- 1Department of Epidemiology, School of Public Health, University of São Paulo, São Paulo, Brazil
- 2Laboratory of Investigation in Metabolism and Diabetes, Gastrocentro, School of Medical Sciences, University of Campinas, Campinas, Brazil
- 3School of Applied Sciences, University of Campinas, Campinas, Brazil
- 4Oswaldo Cruz Foundation, Belo Horizonte, Brazil
- 5Department of Preventive Medicine, Federal University of São Paulo, São Paulo, Brazil
- 6Obesity and Comorbidities Research Center, University of Campinas, Campinas, Brazil
Introduction: Early-life events are associated with the risk of obesity and comorbidities later in life. The gut microbiota—whose composition is influenced by genetics and environmental factors—could be involved. Since the microbiota affects metabolism and fat storage, early-life insults could contribute to the occurrence of obesity driven, in part, by microbiota composition. We examined associations of gut bacteria with early-life events, nutritional status, and body composition in the Nutritionist’s Health Study (NutriHS).
Methods: A cross-sectional study of 114 female participants examining early-life data, body composition, and biological samples was conducted. Fecal microbiota structure was determined targeting the V4 region of the 16S rRNA gene. Principal coordinates analysis (PCoA) and permutational multivariate analysis of variance (PERMANOVA) were used to test the impact of variables on microbial diversity. Profiles were identified using the Jensen-Shannon divergence matrix and Calinski–Harabasz index. Differential abundance between the categories of exclusive breastfeeding duration and nutritional status was tested using DESeq2.
Results: In the sample [median age 28 years and body mass index (BMI) 24.5 kg/m2], 2 microbiota profiles driven by the Blautia or Prevotella genus were identified. An estimated 9.1% of the variation was explained by the profiles (p < 0.001), 2.1% by nutritional status (p = 0.004), and 1.8% by exclusive breastfeeding (p = 0.012). The proportion of participants with BMI <25 kg/m2 and who were breastfed for at least 6 months was higher in the Blautia profile (p < 0.05).
Conclusion: Findings in a Blautia-driven profile of healthy women reinforce that early-life events play a role in defining gut microbiota composition, confirming the importance of exclusive breastfeeding for infant gut colonization in establishing a protective profile against adiposity-related outcomes in adulthood.
Introduction
The importance of prenatal and postnatal events in long-term health outcomes has been consistently recognized (Ravelli et al., 1976; Barker, 1990; Bell et al., 2017; Block and El-Osta, 2017; Cheshmeh et al., 2020; Capra et al., 2021). Nutritional factors during intrauterine life and after birth have a major impact on infant health and later in adulthood, influencing the risk for non-communicable chronic diseases (Garmendia et al., 2014; Cadenas-Sanchez et al., 2017). Early feeding and infant growth rate have been associated with the risk of obesity and cardiometabolic diseases later in life (Kelishadi and Farajian, 2014; Kapourchali and Cresci, 2020). Important underlying mechanisms of these associations involve the gut microbiome (Bouter et al., 2017; Meijnikman et al., 2018). Gut colonization of the newborn starts at birth by bacteria from the mother and the environment. Major determinants of gut microbiota composition in early life are type of delivery, lactation, antibiotic use, and sanitary conditions (Biasucci et al., 2010; Martin et al., 2016; Le Doare et al., 2018; Cheng and Ning, 2019). Evidence indicates that these factors shape the gut microbiota throughout life (Rodríguez et al., 2015; Cheng and Ning, 2019) and that adult microbiota composition shows slight fluctuations around a core of stable colonizers.
Despite similar counts of human cells and microbes throughout the gastrointestinal tract, the gut microbiome contains 100 times more genes (Qin et al., 2010; Shen et al., 2013; Sender et al., 2016). This indicates that microbial communities play vital roles in the host and that an unbalanced microbiota can deteriorate regulatory functions, triggering immune and metabolic disturbances (Levy et al., 2017; Sommer et al., 2017). Factors such as aging (Rodríguez et al., 2015; Cheng and Ning, 2019; Fan and Pedersen, 2021), diet (David et al., 2014), nutritional status, and exercise induce changes (Rodríguez et al., 2015; Mailing et al., 2019) in microbiota composition, hampering understanding of the involvement of this complex ecosystem in pathophysiological processes. Arumugam et al. (2011) proposed analyzing the gut microbiota based on microbial profiles driven by discriminative genera referred to as enterotypes. Long-term dietary patterns have been linked to enterotypes in populations. A carbohydrate-based or vegetarian diet was found to be associated with Prevotella, while the typical Western diet was associated with Bacteroides enterotype (De Filippo et al., 2010; Wu et al., 2011; de Moraes et al., 2017). However, further studies have questioned such discrete profiles, given that these microbial communities proved not to be recurrent across diverse human populations (Gorvitovskaia et al., 2016). Despite controversies, it is clear that the risk or protection against non-communicable chronic diseases conferred by lifestyle is modulated by the gut microbiota, which affects nutrient acquisition, energy regulation, and fat storage (Rosenbaum et al., 2015; Wu et al., 2021). This could be a plausible pathway by which early-life exposures are associated with later body phenotypes.
Our group has been conducting the Nutritionist’s Health Study (NutriHS) involving nutrition undergraduates and nutritionists (Folchetti et al., 2016). This represents a unique opportunity to collect reliable nutrition-related data, accurate body composition measurements, and biological samples to test associations with early-life events and current lifestyle potentially mediated by the gut microbiota. The aim of the present study was to examine associations of gut bacteria with early-life events, current nutritional status, and body composition in NutriHS participants.
Materials and Methods
Study Design and Participants
This cross-sectional analysis was part of the multicenter NutriHS conducted at the School of Public Health of the University of São Paulo State, Brazil, to investigate markers of cardiometabolic diseases (Folchetti et al., 2016). Current data were collected at the University of Campinas (UNICAMP), located in Campinas city in the interior of São Paulo state. The NutriHS was approved by the local research ethics committee, and volunteers signed an electronic informed consent form available on the e-NutriHS system (www.fsp.usp.br/nutrihs). Recruitment of volunteers took place between 2018 and 2019.
Eligibility criteria were female undergraduates or nutritionists aged 19–44 years, body mass index (BMI) between 18.5 and 39.9 kg/m², and individuals whose mothers were alive. Pregnant and nursing women or individuals with diabetes, kidney, heart, and liver diseases, or other severe systemic diseases, in use of medications affecting glucose metabolism and/or body adiposity, or in use of probiotics or antibiotics in the last 3 months were excluded. Participants filled out online structured validated questionnaires. Respondents were then invited to schedule a face-to-face visit for physical examination and collection of biological samples. A total of 248 women answered the questionnaires, 127 met the inclusion criteria, and 114 concluded the full protocol (Figure S1).
Early-Life and Current Data
Regarding information about early-life events, participants were advised to consult birth cards and seek assistance from their mothers. Maternal data collected were pre-pregnancy age, education levels (<11; ≥11 years) and BMI, and gestational diabetes, hypertension, or other complications (yes; no), parity (0; ≥1 pregnancy), tobacco, alcohol, and/or drug use (no; yes), and type of delivery (vaginal; C-section). Maternal gestational weight gain and participants’ birth weight were obtained as continuous variables. Continuous data on participant birth weight and duration of exclusive breastfeeding were further categorized into <2.5 kg, 2.5–4.0 kg, or ≥4.0 kg and into <6 months or ≥6 months, respectively.
Current data collected were skin color (white; non-white), age, family income (<6; ≥6 minimum wages), and engagement in leisure time physical activity (no; yes). Physical activity was assessed using the short version of the International Physical Activity Questionnaire (Craig et al., 2003) validated for use in Brazil (Matsudo et al., 2001). Dietary intake was estimated using a validated food frequency questionnaire for the adult population living in São Paulo, with the previous year as the time frame (Selem et al., 2014). The questionnaire comprised 101 food items, and food equivalents in the USDA National Nutrient Database for Standard Reference were employed (Haytowitz et al., 2019).
Clinical and Body Composition Assessment
Body weight was obtained using a digital scale, and height was measured using a fixed stadiometer. BMI was calculated, and nutritional status was classified according to the WHO standards (WHO, 2015). Adequate nutritional status was defined as BMI >18.5 and <25 kg/m2. Waist circumference was measured at the midpoint between the last rib and iliac crest using an inelastic tape.
Body composition was assessed using dual-energy x-ray absorptiometry (DXA) (GE Lunar iDXA® with EnCore software, Madison, WI, USA) by a trained researcher. Instrument quality control was checked routinely according to the manufacturer’s instructions. Parameters of interest were measurements of total fat and visceral fat mass and of total and appendicular lean mass.
Biochemical Analyses
After a 12-h overnight fast, blood samples were collected for biochemical determinations. Glucose and lipid profile [total cholesterol, high-density lipoprotein (HDL) cholesterol, and triglycerides] were measured using the glucose oxidase and enzymatic colorimetric methods, respectively. Low-density lipoprotein (LDL) cholesterol was calculated by the Friedwald equation. Plasma insulin was obtained using an automated two-site chemiluminescent immunometric assay (Immulite 1000 System, Siemens Health Diagnostics, USA). Homeostasis model assessment of insulin resistance (HOMA-IR) was calculated (Matthews et al., 1985). High-sensitivity C-reactive protein was determined by nephelometry using a BN ProSpec System (Siemens, Marburg, Germany).
Plasma concentrations of short-chain fatty acids (SCFAs: acetate, propionate, and butyrate) were measured by gas chromatography (Wang et al., 2019). Briefly, ethanol, n-hexane, and an internal standard (caprylic acid) were added to serum. Samples were centrifuged and transferred to specific vials, and pH was adjusted to 4.0. A calibration curve with 0.015–0.1 mg/ml SCFA was used in the quantification. Chromatographic analyses were performed using a gas chromatograph-mass spectrometer (model Coupled QP2010 Plus; Shimadzu®, Kyoto, Japan) and a fused-silica capillary Stabilwax column (Restec Corporation, USA) with dimensions of 30 m × 0.25 mm internal diameter and coated with a 0.25-µm-thick layer of polyethylene glycol. Samples were injected at 250°C using a 25:1 split ratio for feces or splitless. High-grade pure helium was used as the carrier gas with a constant flow rate of 1.0 ml/min. Mass conditions were as follows: ionization voltage, 70 eV; ion source temperature, 200°C; full scan mode in the 35–500 mass range with 0.2 s/scan velocity. The butyrate columns did not appear, since the concentration of the acid was not detectable in the samples.
Gut Microbiota Analysis
Fecal samples were refrigerated within 24 h after collection, and aliquots were stored at -80°C until analysis. According to the manufacturer’s instructions, DNA was extracted using the Maxwell® 16 DNA purification kit and the protocol was carried out on the Maxwell® 16 Instrument (Promega, Madison, WI, USA). We used the primers and workflow to generate the amplicon from the V4 region of the 16s rRNA gene according to Penington et al. (2018). The amplicon library produced was sequenced on the Illumina MiSeq platform (Illumina, San Diego, CA, USA), according to the manufacturer’s instructions.
The raw read files were processed in the R environment using the dada2 package [10.1038/nmeth.3869] (Ombrello, 2020). The forward and reverse sequences were trimmed to 150 bases. Reads containing more than two expected errors were removed. Errors in filtered sequences were corrected by the algorithm and joined to form the amplicon sequence variants (ASVs). The chimeric sequences were also removed, and a sample count table was generated. The taxonomic classification was done with the tag.me package [10.1101/263293] using the model 515F-806R (Pires et al., 2018).
Statistical Analyses
All data were recorded, edited, and entered using the Statistical Package for the Social Sciences (SPSS version 20; IBM, NY, USA) and the R package for microbiota analyses. Level of significance was set at a p-value of 5%. Descriptive data were expressed as means [standard deviations (SDs)] or medians {q25–q75 ranges [interquartile range (IQR)]}. The Kolmogorov–Smirnov test was used to test data normality. Parametric tests (Pearson’s correlation coefficient and Student’s t test) and non-parametric tests (Spearman’s correlation coefficient and Mann–Whitney) were applied according to the distribution of variables.
The beta diversity was calculated using principal coordinates analysis (PCoA) and the ade4 R package for each library (Dray and Dufour, 2007). Permutational multivariate analysis of variance (PERMANOVA) was performed using 999 permutations to test the impact of categorical variables on beta diversity. Distance-based redundancy analysis (dbRDA) highlights variables with some association with the individual microbiota dissimilarities (Legendre and Anderson, 1999). Profiles were identified based on the Jensen-Shannon divergence matrix and using the Partitioning Around Medoids (PAM) algorithm, and the optimal number of clusters was determined by the Calinski–Harabasz index. The alpha diversity was measured by the Shannon and Simpson indexes. Differential abundance between profiles according to the categories of exclusive breastfeeding duration and nutritional status was tested using DESeq2, leaving genus with at least 50%-fold change and present in half of the samples (Love et al., 2014).
Macronutrient intakes were expressed as percentage of total energy intake (TEI) and fatty acid intake in grams. Correlations between dietary components and SCFA concentrations and body adiposity parameters were tested using Spearman’s coefficient.
Results
The sample of 114 participants had a median age of 28 (IQR 24–31) years; 41.6% were undergraduates and 58.4% were nutritionists. Sixty-one percent of the participants engaged in moderate physical activity regularly, none was a professional athlete, and 51.0% had normal BMI. Regarding maternal characteristics, 35% had higher-level education and 90% were normal weight before pregnancy and had no clinical complications during the pregnancy. In the total sample, there was a predominance of cesarean delivery (66%) and normal birth weight (90%), 94% were breastfed, and 18% were exclusively breastfed for at least 6 months. In addition, 30% of participants reported overweight/obesity in childhood or adolescence.
The dbRDA (Figure 1) results show that exclusive breastfeeding and adequate nutritional status are located to the right and adiposity parameters to the left of the plot. The relative abundance of Blautia, Anaerostipes, and Lachnoclostridium increased directly on the X-axis representing both breastfeeding and adequate nutritional status (Figure 1). Conversely, inverse relationships for Ruminococcaceae were observed. The results of the redundancy analysis for the most abundant bacteria are shown in the supplementary material (Figure S2).
Beta diversity analysis of the microbiota revealed two bacterial profiles in the samples driven by the Blautia or Prevotella genus. Fifty-six participants were assigned to Blautia and 58 to Prevotella profiles. The PERMANOVA analysis on the Jensen-Shannon divergence values estimated that 9.1% of the variation among the samples was explained by the profiles (p < 0.001), 2.1% by nutritional status (p = 0.004), and 1.8% by exclusive breastfeeding (p = 0.012). Proportions of participants with BMI <25 kg/m2 and of those breastfed for at least 6 months were significantly (p < 0.05) higher in the Blautia-driven profile (Figure 2). A schematic interpretation of the main findings is provided in Figure 3. The proportion categorized by type of delivery (vaginal or cesarean section) or birth weight (adequate or inadequate) did not differ between the 2 groups (not shown in figures).
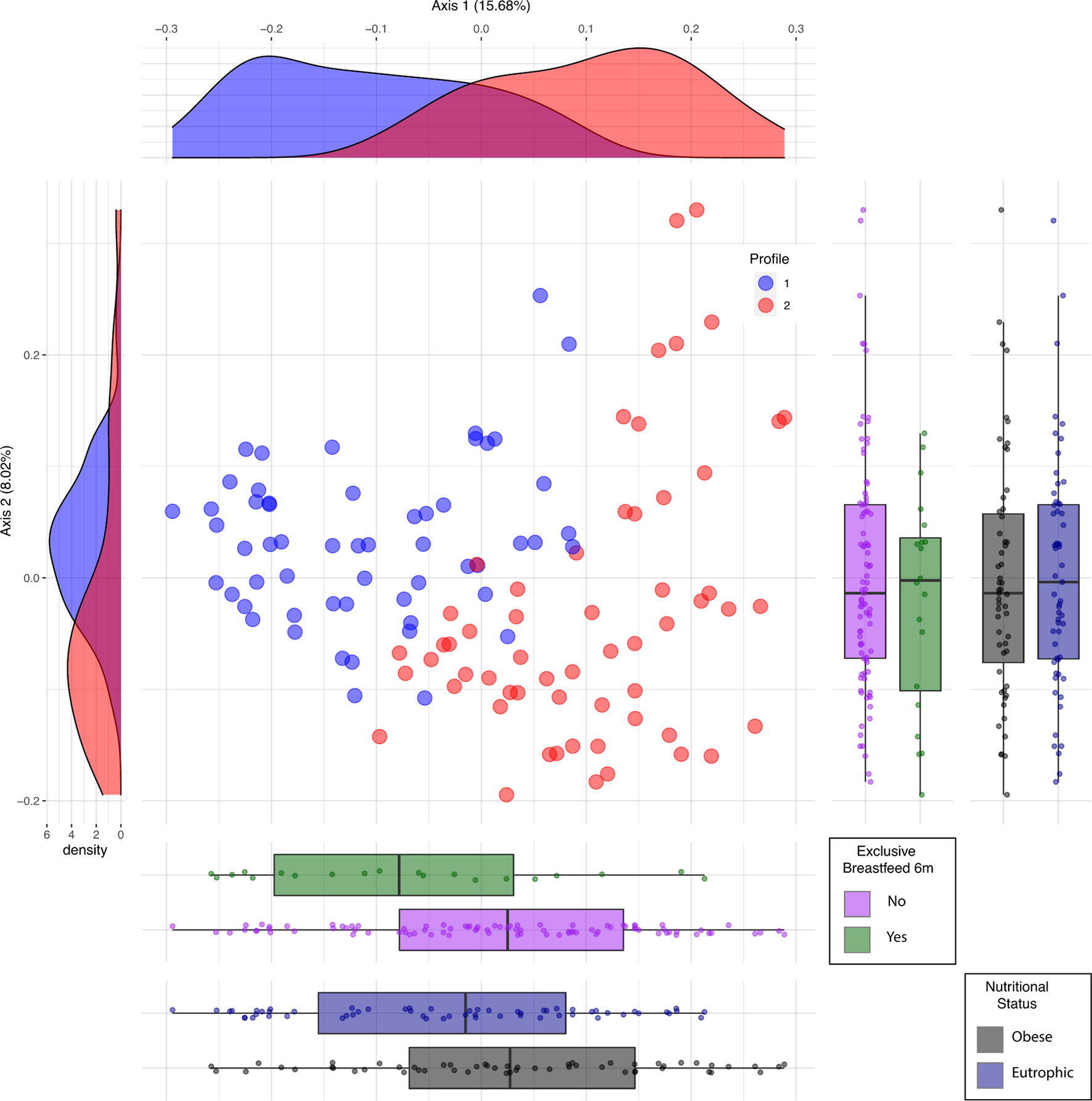
Figure 2 Profiles driven by Blautia (#1) and Prevotella (#2) identified by principal coordinates analysis (PCoA). #1 in blue is driven by Blautia; #2 in red is driven by Prevotella. Vertical boxplots represent the distribution of participants according to categories of breastfeeding and nutritional status (p < 0.05). Horizontal boxplots show the distribution of participants into profiles stratified by these categories.
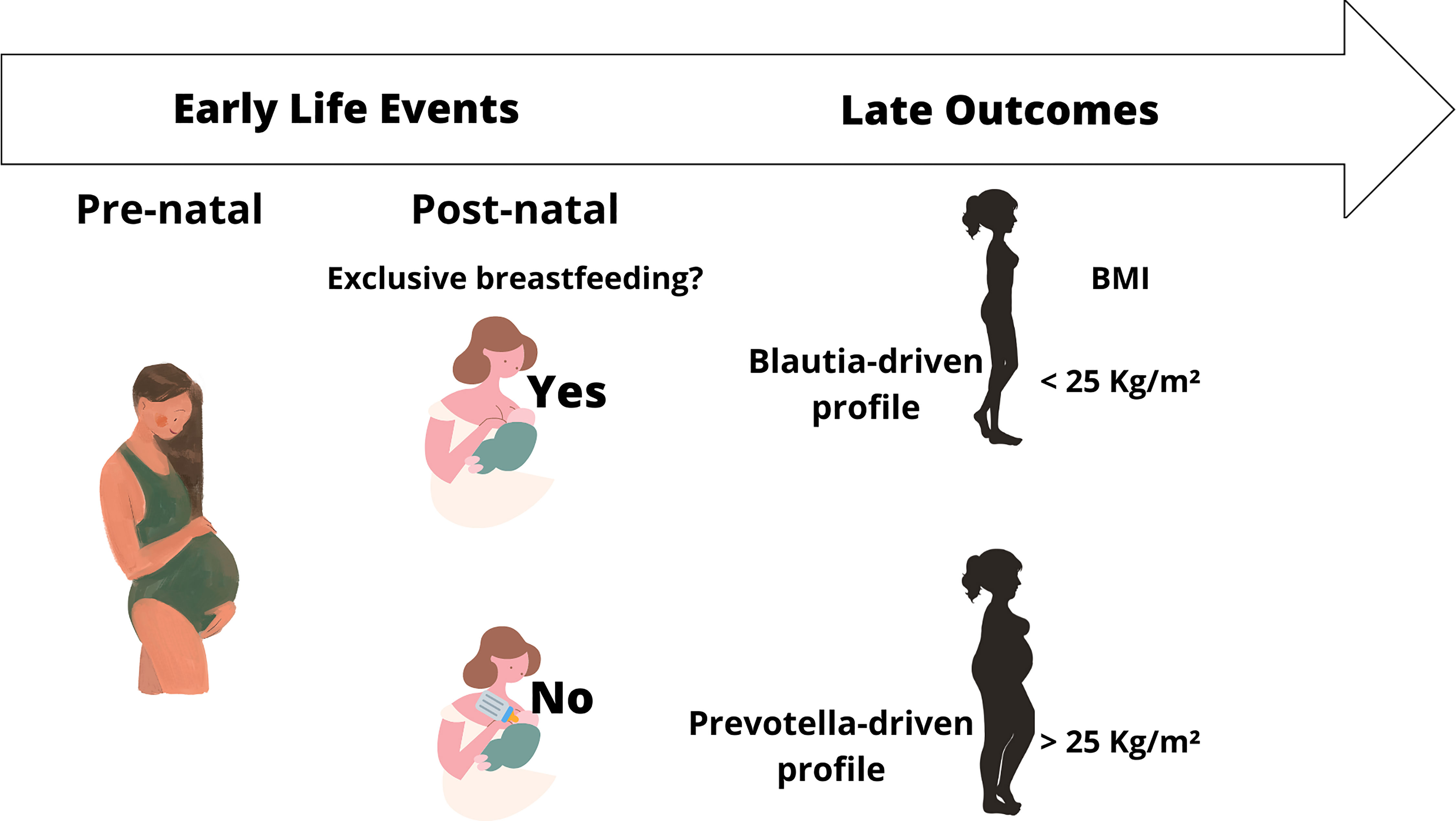
Figure 3 Impact of longer breastfeeding on microbiota composition and adult nutritional status. Credit: Figure produced using Canva graphic design platform (https://www.canva.com/) and brgfx/Freepik.
The differential abundance analysis identified genus drivers used to describe the bacterial composition in the profiles. The candidates present in at least 50% of the fecal samples are shown in the supplementary material (Figure S3), and bacteria differentially abundant between the 2 profiles were listed in Table S1.
Differences in some abundances between the profiles are depicted in Figure 4. Lachnoclostridium (Lachnospiraceae family, Clostridiales order, Clostridia class) abundance was higher in the Blautia profile, whereas several genera from Ruminococcaceae and Christensenellaceae families (both from Clostridiales order, Clostridia class) were predominant in the Prevotella profile.
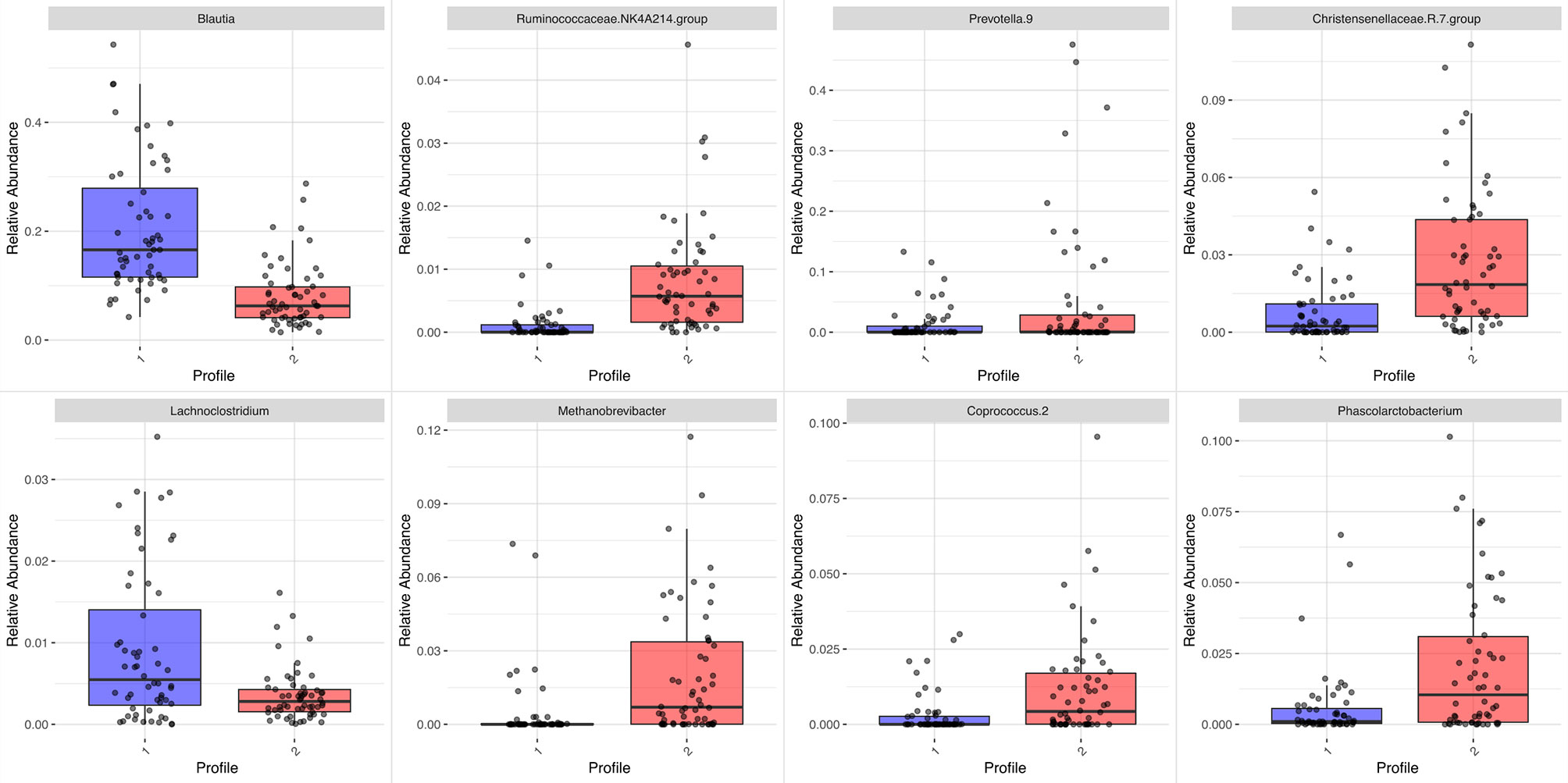
Figure 4 Boxplot of differential abundances of selected genera by profile (#1 in blue is driven by Blautia; #2 in red is driven by Prevotella). Adjusted p-value <0.05.
The main characteristics of participants by profile are given in Table 1. Gestational weight gain, type of delivery, and birth weight did not differ between the groups, but the rate of exclusive breastfeeding ≥6 months was higher in the Blautia- than that in the Prevotella-driven profile (21.4% vs. 6.9%, respectively, p = 0.04). Clinical and body composition variables of both groups were within normal ranges. Butyrate concentrations were undetectable for the whole sample.
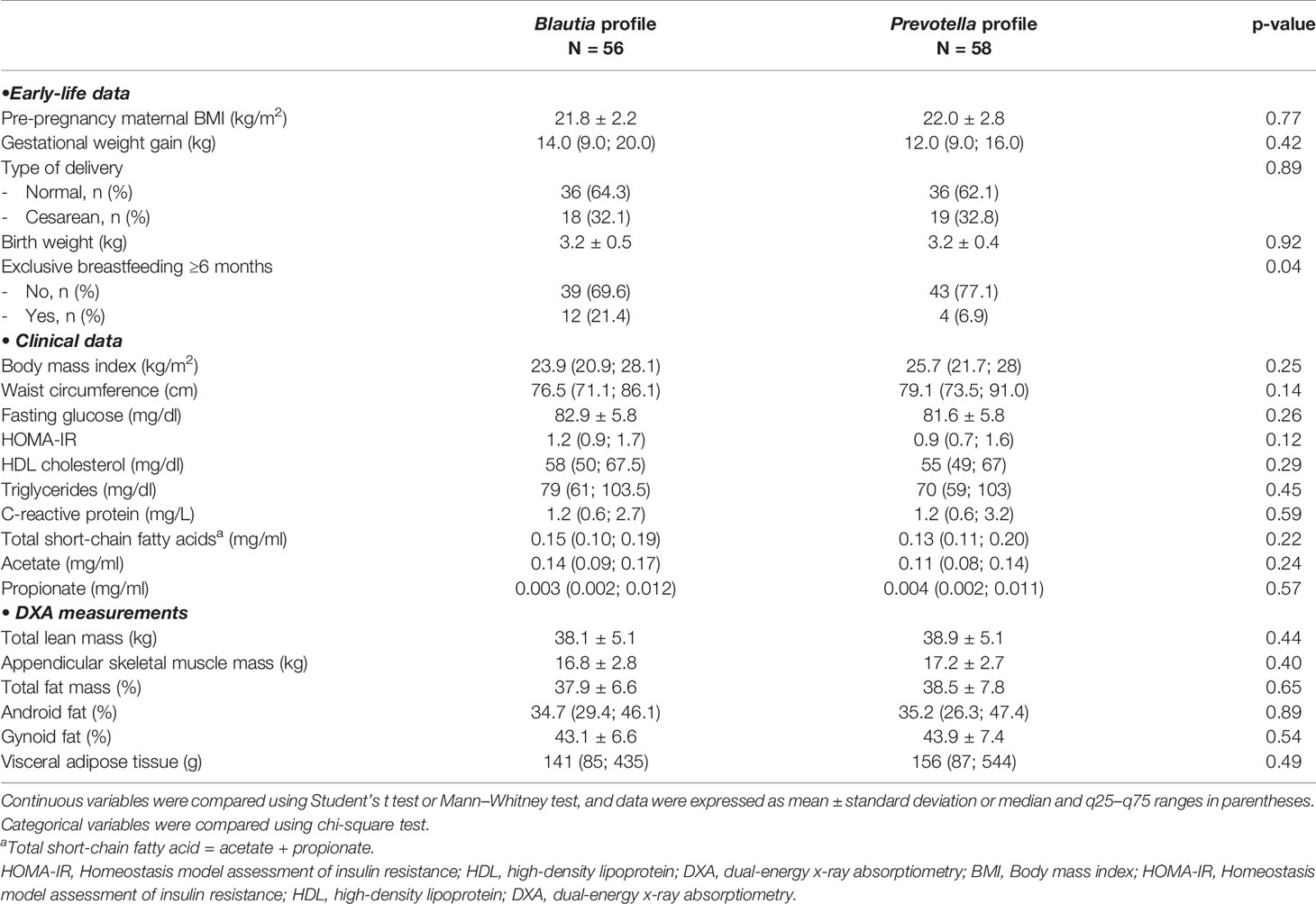
Table 1 Means (standard deviation) or medians (interquartile range) for clinical variables and body composition parameters of the 114 participants according to profile.
Dietary data of participants such as total energy and macronutrient and fatty acid intakes as a percentage of total energy did not differ, but median intakes of total, soluble, and insoluble fibers were higher in the Prevotella- than those in the Blautia-driven profile (Table 2). The dbRDA showed that the percentage of variance explained by diet was low (X-axis with 4.5% and Y-axis with 1.5%), being 94% explained by other factors. No significant correlation of fiber intake with SCFA concentrations and metabolic or body adiposity variables was detected.
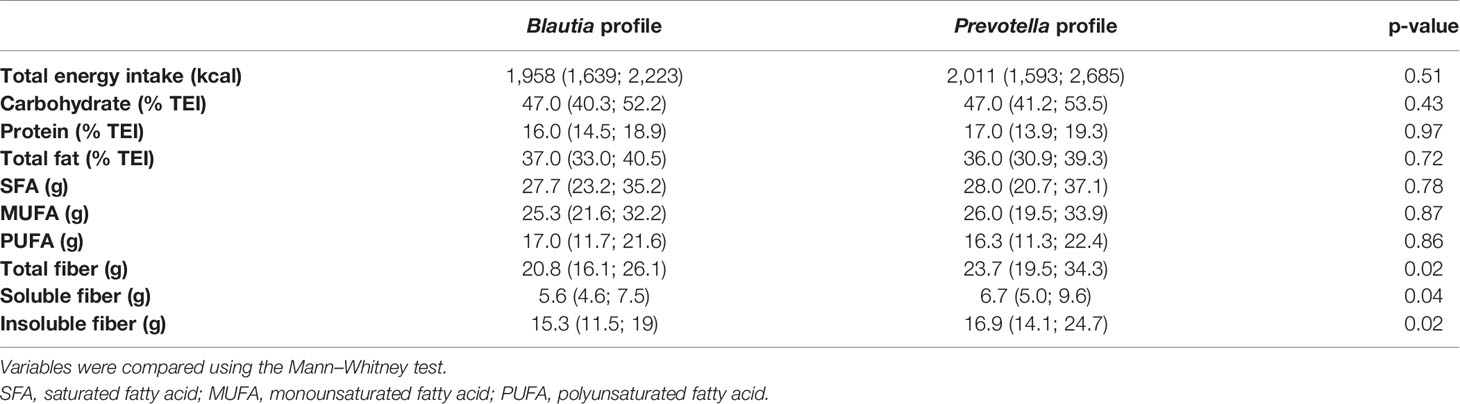
Table 2 Medians (interquartile range) of total energy intake (TEI) and dietary data of the 114 participants according to profile.
Discussion
This study explores the discussion regarding the influence of early-life events on gut microbiota composition in adulthood. A specific sample of women with literacy in nutrition was investigated. The associations suggested that longer breastfeeding impacts both microbiota composition and nutritional status in adulthood. By using a clustering approach to define microbiota profiles, in one profile driven by the genus Blautia, the same associations were confirmed. Both Blautia- and Prevotella-driven profiles are consistent with a healthy diet rich in fibers with an adequate macronutrient distribution and were therefore expected in the individuals studied. The findings in this homogeneous sample revealed the presence of macrostructures in the gut microbiota dominated by Blautia or Prevotella, SCFA-producing genera associated with beneficial metabolic effects. Interestingly, Blautia was associated with exclusive breastfeeding, whose relevance for gut colonization and body systems programming has been previously reported, as well as its health implications throughout the life span. Our findings not only reinforce the relevance of early feeding but also suggest an impact on gut colonization that persists into adulthood, contributing to a beneficial microbiota pattern. Furthering this knowledge could help in the prevention of chronic diseases.
For the overall sample, direct associations of some genera (Blautia, Anaerostipes, and Lachnoclostridium) of the family Lachnospiraceae with the recommended practice of long breastfeeding to prevent chronic diseases (including those related to excess body adiposity) were suggested. In fact, the profile analyses showed that the same genera were also associated with adequate nutritional status in adulthood. These findings contrast with previous reports of an association of Lachnoclostridium species with adiposity (Zhao L. et al., 2017; Sun et al., 2020; Nogal et al., 2021) but are in line with other studies in which Anaerostipes abundance was associated with a lower risk of type 2 diabetes (Yang et al., 2018). With regard to the family Ruminococcaceae, the present dbRDA initially suggested a relationship with unfavorable body adiposity distribution that was not confirmed when correlations to visceral adipose tissue (VAT) were tested. Although Ruminococcus, Anaerostipes, and Blautia produce SCFA (Vital et al., 2014; Koh et al., 2016), which have beneficial metabolic actions (Kasubuchi et al., 2015; Zhao et al., 2018; Müller et al., 2019), other controversial associations have been reported. In Mexican children, these genera were directly associated with obesity (Vazquez-Moreno et al., 2021). Inconsistencies have highlighted the need of improving knowledge about the intestinal bacteria assemblages of individuals from different geographical regions.
Using a clustering approach, early-life events and current characteristics of the sample were compared to verify associations suggesting underlying mechanisms of diseases. It is noteworthy that most participants engaged in physical activity regularly and consumed a fiber-rich diet, factors known to impact microbiota composition. These conditions have also been associated with anti-inflammatory status and favorable clinical profile (Hemmingsen et al., 2017; Nyberg et al., 2020). Normal mean values of C-reactive protein and insulin resistance index (HOMA-IR) indicated a low risk for metabolic disturbances in participants of both profiles. The findings of high abundances of Blautia and Prevotella were expected, since these genera belong to Lachnospiraceae and Prevotellaceae families that have the ability to degrade complex polysaccharides into SCFAs (Biddle et al., 2013; Eren et al., 2015). Measurements of SCFA in feces and blood have represented an indirect way of assessing the effect of fermentable carbohydrates’ intake. The higher the intake of these carbohydrates, the higher the SCFA concentration (So et al., 2018), but, in addition to the substrate availability, SCFA production is affected by intestinal transit time and microbiota composition (Macfarlane and Macfarlane, 2003). Characteristics of the microbiota of our participants should be contributing to improve the status of inflammation and insulin sensitivity, desirable for the prevention of cardiometabolic diseases (Kasubuchi et al., 2015; Zhao et al., 2018; Canfora et al., 2019). However, in the present study, no significant correlation was detected among fiber intake, SCFA concentrations, body adiposity, or metabolic variables. Additionally, the dbRDA showed a low percentage of variance explained by diet. The homogeneity and healthy characteristics of the sample as a whole may have precluded the detection of significant associations between these variables, as well as differences between participants from each profile.
Interestingly, comparisons of early-life events between the profiles showed that participants in the Blautia group had a higher rate of longer duration of exclusive breastfeeding. Considering the importance of gut colonization during this stage of life and given that these microorganisms coexist with the host throughout the life span (Milani et al., 2017), the association found might prove relevant. The first 1,000 days of life are considered a critical developmental window for programming systems and influencing the risk for long-term outcomes (Gluckman et al., 2005; Capra et al., 2021). In addition to the mode of delivery, growing evidence points to the role of early-life nutrition in shaping the offspring’s microbiota (Arrieta et al., 2014; Rodríguez, 2014). Bacteria are transferred through human milk and influence immune and metabolic homeostasis. Our results suggest that longer exposure to human milk might be associated with abundance of the Blautia genus. Despite limitations of linking distant factors with the gut microbiota of grown-up children and young adults, the hypothesis raised is feasible, considering the beneficial effects attributed to these bacteria. Breast milk composition is complex, containing nutrients, bacteria, and many other compounds. Oligosaccharides—present in human milk but not in most formula—serve as prebiotics, i.e., substrates for fermentation favoring the growth of beneficial bacteria such as the Bifidobacterium genus, which uses them to produce SCFA (Bridgman et al., 2017). The breastfed participants may have had their microbiota shaped to favor an abundance of certain commensal genera over others. In this respect, Blautia genus shares properties with Bifidobacterium in producing SCFAs and improving gut barrier functions. An interesting finding of our group previously suggested that breastfeeding duration could influence the offspring’s adherence to a prudent dietary pattern and metabolic parameters in adulthood (Eshriqui et al., 2019; Eshriqui et al., 2020). Another latent factor that could underlie the microbiota variability is the maternal and paternal BMI before conception (Eshriqui et al., 2021; Freitas et al., 2021), but, according to the PERMANOVA adjustments, there was no association between these maternal variables and the offspring’s microbiota structure.
A variety of exposures throughout life should have a role in modulating the microbiota of our participants. The current healthy lifestyle of individuals from the Blautia-driven profile may be contributing to an adequate BMI and normal biochemical profile. It is known that exercise-induced cardiometabolic benefits are in part gut microbiota-mediated (Chen et al., 2018), but there is also evidence on the associations of early-life events with obesity and related diseases (Ptashne, 2007; Garmendia et al., 2014; Cadenas-Sanchez et al., 2017). Lack of breastfeeding and exposure to formula were shown to increase the risk of obesity in infancy and adulthood (Dietz, 2001; Kelishadi and Farajian, 2014), with clear involvement of gut microbiota in this association. There was a predominance of participants with BMI <25 kg/m2 in the Blautia profile. This finding is congruent with evidence that butyrate (Berni Canani et al., 2016; Takahashi et al., 2016; Wang et al., 2018) and acetate produced by Blautia contribute to reduce obesity by regulating G-protein-coupled receptors (Kimura et al., 2013; Liu et al., 2021). In animals, weight gain prevention by SCFA supplementation (Lu et al., 2016) raises the possibility of a novel strategy for controlling human obesity. Our data are also in agreement with previous studies conducted in Spanish children (Benítez-Páez et al., 2020) and in Japanese adults (Ozato et al., 2019). A growing body of evidence indicates the potential on a deeper understanding of the “gut microbiota–host metabolism” interplay for managing prevalent diseases in different populations.
In some respects, the differential abundance analysis showed unexpected results. In the Blautia-driven profile, characterized by a higher proportion of lean individuals, Methanobrevibacter was less abundant. A previous study addressing this genus reported opposite results; however, the study in question involved an older sample of both sexes and had different purposes and methodological approaches (Schwiertz et al., 2010). Acetate-producing Lachnoclostridium was more abundant, in contrast with associations found for diet-induced obesity in animals (Zhao et al., 2017; Sun et al., 2020) and with VAT in female twins (Nogal et al., 2021). Some investigators have speculated that Lachnoclostridium could also be a Trimethylamine (TMA)-producing bacteria and, via the Trimethylamine N-oxide (TMAO) pathway, may increase the cardiometabolic risk (Schugar et al., 2017). In the Prevotella-driven profile, there was a higher abundance of acetate and butyrate-producing bacteria. The Christensenellaceae R7 group, Ruminococcaceae NK4A214, and Phascolarctobacterium have been associated with a favorable cardiometabolic profile. The Christensenellaceae R7 group was associated with less VAT and more lean mass in elderly people (Tavella et al., 2021), while the Christensenellaceae R7 group, Ruminococcaceae NK4A214, and Phascolarctobacterium were inversely correlated to glucose metabolism disturbance (Naderpoor et al., 2019; Chen et al., 2021). In our study, Coprococcus_2 was more abundant in the Prevotella-driven than the Blautia-driven profile. A high abundance of this genus has been described in women with polycystic ovary syndrome (Zhou et al., 2020) and high lifetime cardiovascular disease risk (Kelly et al., 2016). Therefore, it can be concluded that both bacterial profiles identified in the gut microbiota of healthy Brazilian women may include both beneficial and harmful bacteria. Rather than investigating the role of isolated bacteria for risk prediction, a better strategic approach might be to prevent diseases by focusing on the microbial balance and interactions in the host, submitted to multiple exposures during the life course in different habitats.
This study has limitations related to the sample size due to strict inclusion criteria and composition. The sample comprised highly educated women with a healthy clinical profile, precluding generalizing our results to other samples with different characteristics. The sample homogeneity likely led to the detection of fewer differences between profiles, despite using an accurate technique for assessing body compartments. Our study was not designed to establish a causal relationship between exposure and long-term outcomes. Memory bias was also a concern. In order to minimize this type of error, the study included only participants whose mothers were alive, since the evidence shows that mothers are able to report the early life of their offspring with acceptable precision almost 30 years later (Chin et al., 2017). Another limitation was the lack of information regarding several risk factors such as antibiotic use and stressful conditions known to influence microbiota composition from birth to adulthood.
In conclusion, findings in a bacterial profile driven by Blautia present in healthy Brazilian women reinforce that early-life events play a role in defining gut microbiota profile. While acknowledging the need for investigations with appropriate design to further explore this hypothesis, we highlight the relevance of exclusive breastfeeding for gut colonization in early life to guide the establishment of a protective microbiota against adiposity-related outcomes throughout life.
Data Availability Statement
The datasets presented in this study can be found in online repositories. The names of the repository/repositories and accession number(s) can be found below: https://www.ebi.ac.uk/ena, PRJEB49536.
Ethics Statement
The studies involving human participants were reviewed and approved by Comitê de Ética em Pesquisa UNICAMP (CAAE 79775817.4.1001.5404). The patients/participants provided their written informed consent to participate in this study.
Author Contributions
Contributed to conception and design: RB, AV, GR, BA-P, and SF. Contributed to acquisition, analysis, or interpretation: RB, AV, GR, FR, IS, MB, BG, and SF. Drafted the article: RB and SF. Critically revised the article: RB, AV, GR, BG, and SF. Gave final approval and agreed to be accountable for all aspects of work, ensuring integrity and accuracy: RB, AV, GR, FR, IS, MB, BA-P, BG, and SF.
Funding
This work was supported by the Foundation for Research Support of the State of São Paulo–FAPESP (grant 2018/11433-9, 2018/11401-0).
Conflict of Interest
The authors declare that the research was conducted in the absence of any commercial or financial relationships that could be construed as a potential conflict of interest.
Publisher’s Note
All claims expressed in this article are solely those of the authors and do not necessarily represent those of their affiliated organizations, or those of the publisher, the editors and the reviewers. Any product that may be evaluated in this article, or claim that may be made by its manufacturer, is not guaranteed or endorsed by the publisher.
Acknowledgments
We thank the Clinical Pathology Laboratory of “Hospital das Clínicas,” UNICAMP, and Growth and Body Composition Laboratory, Center for Investigation in Pediatrics, UNICAMP, for the scientific partnership. We also thank Vinícius Santos for assisting with clinical examinations.
Supplementary Material
The Supplementary Material for this article can be found online at: https://www.frontiersin.org/articles/10.3389/fcimb.2022.838750/full#supplementary-material
Supplementary Figure 1 | Infographic work of the overall methodology
Supplementary Figure 2 | Redundancy analysis (RDA) highlighting profile drivers
Supplementary Figure 3 | Boxplot of differential abundances of genera by profile (#1 in blue is driven by Blautia; #2 in red is driven by Prevotella). Adjusted p-value <0.05.
Supplementary Table 1 | Differential abundances of bacteria by profile
References
Arrieta, M. C., Stiemsma, L. T., Amenyogbe, N., Brown, E. M., Finlay, B. (2014). The Intestinal Microbiome in Early Life: Health and Disease. Front. Immunol. 5. doi: 10.3389/fimmu.2014.00427
Arumugam, M., Raes, J., Pelletier, E., Le Paslier, D., Yamada, T., Mende, D. R., et al. (2011). Enterotypes of the Human Gut Microbiome. Nature 473 (7346), 174–180. doi: 10.1038/nature09944
Barker, D. J. (1990). The Fetal and Infant Origins of Adult Disease. BMJ 301 (6761), 1111. doi: 10.1136/bmj.301.6761.1111
Bell, K. A., Wagner, C. L., Feldman, H. A., Shypailo, R. J., Belfort, M. B. (2017). Associations of Infant Feeding With Trajectories of Body Composition and Growth. Am. J. Clin. Nutr. 106 (2), 491–498. doi: 10.3945/ajcn.116.151126
Benítez-Páez, A., Gómez Del Pugar, E. M., López-Almela, I., Moya-Pérez, Á., Codoñer-Franch, P., Sanz, Y. (2020). Depletion of Blautia Species in the Microbiota of Obese Children Relates to Intestinal Inflammation and Metabolic Phenotype Worsening. mSystems 5 (2), e00857–e00819. doi: 10.1128/mSystems.00857-19
Berni Canani, R., Sangwan, N., Stefka, A. T., Nocerino, R., Paparo, L., Aitoro, R., et al. (2016). Lactobacillus Rhamnosus GG-supplemented Formula Expands Butyrate-Producing Bacterial Strains in Food Allergic Infants. ISME J. 10 (3), 742–750. doi: 10.1038/ismej.2015.151
Biasucci, G., Rubini, M., Riboni, S., Morelli, L., Bessi, E., Retetangos, C. (2010). Mode of Delivery Affects the Bacterial Community in the Newborn Gut. Early Hum. Dev. 86 Suppl 1, 13–15. doi: 10.1016/j.earlhumdev.2010.01.004
Biddle, A., Stewart, L., Blanchard, J., Leschine, S. (2013). Untangling the Genetic Basis of Fibrolytic Specialization by Lachnospiraceae and Ruminococcaceae in Diverse Gut Communities. Diversity 5 (3), 627–640. doi: 10.3390/d5030627
Block, T., El-Osta, A. (2017). Epigenetic Programming, Early Life Nutrition and the Risk of Metabolic Disease. Atherosclerosis 266, 31–40. doi: 10.1016/j.atherosclerosis.2017.09.003
Bouter, K. E., van Raalte, D. H., Groen, A. K., Nieuwdorp, M. (2017). Role of the Gut Microbiome in the Pathogenesis of Obesity and Obesity-Related Metabolic Dysfunction. Gastroenterology 152 (7), 1671–1678. doi: 10.1053/j.gastro.2016.12.048
Bridgman, S. L., Azad, M. B., Field, C. J., Haqq, A. M., Becker, A. B., Mandhane, P. J., et al. (2017). Fecal Short-Chain Fatty Acid Variations by Breastfeeding Status in Infants at 4 Months: Differences in Relative Versus Absolute Concentrations. Front. Nutr. 4. doi: 10.3389/fnut.2017.00011
Cadenas-Sanchez, C., Henriksson, P., Henriksson, H., Delisle Nyström, C., Pomeroy, J., Ruiz, J. R., et al. (2017). Parental Body Mass Index and its Association With Body Composition, Physical Fitness and Lifestyle Factors in Their 4-Year-Old Children: Results From the MINISTOP Trial. Eur. J. Clin. Nutr. 71 (10), 1200–1205. doi: 10.1038/ejcn.2017.62
Canfora, E. E., Meex, R., Venema, K., Blaak, E. E. (2019). Gut Microbial Metabolites in Obesity, NAFLD and T2DM. Nature Reviews. Endocrinology 15 (5), 261–273. doi: 10.1038/s41574-019-0156-z
Capra, M. E., Pederiva, C., Viggiano, C., De Santis, R., Banderali, G., Biasucci, G. (2021). Nutritional Approach to Prevention and Treatment of Cardiovascular Disease in Childhood. Nutrients 13 (7), 2359. doi: 10.3390/nu13072359
Cheng, M., Ning, K. (2019). Stereotypes About Enterotype: The Old and New Ideas. Genomics Proteomics Bioinf. 17 (1), 4–12. doi: 10.1016/j.gpb.2018.02.004
Chen, J., Guo, Y., Gui, Y., Xu, D. (2018). Physical Exercise, Gut, Gut Microbiota, and Atherosclerotic Cardiovascular Diseases. Lipids Health Dis. 17 (1), 17. doi: 10.1186/s12944-017-0653-9
Chen, Z., Radjabzadeh, D., Chen, L., Kurilshikov, A., Kavousi, M., Ahmadizar, F., et al. (2021). Association of Insulin Resistance and Type 2 Diabetes With Gut Microbial Diversity: A Microbiome-Wide Analysis From Population Studies. JAMA network Open 4 (7), e2118811. doi: 10.1001/jamanetworkopen.2021.18811
Cheshmeh, S., Nachvak, S. M., Rezvani, N., Saber, A. (2020). Effects of Breastfeeding and Formula Feeding on the Expression Level of FTO, CPT1A and PPAR-α Genes in Healthy Infants. Diabetes, Metabolic Syndrome and Obesity:. Targets Ther. 13, 2227–2237. doi: 10.2147/DMSO.S252122
Chin, H. B., Baird, D. D., McConnaughey, D. R., Weinberg, C. R., Wilcox, A. J., Jukic, A. M. (2017). Long-Term Recall of Pregnancy-Related Events. Epidemiology 28 (4), 575–579. doi: 10.1097/EDE.0000000000000660
Craig, C. L., Marshall, A. L., Sjöström, M., Bauman, A. E., Booth, M. L., Ainsworth, B. E., et al. (2003). International Physical Activity Questionnaire: 12-Country Reliability and Validity. Med. Sci. sports exercise. 35 (8), 1381–1395. doi: 10.1249/01.MSS.0000078924.61453.FB
David, L. A., Maurice, C. F., Carmody, R. N., Gootenberg, D. B., Button, J. E., Wolfe, B. E., et al. (2014). Diet Rapidly and Reproducibly Alters the Human Gut Microbiome. Nature 505 (7484), 559–563. doi: 10.1038/nature12820
De Filippo, C., Cavalieri, D., Di Paola, M., Ramazzotti, M., Poullet, J. B., Massart, S., et al. (2010). Impact of Diet in Shaping Gut Microbiota Revealed by a Comparative Study in Children From Europe and Rural Africa. Proc. Natl. Acad. Sci. United States America 107 (33), 14691–14696. doi: 10.1073/pnas.1005963107
de Moraes, A. C., Fernandes, G. R., da Silva, I. T., Almeida-Pititto, B., Gomes, E. P., Pereira, A. D., et al. (2017). Enterotype may Drive the Dietary-Associated Cardiometabolic Risk Factors. Front. Cell. Infection Microbiol. 7. doi: 10.3389/fcimb.2017.00047
Dietz, W. H. (2001). Breastfeeding may Help Prevent Childhood Overweight. Jama 285 (19), 2506–2507. doi: 10.1001/jama.285.19.2506
Dray, S., Dufour, A. B. (2007). The Ade4 Package: Implementing the Duality Diagram for Ecologists. J. Stat. Software 22 (4), 1–20. doi: 10.18637/jss.v022.i04
Eren, A. M., Sogin, M. L., Morrison, H. G., Vineis, J. H., Fisher, J. C., Newton, R. J., et al. (2015). A Single Genus in the Gut Microbiome Reflects Host Preference and Specificity. ISME J. 9 (1), 90–100. doi: 10.1038/ismej.2014.97
Eshriqui, I., Folchetti, L. D., Valente, A., de Almeida-Pititto, B., Ferreira, S. (2019). Breastfeeding Duration Is Associated With Offspring's Adherence to Prudent Dietary Pattern in Adulthood: Results From the Nutritionist's Health Study. Journal of Developmental Origins of Health and Disease 11 (2), 136–145. doi: 10.1017/S204017441900031X
Eshriqui, I., Valente, A., Folchetti, L. D., de Almeida-Pititto, B., Ferreira, S. (2021). Pre-Pregnancy BMI Is Associated with Offspring Body Composition in Adulthood Before Adiposity-Related Disorders: A Retrospective Cohort. Public Health Nutrition 24 (6), 1296–1303. doi: 10.1017/S1368980020005285
Eshriqui, I., Folchetti, L. D., Valente, A., de Almeida-Pititto, B., Ferreira, S. (2020). Pre-Pregnancy BMI Is Associated With Offspring Body Composition in Adulthood Before Adiposity-Related Disorders: A Retrospective Cohort. Public Health Nutrition 24 (6), 1296–1303. doi: 10.1017/S1368980020005285
Fan, Y., Pedersen, O. (2021). Gut Microbiota in Human Metabolic Health and Disease. Nature Reviews. Microbiology 19 (1), 55–71. doi: 10.1038/s41579-020-0433-9
Folchetti, L. G., Silva, I. T., Almeida-Pititto, B. D., Ferreira, S. R. (2016). Nutritionists’ Health Study Cohort: A Web-Based Approach of Life Events, Habits and Health Outcomes. BMJ Open 6 (8), e012081. doi: 10.1136/bmjopen-2016-012081
Freitas, R., Vasques, A., Ribeiro, F. B., Solar, I., Hanada, A. S., Barbosa, M. G., et al (2021). Maternal and Paternal Obesity Are Associated With Offspring Obestatin Levels in the Nutritionists' Health Study. Nutrition (Burbank, Los Angeles County, Calif.) 83, 111067. doi: 10.1016/j.nut.2020.111067
Garmendia, M. L., Corvalan, C., Uauy, R. (2014). Assessing the Public Health Impact of Developmental Origins of Health and Disease (DohaD) Nutrition Interventions. Ann. Nutr. Metab. 64 (3-4), 226–230. doi: 10.1159/000365024
Gluckman, P. D., Cutfield, W., Hofman, P., Hanson, M. A. (2005). The Fetal, Neonatal, and Infant Environments-the Long-Term Consequences for Disease Risk. Early Hum. Dev. 81 (1), 51–59. doi: 10.1016/j.earlhumdev.2004.10.003
Gorvitovskaia, A., Holmes, S. P., Huse, S. M. (2016). Interpreting Prevotella and Bacteroides as Biomarkers of Diet and Lifestyle. Microbiome 4, 15. doi: 10.1186/s40168-016-0160-7
Haytowitz, D. B., Ahuja, J. K. C., Wu, X., Somanchi, M., Nickle, M., Nguyen, Q. A., et al. (2019). Usda National Nutrient Database for Standard Reference, Legacy Release (Beltsville: Nutrient Data Laboratory, Beltsville Human Nutrition Research Center, ARS, USDA). Available at: https://data.nal.usda.gov/dataset/usda-national-nutrient-database-standard-reference-legacy-release.
Hemmingsen, B., Gimenez-Perez, G., Mauricio, D., Roqué I Figuls, M., Metzendorf, M. I., Richter, B. (2017). Diet, Physical Activity or Both for Prevention or Delay of Type 2 Diabetes Mellitus and its Associated Complications in People at Increased Risk of Developing Type 2 Diabetes Mellitus. Cochrane Database Systematic Rev. 12 (12), CD003054. doi: 10.1002/14651858.CD003054.pub4
Kapourchali, F. R., Cresci, G. (2020). Early-Life Gut Microbiome-the Importance of Maternal and Infant Factors in its Establishment. Nutr. Clin. Practice: Off. Publ. Am. Soc. Parenteral Enteral Nutr. 35 (3), 386–405. doi: 10.1002/ncp.10490
Kasubuchi, M., Hasegawa, S., Hiramatsu, T., Ichimura, A., Kimura, I. (2015). Dietary Gut Microbial Metabolites, Short-Chain Fatty Acids, and Host Metabolic Regulation. Nutrients 7 (4), 2839–2849. doi: 10.3390/nu7042839
Kelishadi, R., Farajian, S. (2014). The Protective Effects of Breastfeeding on Chronic non-Communicable Diseases in Adulthood: A Review of Evidence. Advanced Biomed. Res. 3, 3. doi: 10.4103/2277-9175.124629
Kelly, T. N., Bazzano, L. A., Ajami, N. J., He, H., Zhao, J., Petrosino, J. F., et al (2016). Gut Microbiome Associates With Lifetime Cardiovascular Disease Risk Profile Among Bogalusa Heart Study Participants. Circulation Res. 119 (8), 956—964. doi: 10.1161/CIRCRESAHA.116.309219
Kimura, I., Ozawa, K., Inoue, D., Imamura, T., Kimura, K., Maeda, T., et al. (2013). The Gut Microbiota Suppresses Insulin-Mediated Fat Accumulation Via the Short-Chain Fatty Acid Receptor GPR43. Nat. Commun. 4, 1829. doi: 10.1038/ncomms2852
Koh, A., De Vadder, F., Kovatcheva-Datchary, P., Bäckhed, F. (2016). From Dietary Fiber to Host Physiology: Short-chain Fatty Acids as Key Bacterial Metabolites. Cell 165 (6), 1332–1345. doi: 10.1016/j.cell.2016.05.041
Le Doare, K., Holder, B., Bassett, A., Pannaraj, P. S. (2018). Mother’s Milk: A Purposeful Contribution to the Development of the Infant Microbiota and Immunity. Front. Immunol. 9. doi: 10.3389/fimmu.2018.00361
Legendre, P., Anderson, M. J. (1999). Distance-Based Redundancy Analysis: Testing Multispecies Responses in Multifactorial Ecological Experiments. Ecol. Monogr. 69 (1), 1–24. doi: 10.1890/0012-9615(1999)069[0001:DBRATM]2.0.CO;2
Levy, M., Kolodziejczyk, A. A., Thaiss, C. A., Elinav, E. (2017). Dysbiosis and the Immune System. Nature Reviews. Immunology. 17 (4), 219–232. doi: 10.1038/nri.2017.7
Liu, X., Mao, B., Gu, J., Wu, J., Cui, S., Wang, G., et al. (2021). Blautia - A New Functional Genus With Potential Probiotic Properties? Gut Microbes 13 (1), 1–21. doi: 10.1080/19490976.2021.1875796
Love, M. I., Huber, W., Anders, S. (2014). Moderated Estimation of Fold Change and Dispersion for RNA-seq Data With Deseq2. Genome Biol. 15 (12), 550. doi: 10.1186/s13059-014-0550-8
Lu, Y., Fan, C., Li, P., Lu, Y., Chang, X., Qi, K. (2016). Short Chain Fatty Acids Prevent High-Fat-Diet-Induced Obesity in Mice by Regulating G Protein-Coupled Receptors and Gut Microbiota. Sci. Rep. 6, 37589. doi: 10.1038/srep37589
Macfarlane, S., Macfarlane, G. T. (2003). Regulation of Short-Chain Fatty Acid Production. Proc. Nutr. Soc. 62 (1), 67–72. doi: 10.1079/PNS2002207
Mailing, L. J., Allen, J. M., Buford, T. W., Fields, C. J., Woods, J. A. (2019). Exercise and the Gut Microbiome: A Review of the Evidence, Potential Mechanisms, and Implications for Human Health. Exercise Sport Sci. Rev. 47 (2), 75–85. doi: 10.1249/JES.0000000000000183
Martin, R., Makino, H., Cetinyurek Yavuz, A., Ben-Amor, K., Roelofs, M., Ishikawa, E., et al. (2016). Early-Life Events, Including Mode of Delivery and Type of Feeding, Siblings and Gender, Shape the Developing Gut Microbiota. PloS One 11 (6), e0158498. doi: 10.1371/journal.pone.0158498
Matsudo, S., Araújo, T., Marsudo, V., Andrade, D., Andrade, E., Braggion, G. (2001). Questionário Internacional De Atividade Fisica (IPAQ): Estudo De Validade E Reprodutibilidade No Brasil. Rev. Bras. Atividade Física e Saúde 6 (2), 5–18. doi: 10.12820/rbafs.v.6n2p5-18
Matthews, D. R., Hosker, J. P., Rudenski, A. S., Naylor, B. A., Treacher, D. F., Turner, R. C. (1985). Homeostasis Model Assessment: Insulin Resistance and Beta-Cell Function From Fasting Plasma Glucose and Insulin Concentrations in Man. Diabetologia 28 (7), 412–419. doi: 10.1007/BF00280883
Meijnikman, A. S., Gerdes, V. E., Nieuwdorp, M., Herrema, H. (2018). Evaluating Causality of Gut Microbiota in Obesity and Diabetes in Humans. Endocrine Rev. 39 (2), 133–153. doi: 10.1210/er.2017-00192
Milani, C., Duranti, S., Bottacini, F., Casey, E., Turroni, F., Mahony, J., et al. (2017). The First Microbial Colonizers of the Human Gut: Composition, Activities, and Health Implications of the Infant Gut Microbiota. Microbiol. Mol. Biol. Reviews: MMBR. 81 (4), e00036-17. doi: 10.1128/MMBR.00036-17
Müller, M., Hernández, M., Goossens, G. H., Reijnders, D., Holst, J. J., Jocken, J., et al. (2019). Circulating But Not Faecal Short-Chain Fatty Acids are Related to Insulin Sensitivity, Lipolysis and GLP-1 Concentrations in Humans. Sci. Rep. 9 (1), 12515. doi: 10.1038/s41598-019-48775-0
Naderpoor, N., Mousa, A., Gomez-Arango, L. F., Barrett, H. L., Dekker Nitert, M., de Courten, B. (2019). Faecal Microbiota is Related to Insulin Sensitivity and Secretion in Overweight or Obese Adults. J. Clin. Med. 8 (4), 452. doi: 10.3390/jcm8040452
Nogal, A., Louca, P., Zhang, X., Wells, P. M., Steves, C. J., Spector, T. D., et al. (2021). Circulating Levels of the Short-Chain Fatty Acid Acetate Mediate the Effect of the Gut Microbiome on Visceral Fat. Front. Microbiol. 12. doi: 10.3389/fmicb.2021.711359
Nyberg, S. T., Singh-Manoux, A., Pentti, J., Madsen, I., Sabia, S., Alfredsson, L., et al. (2020). Association of Healthy Lifestyle With Years Lived Without Major Chronic Diseases. Jama 180 (5), 760–768. doi: 10.1001/jamainternmed.2020.0618
Ozato, N., Saito, S., Yamaguchi, T., Katashima, M., Tokuda, I., Sawada, K., et al. (2019). Blautia Genus Associated With Visceral Fat Accumulation in Adults 20-76 Years of Age. NPJ Biofilms Microbiomes. 5 (1), 28. doi: 10.1038/s41522-019-0101-x
Penington, J. S., Penno, M., Ngui, K. M., Ajami, N. J., Roth-Schulze, A. J., Wilcox, S. A., et al. (2018). Influence of Fecal Collection Conditions and 16S rRNA Gene Sequencing at Two Centers on Human Gut Microbiota Analysis. Sci. Rep. 8 (1), 4386. doi: 10.1038/s41598-018-22491-7
Pires, D. E. V., Oliveira, F. S., Correa, F. B., Morais, D. K., Fernandes, G. R. (2018). Tag. ME: Taxonomic Assignment of Genetic Markers for Ecology. BioRxiv, 263293. doi: 10.1101/263293
Ptashne, M. (2007). On the Use of the Word ‘Epigenetic’. Curr. biology: CB 17 (7), R233–R236. doi: 10.1016/j.cub.2007.02.030
Qin, J., Li, R., Raes, J., Arumugam, M., Burgdorf, K. S., Manichanh, C., et al. (2010). A Human Gut Microbial Gene Catalogue Established by Metagenomic Sequencing. Nature 464 (7285), 59–65. doi: 10.1038/nature08821
Ravelli, G. P., Stein, Z. A., Susser, M. W. (1976). Obesity in Young Men After Famine Exposure In Utero and Early Infancy. New Engl. J. Med. 295 (7), 349–353. doi: 10.1056/NEJM197608122950701
Rodríguez, J. M. (2014). The Origin of Human Milk Bacteria: Is There a Bacterial Entero-Mammary Pathway During Late Pregnancy and Lactation? Adv. Nutr. 5 (6), 779–784. doi: 10.3945/an.114.007229. (Bethesda, Md.).
Rodríguez, J. M., Murphy, K., Stanton, C., Ross, R. P., Kober, O. I., Juge, N., et al. (2015). The Composition of the Gut Microbiota Throughout Life, With an Emphasis on Early Life. Microbial Ecol. Health Dis. 26, 26050. doi: 10.3402/mehd.v26.26050
Rosenbaum, M., Knight, R., Leibel, R. L. (2015). The Gut Microbiota in Human Energy Homeostasis and Obesity. Trends Endocrinol. Metab. 26 (9), 493–501. doi: 10.1016/j.tem.2015.07.002
Schwiertz, A., Taras, D., Schäfer, K., Beijer, S., Bos, N. A., Donus, C., et al. (2010). Microbiota and SCFA in Lean and Overweight Healthy Subjects. Obesity (Silver Spring, Md.) 18 (1), 190–195. doi: 10.1038/oby.2009.167
Selem, S. S., Carvalho, A. M., Verly-Junior, E., Carlos, J. V., Teixeira, J. A., Marchioni, D. M., et al. (2014). Validity and Reproducibility of a Food Frequency Questionnaire for Adults of São Paulo, Brazil. Braz. J. Epidemiol. 17 (4), 852–859. doi: 10.1590/1809-4503201400040005
Sender, R., Fuchs, S., Milo, R. (2016). Revised Estimates for the Number of Human and Bacteria Cells in the Body. PloS Biol. 14 (8), e1002533. doi: 10.1371/journal.pbio.1002533
Shen, J., Obin, M. S., Zhao, L. (2013). The Gut Microbiota, Obesity and Insulin Resistance. Mol. Aspects Med. 34 (1), 39–58. doi: 10.1016/j.mam.2012.11.001
Schugar, R. C., Shih, D. M., Warrier, , Helsley, M. R.N., Burrows, A., Ferguson, D., et al (2017). The TMAO-Producing Enzyme Flavin-Containing Monooxygenase 3 Regulates Obesity and the Beiging of White Adipose Tissue. Cell Reports 19 (12),2451—61. doi: 10.1016/j.celrep.2017.05.077
Sommer, F., Anderson, J. M., Bharti, R., Raes, J., Rosenstiel, P. (2017). The Resilience of the Intestinal Microbiota Influences Health and Disease. Nature Reviews. Microbiology 15 (10), 630–638. doi: 10.1038/nrmicro.2017.58
So, D., Whelan, K., Rossi, M., Morrison, M., Holtmann, G., Kelly, J. T., et al. (2018). Dietary Fiber Intervention on Gut Microbiota Composition in Healthy Adults: A Systematic Review and Meta-Analysis. Am. J. Clin. Nutr. 107 (6), 965–983. doi: 10.1093/ajcn/nqy041
Sun, X., Zhao, H., Liu, Z., Sun, X., Zhang, D., Wang, S., et al. (2020). Modulation of Gut Microbiota by Fucoxanthin During Alleviation of Obesity in High-Fat Diet-Fed Mice. J. Agric. Food Chem. 68 (18), 5118–5128. doi: 10.1021/acs.jafc.0c01467
Takahashi, K., Nishida, A., Fujimoto, T., Fujii, M., Shioya, M., Imaeda, H., et al. (2016). Reduced Abundance of Butyrate-Producing Bacteria Species in the Fecal Microbial Community in Crohn’s Disease. Digestion 93 (1), 59–65. doi: 10.1159/000441768
Tavella, T., Rampelli, S., Guidarelli, G., Bazzocchi, A., Gasperini, C., Pujos-Guillot, E., et al. (2021). Elevated Gut Microbiome Abundance of Christensenellaceae, Porphyromonadaceae and Rikenellaceae Is Associated With Reduced Visceral Adipose Tissue and Healthier Metabolic Profile in Italian Elderly. Gut Microbes 13 (1), 1–19. doi: 10.1080/19490976.2021.1880221
Vazquez-Moreno, M., Perez-Herrera, A., Locia-Morales, D., Dizzel, S., Meyre, D., Stearns, J. C., et al. (2021). Association of Gut Microbiome With Fasting Triglycerides, Fasting Insulin and Obesity Status in Mexican Children. Pediatr. Obes. 16 (5), e12748. doi: 10.1111/ijpo.12748
Vital, M., Howe, A. C., Tiedje, J. M. (2014). Revealing the Bacterial Butyrate Synthesis Pathways by Analyzing (Meta)Genomic Data. mBio 5 (2), e00889. doi: 10.1128/mBio.00889-14
Wang, R., Fan, C., Fan, X., Zhao, Y., Wang, Y., Li, P., et al. (2019). A Fast and Accurate Way to Determine Short Chain Fatty Acids in Human Serum by GC–MS and Their Distribution in Children With Digestive Diseases. Chromatographia 83 (2), 273–286. doi: 10.1007/s10337-019-03831-9
Wang, Z., Zolnik, C. P., Qiu, Y., Usyk, M., Wang, T., Strickler, H. D., et al. (2018). Comparison of Fecal Collection Methods for Microbiome and Metabolomics Studies. Front. Cell. Infection Microbiol. 8. doi: 10.3389/fcimb.2018.00301
World Health Organization (2015). Obesity: Preventing and Managing the Global Epidemic. Report of a WHO Consulation (WHO Technical Report Series 894) 2000. Available at: https://www.who.int/nutrition/publications/obesity/WHO_TRS_894/en/.
Wu, G. D., Chen, J., Hoffmann, C., Bittinger, K., Chen, Y. Y., Keilbaugh, S. A., et al. (2011). Linking Long-Term Dietary Patterns With Gut Microbial Enterotypes. Science 334 (6052), 105–108. doi: 10.1126/science.1208344
Wu, J., Wang, K., Wang, X., Pang, Y., Jiang, C. (2021). The Role of the Gut Microbiome and its Metabolites in Metabolic Diseases. Protein Cell. 12 (5), 360–373. doi: 10.1007/s13238-020-00814-7
Yang, Q., Lin, S. L., Kwok, M. K., Leung, G. M., Schooling, C. M. (2018). The Roles of 27 Genera of Human Gut Microbiota in Ischemic Heart Disease, Type 2 Diabetes Mellitus, and Their Risk Factors: A Mendelian Randomization Study. Am. J. Epidemiol. 187 (9), 1916–1922. doi: 10.1093/aje/kwy096
Zhao, Y., Liu, J., Hao, W., Zhu, H., Liang, N., He, Z., et al. (2017). Structure-Specific Effects of Short-Chain Fatty Acids on Plasma Cholesterol Concentration in Male Syrian Hamsters. J. Agric. Food Chem. 65 (50), 10984–10992. doi: 10.1021/acs.jafc.7b04666
Zhao, L., Zhang, F., Ding, X., Wu, G., Lam, Y. Y., Wang, X., et al. (2018). Gut Bacteria Selectively Promoted by Dietary Fibers Alleviate Type 2 Diabetes. Science 359 (6380), 1151–1156. doi: 10.1126/science.aao5774
Keywords: gut microbiota, early-life events, DOHAD, breastfeeding, nutritional status
Citation: Freitas RGBdON, Vasques ACJ, Fernandes GdR, Ribeiro FB, Solar I, Barbosa MG, Pititto BdA-, Geloneze B and Ferreira SRG (2022) Associations of Blautia Genus With Early-Life Events and Later Phenotype in the NutriHS. Front. Cell. Infect. Microbiol. 12:838750. doi: 10.3389/fcimb.2022.838750
Received: 18 December 2021; Accepted: 11 April 2022;
Published: 12 May 2022.
Edited by:
Veeranoot Nissapatorn, Walailak University, ThailandReviewed by:
Alexander V. Tyakht, Institute of Gene Biology (RAS), RussiaSelvasankar Murugesan, Sidra Medicine, Qatar
Copyright © 2022 Freitas, Vasques, Fernandes, Ribeiro, Solar, Barbosa, Pititto, Geloneze and Ferreira. This is an open-access article distributed under the terms of the Creative Commons Attribution License (CC BY). The use, distribution or reproduction in other forums is permitted, provided the original author(s) and the copyright owner(s) are credited and that the original publication in this journal is cited, in accordance with accepted academic practice. No use, distribution or reproduction is permitted which does not comply with these terms.
*Correspondence: Sandra Roberta G. Ferreira, c2FuZHJhZnZAdXNwLmJy