- 1Hainan Key Laboratory for Sustainable Utilization of Tropical Bioresource, College of Tropical Crops, Hainan University, Haikou, China
- 2Sanya Nanfan Research Institute of Hainan University, Hainan Yazhou Bay Seed Laboratory, Sanya, China
Multiunit-flavoenzyme NADPH oxidases (NOXs) play multiple roles in living cells via regulating signaling pathways. In several phytopathogenic fungi, NOXs are required for the polarized growth of hyphal tips and pathogenicity to host plants, but the possible mechanisms are still elusive. In our previous study, CgNOXA, CgNOXB, and CgNOXR were identified as components of the NOX complex in Colletotrichum gloeosporioides. The growth and the inoculation assays revealed that CgNOXA/B and CgNOXR regulate vegetative growth and are required for the full pathogenicity of C. gloeosporioides to Hevea leaves. We further demonstrated that the vital roles of CgNOXB and CgNOXR in appressorium formation and the development of invasion hyphae account for their functions in pathogenicity. Moreover, CgNOXB and CgNOXR regulate the production and distribution of ROS in hyphal tips and appressoria, control the specialized remodeling of F-actin in hyphal tips and appressoria, and are involved in fungal cell wall biosynthesis. Taken together, our findings highlight the role of NOXs in fungal pathogenicity through the organization of the actin cytoskeleton.
Introduction
NADPH oxidases (NOXs) are membrane-associated, multiunit flavoenzymes widely present in eukaryotes. The enzymes catalyze the reduction of molecular oxygen to superoxide anion (O2•-) by transferring electrons across biological membranes using NADPH as an electron donor (Lambeth, 2004; Sumimoto, 2008). NOXs were firstly identified and known as the source of the phagocyte respiratory burst; however, in the past decades, NOXs and the reactive oxygen species (ROS) they produced have been involved in many signaling pathways (Brown and Griendling, 2009; Suzuki et al., 2011). In animal cells, a series of members of the NOX family and several regulatory subunits have been identified; these proteins are implicated in cell proliferation, cell signaling, and apoptosis (Lambeth, 2004; Sumimoto, 2008; Brown and Griendling, 2009). Plant NOXs, also known as respiratory burst oxidase homologs (RBOHs), belong to a small multigenic family (Marino et al., 2012); these enzymes play multiple roles in environmental stress response (Torres et al., 2002), plant immunity (Kadota et al., 2015), programmed cell death (Suzuki et al., 2011), and polarized growth of root hairs (Foreman et al., 2003). In filamentous fungi, several NOX isoforms and regulatory components have been identified (Heller and Tudzynski, 2011; Takemoto et al., 2011; Siegmund et al., 2015). Fungal NOXs are necessary for hyphal growth, sexual reproduction, developmental processes such as the formation of appressoria, and virulence (Egan et al., 2007; Takemoto et al., 2007; Cano-Domínguez et al., 2008; Nordzieke et al., 2019). During these developmental processes, the NOX-derived ROS plays a vital role in regulating transitions from non-polarized to polarized cell growth (Egan et al., 2007; An et al., 2016). However, the underlying signaling pathway by which NOXs regulate the polarized growth remains unclear.
The cytoskeleton is a highly organized and dynamic network that exists in all eukaryotic cells; it is composed of microfilaments, microtubules, and intermediate filaments. Of the three cytoskeletal subclasses, microfilaments, made up of linear actin polymers called F-actin, are the most dynamic. The monomeric globular actin (G-actin) could assemble into filaments, and two parallel filaments form a double helix, known as F-actin. This polymerized F-actin usually assembles to elongate at one end called barbed ends and disassemble to shorten at the opposite end called pointed ends. In fungi, three higher-order F-actin structures were firstly found in S. cerevisiae: patches, cables, and rings (Adams and Pringle, 1984; Kilmartin and Adams, 1984). Lately, these three F-actin structures were also identified in the filamentous fungus Aspergillus nidulans (Araujo-Bazán et al., 2008; Berepiki et al., 2011). Actin patches are mainly accumulated in Spitzenkörper (Spk), which is located at subapical regions of hyphal tips (Riquelme and Sánchez-León, 2014); the localization of actin patches indicates their functions in endocytosis and exocytosis and involvement in hyphal tip growth (Shaw et al., 2011; Takeshita et al., 2014). Actin cables are bundles of F-actin crosslinked by tropomyosin and fimbrin (Evangelista et al., 2002); they serve as tracks for the organelle transport and secretory vesicles (Taheri-Talesh et al., 2008; Berepiki et al., 2011; Taheri-Talesh et al., 2012), whereas actin rings participate in septum formation and are required for pathogenesis in Magnaporthe oryzae (Ryder et al., 2013; González-Rodríguez et al., 2016; Dulal et al., 2021).
Colletotrichum gloeosporioides is a notorious phytopathogenic fungus that infects over 470 plant species and causes anthracnose diseases in both aerial plant parts and the postharvest fruits (Dean et al., 2012). In our previous work, two NOX components CgNOXA and CgNOXB and a regulatory protein CgNOXR were identified in C. gloeosporioides. The pathogenicity assay showed that knockout of either CgNOXB or CgNOXR significantly impaired the pathogenicity of C. gloeosporioides (Guo and An, 2018). In this study, we set out to investigate the possible mechanism of NOX in the regulation of pathogenicity, and the results showed that CgNOXB and CgNOXR are required for the polarization of actin organization in the hyphal tip, cell wall component deposition, and appressorium formation. These findings highlight the role of NOX in pathogenicity through the organization of the F-actin network.
Material and Method
Fungal Strains and Culture Conditions
Colletotrichum gloeosporioides from Hevea was isolated and kept previously (BioSample: SAMN17266943). Knockout mutants ΔCgnoxA, ΔCgnoxB, and ΔCgnoxR were constructed in our previous study (Guo and An, 2018). The strains were kept on potato agar (PDA) or cultured in a liquid medium. For the microscope analysis, conidia were cultured on Yeast Casein Sucrose (YCS) medium (1 g l-1 yeast extract, 1 g l-1 acid-hydrolyzed casein, 2% w/v sucrose, pH 6.9).
Construction of the Double Mutant, Complementation, and Actin-Labeled Strains
To generate a double mutant of CgnoxA and CgnoxB, the ΔCgnoxA strain was used as the recipient strain in which CgnoxB was knocked out using a split-marker strategy as described in our established protocol. Briefly, the flanking sequences of CgnoxB were amplified and fused with the split fragments of the neomycin phosphotransferase gene (NPTII) which confers resistance to Geneticin (G418) (Thermo Fisher, Waltham, MA, USA). Then the two recombinant fragments were co-transformed into protoplasts of the ΔCgnoxA strain for the gene knockout.
To generate the complementation strain, the vector pMD-PgTt which contains the terminator of trpC from A. nidulans and the hygromycin phosphotransferase gene (HPT) was used. The nucleotide sequences of Cgnox genes together with their native promoters were amplified and ligated into the vector pMD-PgTt, respectively. Then, the plasmids were linearized before the protoplast transformation. Positive complementation strains were named as Res-ΔCgnoxA, Res-ΔCgnoxB, and Res-ΔCgnoxR.
To label the actin structure, the Lifeact-EGFP-expressing strain was used as the recipient strain, and CgnoxA, CgnoxB, and CgnoxR were knocked out respectively as described previously (Guo and An, 2018). In addition, the double-mutant strains of CgnoxA and CgnoxB were generated as described above. Protoplast preparation and transformation were performed as described in our established protocol (Wang et al., 2018). The primers that were used are listed in Supplementary Table S1.
Colony Growth Assay
For the colony growth assay, disks of mycelium with a diameter of 0.5 cm were inoculated onto the PDA medium (2 g l-1 NaNO3, 0.5 g l-1 KCl, 1 g l-1 KH2PO4, 0.5 g l-1 MgSO4·7H2O, 0.01 g l-1 FeSO4·7H2O, pH 6.9), and colony morphology and diameter were recorded. Each strain contained three replicates, and all of the experiments were performed twice.
Pathogenicity Assay
The pathogenicity assay was carried out as described in our previous report (Gao et al., 2022). Briefly, conidia were collected, washed two times with ddH2O, and resuspended in a solution of 0.5% Sabouraud Maltose Broth (Difco, Franklin Lakes, NJ, USA) to a final concentration of 2 × 105 conidia ml−1. The detached leaves from rubber tree variety 73-3-97 were used for inoculation. The leaves were divided into two groups, with one group of leaves being pre-wounded with a sterile needle and the other group without being wounded. Then, droplets (5 μl) of the conidial suspensions were inoculated onto the leaves. The leaves were kept in a moist chamber at 28°C under natural illumination for 4 days, and the disease symptoms were recorded. Each treatment contained three replicates of 10 leaves, and the entire experiment was repeated three times.
Appressorium Formation Assay
For the calculation of the appressorium formation ratio, conidia resuspended with ddH2O at a concentration of 5 × 105 conidia ml-1 were incubated on hydrophobic plastic plates. After incubation for 12 and 24 h, the germination behavior was observed using Leica DM2000 microscopy. For penetration assays, the conidium droplets (3 × 105 conidia ml-1) were inoculated on the onion epidermis that was plated on water agar plates. After incubation for 16 h, the infection structures were observed using Leica DM2000 microscopy. Each treatment contained three replications, and the entire experiment was conducted twice.
Quantitative RT-PCR Analysis
For the RNA extraction from vegetative mycelia, the conidial suspension was inoculated into the liquid complete medium and cultured at 120 rpm, 28°C, for 2 days. Then, the mycelium was collected, disrupted in liquid nitrogen by grinding in a mortar with a pestle, and used for RNA extraction. For the RNA extraction from appressoria, conidia suspension in ddH2O at a concentration of 1 × 106 conidia ml-1 was incubated on polyester; after incubation for 24 h, appressoria were collected by an RNase-free scraper and used for RNA extraction. For RNA extraction from infectious mycelia, the conidial suspension was inoculated on rubber leaves and incubated for 3 days, then the lesion area was cut from the leaves, disrupted in liquid nitrogen, and used for RNA extraction. The RNA was extracted using the RNAprep Pure Plant Plus Kit (TIANGEN Biotech, Beijing, China). For cDNA synthesis, 1 μg of total RNA from different samples was used for reverse transcription with FastKing gDNA Dispelling RT SuperMix (TIANGEN Biotech, Beijing, China) according to the manufacturer’s recommendations. To analyze the transcription levels of CgnoxA, CgnoxB, and CgnoxR during different stages, a quantitative real-time PCR was performed with QuantStudio 6 (Thermo Fisher, Waltham, MA, USA) in a 20-μl reaction volume using ChamQ SYBR Color qPCR Master Mix (Vazyme, Nanjing, China). The expression levels of chitin synthase genes in the mycelium of mutant strains were analyzed as demonstrated above. The β2-tubulin-coding gene was used as an endogenous control for normalization, and relative expression levels were estimated using the 2-ΔΔCt method (Livak and Schmittgen, 2001). The primers are listed in Supplementary Table S1.
ROS Detection
For DAB staining, conidium droplets (3 × 105 conidia ml-1) were inoculated on the onion epidermis that was plated on water agar plates. After incubation for 12 h, the infection structures were stained with 1 mg/ml DAB (pH3.8, 30 μl) for 12 h under darkness, then the accumulation of ROS was observed with Leica DM2000 microscopy and the average optical density (AOD) of dark-brown polymers were quantified using ImageJ software. For AOD quantification, all the images were changed to eight-bit type at first. Then we selected “Area” and “Integrity density” in “Set Measurements.” After clicking “Calibrate” in “Analyze,” we selected “Uncalibrated OD” in “Function,” and then the gray value 255 equals OD value 0, and gray value 0 becomes OD value 2.71. Each strain contained three replications, and at least 60 appressoria were measured for each replicate.
For visualization of O2•- production, the conidial suspension was inoculated on a YCS medium-coated glass slide and incubated in a moist chamber at 28°C of 5 h before being stained with 0.05% (w/v) NBT aqueous solution for 10 min. Then the O2•- production of hyphae was observed by Leica DM2000 microscopy. In order to analyze the distribution of formazan precipitate in the top of germinated hyphae of different strains, the AOD in two different zones in the hyphal tip was calculated as follows: apex and subapex 7 μm, 0–7 μm from the tip; and shank 8 μm, 7–15 μm from the tip. For each strain, more than 10 hyphae were measured in each experiment, and the entire experiment was conducted twice.
Investigation of the Actin Filament Structure by Confocal Microscopy
To investigate the actin filament structure in germinated hypha, conidial suspensions of WT, ΔCgnoxA, ΔCgnoxB, ΔCgnoxR, and ΔCgnoxAnoxB strains expressing Lifeact-EGFP (5 × 105 conidia ml-1) were incubated on YCS-coated glass slides for 5 h before observation under the confocal. To investigate the actin filament structure in appressoria, conidial suspensions of each strain (2 × 105 conidia ml-1) were incubated on the hydrophobic borosilicate glass coverslips (Thermo Fisher, Waltham, MA, USA) for 24 h before observation. For diphenyleneiodonium (DPI) treatment, the DPI solution was added into conidial suspension to the concentration of 40 μmol l-1. For the microscope analysis, the tip of germinated hypha and appressorium were captured through the Leica TCS SP8 laser scanning confocal microscope, with excitation of 488-nm argon laser and emission wavelength range of 505–525 nm. The projection of z-stack images was performed with ImageJ (http://rsbweb.nih.gov/ij/, version 1.47g). To compare the distribution of actin filaments in the top of germinated hyphae of different strains, the fluorescence intensity in three different zones in the hyphal tip was calculated as follows: apex 2 μm, 0–2 μm from the tip; subapex 5 μm, 2–7 μm from the tip; and shank 8 μm, 7–15 μm from the tip. For each strain, more than 10 hyphae were measured in each experiment, and the entire experiment was conducted twice.
Calcofluor White Staining
For staining with Calcofluor white (CFW), hyphae that incubated on YCS-coated glass slides as mentioned above were stained with a 10-μg ml-1 CFW aqueous solution (Sigma-Aldrich, Merck, USA) for 10 min in the dark. The fluorescence was imaged via the Leica TCS SP8 laser scanning confocal microscope, with excitation of 405-nm UV laser and emission wavelength range of 430–460 nm. The projection of z-stack images was performed with ImageJ (http://rsbweb.nih.gov/ij/, version 1.47g). Quantification of the fluorescent intensity was performed by measuring the mean gray value using ImageJ software. For each strain, more than 10 hyphae were measured in each experiment, and the entire experiment was conducted twice.
Protoplast Release Assay
Conidial suspensions were inoculated into 100 ml liquid complete medium to the initial concentration of 105 conidia ml-1. After incubation at 120 rpm, 28°C, for 16 h, mycelium was collected by miracloth, washed with ddH2O, and drained with filter paper. Then, 0.2 g mycelium was incubated in 10 ml Glucanex solution at 100 rpm, 28°C for 3 h. Then protoplasts were collected by filter with miracloth, and the concentration was measured with a hemocytometer under a microscope. Each strain contained three replications.
Statistical Analysis
Statistical significance analyses were performed in PASW Statistics (IBM, USA). Data with a single variable were analyzed by one-way ANOVA, and mean separations were performed by Duncan’s multiple-range test. Differences at P < 0.05 were considered significant.
Results
CgNOXs Are Involved in Vegetative Growth and Pathogenicity
To investigate whether CgNOXs are involved in vegetative growth, the mutant strains were cultured on PDA medium and the colony growth was recorded. After culture for 5 days, all the mutants showed a similar colony morphology to WT. The colony diameters of ΔCgnoxA and ΔCgnoxB were nearly the same as those of WT, while those of ΔCgnoxR and ΔCgnoxAnoxB were slightly decreased compared with WT (Figure 1). These results suggested that the CgNOX complex is required for vegetative growth.
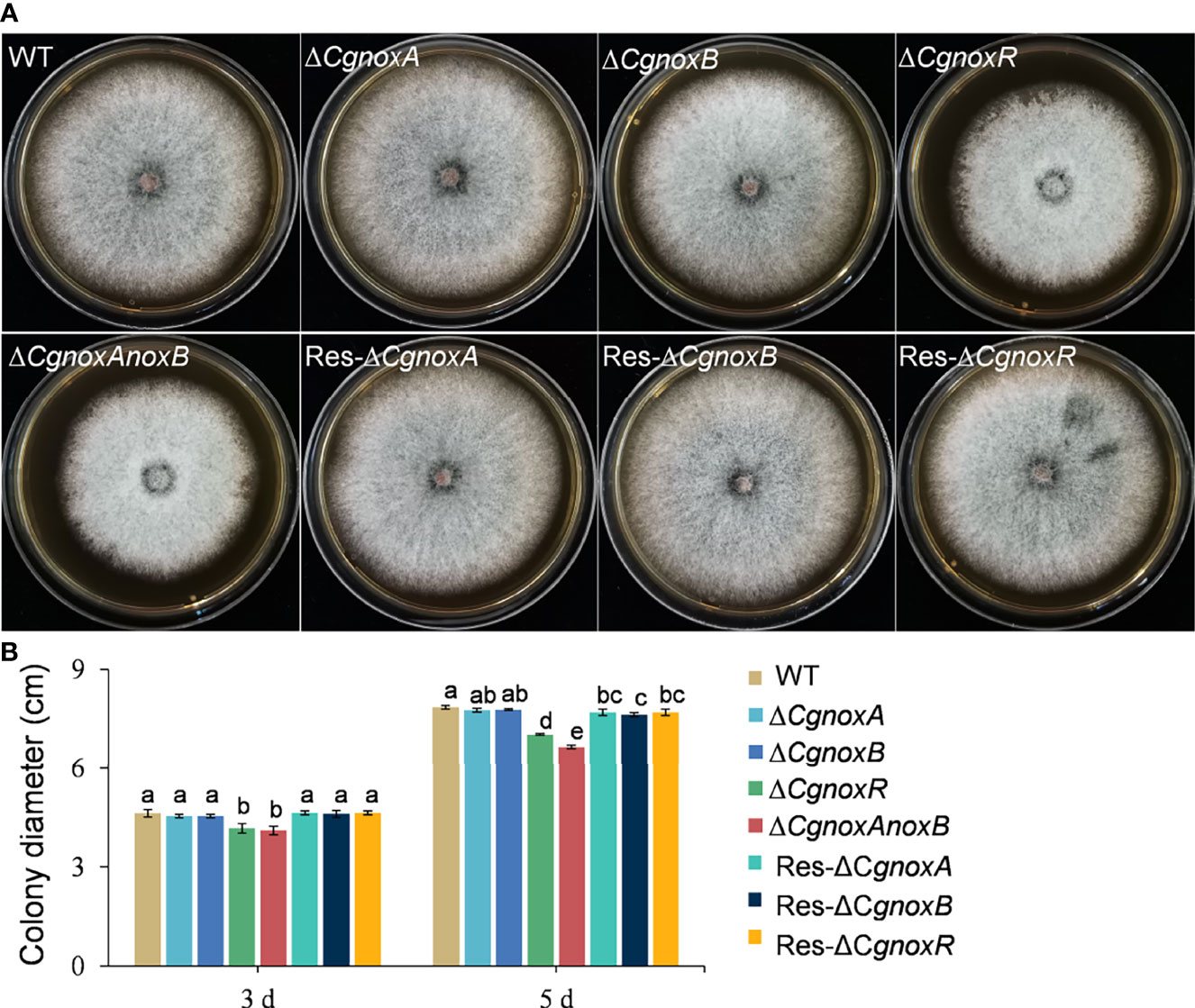
Figure 1 Colony morphology and diameter of C. gloeosporioides strains cultured on PDA medium. (A) Colony morphology after growth on PDA for 5 days. (B) Colony diameter after growth on PDA for 3 and 5 days. Bars represent standard deviations (SD).
The roles of CgNOXA, CgNOXB, and CgNOXR in the pathogenicity of C. gloeosporioides were investigated via inoculation assay on detached leaves with or without wounds. The results showed that when inoculated on the leaf wounds, all the mutants successfully infect the leaves and developed typical anthracnose lesions. However, the lesions caused by ΔCgnoxB, ΔCgnoxR, and ΔCgnoxAnoxB were smaller than those of WT and ΔCgnoxA (Figures 2A–C). Moreover, when inoculated on the intact leaves, the disease incidence of ΔCgnoxR was significantly decreased compared with that of WT and ΔCgnoxA, whereas ΔCgnoxB and ΔCgnoxAnoxB could not even infect the leaves at all (Figures 2D–F). These results revealed that CgNOXB and CgNOXR play vital roles in the pathogenicity and especially the penetration ability of C. gloeosporioides to host plants.
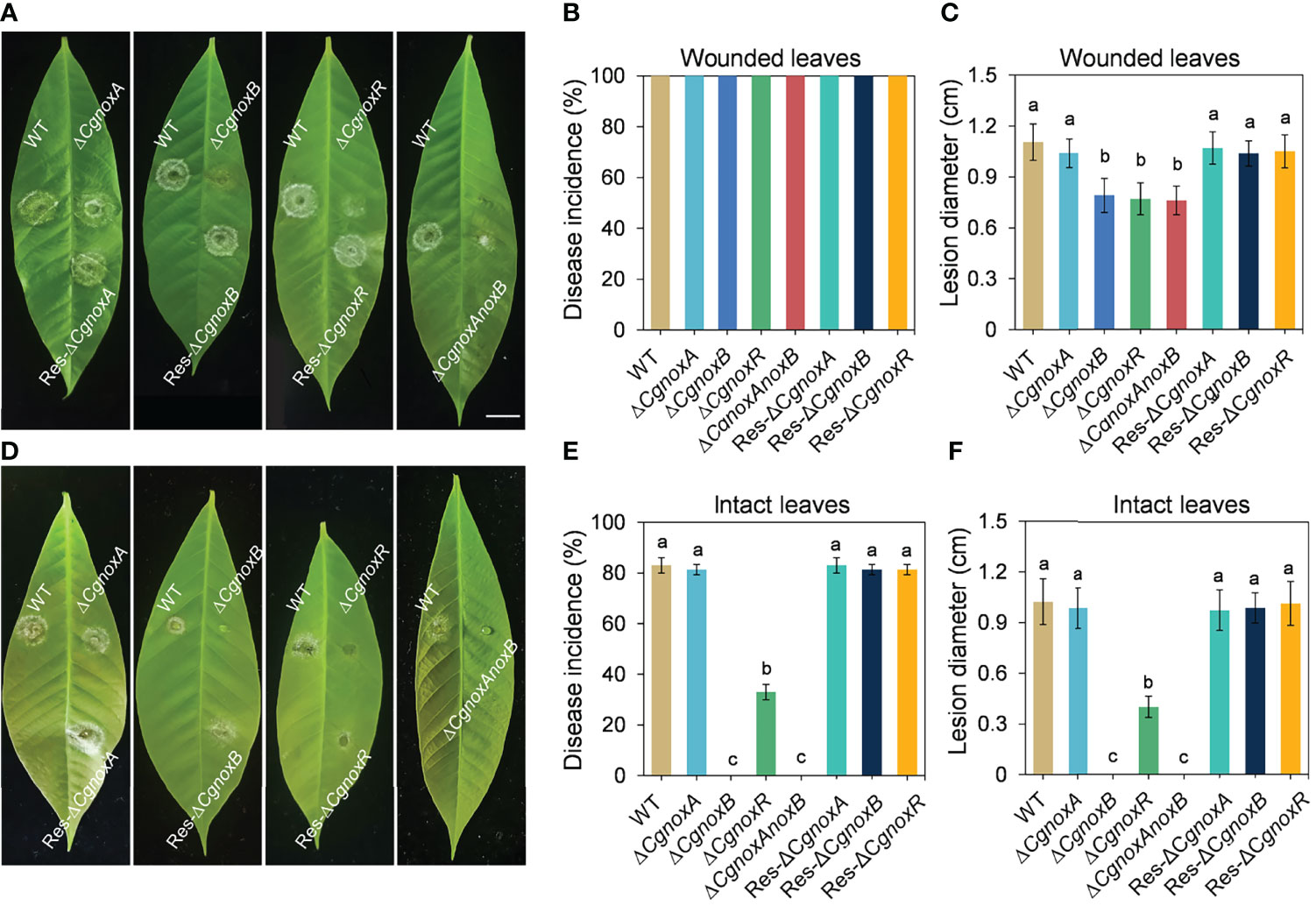
Figure 2 Pathogenicity assay of WT, ΔCgnoxA, ΔCgnoxB, ΔCgnoxR, ΔCgnoxAnoxB, Res-ΔCgnoxA, Res-ΔCgnoxB, and Res-ΔCgnoxR strains on rubber tree leaves. (A) Disease symptoms of pre-wounded rubber tree leaves at 4 day postinoculation (dpi). Scale bar = 1 cm. (B) Disease incidence of WT and mutant strains on pre-wounded leaves after 4 dpi. Values represent mean ± SD. (C) Mean lesion diameters on pre-wounded leaves after 4 dpi. Values represent mean ± SD. (D) Disease symptoms of intact rubber tree leaves at 4 day postinoculation (dpi). (E) Disease incidence of WT and mutant strains on intact leaves after 4 dpi. Values represent mean ± SD. (F) Mean lesion diameters on intact leaves after 4 dpi. Values represent mean ± SD. Columns with different letters indicate significant difference (p < 0.05).
Expression Patterns of CgNOXA, CgNOXB, and CgNOXR
The expression patterns of CgnoxA, CgnoxB, and CgnoxR during vegetative growth in vitro, appressorium formation, and colonization in plant leaves were investigated via a qRT-PCR assay. The results (Figure 3) revealed that in appressoria, the transcription level of CgnoxB was about 2.5-fold higher than that during in vitro growth and in vivo colonization, whereas the transcription levels of CgnoxA and CgnoxR were about 0.5-fold lower than in the other stages. Meanwhile, the expressions of the three genes during in vivo colonization were all down-regulated compared with that during in vitro growth. These results enlightened the role of CgNOXB in the appressorium formation of C. gloeosporioides.
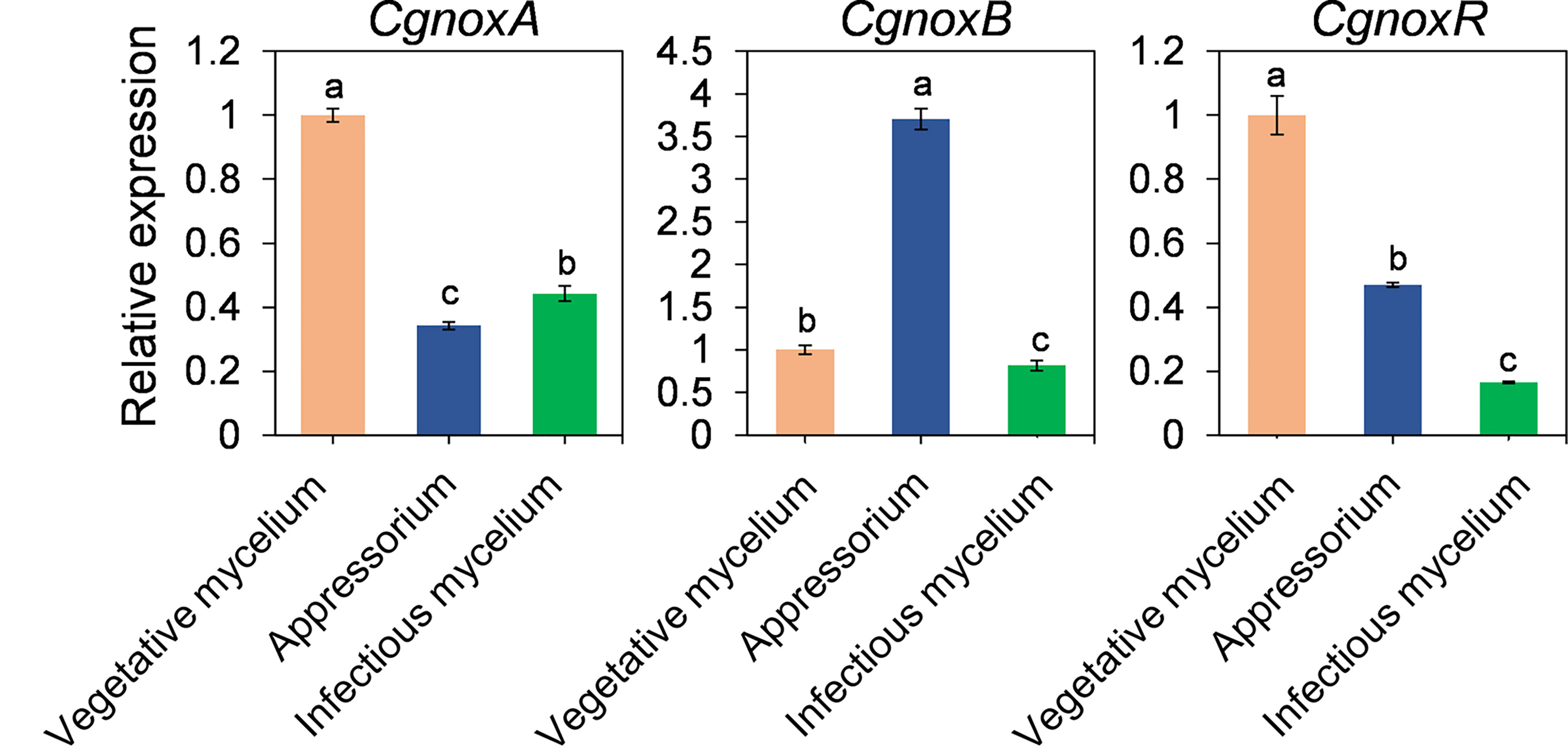
Figure 3 Quantitative RT-PCR of transcript of CgNoxA, CgNoxB, and CgNoxR. Bars represent standard deviations (SD). Columns with different letters indicate significant difference (p < 0.05).
CgNOXB and CgNOXR Regulate Appressorium and Penetration Peg Formation
To explore the roles of NOX in appressorium formation, the germination rates and appressorium formation of the mutants were investigated. The mutants were cultured on hydrophobic plastic plates to induce appressorium formation. After incubation for 12 h, over 80% conidia of WT formed typical appressoria, whereas ΔCgnoxA, ΔCgnoxB, ΔCgnoxR, and ΔCgnoxAnoxB showed decreases in appressorium formation, with about 73.6%, 51.7%, 47.6%, and 52.8%, respectively. After incubation for 24 h, approximately 82.3% and 75.0% conidia from WT and ΔCgnoxA formed appressoria; in comparison, the rates of ΔCgnoxB, ΔCgnoxR, and ΔCgnoxAnoxB were only 52.8%, 65.5%, and 57.7%, respectively (Figures 4A, B). The results revealed that CgNOXs are required for appressorium development.
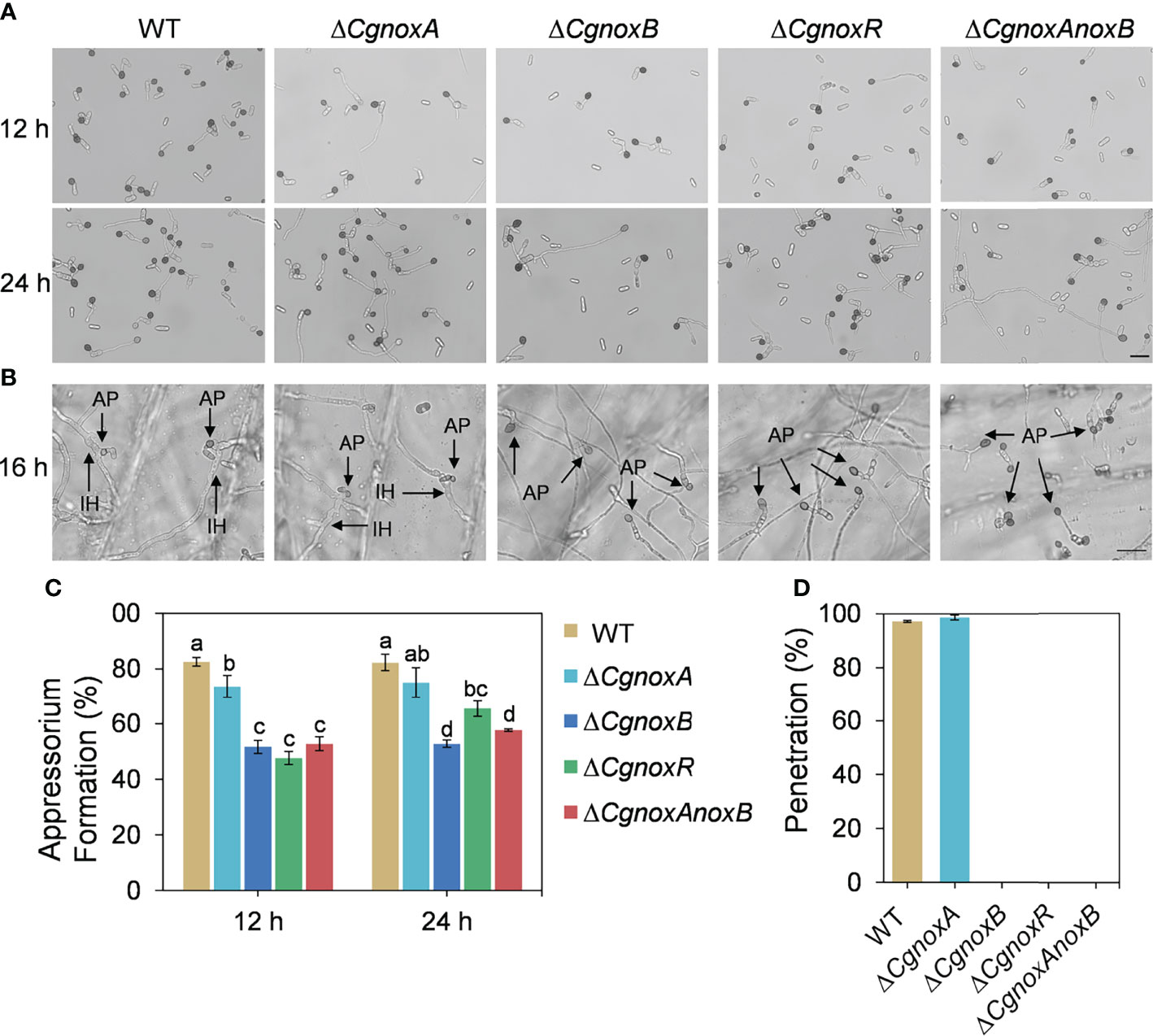
Figure 4 Appressorium formation on hydrophobic plastic plates and onion epidermis. (A) Appressorium formation assay of WT, ΔCgnoxA, ΔCgnoxB, ΔCgnoxR, and ΔCgnoxAnoxB strains incubation on hydrophobic plastic plates for 12 and 24 h. Scale bar = 20 μm. (B) Appressorium formation and penetration on onion epidermis after 16 h. AP: appressorium, IH: invasive hypha. Scale bar = 20 μm. (C) Appressorium formation rate at 12 and 24 h postinoculation. Bars represent standard deviations (SD). Columns with different letters indicate significant difference (p < 0.05). (D) Bar charts show the relative percentage penetration of each strain on onion epidermis at 24 hpi, assessed by recording the frequency of hyphal penetration from an appressorium (three biological replicas).
The formation of penetration peg was further investigated by incubation of the mutants on the onion epidermis. The result (Figures 4C, D) showed that, after incubation for 16 h, most of the appressoria of WT and ΔCgnoxA formed typical invasive hyphae (also named primary hyphae) and successfully penetrated the plant tissue. By contrast, appressoria of ΔCgnoxB, ΔCgnoxR, and ΔCgnoxAnoxB failed to penetrate and invade onion cells. The results suggested that CgNOXB and CgNOXR are required for the penetration ability of C. gloeosporioides to plant.
CgNOXB and CgNOXR Regulate ROS Generation
The appressoria of the mutants were stained with DAB to investigate the ROS generation. After incubation for 24 h on onion epidermis, WT and ΔCgnoxA showed a little accumulation of dark-brown polymers around appressoria. (Figures 5A, B), whereas for the ΔCgnoxB, ΔCgnoxR, and ΔCgnoxAnoxB mutants, the appressoria accumulated an amount of dark-brown polymers, with higher AOD levels than those of WT and ΔCgnoxA (Figures 5A, B). In addition, these three mutants did not form invasive hyphae as mentioned above. To further assess O2•-, the direct product of NOXs, the hyphae of the mutants were stained with NBT. Microscopic observation showed that the WT and ΔCgnoxA accumulated blue formazan precipitate intensively in the apex of hyphal tips (Figures 5C, D), suggesting the polarity distribution of O2•-, whereas for ΔCgnoxB, ΔCgnoxR, and ΔCgnoxAnoxB, the O2•- generation was uniformly distributed in the hyphal tips.
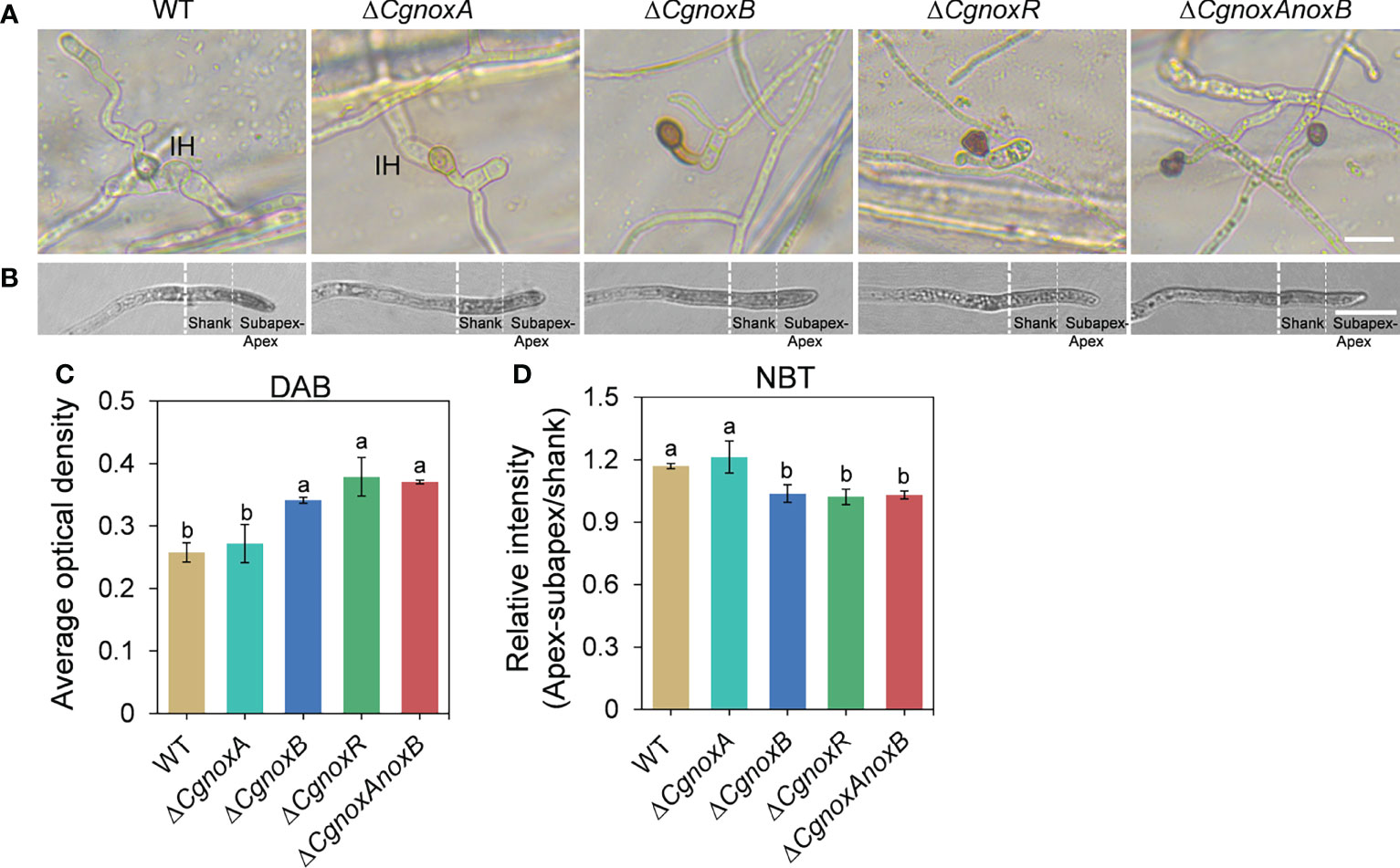
Figure 5 CgNOX regulates reactive oxygen species (ROS) in C. gloeosporioides. (A) DAB staining of WT, ΔCgnoxA, ΔCgnoxB, ΔCgnoxR, and ΔCgnoxAnoxB strains after inoculation on onion epidermis for 12 h. Scale bar = 10 μm. (B) Germinated conidia of WT, ΔCgnoxA, ΔCgnoxB, ΔCgnoxR, and ΔCgnoxAnoxB strains were stained with nitro blue tetrazolium chloride (NBT) and viewed under light microscopy. White dotted lines indicate the regions for the measurement. Scale bar = 10 μm. (C) The deposition of dark brown polymers in appressorium was analyzed using ImageJ software. Bar chart showing AOD (average optical density) of each strain. Bars represent standard deviations (SD). Columns with different letters indicate significant difference (p < 0.05). At least 60 appressoria from each strain were analyzed. (D) The AOD of formazan precipitate of apex–subapex and shank in the hyphal tips were quantified through ImageJ software. Bar chart showing the ratio of AOD of apex–subapex to shank. Bars represent standard deviations (SD). Columns with different letters indicate significant difference (p < 0.05). At least 10 hyphae from each strain were analyzed.
CgNOXB and CgNOXR Regulate the Organization of F-Actin
In our previous work, a widely used Lifeact-EGFP gene fusion was introduced into C. gloeosporioides to observe the organization of F-actin by live-cell imaging (Liu et al., 2021). To understand the roles of CgNOXs in F-actin organization, we then generated the ΔCgnoxA, ΔCgnoxB, and ΔCgnoxR and ΔCgnoxAnoxB mutants that express Lifeact-EGFP. Then the F-actin structure in mutants was investigated.
In the conidia of WT and the mutants, F-actin showed a typical filamentous network and patches, suggesting that the knockout of NOX genes did not influence the F-actin network in conidia (Figure S1). In hyphal tips of WT and ΔCgnoxA, F-actin showed a polarized distribution with patches and cable structures (Figure 6A), whereas in ΔCgnoxB, ΔCgnoxR, and ΔCgnoxAnoxB mutants, this kind of organized distribution was diminished (Figure 6A). To quantitate this polarity distribution of F-actin, the hyphal tips were divided into three regions of apex, subapex, and shank, and the relative fluorescence intensity was calculated. The results were in accordance with that of the microscope observation, revealing that WT and ΔCgnoxA employed a higher intensity at the apex and subapex regions than in the shank region, while ΔCgnoxB, ΔCgnoxR, and ΔCgnoxAnoxB employed uniform intensity all through the hyphal tips (Figure 6A). To further verify whether this kind of F-action polarity was mediated by NOXs, the WT strain was treated with DPI, the inhibitor of NOXs. The microscopic analysis showed that DPI led to a decrease in fluorescence intensity in all three zones of hyphal tips; moreover, the polarity of F-actin was also diminished by DPI.
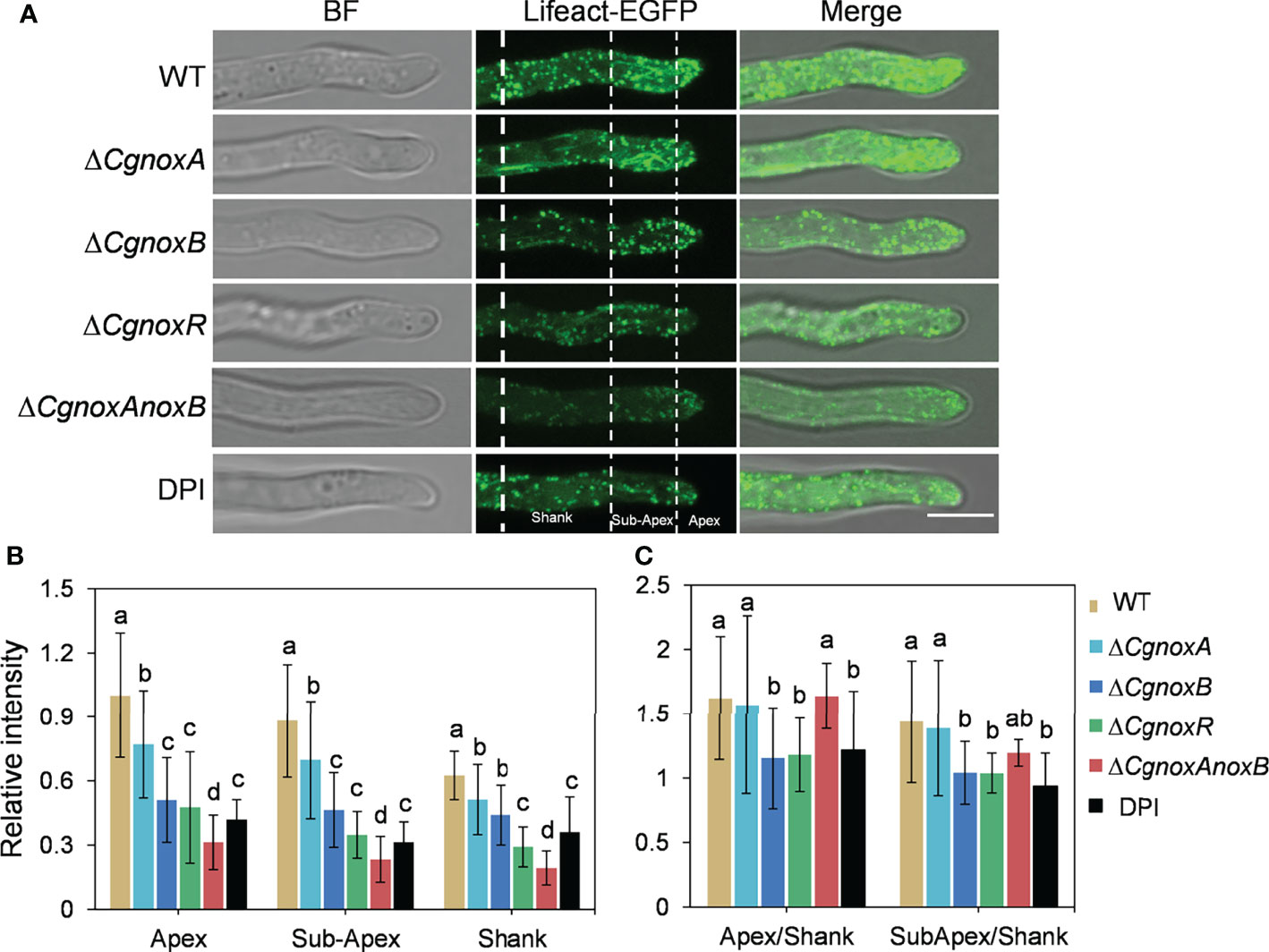
Figure 6 CgNOX regulates the actin filament structure in the tip of the germ tube of C. gloeosporioides. (A) Lifeact-EGFP-labeled actin filament structures in WT (DPI treatment or not), ΔCgnoxA, ΔCgnoxB, ΔCgnoxR, and ΔCgnoxAnoxB strains. The tip of germinated hypha was divided into three zones as apex (0–2 μm from tip), subapex (2–7 μm from tip), and shank (7–15 μm from tip) by white dotted lines. Scale bar = 5 μm. (B) The relative amount of actin filaments in the tip of germinated hypha in WT, ΔCgnoxA, ΔCgnoxB, ΔCgnoxR, and ΔCgnoxAnoxB strains expressing Lifeact-EGFP. The amount of actin filament within the apex, subapex, and shank was measured. The fluorescence intensity of the apex in WT strain was converted to 1, and the relative amount of actin filament was plotted. At least 10 hyphae were measured for each strain. Bars represent standard deviations (SD). Columns with different letters indicate significant difference (p < 0.05). (C) Quantification of the distribution of actin filaments in the tip of germinated hypha in WT (DPI treatment or not), ΔCgnoxA, ΔCgnoxB, ΔCgnoxR and ΔCgnoxAnoxB strains expressing Lifeact-EGFP. At least 10 hyphae were measured for each strain. Bars represent standard deviations (SD). Columns with different letters indicate significant difference (p < 0.05).
Then the F-actin structures in the appressoria were investigated. The results showed that in the appressoria of WT, F-actin was reorganized to a ring structure around the appressorium pore. This ring-shaped F-actin network was also observed in ΔCgnoxA (Figure 7A), whereas in ΔCgnoxB, ΔCgnoxR, and ΔCgnoxAnoxB, F-actin showed a diffused distribution and did not form a ring structure in appressoria. Furthermore, DPI treatment also interfered with the organization of the F-actin network, resulting in a fuzzy ring structure with very low fluorescence intensity (Figure 7A). During incubation on onion epidermis for 15 h, WT and ΔCgnoxA formed typical appressoria and invasive hyphae; furthermore, F-actin in appressoria was diffused with low intensity, suggesting that there was a reorganization of the F-actin structure in appressoria after the formation of invasive hyphae (Figure 7B). In comparison, appressoria of ΔCgnoxB, ΔCgnoxR, and ΔCgnoxAnoxB showed diffused F-actin structures of actin patches in conidia and appressoria; meanwhile, there were no invasive hyphae formed. In addition, to investigate whether the transcription of Lifeact-GFP was interfered in the mutants, a qRT-PCR analysis was conducted. The result showed that the relative expression levels of Lifeact-GFP in these mutants were all below twofold, suggesting that the knockout of NOX-coding genes did not influence the transcription of Lifeact-GFP (Figure S2). These results demonstrate the important roles of CgNOXB and CgNOXR in F-actin organization.
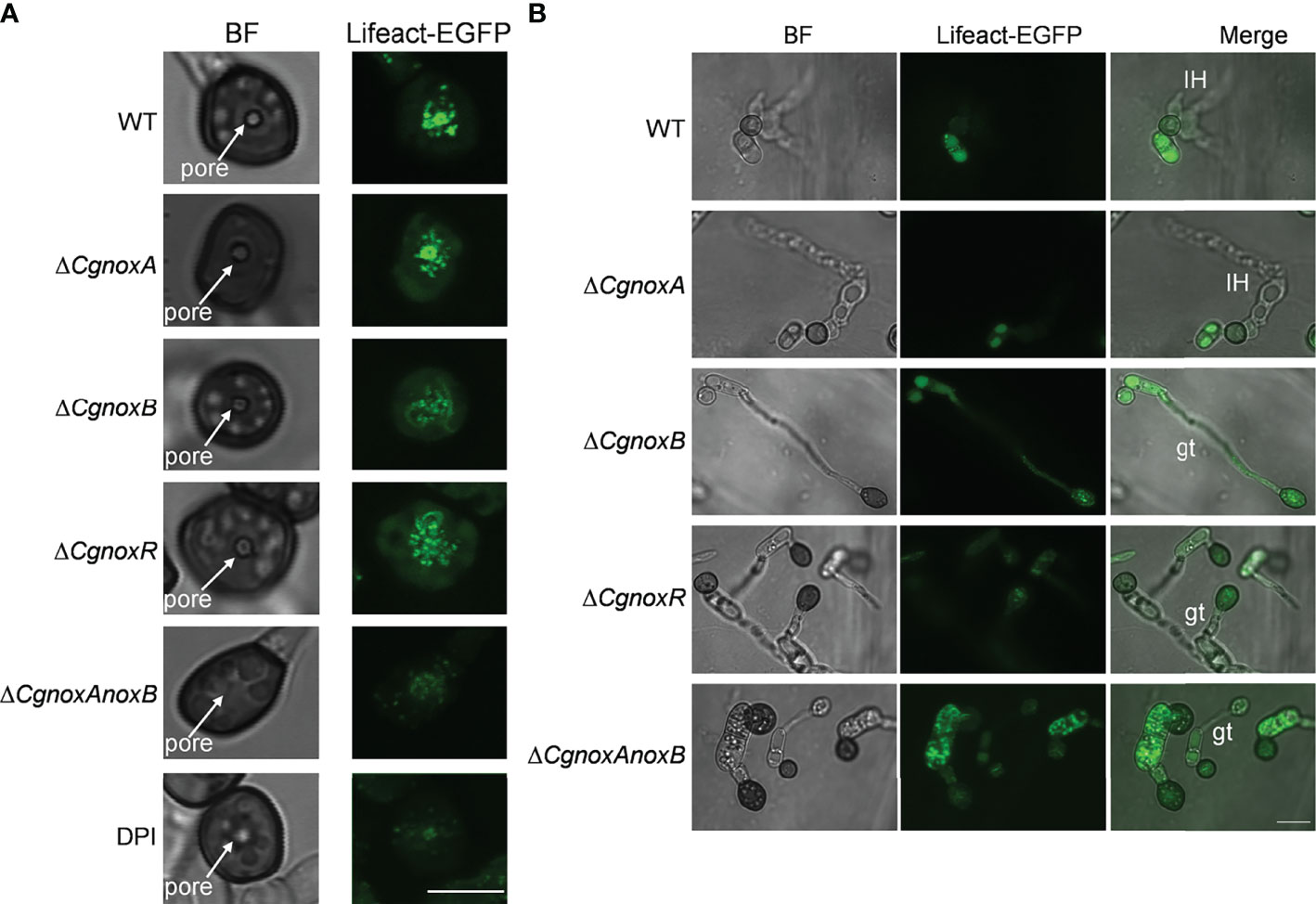
Figure 7 CgNOX regulates F-actin organization during appressorium development and penetration of C. gloeosporioides. (A) Micrographs of F-actin organization in appressorium visualized by expression of Lifeact-EGFP in WT (DPI treatment or not), ΔCgnoxA, ΔCgnoxB, ΔCgnoxR, and ΔCgnoxAnoxB strains. Conidial suspensions at 2 × 105 conidia ml-1 were inoculated onto hydrophobic glass coverslips for 24 h. Scale bar = 5 μm. (B) Micrographs of F-actin organization in appressorium and infectious hyphae of each strain on onion epidermis. Conidial suspensions at 2 × 105 conidia ml-1 were inoculated onto onion epidermis for 15 h. IH represents invasive hyphae, gt means germinated tube. Scale bar = 10 μm.
CgNOXA, CgNOXB, and CgNOXR Are Required for Cell Wall Integrity
To investigate the roles of CgNOXs in fungal cell wall synthesis, the hyphae of the strains were observed with Calcofluor white (CFW) staining. In WT, CFW fluorescence was intensively distributed at the apex regions of hyphal tips, indicating the polarized deposition of the cell wall material, whereas in ΔCgnoxA, the fluorescence is mainly located in subapex regions. Moreover, this kind of polarity was diminished in the hyphal tips of ΔCgnoxB, ΔCgnoxR, and ΔCgnoxAnoxB, in which the fluorescence was uniformly distributed all through the hyphal tips (Figure 8A).
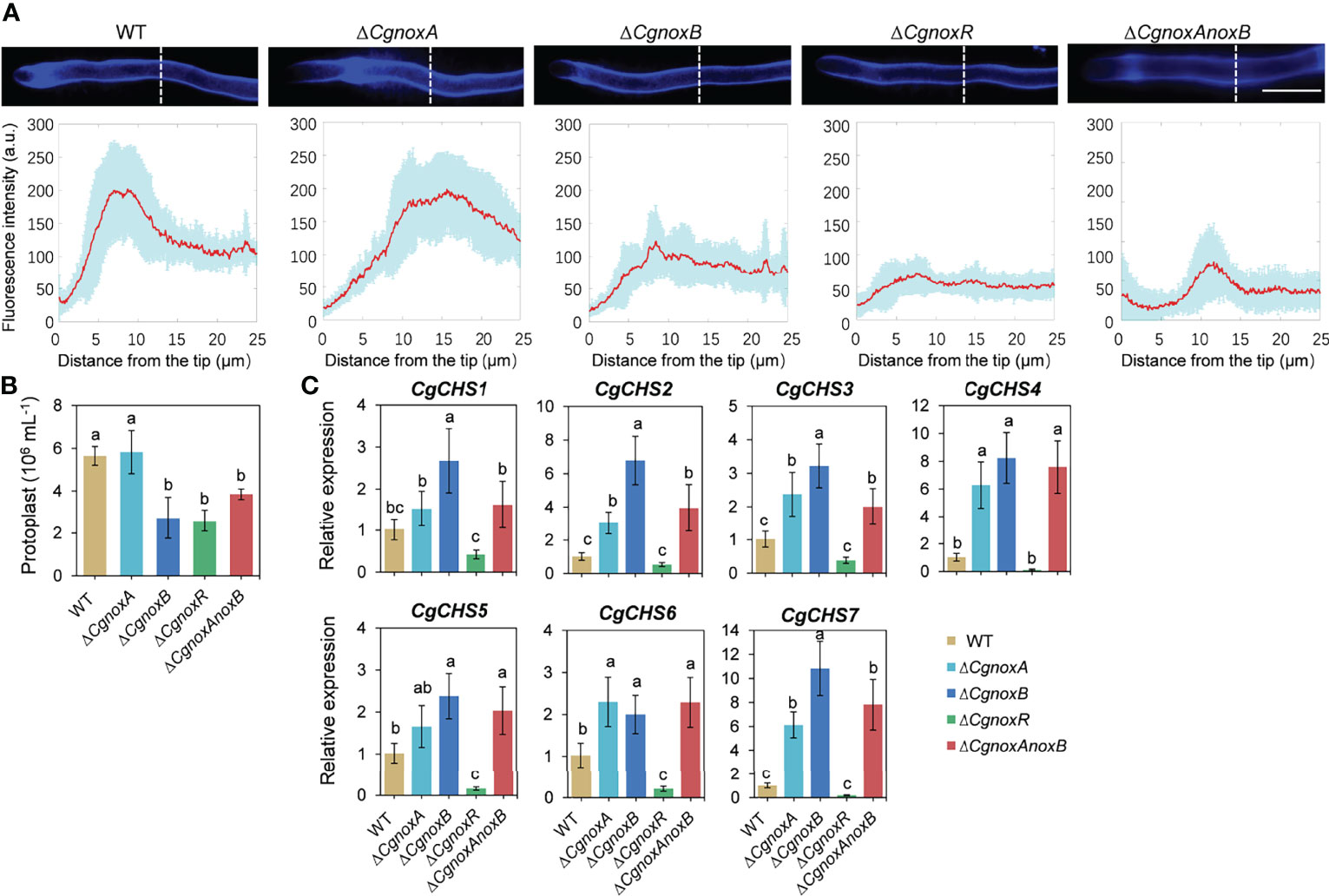
Figure 8 CgNOX regulates cell wall integrity of C. gloeosporioides. (A) CFW staining of hyphal tip from WT, ΔCgnoxA, ΔCgnoxB, ΔCgnoxR, and ΔCgnoxAnoxB strains. White dotted lines indicate the regions for the measurement. Scale bar = 10 μm. Quantitative measurements of the fluorescence intensity along the hypha from the tip. The red lines show the average fluorescence intensity and the blue-shaded regions indicate the SD. At least 10 hyphae were measured for each strain. (B) Bar chart showing frequency of protoplast generation following incubation of mycelium from each strain with lyticase. Bars represent standard deviations (SD). Columns with different letters indicate significant difference (p < 0.05). (C) Bar charts showing the relative expression level of seven chitin synthase genes in each strain. Bars represent standard deviations (SD). Columns with different letters indicate significant difference (p < 0.05).
The protoplast release assay was conducted to assess the sensitivity of the cell wall to enzymatic degradation. The results showed that ΔCgnoxA released a similar number of protoplasts as WT after the treatment with lyases. However, ΔCgnoxB, ΔCgnoxR, and ΔCgnoxAnoxB released fewer protoplasts than WT (Figure 8B). The results indicated the change in the cell wall composition of ΔCgnoxB, ΔCgnoxR, and ΔCgnoxAnoxB. Chitin synthases participate in the biosynthesis of chitin and are involved in the cell wall integrity of filamentous fungi (Yang and Zhang, 2019; Wang et al., 2021). Therefore, the expression changes of chitin synthases in the mutants were investigated. There were seven chitin synthases (CgCHS) identified in C. gloeosporioides (Wang et al., 2021). The qRT-PCR assay (Figure 8C) showed that in ΔCgnoxA, the relative expression levels of CgCHS2, CgCHS3, CgCHS4, CgCHS6, and CgCHS7 increased over twofold; meanwhile, all seven genes were upregulated in ΔCgnoxB. However, in ΔCgnoxR, all the CgCHS genes were dramatically down-regulated. These data suggest that CgNOXA, CgNOXB, and CgNOXR are all involved in the cell wall integrity in C. gloeosporioides.
Discussion
NOXs play important roles in many biological processes in living cells. In filamentous fungi, the NOX-derived ROS regulate many aspects of the life cycle including vegetative hyphal growth, conidiation, secondary metabolism, and pathogenicity of many phytopathogenic fungi (Egan et al., 2007; Giesbert et al., 2008; Segmüller et al., 2008; Heller and Tudzynski, 2011; Tudzynski et al., 2012; Ryder et al., 2013; Wang et al., 2020). Unlike that in M. oryzae and some other phytopathogenic fungi, here we found that knockout of CgnoxA or CgnoxB did not influence the colony morphology or growth rate (Figure 1). However, the vegetative growth of ΔCgnoxR and the double-mutant ΔCgnoxAnoxB was slightly reduced, indicating that CgNOXR plays an important role in the regulation of the vegetative growth, and CgNOXA and CgNOXB may be functionally redundant in the regulation of vegetative growth, at least partially. A pathogenicity assay was conducted subsequently. When inoculated onto the wounds of leaves, all the mutants could infect the host and cause lesions; moreover, ΔCgnoxB, ΔCgnoxR, and ΔCgnoxAnoxB caused smaller lesions in comparison to WT and ΔCgnoxA. However, when inoculated on intact leaves, ΔCgnoxB, ΔCgnoxR, and ΔCgnoxAnoxB lost the ability to infect the hosts (Figure 2). These results suggested that CgNOXB and CgNOXR play important roles in the early infection process of C. gloeosporioides to the host plant. As a hemibiotrophic pathogen, C. gloeosporioides could form the specialized infection structure appressoria to infect plant hosts. The appressoria generate high turgor and physical force to rupture plant cuticle and form a penetration peg which develops into invasive hyphae to penetrate plant tissue (De Jong et al., 1997; Ryder and Talbot, 2015). In addition, NOXs are involved in appressorium formation in several phytopathogenic fungi (Ryder and Talbot, 2015; Wang et al., 2020). The expression pattern assay of CgnoxA, CgnoxB, and CgnoxR showed that CgnoxB was significantly up-regulated in the appressoria (Figure 3). These results enlightened us that CgNOXs, especially CgNOXB, play important roles in appressorium formation in C. gloeosporioides.
Then the appressorium formation and the following infection processes of the mutants were investigated. We found that knockout of CgnoxA did not influence the appressorium formation and invasive hyphae development. However, knockout of CgnoxB or CgnoxR significantly inhibited the two processes (Figure 4). Furthermore, the development of invasive hyphae was even diminished in ΔCgnoxB and ΔCgnoxAnoxB. The result was in accordance with our hypothesis that CgNOXB plays an important role in appressorium formation in C. gloeosporioides. Although the transcription of CgnoxR was not up-regulated in appressoria, knockout of the gene did significantly interfere with the appressorium formation and invasive hyphal development. This might be because NOXR is the main regulatory component of the NOX complex (Takemoto et al., 2006; Cano-Domínguez et al., 2008). These results provided evidence for the roles of CgNOXB and CgNOXR in pathogenicity by regulation of appressorium formation and invasive hyphal development. Our results were partially consistent with findings in M. oryzae, in which Δnox1, Δnox2, ΔnoxR, and Δnox1nox2 are all non-pathogenic due to their defects in the development of the penetration peg (Egan et al., 2007; Ryder et al., 2013).
In plant cells, actin organization is important for polarized growth of root tip and pollen tube growth (Gibbon et al., 1999; Miller et al., 2010). In fungi, NOXs play vital roles in the developmental processes through the regulation of transitions from non-polarized to polarized cell growth (Egan et al., 2007; Kayano et al., 2013). Moreover, the polarized fungal growth is bound up with the remodeling of the F-actin cytoskeleton (Ryder et al., 2013). Therefore, we firstly investigate the F-actin organization in germ tubes. The results showed that there is a significantly polarized distribution of F-actin in the hyphal tips (Figure 6), while this ordered structure was disrupted in ΔCgnoxB, ΔCgnoxR, and ΔCgnoxAnoxB mutants.
During the appressorium formation and maturity, the F-actin remodels the ring structure around the appressorium pore, which is vital for the development of invasive hyphae (Dagdas et al., 2012). Here we found that in WT and ΔCgnoxA, F-actin formed a typical ring structure around the appressorium pore. However, in ΔCgnoxB, ΔCgnoxR, and ΔCgnoxAnoxB, F-actin did not organize into a ring and exhibited in a diffused distribution (Figure 7A). Besides, we treated C. gloeosporioides with the NOX inhibitor DPI and found that DPI treatment led to a fuzzier ring organization of F-actin. Our results were somehow different from the findings in M. oryzae, in which Nox1 is necessary for the F-actin network at the appressorium pore, whereas Nox2 and NoxR are indispensable for the ring structure (Ryder et al., 2013). After the development of invasive hyphae, the appressoria of WT and ΔCgnoxA showed a degradation of the F-actin ring structure, whereas those of ΔCgnoxB, ΔCgnoxR, and ΔCgnoxAnoxB were still with strong actin fluorescence (Figure 7B), suggesting the detention of F-actin remodeling in the mutants.
As the oxidase complex, NOXs produce ROS to regulate downstream signaling. We then investigate the ROS generation via DAB and NBT staining. Visualization of O2•- via NBT showed that in WT, there is a polarized manner of O2•- production in hyphal tips, while knockout CgnoxB or CgnoxR weakened the polarity of O2•- production (Figure 5). Similar results were also observed in the NOX gene knockout mutants of Aspergillus nidulans, Neurospora crassa, and Epichloë festucae (Takemoto et al., 2006; Cano-Domínguez et al., 2008; Semighini and Harris, 2008). Besides, the DAB staining revealed that after infection into the host cells, the appressoria of WT and ΔCgnoxA showed less ROS production than that of ΔCgnoxB, ΔCgnoxR, and ΔCgnoxAnoxB (Figure 5). This might be because the appressoria of WT and ΔCgnoxA had already formed invasive hyphae and infected onion cells, making the invasive hyphae become the new “hot spot” for ROS production. In comparison, the other three mutants were detained in the appressorium stage.
F-Actin patches and cables are involved in exocytosis and vesicle secretion in fungi (Berepiki et al., 2011). Meanwhile, the hyphal growth and appressorium formation of fungi both require the biosynthesis, exocytosis, and deposition of cell wall materials (Read, 2011; Schuster et al., 2012). Since the cell wall integrity (CWI) signaling pathway plays a crucial role in fungal growth and pathogenicity (Malavazi et al., 2014), we set out to investigate whether NOX-dependent F-actin organization is involved in CWI (Figure 8). The CFW staining showed that in the WT strain, the CFW fluoresce was intensively located at the apex region of hyphal tips, suggesting a strong polarity in the deposition of cell wall material, whereas in ΔCgnoxA, the fluoresce was mainly located in the subapex region. Furthermore, in ΔCgnoxB, ΔCgnoxR, and ΔCgnoxAnoxB, the fluorescent signal was uniformly distributed through the hyphal tips. The result indicated that the NOX-dependent F-actin organization is important for cell wall synthesis. In addition, the protoplast release assay showed that the cell wall composition was also affected in ΔCgnoxB, ΔCgnoxR, and ΔCgnoxAnoxB mutants. Moreover, the following qRT-PCR analysis revealed that the relative expression levels of CgCHS genes were up-regulated in ΔCgnoxA, ΔCgnoxB, and ΔCgnoxAnoxB, which might be the main cause for cell wall composition changes in the mutants. However, for ΔCgnoxR, the expressions of the seven CgCHS genes were all significantly down-regulated, suggesting that CgNOXR might play other regulatory functions in addition to its function on the NOX complex.
Taken together, we conclude that CgNOXB and CgNOXR regulate the spatial production of ROS and are necessary for polarized F-actin organization; this polarized F-actin structure regulates cell wall integrity and controlled polarized hyphal growth, appressorium formation, and invasive hyphal development in C. gloeosporioides (Figure 9). Our study extends the understanding of the molecular mechanism by which NOXs regulate the pathogenicity of phytopathogenic fungi.
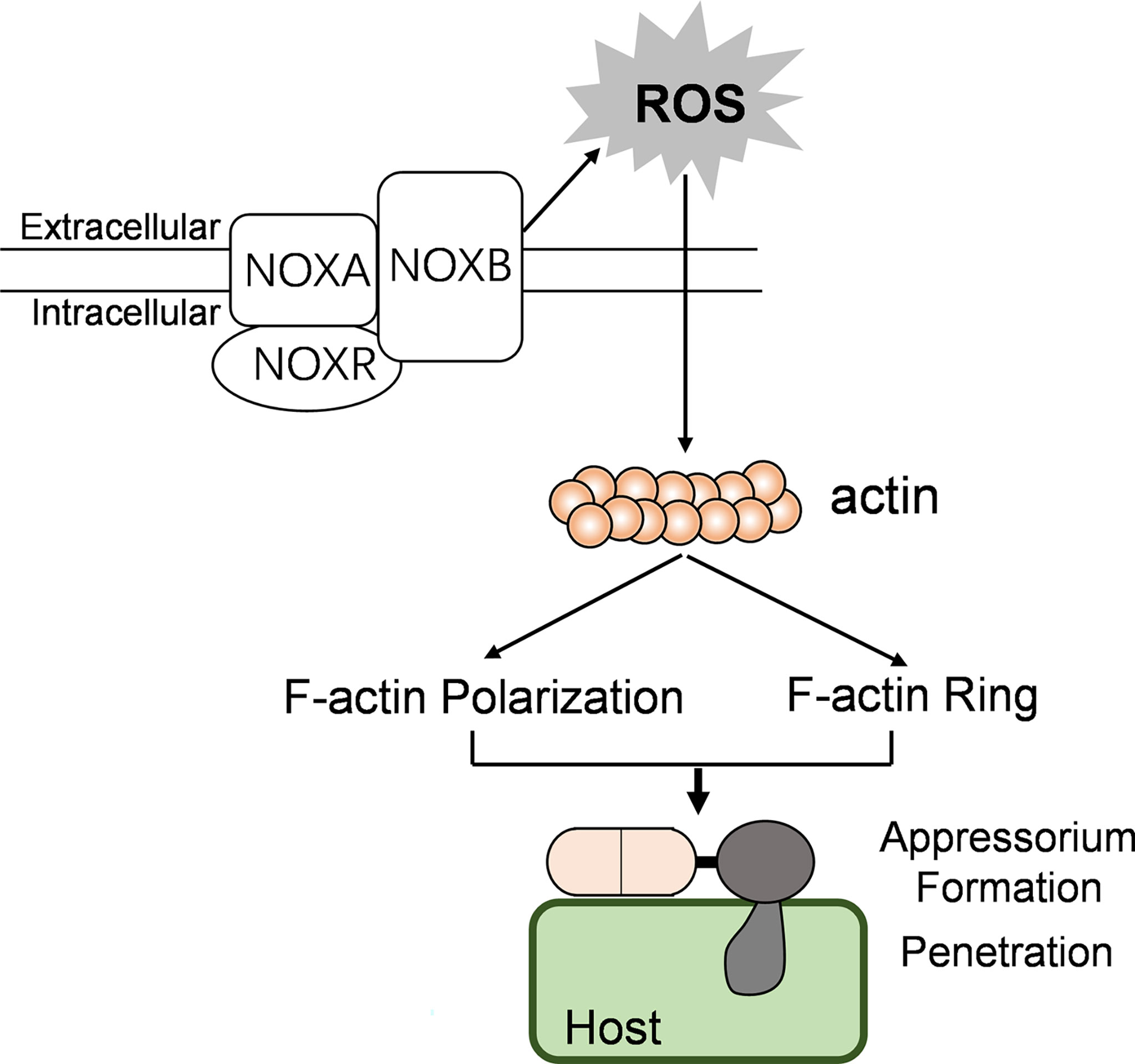
Figure 9 The possible model showing the relationships between NOX, F-actin organization, and pathogenicity in C. gloeosporioides.
Data Availability Statement
The original contributions presented in the study are included in the article/Supplementary Material. Further inquiries can be directed to the corresponding author.
Author Contributions
QW and BA designed the study. NL, WW, and QW performed the experiments. QW, BA, and NL wrote the manuscript. CH and HL revised the manuscript. All authors contributed to the article and approved the submitted version.
Funding
This work was supported by the National Natural Science Foundation of China (32001846, 32000102, 31860478, 32160594).
Conflict of Interest
The authors declare that the research was conducted in the absence of any commercial or financial relationships that could be construed as a potential conflict of interest.
Publisher’s Note
All claims expressed in this article are solely those of the authors and do not necessarily represent those of their affiliated organizations, or those of the publisher, the editors and the reviewers. Any product that may be evaluated in this article, or claim that may be made by its manufacturer, is not guaranteed or endorsed by the publisher.
Supplementary Material
The Supplementary Material for this article can be found online at: https://www.frontiersin.org/articles/10.3389/fcimb.2022.845133/full#supplementary-material
Supplementary Figure 1 | Actin filament structure in conidia of WT, ΔCgnoxA, ΔCgnoxB, ΔCgnoxR, and ΔCgnoxAnoxB strains expressing Lifeact-EGFP. Scale bar = 5 μm.
Supplementary Figure 2 | The relative expression level of Lifeact-GFP in the mutant strains. The β2-tubulin coding gene was used as the endogenous control for normalization and the relative expression level was estimated using the 2-ΔΔCt method. Values are shown as the means ± standard deviations (SD).
References
Adams, A. E., Pringle, J. R. (1984). Relationship of Actin and Tubulin Distribution to Bud Growth in Wild-Type and Morphogenetic-Mutant Saccharomyces Cerevisiae. J. Cell. Biol. 98 (3), 934–945. doi: 10.1083/jcb.98.3.934
An, B., Li, B., Li, H., Zhang, Z., Qin, G., Tian, S. (2016). Aquaporin8 Regulates Cellular Development and Reactive Oxygen Species Production, a Critical Component of Virulence in Botrytis Cinerea. New Phytol. 209 (4), 1668–1680. doi: 10.1111/nph.13721
Araujo-Bazán, L., Peñalva, M. A., Espeso, E. A. (2008). Preferential Localization of the Endocytic Internalization Machinery to Hyphal Tips Underlies Polarization of the Actin Cytoskeleton in Aspergillus Nidulans. Mol. Microbiol. 67 (4), 891–905. doi: 10.1111/j.1365-2958.2007.06102.x
Berepiki, A., Lichius, A., Read, N. D. (2011). Actin Organization and Dynamics in Filamentous Fungi. Nat. Rev. Microbiol. 9 (12), 876–887. doi: 10.1038/nrmicro2666
Brown, D. I., Griendling, K. K. (2009). Nox Proteins in Signal Transduction. Free Radical. Bio. Med. 47 (9), 1239–1253. doi: 10.1016/j.freeradbiomed.2009.07.023
Cano-Domínguez, N., Alvarez-Delfín, K., Hansberg, W., Aguirre, J. (2008). NADPH Oxidases NOX-1 and NOX-2 Require the Regulatory Subunit NOR-1 to Control Cell Differentiation and Growth in Neurospora Crassa. Eukaryot. Cell. 7 (8), 1352–1361. doi: 10.1128/EC.00137-08
Dagdas, Y. F., Yoshino, K., Dagdas, G., Ryder, L. S., Bielska, E., Steinberg, G., et al. (2012). Septin-Mediated Plant Cell Invasion by the Rice Blast Fungus, Magnaporthe Oryzae. Science 336, 1590–1595. doi: 10.1126/science.1222934
Dean, R., Van Kan, J. A., Pretorius, Z. A., Hammond-Kosack, K. E., Di Pietro, A., Spanu, P. D., et al. (2012). The Top 10 Fungal Pathogens in Molecular Plant Pathology. Mol. Plant Pathol. 13 (4), 414–430. doi: 10.1111/j.1364-3703.2011.00783.x
De Jong, J. C., McCormack, B. J., Smirnoff, N., Talbot, N. J. (1997). Glycerol Generates Turgor in Rice Blast. Nature 389 (6648), 244–244. doi: 10.1038/38418
Dulal, N., Rogers, A. M., Proko, R., Bieger, B. D., Liyanage, R., Krishnamurthi, V. R., et al. (2021). Turgor-Dependent and Coronin-Mediated F-Actin Dynamics Drive Septin Disc-to-Ring Remodeling in the Blast Fungus Magnaporthe Oryzae. J. Cell. Sci. 134 (5), jcs251298. doi: 10.1242/jcs.251298
Egan, M. J., Wang, Z. Y., Jones, M. A., Smirnoff, N., Talbot, N. J. (2007). Generation of Reactive Oxygen Species by Fungal NADPH Oxidases is Required for Rice Blast Disease. P. Nal. Acad. Sci. U.S.A. 104 (28), 11772–11777. doi: 10.1073/pnas.0700574104
Evangelista, M., Pruyne, D., Amberg, D. C., Boone, C., Bretscher, A. (2002). Formins Direct Arp2/3-Independent Actin Filament Assembly to Polarize Cell Growth in Yeast. Nat. Cell. Biol. 4 (3), 260–269. doi: 10.1038/ncb770
Foreman, J., Demidchik, V., Bothwell, J. H., Mylona, P., Miedema, H., Torres, M. A., et al. (2003). Reactive Oxygen Species Produced by NADPH Oxidase Regulate Plant Cell Growth. Nature 422 (6930), 442–446. doi: 10.1038/nature01485
Gao, X., Wang, Q., Feng, Q., Zhang, B., He, C., Luo, H., et al. (2022). Heat Shock Transcription Factor CgHSF1 Is Required for Melanin Biosynthesis, Appressorium Formation, and Pathogenicity in Colletotrichum Gloeosporioides. J. Fungi. 8 (2), 175. doi: 10.3390/jof8020175
Gibbon, B. C., Kovar, D. R., Staiger, C. J. (1999). Latrunculin B has Different Effects on Pollen Germination and Tube Growth. Plant Cell. 11 (12), 2349–2363. doi: 10.1105/tpc.11.12.2349
Giesbert, S., Schürg, T., Scheele, S., Tudzynski, P. (2008). The NADPH Oxidase Cpnox1 is Required for Full Pathogenicity of the Ergot Fungus Claviceps Purpurea. Mol. Plant Pathol. 9 (3), 317–327. doi: 10.1111/j.1364-3703.2008.00466.x
González-Rodríguez, V. E., Garrido, C., Cantoral, J. M., Schumacher, J. (2016). The F-Actin Capping Protein is Required for Hyphal Growth and Full Virulence But is Dispensable for Septum Formation in Botrytis Cinerea. Fungal. Biol. 120 (10), 1225–1235. doi: 10.1016/j.funbio.2016.07.007
Guo, Y., An, B. (2018). Functional Analysis of NADPH Oxidases in Colletotrichum Gloeosporioides From Hevea Brasiliensis. Biotechnol. Bull. (in chinese) 34 (10), 165–171. doi: 10.13560/j.cnki.biotech.bull.1985.2018-0344
Heller, J., Tudzynski, P. (2011). Reactive Oxygen Species in Phytopathogenic Fungi: Signaling, Development, and Disease. Annu. Rev. Phytopathol. 49, 369–390. doi: 10.1146/annurev-phyto-072910-095355
Kadota, Y., Shirasu, K., Zipfel, C. (2015). Regulation of the NADPH Oxidase RBOHD During Plant Immunity. Plant Cell. Physiol. 56 (8), 1472–1480. doi: 10.1093/pcp/pcv063
Kayano, Y., Tanaka, A., Akano, F., Scott, B., Takemoto, D. (2013). Differential Roles of NADPH Oxidases and Associated Regulators in Polarized Growth, Conidiation and Hyphal Fusion in the Symbiotic Fungus Epichloë Festucae. Fungal. Genet. Biol. 56, 87–97. doi: 10.1016/j.fgb.2013.05.001
Kilmartin, J. V., Adams, A. E. (1984). Structural Rearrangements of Tubulin and Actin During the Cell Cycle of the Yeast Saccharomyces. J. Cell. Biol. 98 (3), 922–933. doi: 10.1083/jcb.98.3.922
Lambeth, J. D. (2004). NOX Enzymes and the Biology of Reactive Oxygen. Nat. Rev. Immunol. 4 (3), 181–189. doi: 10.1038/nri1312
Liu, N., Liu, S., Wang, Q. (2021). Construction of a Strain With Fluorescence Labeling of Cytoskeleton in Colletotrichum Gloeosporioides. Biotechnol. Bull. (in chinese) 37 (8), 284–293. doi: 10.13560/j.cnki.biotech.bull.1985.2020-1467
Livak, K. J., Schmittgen, T. D. (2001). Analysis of Relative Gene Expression Data Using Real-Time Quantitative PCR and the 2(-Delta Delta C(T)) Method. Methods 25 (4), 402–408. doi: 10.1006/meth.2001.1262
Malavazi, I., Goldman, G. H., Brown, N. A. (2014). The Importance of Connections Between the Cell Wall Integrity Pathway and the Unfolded Protein Response in Filamentous Fungi. Brief Funct. Genomics 13, 456–470. doi: 10.1093/bfgp/elu027
Marino, D., Dunand, C., Puppo, A., Pauly, N. (2012). A Burst of Plant NADPH Oxidases. Trends Plant Sci. 17 (1), 9–15. doi: 10.1016/j.tplants.2011.10.001
Miller, D. D., De Ruijter, N., Bisseling, T., Emons, A. (2010). The Role of Actin in Root Hair Morphogenesis: Studies With Lipochito-Oligosaccharide as a Growth Stimulator and Cytochalasin as an Actin Perturbing Drug. Plant J. 17 (2), 141–154. doi: 10.1046/j.1365-313X.1999.00358.x
Nordzieke, D. E., Fernandes, T. R., El Ghalid, M., Turrà, D., Di Pietro, A. (2019). NADPH Oxidase Regulates Chemotropic Growth of the Fungal Pathogen Fusarium Oxysporum Towards the Host Plant. New Phytol. 224 (4), 1600–1612. doi: 10.1111/nph.16085
Read, N. D. (2011). Exocytosis and Growth do Not Occur Only at Hyphal Tips. Mol. Microbiol. 81 (1), 4–7. doi: 10.1111/j.1365-2958.2011.07702.x
Riquelme, M., Sánchez-León, E. (2014). The Spitzenkörper: A Choreographer of Fungal Growth and Morphogenesis. Curr. Opin. Microbiol. 20, 27–33. doi: 10.1016/j.mib.2014.04.003
Ryder, L. S., Dagdas, Y. F., Mentlak, T. A., Kershaw, M. J., Thornton, C. R., Schuster, M., et al. (2013). NADPH Oxidases Regulate Septin-Mediated Cytoskeletal Remodeling During Plant Infection by the Rice Blast Fungus. P. Natl. Acad. Sci. U.S.A. 110 (8), 3179–3184. doi: 10.1073/pnas.1217470110
Ryder, L. S., Talbot, N. J. (2015). Regulation of Appressorium Development in Pathogenic Fungi. Curr. Opin. Plant Biol. 26, 8–13. doi: 10.1016/j.pbi.2015.05.013
Schuster, M., Treitschke, S., Kilaru, S., Molloy, J., Harmer, N. J., Steinberg, G. (2012). Myosin-5, Kinesin-1 and Myosin-17 Cooperate in Secretion of Fungal Chitin Synthase. EMBO J. 31 (1), 214–227. doi: 10.1038/emboj.2011.361
Segmüller, N., Kokkelink, L., Giesbert, S., Odinius, D., van Kan, J., Tudzynski, P. (2008). NADPH Oxidases are Involved in Differentiation and Pathogenicity in Botrytis Cinerea. Mol. Plant Microbe Interact. 21 (6), 808–819. doi: 10.1094/MPMI-21-6-0808
Semighini, C. P., Harris, S. D. (2008). Regulation of Apical Dominance in Aspergillus Nidulans Hyphae by Reactive Oxygen Species. Genetics 179 (4), 1919–1932. doi: 10.1534/genetics.108.089318
Shaw, B. D., Chung, D. W., Wang, C. L., Quintanilla, L. A., Upadhyay, S. (2011). A Role for Endocytic Recycling in Hyphal Growth. Fungal. Biol. 115 (6), 541–546. doi: 10.1016/j.funbio.2011.02.010
Siegmund, U., Marschall, R., Tudzynski, P. (2015). BcNoxD, a Putative ER Protein, is a New Component of the NADPH Oxidase Complex in Botrytis Cinerea. Mol. Microbiol. 95 (6), 988–1005. doi: 10.1111/mmi.12869
Sumimoto, H. (2008). Structure, Regulation and Evolution of Nox-Family NADPH Oxidases That Produce Reactive Oxygen Species. FEBS J. 275 (13), 3249–3277. doi: 10.1111/j.1742-4658.2008.06488.x
Suzuki, N., Miller, G., Morales, J., Shulaev, V., Torres, M. A., Mittler, R. (2011). Respiratory Burst Oxidases: The Engines of ROS Signaling. Curr. Opin. Plant Biol. 14 (6), 691–699. doi: 10.1016/j.pbi.2011.07.014
Taheri-Talesh, N., Horio, T., Araujo-Bazán, L., Dou, X., Espeso, E. A., Peñalva, M. A., et al. (2008). The Tip Growth Apparatus of Aspergillus Nidulans. Mol. Biol. Cell. 19 (4), 1439–1449. doi: 10.1091/mbc.e07-05-0464
Taheri-Talesh, N., Xiong, Y., Oakley, B. R. (2012). The Functions of Myosin II and Myosin V Homologs in Tip Growth and Septation in Aspergillus Nidulans. PLoS One 7 (2), e31218. doi: 10.1371/journal.pone.0031218
Takemoto, D., Kamakura, S., Saikia, S., Becker, Y., Wrenn, R., Tanaka, A., et al. (2011). Polarity Proteins Bem1 and Cdc24 are Components of the Filamentous Fungal NADPH Oxidase Complex. P. Natl. Acad. Sci. U.S.A. 108 (7), 2861–2866. doi: 10.1073/pnas.1017309108
Takemoto, D., Tanaka, A., Scott, B. (2006). A p67Phox-Like Regulator is Recruited to Control Hyphal Branching in a Fungal-Grass Mutualistic Symbiosis. Plant Cell. 18 (10), 2807–2821. doi: 10.1105/tpc.106.046169
Takemoto, D., Tanaka, A., Scott, B. (2007). NADPH Oxidases in Fungi: Diverse Roles of Reactive Oxygen Species in Fungal Cellular Differentiation. Fungal. Genet. Biol. 44 (11), 1065–1076. doi: 10.1016/j.fgb.2007.04.011
Takeshita, N., Manck, R., Grün, N., de Vega, S. H., Fischer, R. (2014). Interdependence of the Actin and the Microtubule Cytoskeleton During Fungal Growth. Curr. Opin. Microbiol. 20, 34–41. doi: 10.1016/j.mib.2014.04.005
Torres, M. A., Dangl, J. L., Jones, J. D. (2002). Arabidopsis Gp91phox Homologues AtrbohD and AtrbohF are Required for Accumulation of Reactive Oxygen Intermediates in the Plant Defense Response. P. Natl. Acad. Sci. U.S.A. 99 (1), 517–522. doi: 10.1073/pnas.012452499
Tudzynski, P., Heller, J., Siegmund, U. (2012). Reactive Oxygen Species Generation in Fungal Development and Pathogenesis. Curr. Opin. Microbiol. 15 (6), 653–659. doi: 10.1016/j.mib.2012.10.002
Wang, Q., An, B., Hou, X., Guo, Y., Luo, H., He, C. (2018). Dicer-Like Proteins Regulate the Growth, Conidiation, and Pathogenicity of Colletotrichum Gloeosporioides From Hevea Brasiliensis. Front. Microbiol. 8. doi: 10.3389/fmicb.2017.02621
Wang, F., Gao, W., Sun, J., Mao, X., Liu, K., Xu, J., et al. (2020). NADPH Oxidase ClNOX2 Regulates Melanin-Mediated Development and Virulence in Curvularia Lunata. Mol. Plant Microbe In 33 (11), 1315–1329. doi: 10.1094/MPMI-06-20-0138-R
Wang, X., Lu, D., Tian, C. (2021). CgEnd3 Regulates Endocytosis, Appressorium Formation, and Virulence in the Poplar Anthracnose Fungus Colletotrichum Gloeosporioides. Int. J. Mol. Sci. 22 (8), 4029. doi: 10.3390/ijms22084029
Keywords: actin cytoskeleton, appressorium, Colletotrichum gloeosporioides, NADPH oxidases, polarized growth
Citation: Liu N, Wang W, He C, Luo H, An B and Wang Q (2022) NADPH Oxidases Play a Role in Pathogenicity via the Regulation of F-Actin Organization in Colletotrichum gloeosporioides. Front. Cell. Infect. Microbiol. 12:845133. doi: 10.3389/fcimb.2022.845133
Received: 29 December 2021; Accepted: 16 May 2022;
Published: 15 June 2022.
Edited by:
Wei Tang, Fujian Agriculture and Forestry University, ChinaReviewed by:
Ziyi Yin, Shandong Agricultural University, ChinaRongbo Wang, Fujian Academy of Agricultural Sciences, China
Copyright © 2022 Liu, Wang, He, Luo, An and Wang. This is an open-access article distributed under the terms of the Creative Commons Attribution License (CC BY). The use, distribution or reproduction in other forums is permitted, provided the original author(s) and the copyright owner(s) are credited and that the original publication in this journal is cited, in accordance with accepted academic practice. No use, distribution or reproduction is permitted which does not comply with these terms.
*Correspondence: Qiannan Wang, d2FuZ3FpYW5uYW5AaGFpbmFudS5lZHUuY24=
†These authors have contributed equally to this work