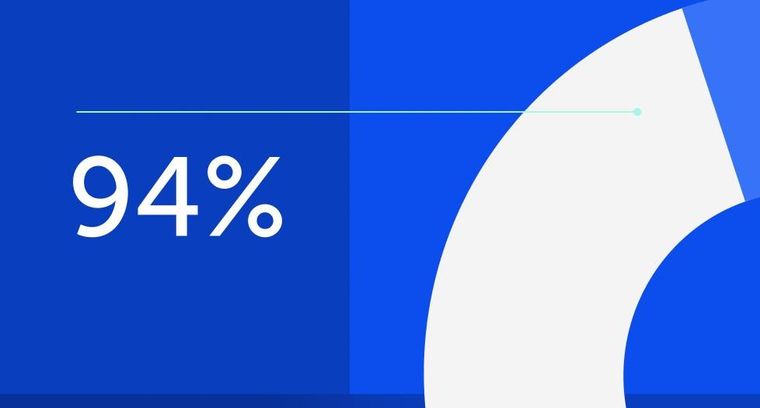
94% of researchers rate our articles as excellent or good
Learn more about the work of our research integrity team to safeguard the quality of each article we publish.
Find out more
REVIEW article
Front. Cell. Infect. Microbiol., 07 March 2022
Sec. Bacteria and Host
Volume 12 - 2022 | https://doi.org/10.3389/fcimb.2022.835181
This article is part of the Research TopicAdvances in Bacterial Subversion of Host Cell MetabolismView all 4 articles
Mitochondria are intracellular organelles that are instrumental in the creation of energy, metabolism, apoptosis, and intrinsic immunity. Mitochondria exhibit an extraordinarily high degree of flexibility, and are constantly undergoing dynamic fusion and fission changes. Chlamydia is an intracellular bacterium that causes serious health problems in both humans and animals. Due to a deficiency of multiple metabolic enzymes, these pathogenic bacteria are highly dependent on their eukaryotic host cells, resulting in a close link between Chlamydia infection and host cell mitochondria. Indeed, Chlamydia increase mitochondrial fusion by inhibiting the activation of dynein-related protein 1 (DRP1), which can regulate host cell metabolism for extra energy. Additionally, Chlamydia can inhibit mitochondrial fission by blocking DRP1 oligomerization, preventing host cell apoptosis. These mechanisms are critical for maintaining a favorable environment for reproduction and growth of Chlamydia. This review discusses the molecular mechanisms of mitochondrial fusion and fission, as well as the mechanisms by which Chlamydia infection alters the mitochondrial dynamics and the prospects of limiting chlamydial development by altering mitochondrial dynamics.
For a long time, mitochondria were merely considered as cell energy factories, generating large quantities of ATP through oxidative phosphorylation (McBride et al., 2006). Subsequent research unraveled a pivotal role of mitochondria in several biological processes, including apoptosis, innate immunity, autophagy, redox signaling, calcium homeostasis, and stem cell reprogramming (Wakabayashi, 1999; Zhou et al., 2008; De Stefani et al., 2012; Shi et al., 2014; Williamson et al., 2020; Levoux et al., 2021). Mitochondria feature a bidirectional membrane structure with a smooth external membrane and an inner membrane that folds inward to produce a ridge-like structure filled with matrix. Live cell imaging techniques have helped uncover the dynamic alterations of mitochondria, including changes in their form, length, and distribution in cells via continual fusion, fission, selective destruction and transit (Lefebvre et al., 2021). These activities are collectively referred to as “mitochondrial dynamics”, which can regulate the quantity, size, and location of mitochondria in the cytoplasm. Mitochondrial dynamics is directly associated with cell homeostasis (Zemirli et al., 2018). For example, stimulation of cells by a variety of internal and external environmental factors (inflammation, pathogen infection, oxidative stress), can cause damage to the internal components of the mitochondria (Stavru et al., 2011; Wu et al., 2011; Zhao et al., 2021). Mitochondrial fusion enables the exchange of material between different mitochondria, while the fission process enables separation of damaged mitochondrial components, thereby achieving a “mitochondrial quality control” effect (Twig et al., 2008). Similarly, highly damaged mitochondria can be destroyed by the ubiquitin protease hydrolysis pathway or encapsulated in autophagic vesicles for lysosomal destruction (Lieber et al., 2019). Mitochondrial fusion occurs when the cellular energy demand exceeds the cellular production of ATP; this phenomenon occurs both physiologically, as with the enhanced skeletal muscle cell function following prolonged exercise (Ruegsegger and Booth, 2018; Arribat et al., 2019), and pathologically, as with the metabolic reprogramming of liver tumor cells (Li et al., 2020).
Chlamydia is a common pathogen that causes disease in humans or animals. The organism lacks the capacity to create growth and development products (Stephens et al., 1998); therefore, utilization of host high-energy metabolites (such as ATP and GTP) are essential for its growth and reproduction. Hence, Chlamydia is sometimes referred to as “energetic parasite” (Chowdhury and Rudel, 2017). It undergoes a unique biphasic developmental cycle from elementary body (EB) to reticulate body (RB); EB is resistant to the harsh external environment and is infectious, while RB has a high metabolic activity (Tan et al., 2012a; Bastidas et al., 2013). The Chlamydia cell cycle may be roughly classified into three stages, as shown by Chlamydia trachomatis. Phase 1 (6–8 hours after infection) involves EB to RB transition, early gene transcription, early effector modification of the inclusion membrane, and capture or incorporation of host lipid vesicles (Tan et al., 2012b; Moore and Ouellette, 2014). Phase 2 (8-16 hours after infection) involves the expression of mid-stage genes, which results in the translation of effector proteins that regulate nutrition uptake and host cell survival; in addition, the bacteria divide by binary fission and there is substantial expansion of inclusion (Elwell and Engel, 2012; Tan et al., 2012c). Phase 3 (24–72 hours after infection): late gene expression, RB to EB transition, host cell lysis and extrusion of inclusion, release of infectious EBs, and initiation of a new infection cycle (Tan et al., 2012b). Studies have revealed that changes in host cell mitochondrial dynamics are associated with ATP acquisition, mitochondrion-fatty acid interplay during persistent infection (Shima et al., 2021), and inhibition of host cell inflammatory responses during the Chlamydial cell cycle; all of these biological behaviors are key nodes in the chlamydial cell cycle (Dzakah et al., 2021), indicating a close relationship between Chlamydia infection and host cell mitochondria. Therefore, in-depth investigation of the link between Chlamydia infection and mitochondrial dynamics is a key imperative for the prevention and treatment of Chlamydia-related disorders.
In this review article, we first discuss the molecular processes of mitochondrial fusion and fission. Then, we discuss the influence of Chlamydia infection on mitochondrial dynamics and the possibility of suppressing Chlamydia development by modifying mitochondrial dynamics. In the future, mitochondrial dynamics might become a novel target for anti-chlamydial infection therapy.
There are two types of mitochondrial fusion: inner mitochondrial membrane fusion and outer mitochondrial membrane fusion. Both types of mitochondrial fusion occur concurrently and in unison. Mitofusin-1 (MFN1), mitofusin-2 (MFN2), and ocular atrophy protein 1 (OPA1) all play critical roles in mitochondrial fusion (Chen et al., 2003; Wai et al., 2015). The fusion process comprises of 3 stages: To begin, the outer mitochondrial membrane fusion proteins MFN1 and MFN2 interact with each other resulting in a decrease in the distance between two mitochondria (Qi et al., 2016). Then, MFN1 and MFN2 form a dimer complex to ensure that dynamin-related GTPase mediates the fusion (Cao et al., 2017). Finally, the OPA1 protein is involved in the fusion of the inner mitochondrial membrane (Meeusen et al., 2006).
MFN1 and MFN2 are critical regulators of mitochondrial outer membrane fusion. MFN1/MFN2 are GTPase-mediated motility proteins that are abundantly found in mammals (Song et al., 2009). Both these proteins include four major domains: the conserved GTPase catalytic binding domain at the N-terminus, the structural domains of the heptad repeat 1 (HR1) and heptad repeat 2 (HR2), and the C-terminal transmembrane structure domain (Figure 1) (Casellas-Diaz et al., 2021). MFNl/2 are capable of forming dimers by connecting hydrophobic heptapeptide repeat structural domains and facilitate outer mitochondrial membrane cohesion through GTPase hydrolysis, in which the fusion mitochondria share adenylate kinases, metabolites, and proteins (Meeusen et al., 2006; Song et al., 2009; Qi et al., 2016; Cao et al., 2017; Casellas-Diaz et al., 2021). A schematic illustration of this procedure is shown in Figure 2.
Figure 1 Schematic representation of the structural elements of the fission and fusion proteins. (A) Between the heptad repeat 1(HR1) and heptad repeat 2 (HR2) domains, MFN1/MFN2 have two transmembrane (TM) domains Between the HR1 and HR2 domains. (B) MFN2 contain proline rich (PR) domains in between HR1 and TM domains. (C) OPA1 have been shown to have five domains: the TM domain, the HR1 domain, the GTPase domain, the middle domain, and the GTPase effector domain (GED) domain, the mitochondrial targeting sequence(MTS) located in the N-terminal. (D) The DRP1 protein contains the following domains: the bundle signalling elements (BSE) domain, the GTPase domain, the middle domain, the variable domain (or B-insert), the GED domain.
Figure 2 Schematic illustration of fusion of the mitochondrial outer membrane. MFN1/2 forms dimers by linking the hydrophobic heptapeptide repeat structural domains and increases outer mitochondrial membrane cohesion through GTPase hydrolysis. After the outer membrane fusion, Mitochondria A and B can share the gap’s contents.
In comparison to outer membrane fusion, mitochondrial inner membrane fusion is a more complex process. OPA1 is a protein involved in the remodeling of the mitochondrial inner membrane that was first discovered in the autosomes of individuals with hereditary ocular atrophy disease (Eiberg et al., 1994). OPA1 is required for the fusion of the inner mitochondrial membrane and the formation of the mitochondrial cristae (Frezza et al., 2006). Not only does OPA1 deficiency result in mitochondrial fragmentation, but it also inhibits the development of mitochondrial inner membrane cristae, which appear as vesicular structures in endosomes under electron microscope (Meeusen et al., 2006). The N-terminal structural domain of OPA1 acts as an anchor, securing it to the inner mitochondrial membrane, whereas the functional structure domain of GTPase is exposed to the membrane gap (Yu et al., 2020). The core machinery proteins are shown in Figure 1. On the inner mitochondrial membrane, OPA1 is expressed in two forms: long-form OPA1 (L-OPA1) and short-form OPA1 (S-OPA1). S-OPA1 is generated by hydrolysis of L-OPA1 by OMA1 zinc metallopeptidase (OMA1) and ATP-dependent metal zinc protease (Anand et al., 2014). Neither L-OPA1 nor S-OPA1 alone can stimulate mitochondrial inner membrane fusion, and this process can only occur in the presence of both of them (Song et al., 2007). Although the relationship between OPA1 subtypes and their specific mechanism for mitochondrial inner membrane fusion has not been fully elucidated, it is clear that the ratio of L-OPA1 to S-OPA1 in mitochondrial inner membrane affects the mitochondrial morphology (Wang et al., 2021), implying that S-OPA1 may indeed regulate mitochondrial fusion via some mechanism. Hu et al. used the OPA1 protein to create an endosomal fusion model (Gao and Hu, 2021). Due to the fact that OPA1 conducts head-to-tail assembly for a brief amount of time, loading results in membrane bending and the formation of unstable tips on the two opposing inner membranes (Yan et al., 2020). When the head-to-tail assembly structure is broken, this membrane bending phenomenon ceases. When opposing unstable tips come together, lipid mixing results in the formation of fusion holes, and with the progressive expansion of the pore, the mitochondrial inner membrane is completely fused (Figure 3).
Figure 3 Schematic illustration of fusion of the mitochondrial inner membrane: OPA1’s N-terminal binds to the inner mitochondrial membrane. The membrane gap exposes the GTPase functional structural domain. In a millisecond, OPA1 completes head-tail assembly, creating membrane bending and unstable tips on the two opposing endosomes with the help of cardiolipin (CL). Preparing for endosomal fusion. When two unstable tips meet, the plasma membranes fuse, generating a small hole that connects mitochondria A and B. The contents of the two mitochondrial matrices can be exchanged.
Mitochondrial fission refers to the division of one mitochondria into two daughter mitochondria. This process is involved in a variety of biological activities, such as organelle inheritance and distribution, mitochondrial distribution, mitochondrial autophagy, and cytochrome C release during apoptosis (Westermann, 2010). Mitochondrial fission in animals is mediated by several proteins such as dynamin-related protein 1(DRP1), Dynamin2(DNM2), mitochondrial elongation factor 2(MIEF2 or MID49), mitochondrial elongation factor 1(MIEF1 or MID51), fission, mitochondrial 1(FIS1), and mitochondrial fission factor(MFF) (Friedman et al., 2011; Schmitt et al., 2018). The DRP1 protein plays a particularly important role in this process (Schmitt et al., 2018). DRP1 is a GTP-dependent kinesin with 5 distinct structural domains (Fröhlich et al., 2013): the bundle signalling elements (BSE) domain, the GTPase domain, the middle domain, the variable domain (or B-insert), the GED domain. DRP1 has bundled signaling components and stalk sections, similar to other kinesins. However, it does not contain the pleckstrin homologous structural domain, the C-terminal proline, arginine-rich structural domain, or the C-terminal of the arginine-rich structural domain (Figure 1).
Mitochondrial fission occurs in a series of steps. The process begins with adherence of the endoplasmic reticulum (ER) tubules to the outer membrane of the mitochondria, shrinking their width from 300–500 nm to 150 nm, providing a spatial foundation for DRP1 oligomerization (Friedman et al., 2011). Subsequently, inverted-formin 2 coupled with the ER tubules interacts with Spire1C protein, initiating the recruitment of DRP1 protein and actin assembly (Korobova et al., 2013; Manor et al., 2015). Then, following recruitment to the outer mitochondrial membrane, DRP1 creates a ring around the mitochondria, which augments the ring contraction force exerted to the mitochondria by ER tubules (Smirnova et al., 2001). Finally, DRP1 hydrolyzes GTP and breaches the outer membrane of the mitochondria (Figure 4). It is important to highlight that the inner mitochondrial membrane fission occurs prior to the formation of DRP1 aggregates in the outer membrane; this process is mediated mostly by ER-derived calcium ions (Chakrabarti et al., 2018). Calcium ions enter the mitochondria through the ER and mitochondrial attachment sites, triggering the cleavage of the inner mitochondrial membrane.
Figure 4 Various phases of mitochondrial fission: (A) ER tubules bind to the outer mitochondrial membrane surface, reducing mitochondrial diameter to 150 nm. (B) DRP1 recruits to the outer mitochondrial membrane, causing its thinning. (C) GTP hydrolysis causes the mitochondrial membrane to contract, preparing the way for the next step of mitochondrial fission. (D) Mitochondria completely split.
Studies have shown that cells can regulate mitochondrial fission by phosphorylating or ubiquitinating DRP1 (Qi et al., 2019; Ma et al., 2020). For example, during mitosis, phosphorylation of the serine residue 585 (S585) of DRP1 results in its oligomerization and attachment to mitochondria, therefore promoting their fission (Taguchi et al., 2007). Additionally, mitogen-activated protein kinase 1 (MAPK1) is required for DRP1 phosphorylation (Song et al., 2021). MAPK1 interacts with DRP1 and phosphorylates serine 616 of DRP1, therefore promoting mitochondrial fission (Kashatus et al., 2015). On the other hand, the biological impact of phosphorylation of the serine residue 637 of DRP1 is opposite to the preceding. Protein kinase A (PKA) has been shown to interact with DRP1 during nutritional deprivation or cell death, retaining it in the cytoplasm and preventing cell lysis and mitochondrial autophagy and degradation (Cribbs and Strack, 2007; Gomes et al., 2011). More modified forms of DRP1 also include SUMOylation, S-nitrosylation, O-GlcNAcylation, and ubiquitination (Jin et al., 2021).
Apoptosis is a key mechanism by which cells resist pathogenic infection (Savill and Fadok, 2000). Infected cells that initiate apoptosis on time can reduce pathogen proliferation. Till date, three apoptotic mechanisms are well characterized: receptor-mediated apoptosis (Trauth et al., 1989), mitochondria-mediated apoptosis (Li et al., 1997), and ER stress-mediated apoptosis (Nakagawa et al., 2000). Studies have shown that mitochondrial dynamics-related proteins such as BCL2 associated X(Bax), DRP1, and telomere protein B1 translocate and accumulate from the cytoplasm to the mitochondria early in the initiation of apoptosis, therefore facilitating mitochondrial fission (Martinou and Youle, 2006; Suen et al., 2008). The outer mitochondrial membrane permeability is altered after mitochondrial fission, resulting in release of cytochrome C into the cytoplasm, which permanently activates the caspase signaling pathway and causes apoptosis (Frank et al., 2001). The impact of host cell apoptosis on chlamydial growth and development is evident; premature host cell death leads to nutrition loss and blocks its reproduction. Chlamydia, on the other hand, seems to have evolved ways to resist apoptosis in the host cell throughout time (Sixt et al., 2017; Weber et al., 2017; Kerr et al., 2017). Chlamydia trachomatis inhibits mitochondrial fragmentation by altering the structure of host cell mitochondria. In a study, after stimulation with H2O2, mitochondria infected with Chlamydia trachomatis did not fragment as much as those in the uninfected group, and the mitochondria grew in length and developed cross-linkages to form a complex network (Chowdhury et al., 2017). In addition, mitoCRWLR, a unique macro script, was utilized to identify changes in mitochondrial movement rate in the infected cells, which is a reliable test for mitochondrial fusion/fission ratio (Okamoto and Shaw, 2005). The findings revealed that stimulation with H2O2 caused severe fragmentation of the mitochondria of uninfected cells and increased the frequency of random mitochondrial movements; however, cells infected with Chlamydia were able to withstand such alterations (Chowdhury et al., 2017). By blocking mitochondrial fission, Chlamydia infection avoids the early commencement of the apoptotic pathway, eventually creating a favorable survival environment for itself. This shows that interfering with Chlamydia’s molecular processes that impact the mitochondrial dynamics is a potential target for the treatment of Chlamydia infection.
The intermediate stage of chlamydial growth is characterized by extensive replication of RBs. The energy necessary for RB replication is sourced from the host cells (Omsland et al., 2012), which results in a significant “ATP deficit” in the host cells. Chlamydia, on the other hand, seems to have developed techniques to influence the metabolic processes of host cells in order to boost the availability of resources for its own use (Wang et al., 2017). Chlamydia can increase the energy output of the host cell by altering mitochondrial dynamics: Kurihara et al. observed that the cellular oxygen consumption of HeLa cells continued to increase following infection with Chlamydia trachomatis, and the oxygen consumption surpassed the predicted limit after 6-7 hours (Kurihara et al., 2019). Furthermore, they discovered that the increase in cellular ATP generation rate corresponded with mitochondrial fusion, which was validated by knocking down genes associated with mitochondrial fusion proteins. This seems to imply that Chlamydia promotes mitochondrial elongation as a means of obtaining more ATP. Previous research has demonstrated that variations in cell energy needs (ADP/ATP ratio) influence the mitochondrial dynamics, and mitochondrial elongation may also increase cell productivity (Molina et al., 2009).However, whether the modification of mitochondrial dynamics is just a phenomenon or a consequence of chlamydial infection is not clear. Further studies are required to determine whether the outcome is due to the influence of the change in the cellular ADP/ATP ratio or to the active action of Chlamydia.
Mitochondria are in a perpetual state of a highly dynamic process of fusion and fission that is intimately connected with the cell cycle, immunity, apoptosis, and mitochondrial quality control. Numerous variables may influence mitochondrial fusion and fission, including reactive oxygen species (ROS), intracellular metabolic activity, and changes in mitochondrial proteins (Bota and Davies, 2002; Molina et al., 2009; Watanabe et al., 2014). Chlamydia infection has been shown to alter the mitochondrial dynamics of host cells in general (Chowdhury et al., 2017). As an exclusively intracellular parasitic bacteria, this change in mitochondrial dynamics is likely critical to the developmental cycle of the bacterium. In-depth characterization of the underlying mechanisms of the altered mitochondrial dynamics associated with chlamydial infection is critical for prevention and therapy.
Initially, ROS were believed to be metabolic byproducts of mitochondrial oxidative phosphorylation (Yang et al., 2016). Increasing evidence indicates that ROS may operate as signaling molecules in a variety of organismal life processes, including inflammation, death, and cell cycle (Moloney and Cotter, 2018). Indeed, ROS-induced changes in mitochondrial dynamics are closely connected; several studies have shown that ROS in mitochondria may affect the state of mitochondrial fusion and fission through their influence on mitochondrial dynamin (Chuang et al., 2020). Chlamydia enters host cells and releases virulence factors through type III secretion system(T3SS) effectors, resulting in potassium ion efflux across the cell membrane, activation of the nicotinamide adenine dinucleotide phosphate (NADPH) oxidase, and formation of ROS (Abdul-Sater et al., 2010). It is worth noting that mitochondria, not NADPH oxidase, are the principal source of intracellular ROS. NADPH oxidase-produced ROS acts more like a signaling spark that lays the scene for subsequent mitochondrial ROS generation. Initially, ROS produced in the cytoplasm by NADPH oxidase activates the NOD-like receptor X1 (NLX1) protein, a member of the NOD family that is normally localized to the outer mitochondrial membrane (Tattoli et al., 2008; Nagai-Singer et al., 2019). This induces the translocation of NLRX1 to the inner membrane and its binding to mitochondrial complex III, thereby inhibiting electron transfer and ultimately increasing mitochondrial ROS with mitochondrial depolarization (Abdul-Sater et al., 2010). Available evidence suggests that mitochondria and ER are significant sources of intracytoplasmic calcium ions (Ishii et al., 2006), and mitochondrial depolarization increases intracytoplasmic calcium ions, activating the cytoplasmic phosphatase calcium-regulated phosphatase; this induces phosphorylation of the serine residue 585 of DRP1, resulting in its activation (Bustillo-Zabalbeitia et al., 2014; Macdonald et al., 2014). Activated DRP1 translocates to the outer mitochondrial membrane and promotes mitochondrial fission (Taguchi et al., 2007). Additionally, ROS generated by chlamydial infection may influence mitochondrial dynamics by deactivating ROS modulator 1 (ROMO1), a protein that modulates the function of the OPA1 protein (Norton et al., 2014). Thus, during the early stages of chlamydial infection (0-6 h), there is increase in mitochondrial ROS (mtROS) (Kurihara et al., 2019). However, chronic increase in mtROS has adverse consequences such as apoptosis and cellular energy starvation, which are clearly unfavorable for chlamydial growth (Zhang et al., 2019). Thus, mtROS synthesis is restricted during the mid-stage of the chlamydial growth cycle (Chowdhury et al., 2017), when Chlamydia has a high need for energy. However, the specific mechanism by which mtROS is inhibited during the intermediate stage of chlamydial infection is not clear. Given the intimate relationship between NLRX1 and mitochondrial ROS generation, we hypothesize that some phenomena occurs during the middle stage of chlamydial infection that restricts the translocation of NLXR protein to the mitochondrial inner membrane, reducing mtROS production. This is an important subject for future research.
Interestingly, when Chlamydia pneumoniae infects host cells, the mitochondrial destiny seems to reverse—mitochondrial dysfunction, as seen by decreased ATP generation and increased ROS production (Kading et al., 2017). Additionally, the researchers discovered that mitochondrial dysfunction promotes Chlamydia pneumoniae proliferation and development. It seems to contradict prior research findings that Chlamydia trachomatis infection boosts mitochondrial fusion and ATP generation in host cells. Thus far, reasonable explanations can be offered: Mitochondrial dysfunction promotes increased ROS release, which can act as upstream signaling molecules activating caspase-1 and hypoxia-inducible factor-1 (HIF-1) (Prusty et al., 2012), thereby promoting the development of Chlamydia trachomatis and possibly also Chlamydia pneumoniae growth. Additionally, Different metabolic characteristics might occur due to the different tissue tropism and differences in their genome, which requires an individual adaption of the two species (Kading et al., 2017). These speculations still need follow-up in vivo experiments to confirm.
Chlamydia and mitochondria have a close interaction, which is maintained by post-translational modification of the DRP1 protein. Recent research has shown that Chlamydia trachomatis induces an increase in intracellular cyclic adenosine monophosphatec(AMP) during the early stages of infection, followed by the phosphorylation of cleavage-inactive serine residue 637 (S637) of DRP1 (Kurihara et al., 2019). This eventually results in mitochondrial fusion. Chlamydial infection induces a significant downregulation of the tumor suppressor P53 via murine double minute2(Mdm2) regulation of the phosphoinositide 3-kinase Akt (Siegl et al., 2014). Because P53 is a critical regulator of DRP1 dephosphorylation, its downregulation directly affects the activation of DRP1, which eventually manifests as mitochondrial fusion. Additionally, in a study by Rudel et al, infection of human umbilical vein endothelial cells with Chlamydia trachomatis was found to induce downregulation of P53 mRNA, resulting in decreased protein expression of P53 in the cytoplasm and decreased phosphorylation of DRP1, inhibiting mitochondrial fission; this change was caused by overexpression of miRNA-30c-5p in the host cells (Chowdhury et al., 2017).
Tail-anchored MFF is an upstream regulator of DRP1 that is responsible for the preferential recruitment of DRP1 protein to the outer mitochondrial membrane (Liu and Chan, 2015), MFF deregulation can result in a decrease in the quantity of DRP1 in the outer mitochondrial membrane, resulting in mitochondrial elongation. On the other hand, overexpression of MFF may result in considerable mitochondrial fragmentation, which is a necessary condition for mitochondrial fission (Loson et al., 2013). Myeloid cell leukemia sequence 1(Mcl-1) acts as an anti-apoptotic protein (Huang et al., 2019), inhibiting the oligomerization of DRP1 protein mediated by MFF protein and preventing mitochondrial fragmentation, hence acting as an anti-apoptotic protein (Liu et al., 2020). Chlamydia increases the degree of Mcl-1 deubiquitination and the cytoplasmic concentration of Mcl-1 protein, consequently limiting mitochondrial fission (Fischer et al., 2017). In host cells infected with Chlamydia, the relevant genes initiate transcription and translation of the ChlaDub1(Cdu1) protein expressed on the inclusion membrane; the subsequent accumulation of the Mcl-1 protein in the cytoplasm results in the formation of Cdu1-Mcl-1 complex which directly protects Mcl-1 from K48-ubiquitinated degradation (Pruneda et al., 2016).
Thus, Chlamydia suppresses DRP1 protein function via a variety of mechanisms, inhibiting mitochondrial fission (Figure 5). As we shall detail below, impeding Chlamydia reproduction in host cells by interfering with mitochondrial dynamics seems to be a novel and extremely promising treatment strategy.
Figure 5 Mechanisms by which chlamydial infection alters mitochondrial dynamics. (A) Chlamydia infects host cells and releases virulence factors through T3SS effectors, resulting in potassium efflux from the cell membrane and activation of the NADPH oxidase. This induces the production of reactive oxygen species (ROS) in the cytoplasm, which acts as a secondary signal to activate the NLRX1 protein in the outer mitochondrial membrane. The activated NLRX1 protein translocates and binds to mitochondrial complex 3, resulting in massive mitochondrial ROS production and phosphorylation of the serine residue 5 8 5 of DRP1, activating DRP1. (B) Chlamydia triggers the PI3K-ATK-HMD2 signaling pathway, which results in the ubiquitination of the P53 protein and subsequently suppresses the activation of the DRP1 protein. (C) Chlamydia increases host cell miRNA-30c-5p production, which binds to the mRNA of the P53 protein and blocks its translation, hence inhibiting DRP1 protein activation. (D) Chlamydia produces the cdu1 protein, which binds to Mcl-1 and shields it from ubiquitinated degradation, hence raising Mcl-1 protein levels and preventing DRP1 oligomerization. (E) C. pneumoniae infects host cells, resulting in mitochondria dysfunction and switch of OXPHOS to glycolysis, which enhances the bacteria growth.
Development of drugs that regulate mitochondrial fusion, fission, and autophagy is a contemporary research hotspot, indicating that mitochondrial dynamics is a potential therapeutic target for many diseases (Jheng et al., 2015; Anis et al., 2020; Axelrod et al., 2021). For example, mitochondrial fission inhibitor-1 (Mdivi-1), a GTP synthase inhibitor, has been studied for its ability to limit mitochondrial function by reducing mitochondrial mass, inducing apoptosis, reducing vascular cell bioenergetics, and inhibiting cell proliferation (Kim et al., 2013). Because of its capacity to prevent cell growth, Mdivi-1 has long been considered a potential cancer therapy. Similarly, after infecting host cells, Chlamydia needs to prevent apoptosis in order to maintain a favorable survival environment, at least in the early and intermediate phases of infection. Tumors are similar in their method of interfering with the natural apoptotic process of cells. Thus, mdivi-1 may be useful in the treatment of chlamydial infection.
On the other hand, chlamydial infection induces activation of the phosphatidylinositol-3-kinase(PI3K)-autologous tumor killing(ATK)-Mdm2 signaling pathway in order to maintain low intracellular P53 levels (Zou et al., 2019), which further limits the activation of DRP1 and results in widespread host cell mitochondrial fusion. As a result, PI3K inhibitors may have therapeutic utility. For example, use of a PI3K inhibitor (LY294002) was investigated in an in vitro study of chlamydial infection (Siegl et al., 2014), in this study, PI3k inhibition dramatically stabilized P53 levels in infected human umbilical vein endothelial cells. Likewise, Al-Zeer et al. discovered that chlamydial infection can result in phosphorylation of the serine residue 637 of pyruvate dehydrogenase kinase 1(PDK1) in host cells, hence stabilizing the production of WYC protein. Consistent MYC expression can induce hexokinase II(HKII) upregulation (Al-Zeer et al., 2017). This is critical for cell survival, because the mitochondria-HKII association favorably influences mitochondrial energetics and cell survival by preventing pro-apoptotic Bak and Bax oligomerization and binding at the level of the mitochondrion (Robey and Hay, 2006). It is also crucial for inhibiting cytochrome c release and apoptosis, which is controlled by the PI3K cascade (Kennedy et al., 1999). Thus, genetic and pharmacological means to inhibit MYC and 3-Phosphoinositide Dependent Protein Kinase 1(PDPK1) may also block chlamydial replication. TH2-mpeoDM1(HMD2) is a critical component in the ubiquitination and degradation of the P53 protein. HDM2 interacts with P53 in the nucleus, facilitates its export to the cytoplasm, and catalyzes the production of ubiquitin chains on P53 (Bhatt et al., 2012). In other words, suppressing the interaction between HMD2 and P53 may result in stabilization of P53 protein level in cells. Nutlin-3, a cis-imidazoline, selectively binds to HDM2 and competes with P53 protein, limiting the binding of HDM2 to the N-terminal end of P53 (van Leeuwen et al., 2011), but still maintaining intracellular P53 levels, facilitating mitochondrial fission.
Additionally, phosphorylation is a significant post-translational alteration of the DRP1 protein. Numerous phosphorylation modification sites on the DRP1 protein have been identified so far, including Ser-579, Ser-40, Ser-585, Ser-44, Ser-592, Ser-656, Ser-616, Ser-637, and Ser-693 (Qi et al., 2019). Among these, phosphorylation of Ser-616 on DRP1 may activate the protein and cause mitochondrial fission. Rho-associated protein kinase (ROCK), protein kinase C delta (PKC), cyclin-dependent kinase 1 (CDK1), extracellular signal-regulated kinase 1/2 (ERK1/2), and calmodulin-dependent protein kinase II (CaMKII) were discovered to catalyze this site (Bo et al., 2018; Adaniya et al., 2019). Unlike the Ser-616 location, phosphorylation of the serine residue 637 of DRP1 results in a decrease in GTPase hydrolysis activity (Chang and Blackstone, 2007; Cribbs and Strack, 2007). That is, phosphorylation of this region results in a decrease in DRP1 activity. In theory, regulating the expression of the above enzymes can indirectly affect the phosphorylation of the serine Ser-616 and Ser637 of DRP1, activate DRP1 protein, promote mitochondrial fission events, and ultimately disrupt the environment in which Chlamydia survives.
Finally, Chlamydia’s unique “Cdu1-Mcl-1” interaction mechanism, which results in Mcl-1 deubiquitination, is another potential therapeutic target. Cdu1-Mcl-1 binding was shown to decrease Mcl-1 ubiquitination and hinder DRP1 protein oligomerization, preventing mitochondrial fission and enhancing mitochondrial fusion (Fischer et al., 2017). Thereby, it seems to be an extremely promising strategy to create ligands that exclusively bind to Cdu1 as a competitive inhibitor of Mcl-1 in order to increase the ubiquitination level of Mcl-1 and thus impact mitochondrial dynamics. Similar investigations are currently ongoing: Caroline Kisker’s team discovered that the particular inhibitors cyanopyrimidine 3 and cyanopyrimidine 5 may form covalent connections with Cdu1 and disrupt the protein’s deubiquitination function (Ramirez et al., 2018). This indicates that the chemical may be capable of combating chlamydial infection.
However, it is critical to consider drug targeting when using mitochondrial dynamics as a therapeutic target for chlamydial infection. As a rule of thumb, it is preferable to have more frequent mitochondrial fission events in certain infected cells rather than all cells of the organism; otherwise, it may result in adverse effects such as decreased collective energy metabolism and increased apoptosis. The question of how to design pro-mitochondrial fission drugs that act only in chlamydial infected cells deserves to be researched in greater depth.
Mitochondria are ephemeral organelles with a high degree of dynamic activity. The homeostasis between fusion and fission is critical for cell activities. This has an effect on not just ATP synthesis, but also on the classic apoptotic pathway. Chlamydial infection affects the mitochondrial dynamics, and altered mitochondrial dynamics can have an effect on Chlamydia survival. Chlamydia inhibits mitochondrial fission, preventing the host cell from commencing the apoptotic pathway and enabling the cell to continue its cycle. Additionally, Chlamydia controls the efficiency of energy generation by the host cells by stimulating mitochondrial fusion to satisfy self-replication and reproduction requirements. Chlamydia suppresses mitochondrial fission and promotes mitochondrial fusion in the host cell via lowering ROS generation, inhibiting P53 protein transcription, increasing P53 protein ubiquitination levels, and inhibiting DRP1 protein activity. These mechanisms are potential therapeutic targets. This review explored the effect of chlamydial infection on mitochondrial dynamics and the underlying mechanisms. We also reviewed the available evidence pertaining to other drugs that impede mitochondrial fusion on this basis, including mdivi-1, LY294002, Nutlin-3, cyanopyrimidine 3, and cyanopyrimidine 5. Although medication targeting remains an open question, with breakthroughs in research on drug release and delivery systems, pharmacological targeting of the mitochondrial dynamics of infected cells seems to be a viable therapeutic strategy for chlamydial infection.
Manuscript conceptualization, ZL and YY. Writing original manuscript draft, YY. Literature search and articles acquisition, YW and LZ. Figures drawing, WL. All authors contributed to the article and approved the submitted version.
This work was supported by the National Natural Science Foundation of China (No. 32070189 and 81772210), the Key Program of Hunan Provincial Department of Education (No. 20A421), Hunan Provincial Natural Science Foundation of China (No.2021JJ30594), Clinical Research Project of University of South of China (No. USCKF201902K01).
The authors declare that the research was conducted in the absence of any commercial or financial relationships that could be construed as a potential conflict of interest.
All claims expressed in this article are solely those of the authors and do not necessarily represent those of their affiliated organizations, or those of the publisher, the editors and the reviewers. Any product that may be evaluated in this article, or claim that may be made by its manufacturer, is not guaranteed or endorsed by the publisher.
Research in our laboratories was supported by the National Natural Science Foundation of China (No. 32070189 and 81772210), the Key Program of Hunan Provincial Department of Education (No. 20A421), Hunan Provincial Natural Science Foundation of China (No. 2021JJ30594), and Clinical Research Project of University of South of China (No. USCKF201902K01).
Abdul-Sater, A. A., Saïd-Sadier, N., Lam, V. M., Singh, B., Pettengill, M. A., Soares, F., et al. (2010). Enhancement of Reactive Oxygen Species Production and Chlamydial Infection by the Mitochondrial Nod-Like Family Member NLRX1. J. Biol. Chem. 285 (53), 41637–41645. doi: 10.1074/jbc.M110.137885
Adaniya, S. M., O-Uchi, J., Cypress, M. W., Kusakari, Y., Jhun, B. S. (2019). Posttranslational Modifications of Mitochondrial Fission and Fusion Proteins in Cardiac Physiology and Pathophysiology. Am. J. Physiol. Cell Physiol. 316 (5), C583–C604. doi: 10.1152/ajpcell.00523.2018
Al-Zeer, M. A., Xavier, A., Abu Lubad, M., Sigulla, J., Kessler, M., Hurwitz, R., et al. (2017). Chlamydia Trachomatis Prevents Apoptosis via Activation of PDPK1-MYC and Enhanced Mitochondrial Binding of Hexokinase II. EBioMedicine 23, 100–110. doi: 10.1016/j.ebiom.2017.08.005
Anand, R., Wai, T., Baker, M. J., Kladt, N., Schauss, A. C., Rugarli, E., et al. (2014). The I-AAA Protease YME1L and OMA1 Cleave OPA1 to Balance Mitochondrial Fusion and Fission. J. Cell Biol. 204 (6), 919–929. doi: 10.1083/jcb.201308006
Anis, E., Zafeer, M. F., Firdaus, F., Islam, S. N., Anees Khan, A., Ali, A., et al. (2020). Ferulic Acid Reinstates Mitochondrial Dynamics Through PGC1α Expression Modulation in 6-Hydroxydopamine Lesioned Rats. Phytotherapy Res. PTR. 34 (1), 214–226. doi: 10.1002/ptr.6523
Arribat, Y., Broskey, N. T., Greggio, C., Boutant, M., Conde Alonso, S., Kulkarni, S. S., et al. (2019). Distinct Patterns of Skeletal Muscle Mitochondria Fusion, Fission and Mitophagy Upon Duration of Exercise Training. Acta Physiol. (Oxf) 225 (2), e13179. doi: 10.1111/apha.13179
Axelrod, C. L., Fealy, C. E., Erickson, M. L., Davuluri, G., Fujioka, H., Dantas, W. S., et al. (2021). Lipids Activate Skeletal Muscle Mitochondrial Fission and Quality Control Networks to Induce Insulin Resistance in Humans. Metabolism: Clin. Experimental 121, 154803. doi: 10.1016/j.metabol.2021.154803
Bastidas, R. J., Elwell, C. A., Engel, J. N., Valdivia, R. H. (2013). Chlamydial Intracellular Survival Strategies. Cold Spring Harbor Perspect. Med. 3 (5), a10256. doi: 10.1101/cshperspect.a010256
Bhatt, P., D'Avout, C., Kane, N. S., Borowiec, J. A., Saxena, A. (2012). Specific Domains of Nucleolin Interact With Hdm2 and Antagonize Hdm2-Mediated P53 Ubiquitination. FEBS J. 279 (3), 370–383. doi: 10.1111/j.1742-4658.2011.08430.x
Bota, D. A., Davies, K. J. (2002). Lon Protease Preferentially Degrades Oxidized Mitochondrial Aconitase by an ATP-Stimulated Mechanism. Nat. Cell Biol. 4 (9), 674–680. doi: 10.1038/ncb836
Bo, T., Yamamori, T., Suzuki, M., Sakai, Y., Yamamoto, K., Inanami, O. (2018). Calmodulin-Dependent Protein Kinase II (Camkii) Mediates Radiation-Induced Mitochondrial Fission by Regulating the Phosphorylation of Dynamin-Related Protein 1 (Drp1) at Serine 616. Biochem. Biophys. Res. Commun. 495 (2), 1601–1607. doi: 10.1016/j.bbrc.2017.12.012
Bustillo-Zabalbeitia, I., Montessuit, S., Raemy, E., Basañez, G., Terrones, O., Martinou, J. C. (2014). Specific Interaction With Cardiolipin Triggers Functional Activation of Dynamin-Related Protein 1. PLoS One 9 (7), e102738. doi: 10.1371/journal.pone.0102738
Cao, Y. L., Meng, S., Chen, Y., Feng, J. X., Gu, D. D., Yu, B., et al. (2017). MFN1 Structures Reveal Nucleotide-Triggered Dimerization Critical for Mitochondrial Fusion. Nature 542 (7641), 372–376. doi: 10.1038/nature21077
Casellas-Diaz, S., Larramona-Arcas, R., Rique-Pujol, G., Tena-Morraja, P., Muller-Sanchez, C., Segarra-Mondejar, M., et al. (2021). Mfn2 Localization in the ER is Necessary for its Bioenergetic Function and Neuritic Development. EMBO Rep. 22 (9), e51954. doi: 10.15252/embr.202051954
Chakrabarti, R., Ji, W. K., Stan, R. V., de Juan Sanz, J., Ryan, T. A., Higgs, H. N. (2018). INF2-Mediated Actin Polymerization at the ER Stimulates Mitochondrial Calcium Uptake, Inner Membrane Constriction, and Division. J. Cell Biol. 217 (1), 251–268. doi: 10.1083/jcb.201709111
Chang, C. R., Blackstone, C. (2007). Cyclic AMP-Dependent Protein Kinase Phosphorylation of Drp1 Regulates its Gtpase Activity and Mitochondrial Morphology. J. Biol. Chem. 282 (30), 21583–21587. doi: 10.1074/jbc.C700083200
Chen, H., Detmer, S. A., Ewald, A. J., Griffin, E. E., Fraser, S. E., Chan, D. C. (2003). Mitofusins Mfn1 and Mfn2 Coordinately Regulate Mitochondrial Fusion and Are Essential for Embryonic Development. J. Cell Biol. 160 (2), 189–200. doi: 10.1083/jcb.200211046
Chowdhury, S. R., Reimer, A., Sharan, M., Kozjak-Pavlovic, V., Eulalio, A., Prusty, B. K., et al. (2017). Chlamydia Preserves the Mitochondrial Network Necessary for Replication via MicroRNA-Dependent Inhibition of Fission. J. Cell Biol. 216 (4), 1071–1089. doi: 10.1083/jcb.201608063
Chowdhury, S. R., Rudel, T. (2017). Chlamydia and Mitochondria - an Unfragmented Relationship. Microb. Cell. 4 (7), 233–235. doi: 10.15698/mic2017.07.582
Chuang, K. C., Chang, C. R., Chang, S. H., Huang, S. W., Chuang, S. M., Li, Z. Y., et al. (2020). Imiquimod-Induced ROS Production Disrupts the Balance of Mitochondrial Dynamics and Increases Mitophagy in Skin Cancer Cells. J. Dermatol. Sci 98 (3), 152–162. doi: 10.1016/j.jdermsci.2020.03.009
Cribbs, J. T., Strack, S. (2007). Reversible Phosphorylation of Drp1 by Cyclic AMP-Dependent Protein Kinase and Calcineurin Regulates Mitochondrial Fission and Cell Death. EMBO Rep. 8 (10), 939–944. doi: 10.1038/sj.embor.7401062
De Stefani, D., Bononi, A., Romagnoli, A., Messina, A., De Pinto, V., Pinton, P., et al. (2012). VDAC1 Selectively Transfers Apoptotic Ca2+ Signals to Mitochondria. Cell Death Differ. 19 (2), 267–273. doi: 10.1038/cdd.2011.92
Dzakah, E. E., Huang, L., Xue, Y., Wei, S., Wang, X., Chen, H., et al. (2021). Host Cell Response and Distinct Gene Expression Profiles at Different Stages of Chlamydia Trachomatis Infection Reveals Stage-Specific Biomarkers of Infection. BMC Microbiol. 21 (1), 3. doi: 10.1186/s12866-020-02061-6
Eiberg, H., Kjer, B., Kjer, P., Rosenberg, T. (1994). Dominant Optic Atrophy (OPA1) Mapped to Chromosome 3q Region. I. Linkage Analysis. Hum. Mol. Genet. 3 (6), 977–980. doi: 10.1093/hmg/3.6.977
Elwell, C. A., Engel, J. N. (2012). Lipid Acquisition by Intracellular Chlamydiae. Cell Microbiol. 14 (7), 1010–1018. doi: 10.1111/j.1462-5822.2012.01794.x
Fischer, A., Harrison, K. S., Ramirez, Y., Auer, D., Chowdhury, S. R., Prusty, B. K., et al. (2017). Chlamydia Trachomatis-Containing Vacuole Serves as Deubiquitination Platform to Stabilize Mcl-1 and to Interfere With Host Defense. eLife 28 (6), e21465. doi: 10.7554/eLife.21465.039
Frank, S., Gaume, B., Bergmann-Leitner, E. S., Leitner, W. W., Robert, E. G., Catez, F., et al. (2001). The Role of Dynamin-Related Protein 1, a Mediator of Mitochondrial Fission, in Apoptosis. Dev. Cell. 1 (4), 515–525. doi: 10.1016/S1534-5807(01)00055-7
Frezza, C., Cipolat, S., Martins De Brito, O., Micaroni, M., Beznoussenko, G. V., Rudka, T., et al. (2006). OPA1 Controls Apoptotic Cristae Remodeling Independently From Mitochondrial Fusion. Cell 126 (1), 177–189. doi: 10.1016/j.cell.2006.06.025
Friedman, J. R., Lackner, L. L., West, M., DiBenedetto, J. R., Nunnari, J., Voeltz, G. K. (2011). ER Tubules Mark Sites of Mitochondrial Division. Science 334 (6054), 358–362. doi: 10.1126/science.1207385
Fröhlich, C., Grabiger, S., Schwefel, D., Faelber, K., Rosenbaum, E., Mears, J., et al. (2013). Structural Insights Into Oligomerization and Mitochondrial Remodelling of Dynamin 1-Like Protein. EMBO J. 32 (9), 1280–1292. doi: 10.1038/emboj.2013.74
Gao, S., Hu, J. (2021). Mitochondrial Fusion: The Machineries in and Out. Trends Cell Biol. 31 (1), 62–74. doi: 10.1016/j.tcb.2020.09.008
Gomes, L. C., Di Benedetto, G., Scorrano, L. (2011). During Autophagy Mitochondria Elongate, Are Spared From Degradation and Sustain Cell Viability. Nat. Cell Biol. 13 (5), 589–598. doi: 10.1038/ncb2220
Huang, K., O'Neill, K. L., Li, J., Zhou, W., Han, N., Pang, X., et al. (2019). BH3-Only Proteins Target BCL-Xl/MCL-1, Not BAX/BAK, to Initiate Apoptosis. Cell Res. 29 (11), 942–952. doi: 10.1038/s41422-019-0231-y
Ishii, K., Hirose, K., Iino, M. (2006). Ca2+ Shuttling Between Endoplasmic Reticulum and Mitochondria Underlying Ca2+ Oscillations. EMBO Rep. 7 (4), 390–396. doi: 10.1038/sj.embor.7400620
Jheng, H. F., Huang, S. H., Kuo, H. M., Hughes, M. W., Tsai, Y. S. (2015). Molecular Insight and Pharmacological Approaches Targeting Mitochondrial Dynamics in Skeletal Muscle During Obesity. Ann. N Y Acad. Sci. 1350, 82–94. doi: 10.1111/nyas.12863
Jin, J. Y., Wei, X. X., Zhi, X. L., Wang, X. H., Meng, D. (2021). Drp1-Dependent Mitochondrial Fission in Cardiovascular Disease. Acta Pharmacol. Sin. 42 (5), 655–664. doi: 10.1038/s41401-020-00518-y
Kading, N., Kaufhold, I., Muller, C., Szaszak, M., Shima, K., Weinmaier, T., et al. (2017). Growth of Chlamydia Pneumoniae is Enhanced in Cells With Impaired Mitochondrial Function. Front. Cell Infect. Microbiol. 7, 499. doi: 10.3389/fcimb.2017.00499
Kashatus, J. A., Nascimento, A., Myers, L. J., Sher, A., Byrne, F. L., Hoehn, K. L., et al. (2015). Erk2 Phosphorylation of Drp1 Promotes Mitochondrial Fission and MAPK-Driven Tumor Growth. Mol. Cell. 57 (3), 537–551. doi: 10.1016/j.molcel.2015.01.002
Kennedy, S. G., Kandel, E. S., Cross, T. K., Hay, N. (1999). Akt/Protein Kinase B Inhibits Cell Death by Preventing the Release of Cytochrome C From Mitochondria. Mol. Cell. Biol. 19 (8), 5800–5810. doi: 10.1128/MCB.19.8.5800
Kerr, M. C., Gomez, G. A., Ferguson, C., Tanzer, M. C., Murphy, J. M., Yap, A. S., et al. (2017). Laser-Mediated Rupture of Chlamydial Inclusions Triggers Pathogen Egress and Host Cell Necrosis. Nat. Commun. 8, 14729. doi: 10.1038/ncomms14729
Kim, B., Kim, J. S., Yoon, Y., Santiago, M. C., Brown, M. D., Park, J. Y. (2013). Inhibition of Drp1-Dependent Mitochondrial Division Impairs Myogenic Differentiation. Am. J. Physiol. Regul. Integr. Comp. Physiol. 305 (8), R927–R938. doi: 10.1152/ajpregu.00502.2012
Korobova, F., Ramabhadran, V., Higgs, H. N. (2013). An Actin-Dependent Step in Mitochondrial Fission Mediated by the ER-Associated Formin INF2. Science 339 (6118), 464–467. doi: 10.1126/science.1228360
Kurihara, Y., Itoh, R., Shimizu, A., Walenna, N. F., Chou, B., Ishii, K., et al. (2019). Chlamydia Trachomatis Targets Mitochondrial Dynamics to Promote Intracellular Survival and Proliferation. Cell Microbiol. 21 (1), e12962. doi: 10.1111/cmi.12962
Lefebvre, A. E. Y. T., Ma, D., Kessenbrock, K., Lawson, D. A., Digman, M. A. (2021). Automated Segmentation and Tracking of Mitochondria in Live-Cell Time-Lapse Images. Nat. Methods 18 (9), 1091–1102. doi: 10.1038/s41592-021-01234-z
Levoux, J., Prola, A., Lafuste, P., Gervais, M., Chevallier, N., Koumaiha, Z., et al. (2021). Platelets Facilitate the Wound-Healing Capability of Mesenchymal Stem Cells by Mitochondrial Transfer and Metabolic Reprogramming. Cell Metab. 33 (3), 688–690. doi: 10.1016/j.cmet.2021.02.003
Lieber, T., Jeedigunta, S. P., Palozzi, J. M., Lehmann, R., Hurd, T. R. (2019). Mitochondrial Fragmentation Drives Selective Removal of Deleterious Mtdna in the Germline. Nature 570 (7761), 380–384. doi: 10.1038/s41586-019-1213-4
Li, P., Nijhawan, D., Budihardjo, I., Srinivasula, S. M., Ahmad, M., Alnemri, E. S., et al. (1997). Cytochrome C and Datp-Dependent Formation of Apaf-1/Caspase-9 Complex Initiates an Apoptotic Protease Cascade. Cell 91 (4), 479–489. doi: 10.1016/S0092-8674(00)80434-1
Liu, R., Chan, D. C. (2015). The Mitochondrial Fission Receptor Mff Selectively Recruits Oligomerized Drp1. Mol. Biol. Cell. 26 (24), 4466–4477. doi: 10.1091/mbc.E15-08-0591
Liu, Y., Ma, Y., Tu, Z., Zhang, C., Du, M., Wang, Y., et al. (2020). Mcl-1 Inhibits Mff-Mediated Mitochondrial Fragmentation and Apoptosis. Biochem. Biophys. Res. Commun. 523 (3), 620–626. doi: 10.1016/j.bbrc.2019.12.104
Li, M., Wang, L., Wang, Y., Zhang, S., Zhou, G., Lieshout, R., et al. (2020). Mitochondrial Fusion via OPA1 and MFN1 Supports Liver Tumor Cell Metabolism and Growth. Cells 9 (1), 121. doi: 10.3390/cells9010121
Loson, O. C., Song, Z., Chen, H., Chan, D. C. (2013). Fis1, Mff, Mid49, and Mid51 Mediate Drp1 Recruitment in Mitochondrial Fission. Mol. Biol. Cell. 24 (5), 659–667. doi: 10.1091/mbc.e12-10-0721
Macdonald, P. J., Stepanyants, N., Mehrotra, N., Mears, J. A., Qi, X., Sesaki, H., et al. (2014). A Dimeric Equilibrium Intermediate Nucleates Drp1 Reassembly on Mitochondrial Membranes for Fission. Mol. Biol. Cell. 25 (12), 1905–1915. doi: 10.1091/mbc.e14-02-0728
Ma, R., Ma, L., Weng, W., Wang, Y., Liu, H., Guo, R., et al. (2020). DUSP6 Sumoylation Protects Cells From Oxidative Damage via Direct Regulation of Drp1 Dephosphorylation. Sci. Adv. 6 (13), z361. doi: 10.1126/sciadv.aaz0361
Manor, U., Bartholomew, S., Golani, G., Christenson, E., Kozlov, M., Higgs, H., et al. (2015). A Mitochondria-Anchored Isoform of the Actin-Nucleating Spire Protein Regulates Mitochondrial Division. Elife 4, e08828. doi: 10.7554/eLife.08828.023
Martinou, J. C., Youle, R. J. (2006). Which Came First, the Cytochrome C Release or the Mitochondrial Fission? Cell Death Differ. 13 (8), 1291–1295. doi: 10.1038/sj.cdd.4401985
McBride, H. M., Neuspiel, M., Wasiak, S. (2006). Mitochondria: More Than Just a Powerhouse. Curr. Biol. 16 (14), R551–R560. doi: 10.1016/j.cub.2006.06.054
Meeusen, S., DeVay, R., Block, J., Cassidy-Stone, A., Wayson, S., McCaffery, J. M., et al. (2006). Mitochondrial Inner-Membrane Fusion and Crista Maintenance Requires the Dynamin-Related Gtpase Mgm1. Cell 127 (2), 383–395. doi: 10.1016/j.cell.2006.09.021
Molina, A. J., Wikstrom, J. D., Stiles, L., Las, G., Mohamed, H., Elorza, A., et al. (2009). Mitochondrial Networking Protects Beta-Cells From Nutrient-Induced Apoptosis. Diabetes 58 (10), 2303–2315. doi: 10.2337/db07-1781
Moloney, J. N., Cotter, T. G. (2018). ROS Signalling in the Biology of Cancer. Semin. Cell Dev. Biol. 80, 50–64. doi: 10.1016/j.semcdb.2017.05.023
Moore, E. R., Ouellette, S. P. (2014). Reconceptualizing the Chlamydial Inclusion as a Pathogen-Specified Parasitic Organelle: An Expanded Role for Inc Proteins. Front. Cell. Infect Microbiol. 4, 157. doi: 10.3389/fcimb.2014.00157
Nagai-Singer, M. A., Morrison, H. A., Allen, I. C. (2019). NLRX1 is a Multifaceted and Enigmatic Regulator of Immune System Function. Front. Immunol. 10, 2419. doi: 10.3389/fimmu.2019.02419
Nakagawa, T., Zhu, H., Morishima, N., Li, E., Xu, J., Yankner, B. A., et al. (2000). Caspase-12 Mediates Endoplasmic-Reticulum-Specific Apoptosis and Cytotoxicity by Amyloid-Beta. Nature 403 (6765), 98–103. doi: 10.1038/47513
Norton, M., Ng, A. C., Baird, S., Dumoulin, A., Shutt, T., Mah, N., et al. (2014). ROMO1 is an Essential Redox-Dependent Regulator of Mitochondrial Dynamics. Sci. Signaling 7 (310), a10. doi: 10.1126/scisignal.2004374
Okamoto, K., Shaw, J. M. (2005). Mitochondrial Morphology and Dynamics in Yeast and Multicellular Eukaryotes. Annu. Rev. Genet. 39, 503–536. doi: 10.1146/annurev.genet.38.072902.093019
Omsland, A., Sager, J., Nair, V., Sturdevant, D. E., Hackstadt, T. (2012). Developmental Stage-Specific Metabolic and Transcriptional Activity of Chlamydia Trachomatis in an Axenic Medium. Proc. Natl. Acad. Sci. U. S. A. 109 (48), 19781–19785. doi: 10.1073/pnas.1212831109
Pruneda, J. N., Durkin, C. H., Geurink, P. P., Ovaa, H., Santhanam, B., Holden, D. W., et al. (2016). The Molecular Basis for Ubiquitin and Ubiquitin-Like Specificities in Bacterial Effector Proteases. Mol. Cell. 63 (2), 261–276. doi: 10.1016/j.molcel.2016.06.015
Prusty, B. K., Böhme, L., Bergmann, B., Siegl, C., Krause, E., Mehlitz, A., et al. (2012). Imbalanced Oxidative Stress Causes Chlamydial Persistence During non-Productive Human Herpes Virus Co-Infection. PLoS One 7 (10), e47427. doi: 10.1371/journal.pone.0047427
Qi, Z., Huang, Z., Xie, F., Chen, L. (2019). Dynamin-Related Protein 1: A Critical Protein in the Pathogenesis of Neural System Dysfunctions and Neurodegenerative Diseases. J. Cell. Physiol. 234 (7), 10032–10046. doi: 10.1002/jcp.27866
Qi, Y., Yan, L., Yu, C., Guo, X., Zhou, X., Hu, X., et al. (2016). Structures of Human Mitofusin 1 Provide Insight Into Mitochondrial Tethering. J. Cell Biol. 215 (5), 621–629. doi: 10.1083/jcb.201609019
Ramirez, Y. A., Adler, T. B., Altmann, E., Klemm, T., Tiesmeyer, C., Sauer, F., et al. (2018). Structural Basis of Substrate Recognition and Covalent Inhibition of Cdu1 From Chlamydia Trachomatis. ChemMedChem 13 (19), 2014–2023. doi: 10.1002/cmdc.201800364
Robey, R. B., Hay, N. (2006). Mitochondrial Hexokinases, Novel Mediators of the Antiapoptotic Effects of Growth Factors and Akt. Oncogene 25 (34), 4683–4696. doi: 10.1038/sj.onc.1209595
Ruegsegger, G. N., Booth, F. W. (2018). Health Benefits of Exercise. Cold Spring Harb. Perspect. Med. 8 (7), a029694,. doi: 10.1101/cshperspect.a029694
Savill, J., Fadok, V. (2000). Corpse Clearance Defines the Meaning of Cell Death. Nature 407 (6805), 784–788. doi: 10.1038/35037722
Schmitt, K., Grimm, A., Dallmann, R., Oettinghaus, B., Restelli, L. M., Witzig, M., et al. (2018). Circadian Control of DRP1 Activity Regulates Mitochondrial Dynamics and Bioenergetics. Cell Metab. 27 (3), 657–666. doi: 10.1016/j.cmet.2018.01.011
Shima, K., Kaufhold, I., Eder, T., Käding, N., Schmidt, N., Ogunsulire, I. M., et al. (2021). Regulation of the Mitochondrion-Fatty Acid Axis for the Metabolic Reprogramming of Chlamydia Trachomatis During Treatment With β-Lactam Antimicrobials. mBio 12 (2), e00023–21. doi: 10.1128/mBio.00023-21
Shi, C. S., Qi, H. Y., Boularan, C., Huang, N. N., Abu-Asab, M., Shelhamer, J. H., et al. (2014). SARS-Coronavirus Open Reading Frame-9b Suppresses Innate Immunity by Targeting Mitochondria and the MAVS/TRAF3/TRAF6 Signalosome. J. Immunol. (Baltimore Md. 1950) 193 (6), 3080–3089. doi: 10.4049/jimmunol.1303196
Siegl, C., Prusty, B. K., Karunakaran, K., Wischhusen, J., Rudel, T. (2014). Tumor Suppressor P53 Alters Host Cell Metabolism to Limit Chlamydia Trachomatis Infection. Cell Rep. 9 (3), 918–929. doi: 10.1016/j.celrep.2014.10.004
Sixt, B. S., Bastidas, R. J., Finethy, R., Baxter, R. M., Carpenter, V. K., Kroemer, G., et al. (2017). The Chlamydia Trachomatis Inclusion Membrane Protein Cpos Counteracts STING-Mediated Cellular Surveillance and Suicide Programs. Cell Host Microbe 21 (1), 113–121. doi: 10.1016/j.chom.2016.12.002
Smirnova, E., Griparic, L., Shurland, D. L., van der Bliek, A. M. (2001). Dynamin-Related Protein Drp1 is Required for Mitochondrial Division in Mammalian Cells. Mol. Biol. Cell. 12 (8), 2245–2256. doi: 10.1091/mbc.12.8.2245
Song, Z., Chen, H., Fiket, M., Alexander, C., Chan, D. C. (2007). OPA1 Processing Controls Mitochondrial Fusion and is Regulated by mRNA Splicing, Membrane Potential, and Yme1L. J. Cell Biol. 178 (5), 749–755. doi: 10.1083/jcb.200704110
Song, C. X., Chen, J. Y., Li, N., Guo, Y. (2021). CTRP9 Enhances Efferocytosis in Macrophages via MAPK/Drp1-Mediated Mitochondrial Fission and Adipor1-Induced Immunometabolism. J. Inflammation Res. 14, 1007–1017. doi: 10.2147/JIR.S302944
Song, Z., Ghochani, M., McCaffery, J. M., Frey, T. G., Chan, D. C. (2009). Mitofusins and OPA1 Mediate Sequential Steps in Mitochondrial Membrane Fusion. Mol. Biol. Cell. 20 (15), 3525–3532. doi: 10.1091/mbc.e09-03-0252
Stavru, F., Bouillaud, F., Sartori, A., Ricquier, D., Cossart, P. (2011). Listeria Monocytogenes Transiently Alters Mitochondrial Dynamics During Infection. Proc. Natl. Acad. Sci. U. S. A. 108 (9), 3612–3617. doi: 10.1073/pnas.1100126108
Stephens, R. S., Kalman, S., Lammel, C., Fan, J., Marathe, R., Aravind, L., et al. (1998). Genome Sequence of an Obligate Intracellular Pathogen of Humans: Chlamydia Trachomatis. Science (New York N.Y.) 282 (5389), 754–759. doi: 10.1126/science.282.5389.754
Suen, D. F., Norris, K. L., Youle, R. J. (2008). Mitochondrial Dynamics and Apoptosis. Genes Dev. 22 (12), 1577–1590. doi: 10.1101/gad.1658508
Taguchi, N., Ishihara, N., Jofuku, A., Oka, T., Mihara, K. (2007). Mitotic Phosphorylation of Dynamin-Related Gtpase Drp1 Participates in Mitochondrial Fission. J. Biol. Chem. 282 (15), 11521–11529. doi: 10.1074/jbc.M607279200
Tan, M., Bavoil, P., Tan, M., Bavoil, P. (2012a). Intracellular Pathogens1: Chlamydiales. ASM Press 1, 74–96. doi: 10.1128/9781555817329
Tan, M., Bavoil, P., Tan, M., Bavoil, P. (2012b). Intracellular Pathogens1: Chlamydiales. ASM Press 1, 149–169. doi: 10.1128/9781555817329
Tan, M., Bavoil, P., Tan, M., Bavoil, P. (2012c). Intracellular Pathogens1: Chlamydiales. ASM Press 1, 126–148. doi: 10.1128/9781555817329
Tattoli, I., Carneiro, L. A., Jéhanno, M., Magalhaes, J. G., Shu, Y., Philpott, D. J., et al. (2008). NLRX1 is a Mitochondrial NOD-Like Receptor That Amplifies NF-Kappab and JNK Pathways by Inducing Reactive Oxygen Species Production. EMBO Rep. 9 (3), 293–300. doi: 10.1038/sj.embor.7401161
Trauth, B. C., Klas, C., Peters, A. M., Matzku, S., Möller, P., Falk, W., et al. (1989). Monoclonal Antibody-Mediated Tumor Regression by Induction of Apoptosis. Science (New York N.Y.) 245 (4915), 301–305. doi: 10.1126/science.2787530
Twig, G., Elorza, A., Molina, A. J., Mohamed, H., Wikstrom, J. D., Walzer, G., et al. (2008). Fission and Selective Fusion Govern Mitochondrial Segregation and Elimination by Autophagy. EMBO J. 27 (2), 433–446. doi: 10.1038/sj.emboj.7601963
van Leeuwen, I. M., Higgins, M., Campbell, J., Brown, C. J., McCarthy, A. R., Pirrie, L., et al. (2011). Mechanism-Specific Signatures for Small-Molecule P53 Activators. Cell Cycle (Georgetown Tex.) 10 (10), 1590–1598. doi: 10.4161/cc.10.10.15519
Wai, T., García-Prieto, J., Baker, M. J., Merkwirth, C., Benit, P., Rustin, P., et al. (2015). Imbalanced OPA1 Processing and Mitochondrial Fragmentation Cause Heart Failure in Mice. Science (New York N.Y.) 350 (6265), d116. doi: 10.1126/science.aad0116
Wakabayashi, T. (1999). Structural Changes of Mitochondria Related to Apoptosis: Swelling and Megamitochondria Formation. Acta Biochim. Polonica 46 (2), 223–237. doi: 10.18388/abp.1999_4156
Wang, X., Hybiske, K., Stephens, R. S. (2017). Orchestration of the Mammalian Host Cell Glucose Transporter Proteins-1 and 3 by Chlamydia Contributes to Intracellular Growth and Infectivity. Pathog. Dis. 75 (8), ftx108. doi: 10.1093/femspd/ftx108
Wang, R., Mishra, P., Garbis, S. D., Moradian, A., Sweredoski, M. J., Chan, D. C. (2021). Identification of New OPA1 Cleavage Site Reveals That Short Isoforms Regulate Mitochondrial Fusion. Mol. Biol. Cell. 32 (2), 157–168. doi: 10.1091/mbc.E20-09-0605
Watanabe, T., Saotome, M., Nobuhara, M., Sakamoto, A., Urushida, T., Katoh, H., et al. (2014). Roles of Mitochondrial Fragmentation and Reactive Oxygen Species in Mitochondrial Dysfunction and Myocardial Insulin Resistance. Exp. Cell Res. 323 (2), 314–325. doi: 10.1016/j.yexcr.2014.02.027
Weber, M. M., Lam, J. L., Dooley, C. A., Noriea, N. F., Hansen, B. T., Hoyt, F. H., et al. (2017). Absence of Specific Chlamydia Trachomatis Inclusion Membrane Proteins Triggers Premature Inclusion Membrane Lysis and Host Cell Death. Cell Rep. 19 (7), 1406–1417. doi: 10.1016/j.celrep.2017.04.058
Westermann, B. (2010). Mitochondrial Fusion and Fission in Cell Life and Death. Nat. Rev. Mol. Cell Biol. 11 (12), 872–884. doi: 10.1038/nrm3013
Williamson, J., Hughes, C. M., Cobley, J. N., Davison, G. W. (2020). The Mitochondria-Targeted Antioxidant Mitoq, Attenuates Exercise-Induced Mitochondrial DNA Damage. Redox Biol. 36, 101673. doi: 10.1016/j.redox.2020.101673
Wu, S., Zhou, F., Zhang, Z., Xing, D. (2011). Mitochondrial Oxidative Stress Causes Mitochondrial Fragmentation via Differential Modulation of Mitochondrial Fission-Fusion Proteins. FEBS J. 278 (6), 941–954. doi: 10.1111/j.1742-4658.2011.08010.x
Yang, Y., Karakhanova, S., Hartwig, W., D'Haese, J. G., Philippov, P. P., Werner, J., et al. (2016). Mitochondria and Mitochondrial ROS in Cancer: Novel Targets for Anticancer Therapy. J. Cell Physiol. 231 (12), 2570–2581. doi: 10.1002/jcp.25349
Yan, L., Qi, Y., Ricketson, D., Li, L., Subramanian, K., Zhao, J., et al. (2020). Structural Analysis of a Trimeric Assembly of the Mitochondrial Dynamin-Like Gtpase Mgm1. Proc. Natl. Acad. Sci. U. S. A. 117 (8), 4061–4070. doi: 10.1073/pnas.1919116117
Yu, C., Zhao, J., Yan, L., Qi, Y., Guo, X., Lou, Z., et al. (2020). Structural Insights Into G Domain Dimerization and Pathogenic Mutation of OPA1. J. Cell Biol. 219 (7), e201907098. doi: 10.1083/jcb.201907098
Zemirli, N., Morel, E., Molino, D. (2018). Mitochondrial Dynamics in Basal and Stressful Conditions. Int. J. Mol. Sci. 19 (2), 564. doi: 10.3390/ijms19020564
Zhang, F., Dong, Z., Gao, S., Chen, G., Liu, D. (2019). AT1R-Mediated Apoptosis of Bone Marrow Mesenchymal Stem Cells is Associated With Mtros Production and Mtdna Reduction. Oxid. Med. Cell. Longevity 2019, 4608165. doi: 10.1155/2019/4608165
Zhao, M., Liu, S., Wang, C., Wang, Y., Wan, M., Liu, F., et al. (2021). Correction to "Mesenchymal Stem Cell-Derived Extracellular Vesicles Attenuate Mitochondrial Damage and Inflammation by Stabilizing Mitochondrial DNA". ACS Nano 15 (12), 20692. doi: 10.1021/acsnano.1c09895
Zhou, C., Huang, Y., Shao, Y., May, J., Prou, D., Perier, C., et al. (2008). The Kinase Domain of Mitochondrial PINK1 Faces the Cytoplasm. Proc. Natl. Acad. Sci. U. S. A 105 (33), 12022–12027. doi: 10.1073/pnas.0802814105
Keywords: Chlamydia, mitochondrial dynamics, DRP1, P53, ATP
Citation: Yang Y, Lei W, Zhao L, Wen Y and Li Z (2022) Insights Into Mitochondrial Dynamics in Chlamydial Infection. Front. Cell. Infect. Microbiol. 12:835181. doi: 10.3389/fcimb.2022.835181
Received: 14 December 2021; Accepted: 16 February 2022;
Published: 07 March 2022.
Edited by:
Stanimir Ivanov, Louisiana State University Health Shreveport, United StatesReviewed by:
Mary M. Weber, The University of Iowa, United StatesCopyright © 2022 Yang, Lei, Zhao, Wen and Li. This is an open-access article distributed under the terms of the Creative Commons Attribution License (CC BY). The use, distribution or reproduction in other forums is permitted, provided the original author(s) and the copyright owner(s) are credited and that the original publication in this journal is cited, in accordance with accepted academic practice. No use, distribution or reproduction is permitted which does not comply with these terms.
*Correspondence: Zhongyu Li, bHpoeTEwMjNAdXNjLmVkdS5jbg==
Disclaimer: All claims expressed in this article are solely those of the authors and do not necessarily represent those of their affiliated organizations, or those of the publisher, the editors and the reviewers. Any product that may be evaluated in this article or claim that may be made by its manufacturer is not guaranteed or endorsed by the publisher.
Research integrity at Frontiers
Learn more about the work of our research integrity team to safeguard the quality of each article we publish.