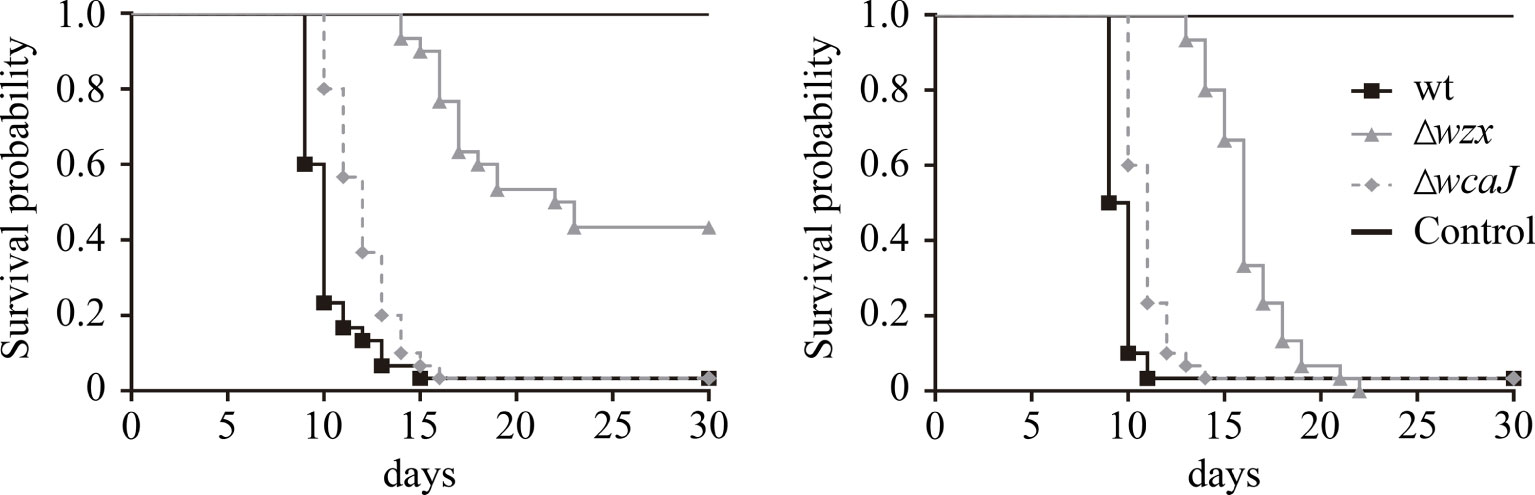
Figure 9 In vivo virulence of the mutants and wild-type strain. Results obtained from two tanks, each containing three treatment and one control group each consisting of 35 fish. Differences in the probability of survival were statistically significant for the Δwzx mutant group in both tanks (p < 0.001).
Discussion
Classical works done on the subject of colony variants were reported at the very beginning of microbiology. These studies did not only provide insight into a biological phenomenon, but also laid the foundation for the development of bacterial attenuated vaccines (Van der Woude and Baumler, 2004). Notwithstanding, colony variants are hard to be observed for P. salmonis. In the present study, we had to use a variety of indirect techniques to establish the differences between the engineered strains.
Our findings are consistent with response evasion mechanisms conferred by LPS and EPS for P. salmonis. On the one hand, LPS with large, heteropolymeric O-antigens displays a typical ladder-like banding pattern of the repeating sugar units in SDS-PAGE, but this pattern cannot be reproduced with P. salmonis LPS (Figure 4A). In addition, the Δwzx mutant presented levels of resistance to serum and MIC values very similar to its parent strain. In light of the alteration of LPS, this was an unexpected finding, but similar results have been reported for the Gram-negative fish pathogen Aliivibrio salmonicida. The LPS structure of that bacterium resembles a lipooligosaccharide (LOS) rather than a classical, long O-chain furnished LPS, and a structural LPS mutant did not present alterations of the serum resistance phenotype when compared with its parent strain (Norstebo et al., 2018). So as to explain the reduced susceptibility to polymyxin B manifested by P. salmonis, we may refer to findings obtained in naturally virulent, rough species of the Brucella genus. These pathogens have a truncated O-chain LPS and are not only resistant to the action of complement, but also to that of positively charged antimicrobial peptides, including polymyxin B (Stranahan and Arenas-Gamboa, 2021). Taking into account these precedents, our results obtained in serum survival and polymyxin B MIC tests may be related to P. salmonis having evolved carrying an LPS with an atypical short O-antigen, suggesting that the LOS structure conferred an evolutionary advantage to this pathogen.
On the other hand, the ΔwcaJ mutant proved to be fully susceptible to serum, and this phenotype closely resembles capsule-deficient strains that have already been described in the literature (Renzi et al., 2016; Tan et al., 2020). We may hypothesize that the loosely attached EPS of the ΔwcaJ mutant results in a decreased ability to sterically hinder the access of complement components to the bacterial surface, thereby increasing its susceptibility to serum (Rautemaa and Meri, 1999; Holland and Lambris, 2002). In this context, it is tempting to speculate that the EPS is masking some binding sites of complement proteins, which may remain exposed in the ΔwcaJ mutant. This idea also supports the notion that a fraction of EPS is attached to the membrane. Beyond this speculation, it is clear that the biosynthesis of the P. salmonis LPS is not affected by the disruption of wcaJ, which is probably participating in a biosynthesis pathway of a different glycan. The differential effects of wzx and wcaJ mutations on the cell envelope structure of P. salmonis are illustrated in Figure 10.
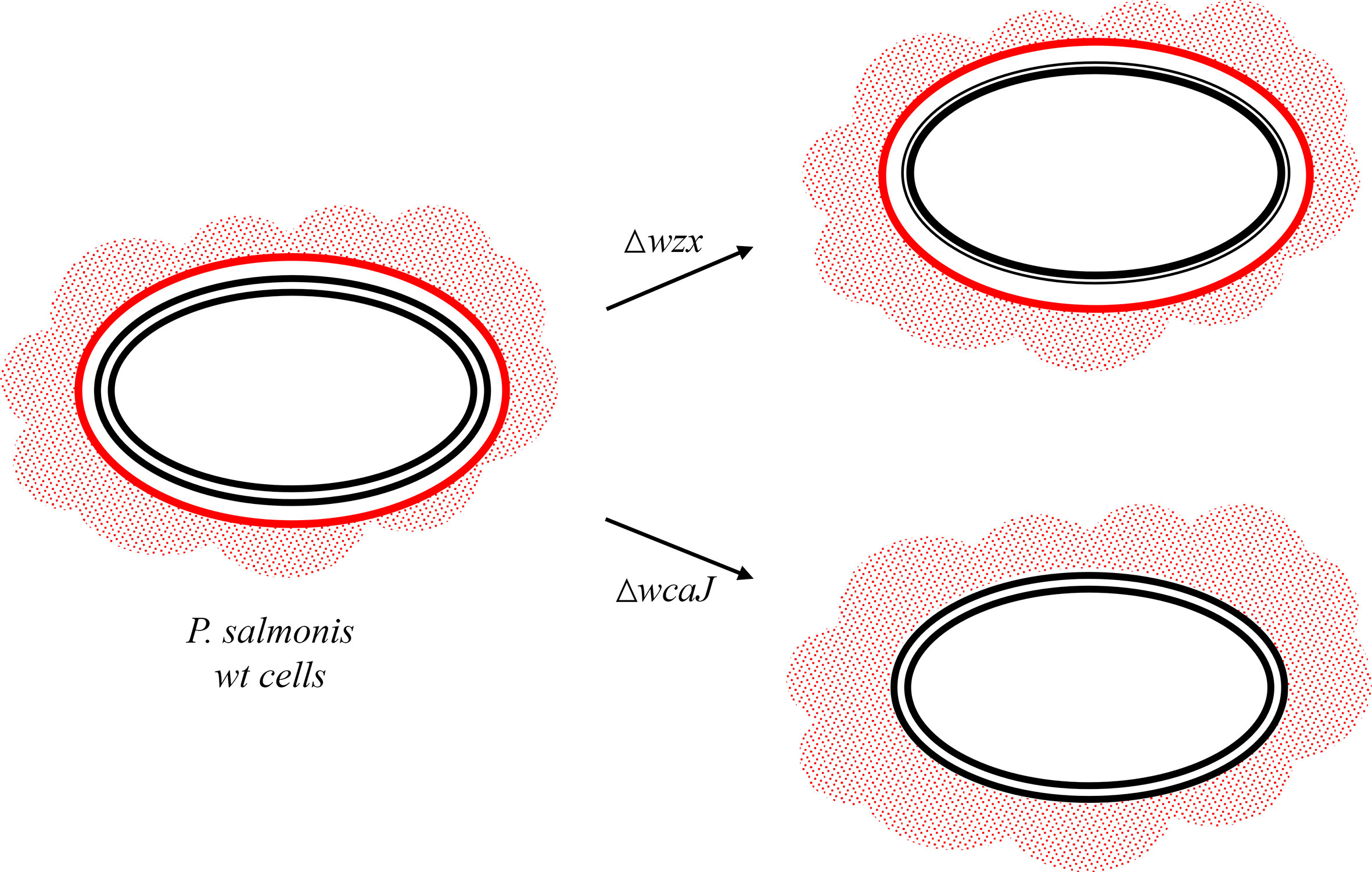
Figure 10 Model of cell envelope defects in P. salmonis mutants. In the Δwzx mutant, the most external leaflet of the lipid bilayer (black, double line) is devoid of O-chain LPS. In contrast, ΔwcaJ behaves as a capsule-deficient strain (single red line) that retains its ability to form biofilm (red dotted area).
Even though our study did not aim at resolving the structure of P. salmonis LPS, our findings allow to draw some conclusions on this topic. According to the work of Vinogradov and colleagues, the oligosaccharide backbone structure of P. salmonis LPS is consistent with a LOS structure. We observed a single band pattern with no higher molecular mass bands in silver-stained gels, but samples obtained from the Δwzx mutant under mild conditions were lacking an immunoreactive band characteristic of the wild-type strain (Figure 4B). Our interpretation of this band is that it does represent a carbohydrate antigen, most probably the core-lipid A plus O-antigen. The fact that this band is absent in silver-stained gels may be explained by the labile nature of the covalent bond linking this carbohydrate unit to the core-lipid A. This hypothesis is strongly supported by Western blot patterns of LPS samples extracted without organic solvent (Figure 4C). Additional evidence comes from Δwzx mutant LPS samples purified by the Westphal phenol method, which exhibit only the band corresponding to the O-antigen-devoid core-lipid A structure (Figure S7). Increased sensitivity to organic solvents of the bond bridging the O-chain and core-lipid A has also been observed in unrelated bacteria such as Pseudomonas aeruginosa (Abeyrathne et al., 2005). Therefore, our results confirm the findings of Vinogradov et al. (2013) and extend their conclusion to a putative single O-antigen unit bound to the core-lipid A backbone of wild-type P. salmonis LPS.
It has been reported that P. salmonis hexacyl lipid A reminds canonical Enterobacteriaceae structure (Vadovic et al., 2007). Furthermore, it has been demonstrated for members of this family that colanic acid forms part of the EPS and disruption of wcaJ (also known as wecA) hampers the biosynthesis of this molecule, thereby affecting biofilm formation and other phenotypic traits (Raetz and Whitfield, 2002). Indeed, biofilm formation can be diversely affected by wcaJ deletion and thus we deduced that wcaJ may be involved in the biosynthesis of EPS in P. salmonis. Contrary to our expectations, the ΔwcaJ mutant yielded more biofilm than the parent strain. The mechanisms underlying this phenotype are not clear and findings in the literature are contradictory, e.g., a K. pneumoniae ΔwcaJ mutant was shown to produce relatively higher amounts of biofilm (Pal et al., 2019). One possibility is that P. salmonis does not synthesize colanic acid, but a compositionally different EPS as part of a capsule. Since undecaprenyl phosphate, also known as bactoprenol, is a common substrate for the biosynthesis of diverse bacterial glycans, the disruption of wcaJ may affect the availability of this precursor in the EPS pathway. Thus, material may be left for export and contribute to the unorganized biofilm architecture rather than being available for the assembly of a capsule covering the bacterium. In support of this notion, a capsular polysaccharide biosynthesis gene cluster was evidenced by sequence similarity. This finding argues in favor of a capsular antigen in P. salmonis, but also provides genetic evidence for the modifications related to diacetylated pseudaminic acid residues found in the LPS core structure described previously (Vinogradov et al., 2013).
Altogether, we provide evidence supporting the hypothesis that the P. salmonis LPS O-chain is transported across the membrane via a Wzx-Wzy dependent pathway and that its disruption results in a structural LPS mutant. Moreover, complete LPS is required for full virulence of the pathogen in the intraperitoneal model of infection in S. salar as it can be deduced from the results of our in vivo assay. Further studies are needed to elucidate the genetic basis of oligosaccharide core modifications, which is decorated with chemical groups that may help to evade innate immune mechanisms such as antimicrobial peptides and complement. In the case of EPS, future research is warranted since our results suggest that this biopolymer may be more complexly structured than assumed to date and that the presence of a capsule cannot be discarded. At this point in time, however, we also cannot rule out a role of EPS in pathogenicity. In light of this seemingly contradictory conclusion, we note the fact that our intraperitoneal infection model does not necessarily mimic the natural route of transmission of piscirickettsiosis. Additionally, the EPS seems to be masking some pathogen-associated molecular pattern (PAMP) that modulates the immune response in the host, as can be inferred from the differential gene expression patterns prompted by the ΔwcaJ mutant. Indeed, our results suggest that the hypothetical exposed PAMP in that mutant downregulates the expression of ifnγ, in vitro and in vivo, and upregulates tnfα in vitro, while the Δwzx mutant does not seem to modify the expression profiles of cytokine genes studied in vivo, but yields a significant downregulation of tnfα in vitro. With regard to the cause of differences between in vitro and in vivo results, it must be considered that the in vivo response reflects transcript levels, which are representative only for the tissue tested. Measured values may not be directly comparable to those detected in samples derived from a stimulated cell line. Further research is needed to give a satisfactory explanation for the differences detected in both models, but the response induced by the mutants clearly accounts for an altered host-pathogen interaction as compared with the one elicited by the wild-type strain.
In sum, our findings on P. salmonis cell envelope components are consistent with a stealth strategy, in which the pathogen evades the detection by the host immune system in the early stages of infection. This view is also complementary to the imbalance towards a suppressive immune response that takes place during the late intracellular replication of P. salmonis cells.
Data Availability Statement
The original contributions presented in the study are included in the article/Supplementary Material. Further inquiries can be directed to the corresponding authors.
Ethics Statement
The animal study was reviewed and approved by ADL Diagnostic Chile ethics committee.
Author Contributions
VH carried out the in vitro characterization of bacterial strains, along with NO. Gene expression analysis was also performed by VH in collaboration with YY. JS, and OA performed the mutagenesis experiments. PB provided approval for publication. MM conceived and designed the study with the help of OA, and both wrote the paper. All authors contributed to the article and approved the submitted version.
Funding
This research was funded by Corporación de Fomento de la Producción de Chile, CORFO, grants 16ITE1-71014 and 17PIDE-80700, and Fondo de Desarrollo Científico y Tecnológico, FONDECYT/ANID, postdoctoral grant 3170340. The authors declare that this study received funding from Blue Genomics SpA and ADL Diagnostic Chile. The funders were not involved in the study design, collection, analysis, interpretation of data, the writing of this article or the decision to submit it for publication.
Conflict of Interest
Authors VH and OA were employed by Blue Genomics SpA.
The remaining authors declare that the research was conducted in the absence of any commercial or financial relationships that could be construed as a potential conflict of interest.
Publisher’s Note
All claims expressed in this article are solely those of the authors and do not necessarily represent those of their affiliated organizations, or those of the publisher, the editors and the reviewers. Any product that may be evaluated in this article, or claim that may be made by its manufacturer, is not guaranteed or endorsed by the publisher.
Acknowledgments
We are grateful for the skillful assistance of Aquainnovo’s technical staff who managed the in vivo testing. We also thank Dr Melanie Kaiser for editing the manuscript.
Supplementary Material
The Supplementary Material for this article can be found online at: https://www.frontiersin.org/articles/10.3389/fcimb.2022.845661/full#supplementary-material
References
Abeyrathne, P. D., Daniels, C., Poon, K. K., Matewish, M. J., Lam, J. S. (2005). Functional Characterization of WaaL, a Ligase Associated With Linking O-Antigen Polysaccharide to the Core of Pseudomonas Aeruginosa Lipopolysaccharide. J. Bacteriol. 187, 3002–3012. doi: 10.1128/JB.187.9.3002-3012.2005
Albornoz, R., Valenzuela, K., Pontigo, J. P., Sanchez, P., Ruiz, P., Avendano-Herrera, R., et al. (2017). Identification of Chemotaxis Operon cheYZA and cheA Gene Expression Under Stressful Conditions in Piscirickettsia Salmonis. Microb. Pathog. 107, 436–441. doi: 10.1016/j.micpath.2017.04.030
Almarza, O., Valderrama, K., Ayala, M., Segovia, C., Santander, J. (2016). A Functional Ferric Uptake Regulator (Fur) Protein in the Fish Pathogen Piscirickettsia Salmonis. Int. Microbiol. 19, 49–55. doi: 10.2436/20.1501.01.263
Bohle, H., Henriquez, P., Grothusen, H., Navas, E., Sandoval, A., Bustamante, F., et al. (2014). Comparative Genome Analysis of Two Isolates of the Fish Pathogen Piscirickettsia Salmonis From Different Hosts Reveals Major Differences in Virulence-Associated Secretion Systems. Genome Announc. 2, e01219-14. doi: 10.1128/genomeA.01219-14
Calquin, P., Ruiz, P., Oliver, C., Sanchez, P., Haro, R., Oliva, H., et al. (2017). Physiological Evidence That Piscirickettsia Salmonis Produces Siderophores and Uses Iron From Different Sources. J. Fish Dis. 41, 553–558. doi: 10.1111/jfd.12745
Carril, G. P., Gomez, F. A., Marshall, S. H. (2016). Expression of Flagellin and Key Regulatory Flagellar Genes in the Non-Motile Bacterium Piscirickettsia Salmonis. Dis. Aquat. Organ 123, 29–43. doi: 10.3354/dao03079
Conde-Alvarez, R., Grillo, M. J., Salcedo, S. P., De Miguel, M. J., Fugier, E., Gorvel, J. P., et al. (2006). Synthesis of Phosphatidylcholine, A Typical Eukaryotic Phospholipid, is Necessary for Full Virulence of the Intracellular Bacterial Parasite Brucella Abortus. Cell Microbiol. 8, 1322–1335. doi: 10.1111/j.1462-5822.2006.00712.x
Donot, F., Fontana, A., Baccou, J. C., Schorr-Galindo, S. (2012). Microbial Exopolysaccharides: Main Examples of Synthesis, Excretion, Genetics and Extraction. Carbohydr. Polym. 87, 951–962. doi: 10.1016/j.carbpol.2011.08.083
Fryer, J. L., Hedrick, R. P. (2003). Piscirickettsia Salmonis: A Gram-Negative Intracellular Bacterial Pathogen of Fish. J. Fish Dis. 26, 251–262. doi: 10.1046/j.1365-2761.2003.00460.x
Gomez, F. A., Tobar, J. A., Henriquez, V., Sola, M., Altamirano, C., Marshall, S. H. (2013). Evidence of the Presence of a Functional Dot/Icm Type IV-B Secretion System in the Fish Bacterial Pathogen Piscirickettsia Salmonis. PloS One 8, e54934. doi: 10.1371/journal.pone.0054934
Henriquez, P., Kaiser, M., Bohle, H., Bustos, P., Mancilla, M. (2016). Comprehensive Antibiotic Susceptibility Profiling of Chilean Piscirickettsia Salmonis Field Isolates. J. Fish Dis. 39, 441–448. doi: 10.1111/jfd.12427
Hierholzer, J. C., Killington, R. A. (1996). “Virus Isolation and Quantitation” in Virology Methods Manual. Eds. Mahy, B. W. J., Kangro, H. O. (Academic Press), 25–46. doi: 10.1016/B978-012465330-6/50003-8
Hitchcock, P. J., Brown, T. M. (1983). Morphological Heterogeneity Among Salmonella Lipopolysaccharide Chemotypes in Silver-Stained Polyacrylamide Gels. J. Bacteriol. 154, 269–277. doi: 10.1128/jb.154.1.269-277.1983
Holland, M. C., Lambris, J. D. (2002). The Complement System in Teleosts. Fish Shellfish Immunol. 12, 399–420. doi: 10.1006/fsim.2001.0408
House, M., Bartholomew, J., Winton, J., Fryer, J. (1999). Relative Virulence of Three Isolates of Piscirickettsia Salmonis for Coho Salmon Oncorhynchus Kisutch. Dis. Aquat. Organ 35, 107–113. doi: 10.3354/dao035107
Kuzyk, M. A., Thorton, J. C., Kay, W. W. (1996). Antigenic Characterization of the Salmonid Pathogen Piscirickettsia Salmonis. Infect. Immun. 64, 5205–5210. doi: 10.1128/iai.64.12.5205-5210.1996
Mancilla, M., Saavedra, J., Grandon, M., Tapia, E., Navas, E., Grothusen, H., et al. (2018). The Mutagenesis of a Type IV Secretion System Locus of Piscirickettsia Salmonis Leads to the Attenuation of the Pathogen in Atlantic Salmon, Salmo Salar. J. Fish Dis. 41, 625–634. doi: 10.1111/jfd.12762
Marshall, S. H., Gomez, F. A., Ramirez, R., Nilo, L., Henriquez, V. (2012). Biofilm Generation by Piscirickettsia Salmonis Under Growth Stress Conditions: A Putative In Vivo Survival/Persistence Strategy in Marine Environments. Res. Microbiol. 163, 557–566. doi: 10.1016/j.resmic.2012.08.002
Moradali, M. F., Rehm, B. H. A. (2020). Bacterial Biopolymers: From Pathogenesis to Advanced Materials. Nat. Rev. Microbiol. 18, 195–210. doi: 10.1038/s41579-019-0313-3
Naves, P., Del Prado, G., Huelves, L., Gracia, M., Ruiz, V., Blanco, J., et al. (2008). Measurement of Biofilm Formation by Clinical Isolates of Escherichia Coli is Method-Dependent. J. Appl. Microbiol. 105, 585–590. doi: 10.1111/j.1365-2672.2008.03791.x
Norstebo, S. F., Lotherington, L., Landsverk, M., Bjelland, A. M., Sorum, H. (2018). Aliivibrio Salmonicida Requires O-Antigen for Virulence in Atlantic Salmon (Salmo Salar L.). Microb. Pathog. 124, 322–331. doi: 10.1016/j.micpath.2018.08.058
Ortiz-Severin, J., Travisany, D., Maass, A., Cambiazo, V., Chavez, F. P. (2020). Global Proteomic Profiling of Piscirickettsia Salmonis and Salmon Macrophage-Like Cells During Intracellular Infection. Microorganisms 8, 1845. doi: 10.3390/microorganisms8121845
Pal, S., Verma, J., Mallick, S., Rastogi, S. K., Kumar, A., Ghosh, A. S. (2019). Absence of the Glycosyltransferase WcaJ in Klebsiella Pneumoniae ATCC13883 Affects Biofilm Formation, Increases Polymyxin Resistance and Reduces Murine Macrophage Activation. Microbiol. (Reading) 165, 891–904. doi: 10.1099/mic.0.000827
Perez-Stuardo, D., Morales-Reyes, J., Tapia, S., Ahumada, D. E., Espinoza, A., Soto-Herrera, V., et al. (2019). Non-Lysosomal Activation in Macrophages of Atlantic Salmon (Salmo Salar) After Infection With Piscirickettsia Salmonis. Front. Immunol. 10, 434. doi: 10.3389/fimmu.2019.00434
Pfaffl, M. W. (2001). A New Mathematical Model for Relative Quantification in Real-Time RT-PCR. Nucleic Acids Res. 29, e45. doi: 10.1093/nar/29.9.e45
Pulgar, R., Hodar, C., Travisany, D., Zuniga, A., Dominguez, C., Maass, A., et al. (2015). Transcriptional Response of Atlantic Salmon Families to Piscirickettsia Salmonis Infection Highlights the Relevance of the Iron-Deprivation Defence System. BMC Genomics 16, 495. doi: 10.1186/s12864-015-1716-9
Quandt, J., Hynes, M. F. (1993). Versatile Suicide Vectors Which Allow Direct Selection for Gene Replacement in Gram-Negative Bacteria. Gene 127, 15–21. doi: 10.1016/0378-1119(93)90611-6
Raetz, C. R., Whitfield, C. (2002). Lipopolysaccharide Endotoxins. Annu. Rev. Biochem. 71, 635–700. doi: 10.1146/annurev.biochem.71.110601.135414
Rautemaa, R., Meri, S. (1999). Complement-Resistance Mechanisms of Bacteria. Microbes Infect. 1, 785–794. doi: 10.1016/s1286-4579(99)80081-1
Reid, A. N., Szymanski, C. M. (2010). “Biosynthesis and Assembly of Capsular Polysaccharides” in Microbial Glycobiology. Eds. Holst, O., Brennan, P. J., Von Itzstein, M., Moran, A. (Academic Press), 351–373. doi: 10.1016/B978-0-12-374546-0.00020-1
Renzi, F., Ittig, S. J., Sadovskaya, I., Hess, E., Lauber, F., Dol, M., et al. (2016). Evidence for a LOS and a Capsular Polysaccharide in Capnocytophaga Canimorsus. Sci. Rep. 6, 38914. doi: 10.1038/srep38914
Rozas, M., Enriquez, R. (2014). Piscirickettsiosis and Piscirickettsia Salmonis in Fish: A Review. J. Fish Dis. 37, 163–188. doi: 10.1111/jfd.12211
Saavedra, J., Grandon, M., Villalobos-Gonzalez, J., Bohle, H., Bustos, P., Mancilla, M. (2018). Isolation, Functional Characterization and Transmissibility of P3ps10, a Multidrug Resistance Plasmid of the Fish Pathogen Piscirickettsia Salmonis. Front. Microbiol. 9, 923. doi: 10.3389/fmicb.2018.00923
Saavedra, J., Hernandez, N., Osses, A., Castillo, A., Cancino, A., Grothusen, H., et al. (2017). Prevalence, Geographic Distribution and Phenotypic Differences of Piscirickettsia Salmonis EM-90-Like Isolates. J. Fish Dis. 40, 1055–1063. doi: 10.1111/jfd.12581
Samuel, G., Reeves, P. (2003). Biosynthesis of O-Antigens: Genes and Pathways Involved in Nucleotide Sugar Precursor Synthesis and O-Antigen Assembly. Carbohydr. Res. 338, 2503–2519. doi: 10.1016/j.carres.2003.07.009
Sánchez, P., Oliver, C., Hernández, M., Cortés, M., Rauch, M. C., Valenzuela, K., et al. (2018). In Vitro Genomic and Proteomic Evidence of a Type IV Pili-Like Structure in the Fish Pathogen Piscirickettsia Salmonis. FEMS Microbiol. Lett. 365. doi: 10.1093/femsle/fny169
Sandoval, R., Oliver, C., Valdivia, S., Valenzuela, K., Haro, R. E., Sanchez, P., et al. (2016). Resistance-Nodulation-Division Efflux Pump acrAB Is Modulated by Florfenicol and Contributes to Drug Resistance in the Fish Pathogen Piscirickettsia Salmonis. FEMS Microbiol. Lett. 363, fnw102. doi: 10.1093/femsle/fnw102
Sepulveda, D., Bohle, H., Labra, A., Grothusen, H., Marshall, S. H. (2013). Design and Evaluation of a Unique RT-qPCR Assay for Diagnostic Quality Control Assessment That Is Applicable to Pathogen Detection in Three Species of Salmonid Fish. BMC Vet. Res. 9, 183. doi: 10.1186/1746-6148-9-183
Simon, R., Priefer, U., Pehle, A. (1983). A Broad Host Range Mobilization System for the In Vitro Genetic Engineering: Transposon Mutagenesis in Gram Negative Bacteria. Nat. Biotechnol. 1, 784–890. doi: 10.1038/nbt1183-784
Stranahan, L. W., Arenas-Gamboa, A. M. (2021). When the Going Gets Rough: The Significance of Brucella Lipopolysaccharide Phenotype in Host-Pathogen Interactions. Front. Microbiol. 12, 713157. doi: 10.3389/fmicb.2021.713157
Tan, Y. H., Chen, Y., Chu, W. H. W., Sham, L. T., Gan, Y. H. (2020). Cell Envelope Defects of Different Capsule-Null Mutants in K1 Hypervirulent Klebsiella Pneumoniae can Affect Bacterial Pathogenesis. Mol. Microbiol. 113, 889–905. doi: 10.1111/mmi.14447
Vadovic, P., Fodorova, M., Toman, R. (2007). Structural Features of Lipid A Piscirickettsia Salmonis, the Etiological Agent of Salmonid Rickettsial Septicemia. Acta Virol. 51, 249–259.
Valenzuela, B., Imarai, M., Torres, R., Modak, B. (2013). Immunomodulatory Effects of the Aromatic Geranyl Derivative Filifolinone Tested by the Induction of Cytokine Expression. Dev. Comp. Immunol. 41, 675–682. doi: 10.1016/j.dci.2013.08.001
Van der Woude, M. W., Baumler, A. J. (2004). Phase and Antigenic Variation in Bacteria. Clin. Microbiol. Rev. 17, 581–611. doi: 10.1128/CMR.17.3.581-611.2004
Vera, T. (2013). Differential Gene Expression of Piscirickettsia Salmonis Strains in Adaptive Response to Different Culture Systems: A Strategy to Identify Possible Intracellular Survival Mechanisms and Pathogenicity (Valdivia, CL: Universidad Austral de Chile).
Vinogradov, E., Frimmelova, M., Toman, R. (2013). Chemical Structure of the Carbohydrate Backbone of the Lipopolysaccharide From Piscirickettsia Salmonis. Carbohydr. Res. 378, 108–113. doi: 10.1016/j.carres.2013.04.010
Yi, E. C., Hackett, M. (2000). Rapid Isolation Method for Lipopolysaccharide and Lipid A From Gram-Negative Bacteria. Analyst 125, 651–656. doi: 10.1039/b000368i
Yu, M., Xu, Y., Xu, T., Wang, B., Sheng, A., Zhang, X. (2015). WcaJ, the Initiating Enzyme for Colanic Acid Synthesis, is Required for Lipopolysaccharide Production, Biofilm Formation and Virulence in Edwardsiella Tarda. Aquaculture 437, 287–291. doi: 10.1016/j.aquaculture.2014.12.011
Keywords: Piscirickettsia salmonis, piscirickettsiosis, lipopolysaccharide, virulence, Salmo salar, SRS, biofilm
Citation: Herrera V, Olavarría N, Saavedra J, Yuivar Y, Bustos P, Almarza O and Mancilla M (2022) Complete Lipopolysaccharide of Piscirickettsia salmonis Is Required for Full Virulence in the Intraperitoneally Challenged Atlantic Salmon, Salmo salar, Model. Front. Cell. Infect. Microbiol. 12:845661. doi: 10.3389/fcimb.2022.845661
Received: 30 December 2021; Accepted: 21 February 2022;
Published: 18 March 2022.
Edited by:
Pamela Ruiz, University of Concepcion, ChileReviewed by:
Juan Pablo Pontigo, San Sebastián University, ChileJavier Santander, Memorial University of Newfoundland, Canada
Copyright © 2022 Herrera, Olavarría, Saavedra, Yuivar, Bustos, Almarza and Mancilla. This is an open-access article distributed under the terms of the Creative Commons Attribution License (CC BY). The use, distribution or reproduction in other forums is permitted, provided the original author(s) and the copyright owner(s) are credited and that the original publication in this journal is cited, in accordance with accepted academic practice. No use, distribution or reproduction is permitted which does not comply with these terms.
*Correspondence: Oscar Almarza, b3NjYXJhbG1hcnphQGdtYWlsLmNvbQ==; Marcos Mancilla, bW1hbmNpbGxhQGFkbGRpYWdub3N0aWMuY2w=
†These authors have contributed equally to this work and share first authorship