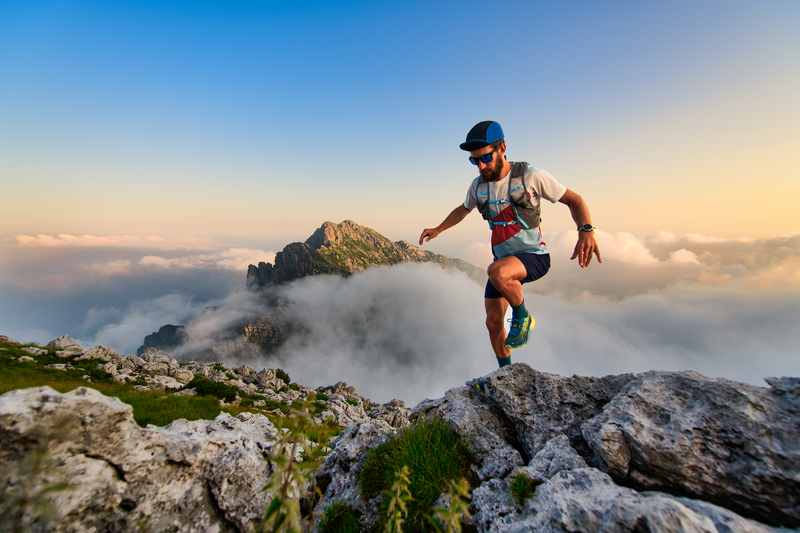
95% of researchers rate our articles as excellent or good
Learn more about the work of our research integrity team to safeguard the quality of each article we publish.
Find out more
ORIGINAL RESEARCH article
Front. Cell. Infect. Microbiol. , 17 February 2022
Sec. Parasite and Host
Volume 12 - 2022 | https://doi.org/10.3389/fcimb.2022.826818
This article is part of the Research Topic Babesia: Biology, Interactions, and Mechanisms of Pathogenesis in Ticks and Its Hosts View all 9 articles
Babesiosis poses a serious threat to immunocompromised individuals and the major etiological species of Babesia for human babesiosis is Babesia microti. Merozoites are a critical stage in the life cycle of Babesia microti. Several merozoite proteins have been demonstrated to play important roles in this process; however, most of the merozoite proteins of B. microti remain unknown. In the present study, we identified a novel merozoite protein of B. microti with similar structure to the thioredoxin (Trx)-like domain of the Trx family, which was named as B. microti Trx-like protein (BmTLP). Western blot assays demonstrated that this protein was expressed by B. microti during the erythrocytic infection process, and its expression peaked on day 7 post-infection in vivo. Immunofluorescence assay further showed that this protein is mainly expressed in B. microti merozoites. BmTLP hold both heparin- and erythrocyte-binding properties, which are critical functions of invasion-related proteins. Immunization with recombinant BmTLP imparted significant protection against B. microti infection in mice. Taken together, these results suggest that the novel merozoite protein, BmTLP, is an important pathogenic molecule of B. microti and may be a possible target for the design of babesiosis control strategy.
Babesiosis is a parasitic disease of the blood caused by Babesia, a pathogenic protozoon transmitted between humans and animals by hard ticks. Babesia belongs to the phylum Apicomplexa and comprises more than 100 species that infect a wide array of wild and domestic animals, but only few have been shown to infect humans, including B. microti, B. venatorum, B. bovis, B. divergens, B. duncani, B. bigemina, and B. crassa (Gorenflot et al., 1998; Schnittger et al., 2012). Nonetheless, for the majority of the reported cases of human babesiosis, the major etiological species is B. microti.
Babesiosis poses a serious threat to immunocompromised individuals. Asymptomatic, mild, and moderate infections generally occur in individuals who are immunocompetent (Krause et al., 2003; Krause et al., 2008; Vannier et al., 2008; de Ramon et al., 2016), whereas severe infections are common among immunocompromised patients and the elderly (Falagas and Klempner, 1996; Hatcher et al., 2001; Stowell et al., 2007; Krause et al., 2008; Wormser et al., 2010). The fatality rate is up to 21% among those with immunosuppression and 6–9% among hospitalized patients (Hatcher et al., 2001; Krause et al., 2008). Since the first case reported of human infection with Babesia in Yugoslavia in 1957 (Skrabalo and Deanovic, 1957), thousands of cases have been reported worldwide (Brasseur and Gorenflot, 1992; Quick et al., 1993; Kim et al., 2007; Gray et al., 2010; Senanayake et al., 2012). Indeed, babesiosis is now considered a global parasitic disease of humans and is attracting significant attention. Hence, there is an urgent need to identify new vaccines for effective control of babesiosis.
During the blood stage of their life cycle, Babesia merozoites invade the host erythrocytes, where they develop and multiply. After the divided trophozoites egress from the red blood cell (RBC) by rupturing host cells, the progeny merozoites invade new RBCs again (Yokoyama et al., 2002; Yokoyama et al., 2003; Kumar et al., 2004). Extracellular merozoites are directly exposed in the peripheral blood of hosts and can be eliminated by humoral immunity, whereas antibodies could never reach the parasites inside the RBCs (Yokoyama et al., 2002). Thus, merozoite proteins are potential antigen candidates for babesiosis vaccine and medication development. At present, the research on merozoite proteins of Babesia mainly focuses on surface molecules coating the extracellular merozoites, which attach to target erythrocytes and play key roles in erythrocyte invasion, such as merozoite surface antigen (MSA)-1, MSA-2a1, MSA-2a2, MSA-2b, and MSA-2c in B. bovis (Florin-Christensen et al., 2002), surface antigen of B. microti merozoites 44 (Wang et al., 2020), B. microti surface antigen 1 (Li et al., 2020). However, the detailed molecular interactions between Babesia merozoites and the host RBCs and the critical molecules in merozoite development are poorly understood. Identification of novel merozoite molecules is required for the development of preventive measures against babesiosis.
Thioredoxin (Trx) is one of the three major components of thioredoxin system, which has been identified in multiple eukaryotes and prokaryotes to maintain the redox balance (Jortzik and Becker, 2012; Holzerova et al., 2016). Moreover, Trx systems play critical roles in the immune response, virus infection, and cell death via interaction with thioredoxin-interacting protein (Lu and Holmgren, 2014). Besides Trxs, Trx-like proteins (TLPs) were also found in many pathogens. TLPs of Strongyloides ratti and Trichuris suis were secreted to bind to monocyte and induce release of cytokines to affect the host intestinal mucosa (Ditgen et al., 2016). Escherichia coli thioredoxin-like protein YbbN play a role in integrating the activities of different chaperone pathways in E. coli and related bacteria (Lin and Wilson, 2011). A novel protein of the thioredoxin (Trx) family, named PfTrx-mero protein, was revealed to localize on merozoites and judged to be an important ligand participating in erythrocyte invasion by P. falciparum (Zhang et al., 2013). There are 6 Trx genes of B. microti (Huang et al., 2018a). BmTrx2 and BmTrx3 were obtained and investigated. Both of them localize in the cell cytoplasm of B. microti merozoites and possess antioxidant enzyme activity (Huang et al., 2018a; Huang et al., 2018b). However, TLP of B. microti has not been reported so far. Understanding the molecular characterization and function of BmTrxs and BmTLPs may help to develop Babesiosis vaccines and anti-Babesia drugs.
In our study, the sequence alignment of PfTrx-mero protein, which localizes on the surface of Plasmodium merozoites, showed a homolog in B. microti, named as B. microti Trx-like protein (BmTLP). Due to the similar mechanism of host-parasite interaction between the two Apicomplexa parasites Plasmodium and Babesisa, the Trx-like protein of Babesia is likely to be a merozoite protein and play an important role in Babesia proliferation. Thus, in the present study, we generated recombinant BmTLP, and further studied its function and expression in B. microti. In addition, mice were immunized with recombinant BmTLP, and challenge experiments were performed to evaluate the value of this novel protein as target antigen of immunoprotection for babesiosis.
All animal procedures were conducted in accordance with the animal husbandry guidelines of the Chinese Academy of Medical Sciences and with permission from the Experimental Animal Committee of the Chinese Academy of Medical Sciences with the Ethical Clearance Number BYS20010.
B. microti strain ATCC PRA-99 was obtained from the American Type Culture Collection (Manassas, VA, USA) and stored in liquid nitrogen. Six-week-old male BALB/c mice (special pathogen free) were purchased from Vital River Laboratory Animal Technology Co. (Beijing, China). The parasites were cultured according to standard methods (Lu et al., 2012). Briefly, BALB/c mice were intraperitoneally injected with the immunosuppressant dexamethasone 0.5 mg per mice (18–22g) every day for five consecutive days to suppress immunity. Then, these mice were intraperitoneally inoculated with 200 μL of frozen B. microti-infected blood suspension. Smears were prepared with tail blood from mice, and Giemsa staining was performed to monitor the growth and proliferation of B. microti. The parasites were used as a stock for the following experiments, 6-week-old male BALB/c mice or immunized mice were intraperitoneally injected with 1 × 106 parasitized erythrocytes. For parasites isolation, the peripheral blood of infected mice was collected and filtered using Plasmodipur (EuroProxima, Amhem, The Netherlands) to remove leukocytes, according to the manufacturer’s instruction. Saponin (10% in phosphate-buffered saline [PBS]) was used to lyse erythrocytes, and the parasite precipitate was collected for the following experiments.
The gene sequence (https://www.ncbi.nlm.nih.gov/nuccore/XM_021482140.1) and amino acid sequence (https://www.ncbi.nlm.nih.gov/protein/1206245601/) of BmTLP were obtained from the National Center for Biotechnology Information. The signal peptide and transmembrane regions were predicted using Signal P 4.1 Server and TMHMM 2.0, respectively (Petersen et al., 2011). The overall domain structure was then predicted using the CDD protein annotation resource (https://www.ncbi.nlm.nih.gov/Structure/cdd/wrpsb.cgi). The amino acid sequence of BmTLP was aligned with that of PfTrx-like-mero protein using the DNAMAN V6 tool (Lynnon Biosoft, San Ramon, CA, USA). Further alignments were performed with BmTLP, PfTrx-like-mero protein, and other Trx domain-containing proteins from Homo sapiens (NP_001273876.1), Mus musculus (AY243534.1), Drosophila melanogaster (NP_572212.1), Arabiodopsis thaliana (AAF04439.1), and Schistosoma japonicum (CAX71381.1) to determine the functional sites of the Trx domain. All these protein sequences were obtained from the National Center for Biotechnology Information.
Total RNA was extracted from B. microti parasites using TRIzol reagent (Life Technologies, Waltham, MA, USA) as previously described (Zhang et al., 2016). Genomic DNA was removed from total RNA using the TURBO DNA-free TM Kit (Thermo Fisher Scientific, Waltham, MA, USA), and reverse transcription was performed using SuperScript III Reverse Transcriptase (Thermo Fisher Scientific) according to the manufacturer’s instructions. The gene fragment encoding BmTLP without the 17 signal peptide amino acid at N-terminal was amplified using high fidelity Phusion DNA polymerase (Finnzymes Oy, Espoo, Finland). All primers used in this study (Supplementary Table 1) were designed using Primer BLAST (https://www.ncbi.nlm.nih.gov/tools/primer-blast/). The amplified product was purified using a DNA Gel Extraction Kit (Axygen, Corning, NY, USA), cloned into PGEX-4T-1 and PET-28a expression vectors, respectively. The sequence coding for a lysine-to-alanine mutation at the 58 and 353 positions within the two GAG binding motifs of BmTLP was chemically synthesized and cloned into the PGEX-4T-1 vectors. All clones were expressed in Escherichia coli BL21 (DE3) (Smith and Johnson, 1988; Flick et al., 2004). The glutathione S-transferase (GST) and His-tagged recombinant proteins were purified using glutathione-Sepharose 4B and His Gravi Trap affinity columns (GE Healthcare, Chicago, IL, USA) respectively, according to the manufacturer’s instructions. All proteins were analyzed by 12% sodium dodecyl-sulfate polyacrylamide gel electrophoresis (SDS-PAGE) and western blot with monoclonal antibodies against His-tag or GST-tag (Cell Signaling Technology, Danvers, MA, USA).
B. microti parasites were collected at 0, 1, 3, 5, 7, 9, 11, and 13 days post-infection. Total RNA was extracted, and reverse transcription was performed as described above. Real-time quantitative polymerase chain reaction (qPCR) experiments were performed using the SYBR Premix Ex Taq II (Takara, Kusatsu, Japan), according to the manufacturer’s instructions, on a 7300 Real-Time PCR System (Applied Biosystems, Waltham, MA, USA). Each reaction was performed in a final volume of 25 μL, containing 12.5 μL of 2 × Brilliant II SYBR green QPCR master mix, 100 ng cDNA, and 1 μL (10 μM) paired primers. The PCR program was performed for 40 cycles with denaturation at 95°C for 30 s, followed by annealing and extension at 60°C for 1 min. Relative expression was analyzed using the SDS 1.4 software (Applied Biosystems). The specific primers for qPCR were designed using Primer BLAST (https://www.ncbi.nlm.nih.gov/tools/primer-blast/) and are listed in Supplementary Table 1. 18S ribosomal RNA of B. microti (Bm18S) (GenBank ID: M93660.1) was used as reference gene (Huang et al., 2018a). The efficiency of the primer pairs was calculated using the LinRegPCR program based on the raw fluorescence data generated by qPCR (Ruijter et al., 2009).
To confirm the expression of BmTLP in the parasites, rabbit polyclonal antibodies were prepared at Beijing Protein Innovation (Beijing, China) by immunizing New Zealand white rabbits with recombinant BmTLP. 10% Saponin (in PBS) was used to lyse erythrocytes, the parasites were washed for three times with PBS and collected by centrifugation. Total protein was extracted from the parasites at the indicated time points (0, 1, 3, 5, 7, 9, 11, and 13 days post-infection) with RIPA buffer (Solarbio Life Science, Beijing, China). Protein concentrations were quantified by using a BCA kit (Pierce Biotechnology, Waltham, MA, USA), according to the manufacturer’s instructions. The extracted protein and recombinant proteins were separated on a 12% SDS-PAGE gel and analyzed by western blot. After electrophoresis, the proteins were transferred to polyvinylidene fluoride membranes (Millipore, Burlington, MA, USA). Rabbit anti-rBmTLP serum (1:1,000 dilutions) was used as primary antibody, and IRDye 800 CW conjugated goat anti-rabbit IgG (H+L) antibody (1:10,000 dilution, Li-COR Biosciences, Lincoln, NE, USA) was used as secondary antibody. In this study, we performed parallel experiments using rabbit glyceraldehyde-3-phosphate dehydrogenase (GAPDH) (Cell Signaling Technology) as the primary antibody (Huang et al., 2018a). Detections were then made using Odyssey (Li-COR Biosciences).
To further assess the location of the BmTrx-like protein in the parasite, immunofluorescence assays were performed. Blood smears were prepared with tail blood from B. microti-infected mice at day 7 post-infection. After fixing with methanol for 10 min at 4°C, 0.1% TritonX-100 was used to penetrate. Then the slides were incubated with 5% bovine serum albumin for 1 h and then washed three times with PBS. Rabbit anti-BmTLP serum (diluted 1:500 in 3% bovine serum albumin) was applied as primary antibody at 4°C overnight. The slides were then incubated with Alexa-Fluor 488 conjugated goat anti-rabbit IgG antibody and 4ʹ, 6-diamidino-2-phenylindole (DAPI) (Invitrogen, Waltham, MA, USA). The samples were examined using a confocal laser-scanning microscope (Zeiss LSM 880, Oberkochen, Germany).
The binding of BmTLP to heparin was studied as previously described with some improvement (Chen et al., 1998). In briefly, BmTLP (final concentration 0.1 μg/μl) and equal amounts of GST protein were mixed with 100 µL heparin-sepharose or uncoupled sepharose 4B (GE Healthcare) in a 200 µL reaction system in PBS at 25°C for 2 h. Binding proteins were eluted by using 1 M sodium chloride buffer and detected by western blot using monoclonal antibodies to the GST-tag.
The binding activity of BmTLP toward human and mouse erythrocytes was studied as previously described with some improvements (Wang et al., 2018). In briefly, BmTLP (final concentration 0.1 μg/μl) and equal amount of GST protein were mixed with 100 µL human or mouse erythrocyte in a total of 200 µL system in RBC binding buffer (50 mM Tris HCl, 200 mM NaCl, 1 mM EDTA, 2.5 mM MgCl2, 2.0 mM DTT, 1% glycerin, pH 8.0), respectively, at 25°C for 2 h. The mixture was washed three times with RBC binding buffer. Binding proteins were subsequently eluted with 1 M sodium chloride. The bound proteins were then detected by western blot using monoclonal antibodies to GST-tag.
To assess the protective role of BmTLP immunization against babesiosis infection, immunization was performed with His-tagged recombinant BmTLP and PBS as control. Mice were evenly divided into immunization and control groups. In the first immunization, each mouse (6-week-old male BALB/c mice) in the immunization group was subcutaneously injected with 60 µg His-tagged BmTLP or corresponding volume of PBS emulsified with complete Freund’s adjuvant. In the three subsequent immunizations, each mouse was injected with 30 µg recombinant proteins or corresponding volume of PBS with incomplete Freund’s adjuvant every 2 weeks. The antibody titer was measured using enzyme-linked immunosorbent assay (ELISA) as previously described (Wang et al., 2018). After successful immunization, mice were challenged with 1 × 106 infected (i)RBCs. Thin blood smears were made with tail blood from mice and stained with Giemsa to assess parasitemia every other day by counting 1,000 RBCs per smear and totally three smears as previously described (Wang et al., 2018). The interferon (IFN)-γ and tumor necrosis factor (TNF)-α levels in mouse serum were determined using Mouse IFN-γ ELISA Kit and Mouse TNF-α ELISA Kit (R&D Systems, Minneapolis, MN, USA), according to the manufacturer’s instructions.
The data were analyzed using GraphPad Prism 5.0 (GraphPad Software, San Diego, CA, USA) and Microsoft Excel 2010. The statistical significance of experimental data was evaluated using Mann-Whitney test between two groups and one-way analysis of variance (ANOVA) among more groups. Values of p < 0.05 were deemed significant differences.
In our earlier study on heparin-binding merozoite proteins of P. falciparum, a novel protein, PfTrx-mero protein, containing a conserved domain of PfTrx-like-mero was identified (Zhang et al., 2013; Wang et al., 2018). In the present study, we report a novel protein encoded by BMR1_03g03170 in B. microti, which was homologous to PfTrx-like-mero of P. falciparum (Identities: 36%, Figure 1A). The amino acid sequence of this Babesia protein was found to contain two Trx-like domains (Figure 1C); thus, it was named as B. microti BmTrx-like protein (BmTLP). The Cys-X-X-Cys (CXXC) active site, which is essential for antioxidant function, was identified within the sequence of most of the Trx-like proteins. However, the ‘-CXXC-’ motif was missing in the sequence of both, PfTrx-mero and BmTrx-like proteins (Figure 1B). The sequence of BmTLP contained a 17-amino acid long signal peptide (Figure 1C), indicating that the protein may have a role on the surface or in the endoplasmic reticulum of the parasites, or even outside of the organism.
Figure 1 Amino acid sequence alignments and schematic diagram of BmTrx-like protein. (A) Amino acid sequence alignment of Trx-like proteins of B. microti and P. falciparum. (B) Amino acid sequence alignment of the Trx-like protein of B. microti with Trx proteins of other species. A gap (blue double-headed arrow) was observed in both BmTrx-like protein and PfTrx-like-mero protein sequences due to the deletion of the Cys-X-X-Cys (CXXC) active site. (C) Schematic diagram of the BmTrx-like protein. This protein contained a signal peptide (SP; aa 1–17) at the N-terminus and two conserved Trx domains (Thioredoxin_like superfa, aa 28–95; Thioredoxin_6, aa 175–375). The blue boxes indicate the two glycosaminoglycan (GAG)-binding motifs.
Recombinant BmTLP (rBmTLP) with a His-tag or a GST-tag was generated. The molecular weight was 46 kDa for the recombinant His-tagged BmTLP and 72 kDa for the GST-tagged protein (Figure 2A, B). Anti-B. microti serum, obtained from mice 21 days post-infection of B. microti, was used to detect the rBmTLP by western blot. As shown in the western blot analyses, recombinant rBmTLP could be identified by infected serum but not by serum from uninfected mice (Figure 2C).
Figure 2 Detection of recombinant BmTrx-like protein (BmTLP). (A) Recombinant BmTLP were detected by SDS-PAGE and Coomassie brilliant blue staining. Lane 1, His-tagged rBmTLP; Lane 2, GST-tagged rBmTLP. (B) His-tagged (lane 1) and GST-tagged (lane 2) recombinant BmTLP were detected by western blot with monoclonal antibodies against His-tag (Lane 1) or GST-tag (Lane 2) respectively. (C) His-tagged recombinant BmTLP were detected by western blot with a mixture of serum samples (equal volumes) from seven normal mice (Lane 1) or seven B. microti-infected BALB/c mice 21-day post-infection (Lane 2). Lane M, standard protein molecular weight marker.
Mice were infected with B. microti to obtain parasites at indicated times, the dynamic parasitemia of the mice were showed in Supplementary Figure 2. The expression features of BmTLP were further explored. Transcriptional expression of BmTLP-encoding gene was detected by real-time qPCR at 1, 3, 5, 7, 9, 11, and 13 days post-infection. Both the primers of BmTLP and Bm18S showed high amplification efficiency (96.7% for BmTLP and 91.1% for Bm18S). Specific gene expression increased on day 3 post-infection, peaked on day 7, and then gradually decreased (Figure 3A). Rabbit anti-BmTLP serum was used to detect native protein in the parasites by western blot. Specific bands could be detected in parasite lysates since day 1 post-infection, peaked at day 7 post-infection, and a sharp decline of the expression was observed on day 13 post-infection (Figure 3B). The location of BmTLP was detected by indirect immunofluorescence, with DAPI showing the nuclear chromatin (Figure 3C). BmTLP may locate in the cytoplasm of merozoites. There was no positive fluorescence of BmTLP in infected erythrocytes incubated with normal rabbit IgG. Results indicated that BmTLP is expressed in merozoites of B. microti.
Figure 3 Expression of Trx-like protein (BmTLP) in B. microti. B. microti parasites were collected from Babesia-infected mice 0, 1, 3, 5, 7, 9, 11, and 13 days post-infection (dpi). (A) Transcriptional expression of BmTLP-encoding gene in B. microti parasites from infected mice detected by real-time qPCR with 18S ribosomal RNA of B. microti used as reference gene. The results are representative of three independent experiments, with data indicating mean + standard deviation. Asterisks indicate comparison with the previous group with *p < 0.05, **p < 0.01, and ***p < 0.0001. (B) Expression of BmTLP detected by western blot. Lane M, standard protein molecular weight marker. Lysates of parasite were detected with rabbit anti- BmTLP serum. Glyceraldehyde-3-phosphate dehydrogenase (GAPDH) was used as internal reference. (C) Localization of BmTLP detected by immunofluorescence assay. Thin blood smears were prepared with tail blood from B. microti-infected BABL/c mice at day 7 post-infection. Proteins were detected with rabbit anti-rBmTLP serum or rabbit IgG control, followed by anti-rabbit IgG secondary antibody conjugated with Alexa-488 (green). Nuclei were stained with DAPI (blue). Scale bar, 2 µm.
To elucidate the role of the BmTLP in the invasion process, GST-tagged recombinant proteins were separately incubated with heparin-sepharose, and the binding proteins were then detected by western blot. As shown in Figure 4A, GST-tagged rBmTLP could bind heparin, whereas the GST protein did not. Further assays were performed to detect the binding activity of the BmTLP to the host erythrocytes. The results showed that BmTLP can bind both human (Figure 4B) and mouse erythrocytes (Figure 4C). To further investigate whether the binding function of BmTLP plays a role through the GAG binding motifs, we introduced a lysine-to-alanine mutation at the 58 and 353 positions within the two GAG binding motifs of BmTLP. The mutant rBmTLP showed no binding activity (Supplementary Figure 1).
Figure 4 Binding activity of recombinant BmTrx-like protein (BmTLP). The glutathione S-transferase (GST) tagged rBmTLP (rTLP) and GST protein (GST) were incubated with (A) heparin-sepharose (h-sepharose), (B) mouse erythrocytes, or (C) human erythrocytes at 25°C for 2 h Recombinant proteins was incubated with sepharose without heparin as negative control. Eluted proteins were collected and detected by western blot. The GST tagged rBmTLP and GST were used as positive controls. Rabbit anti-GST Tag monoclonal antibody was used as primary antibody. The results are representative of three independent experiments.
Immunization with PfTrx-mero protein has been proven to provide significant protection against Plasmodium infection (Wang et al., 2018); thus, we further studied the protective role of BmTLP immunization against B. microti infection in vivo. His-tagged BmTLP was used to immunize BALB/C mice. The titers of antibodies in mice serum were detected by ELISA after the third booster immunization (Supplementary Figure 3B). After successful immunization confirmed by ELISA, a challenge with 1 × 106 B. microti-iRBCs was implemented. The parasitemia was significantly lower in the BmTLP immunized groups; especially at day 9 post-infection, the parasitemia decreased more than 50%, compared to that in the PBS control group (BmTLP group vs. PBS group, 2.43% ± 0.33% vs. 5.95% ± 0.30%, p < 0.0001, Figure 5A). Although there was no significant difference in the body weight of mice between the two groups (Supplementary Figure 4A), the spleen weight of the BmTLP immunized mice was significantly lower than that of the PBS control (Supplementary Figure 4B). Furthermore, the levels of the inflammatory cytokines IFN-γ and TNF-α in BmTLP immunized mice were much lower than those in PBS control mice (Figures 5B, C), indicating that rBmTLP immunization may inhibit the excessive inflammatory response induced by Babesia infection. These results suggest that BmTLP immunization reduces erythrocyte invasion of the parasites and inflammation level of the host under the B. microti infection.
Figure 5 Immunization with recombinant BmTrx-like protein (BmTLP) protected mice against B. microti infection. His-tagged BmTLP or PBS was used to immunize BALB/C mice (seven mice per group). After successful immunization, a challenge with 1 × 106 B. microti-infected red blood cells was implemented. Parasitemia (A) was examined with tail blood by performing Giemsa staining and IFN-γ (B) and TNF-α (C) in mice serum were detected by ELISA 0, 1, 3, 5, 7, 9, 11, 13, and 15 days post-infection (dpi). The results are representative of two independent experiments. Data indicate the mean ± standard deviation. Asterisks indicate comparison between the two groups at each time point with *p < 0.05, **p < 0.01, and ***p < 0.0001.
Babesiosis imposes an enormous threat on immunocompromised individuals, with death from babesiosis occurs in up to 20% individuals within this group (Hatcher et al., 2001; Krause et al., 2008). The incidence of B. microti infections has increased and the geographic range has expanded globally in the past two decades (Gorenflot et al., 1998; Vannier and Krause, 2012). Treatment of babesiosis generally depends on antibiotics, such as atovaquone and azithromycin (Krause et al., 2000; Kletsova et al., 2017). However, antibiotic therapy may not adequately treat immunocompromised patients (Gorenflot et al., 1998). A potent vaccine based on microbial recombinant antigens would be the most effective tool to prevent this disease. Effective B. bovis and B. bigemina vaccines have been developed for use in cattle (Brown and Palmer, 1999), and a B. rossi vaccine has been used for dogs (Schetters et al., 2007), but no human Babesia vaccine has been developed to date. Progress has been limited due to the small quantity of identified parasite proteins in human Babesia. Thus, exploration of novel proteins that play critical roles in the development stages of Babesia will provide candidates for human babesiosis vaccine development.
In the erythrocytic phase of human Babesia, merozoite is the only stage that directly contacts with the host peripheral blood (Gorenflot et al., 1998; Vannier and Krause, 2012). The other stages all occur within erythrocytes. Erythrocytes have only basic metabolic activity and no antigen-presenting pathways; therefore, parasitism of erythrocytes provides Babesia with an evolutionary advantage in evading host recognition (Gorenflot et al., 1998). Furthermore, merozoite proteins play key roles in erythrocyte invasion by adherence to and penetration into erythrocytes (Baum et al., 2009). Thus, the proteins of merozoite are optimal candidate antigens for a Babesiosis vaccine. In the present study, a novel B.microti Trx-like protein BmTLP was identified and found to localize in the cell cytoplasm of B. microti merozoites.
The amino acid sequence of BmTLP contains two Trx-like domains. Trxs are disulfide oxidoreductases that utilize a pair of cysteines in the active site, Cys-X-X-Cys (CXXC), to reduce disulfide bonds (Ju et al., 2015; Wedmann et al., 2016; Peng et al., 2017). The active site cysteines of mammalian thioredoxin were reported to be important for the reduction of a protein persulfide since mutation of the attacking cysteine of Trx weakens the binding affinity to persulfidated GAPDH (Ju et al., 2015). However, the CXXC motif was missing in the sequence of both PfTrx-mero and BmTrx-like proteins. The Trx domain of Escherichia coli Trx-like protein YbbN also lacks a canonical CXXC active site architecture and YbbN is not a functional oxidoreductase, but it can regulate the activities of chaperones (Lin and Wilson, 2011). PfTrx-mero protein was judged to be an important ligand participating in erythrocyte invasion by P. falciparum (Zhang et al., 2013). Thus, BmTLP may be primarily useful for B. microti in other ways than antioxidant.
Trxs, despite its function as an intracellular disulfide reducing enzyme, has been found to play some extracellular roles. Human Trx was first identified as a secreted protein of the adult T cell leukemia and implicated in a wide variety of cell-to-cell communication systems, acting as a cytokine or a chemokine (Tagaya et al., 1989). The same extracellular Trx was identified in the conditioned medium of Epstein-Barr virus-transformed lymphocytes and acts as an autocrine growth factor and synergizes with interleukin 1 and interleukin 2 (Wakasugi et al., 1990). However, BmTLP is localized in the cell cytoplasm of merozoites in B. microti-infected erythrocytes. Unlike mammalian Trx that are lack of signal sequences, a classical signal peptide sequence was predicted at the N-terminus of both PfTrx-like-mero protein and BmTLP. Even in the absence of signal peptides, Trxs can be secreted through unconventional protein secretion pathways, but not the endoplasmic reticulum and Golgi pathway (Rubartelli et al., 1992). These data strongly suggests that BmTLP may be secreted extracellular.
The biological functions of extracellular thioredoxin have also been proved to be involved in the immune response with or without thiol-oxidoreductase activity. Trx is secreted by CD4+ T cells and can reduce the disulfide in domain 2 of CD4, which are important for the entry of HIV-I into susceptible cells (Matthias et al., 2002). Trx is shown to be a growth promoting factor for several lymphoid cells with synergistic effects with cytokines such as IL-1 and IL-2 (Tagaya et al., 1990; Wakasugi et al., 1990). TRX80, a proteolytic product of Trx 1, were recruited by the immune system to exert cell communication functions, such as promoting proinflammatory macrophage phenotype (Mahmood et al., 2013) and activating the classical and alternative pathways of complement activation (King et al., 2012). The thioredoxin-like protein can down-modulate the innate immune responses by inhibiting ASK1-MAPKs signaling cascades during Edwardsiella piscicida infection (Yang et al., 2019). Trx-like protein from gastrointestinal parasitic nematodes Strongyloides ratti and Trichuris suis is secreted and bind to monocytic and intestinal epithelial cells and induce the time-dependent release of cytokines such as TNF-α, IL-22, and TSLP (Ditgen et al., 2016). Our in vivo experiments showed that rBmTLP immunization could reduce the production of inflammatory cytokines IFN-γ and TNF-α in host circulation, thus may inhibit the excessive inflammatory response induced by Babesia infection. However, the immunoregulatory function of Trx is quite complicated and needs further study.
BmTLP contains two Trx domains and two glycosaminoglycans-binding motifs, which are both believed to be related to invasion or adhesion of the parasite (Wang et al., 2018). In vitro experiments showed that recombinant BmTLP could bind to host erythrocytes and had heparin-binding activity. Heparin-binding molecules on the surface of merozoites play a crucial role in the erythrocyte invasion process (Bork et al., 2004). Heparin sulfate-like moieties on the surface of human erythrocytes have been shown to be receptors for merozoite-derived proteins of Plasmodium falciparum (Vogt et al., 2004; Boyle et al., 2010). Toxoplasma gondii surface antigen also has heparin-binding properties and mediates attachment of the tachyzoite to the cellular heparin sulfate proteoglycans of host cells (Jacquet et al., 2001). These data suggested BmTLP may be involved in merozoite-erythrocyte communication. However, whether and how BmTLP could be secreted by B. microti needs further study.
Finally, in vivo assays were performed to demonstrate the role of the BmTLP in parasite development and proliferation. The rBmTLP immunized mice showed much lower parasitemia during infection and early clearance of the parasites. In line with the parasitemia, the serum level of inflammatory cytokines was much lower in the rBmTLP immunized mice, which may lead to milder symptoms during disease progression, as inflammation induced by the parasites is the main cause of clinical symptoms in protozoan infection (Schofield and Grau, 2005). Thus, immunization with BmTLP resulted in accelerated clearance of the parasite during the infection with lower inflammatory levels. These data indicate that BmTLP plays critical roles in Babesia infection. However, until now, the in vitro cultivated system and gene manipulating methods of B. microti are not mature and it is difficult to confirm the direct involvement of BmTLP in parasite invasion in vitro. Recently, two studies reported the establishment of transient or stable transfection method in B. microti and the discovery of a novel bidirectional promoter of B. microti (Jaijyan et al., 2020; Liu et al., 2020), raising hope for gene-editing research in Babesia.
In summary, we have identified a novel Trx-like protein in B. microti, named as BmTLP, which mainly expressed in Babesia merozoites and holds heparin and erythrocyte-binding properties. Immunization with rBmTLP provided significant protection against B. microti infection in vivo, thereby suggesting the important roles of this protein in parasite pathogenicity and its potential value for the development of babesiosis vaccines. However, the exact pathogenic mechanism of BmTLP still needs to be investigated.
The original contributions presented in the study are included in the article/Supplementary Material. Further inquiries can be directed to the corresponding authors.
The animal study was reviewed and approved by the Experimental Animal Committee of the Chinese Academy of Medical Sciences.
NH and QC conceived and designed experiments. XP and YM performed the majority of the experiments. SL performed some experiments. NH, XP, and QC analyzed the data and wrote the manuscript. All authors reviewed the results and approved the final version of the manuscript.
This research was funded by the CAMS Innovation Fund for Medical Sciences (CIFMS) (grant numbers 2021-I2M-1-038 and 2019-I2M-5-042), the National Natural Science Foundation of China (grant number 81672050).
The authors declare that the research was conducted in the absence of any commercial or financial relationships that could be construed as a potential conflict of interest.
All claims expressed in this article are solely those of the authors and do not necessarily represent those of their affiliated organizations, or those of the publisher, the editors and the reviewers. Any product that may be evaluated in this article, or claim that may be made by its manufacturer, is not guaranteed or endorsed by the publisher.
We would like to thank Editage (www.editage.cn) for English language editing.
The Supplementary Material for this article can be found online at: https://www.frontiersin.org/articles/10.3389/fcimb.2022.826818/full#supplementary-material
Baum, J., Chen, L., Healer, J., Lopaticki, S., Boyle, M., Triglia, T., et al. (2009). Reticulocyte-Binding Protein Homologue 5-An Essential Adhesin Involved in Invasion of Human Erythrocytes by Plasmodium Falciparum. Int. J. Parasitol. 39 (3), 371–380. doi: 10.1016/j.ijpara.2008.10.006
Bork, S., Yokoyama, N., Ikehara, Y., Kumar, S., Sugimoto, C., Igarashi, I. (2004). Growth-Inhibitory Effect of Heparin on Babesia Parasites. Antimicrob. Agents Chemother. 48 (1), 236–241. doi: 10.1128/Aac.48.1.236-241.2004
Boyle, M. J., Richards, J. S., Gilson, P. R., Chai, W. G., Beeson, J. G. (2010). Interactions With Heparin-Like Molecules During Erythrocyte Invasion by Plasmodium Falciparum Merozoites. Blood 115 (22), 4559–4568. doi: 10.1182/blood-2009-09-243725
Brasseur, P., Gorenflot, A. (1992). Human Babesiosis in Europe. Memorias. Do. Inst. Oswaldo. Cruz. 87, 131–132. doi: 10.1590/S0074-02761992000700019
Brown, W. C., Palmer, G. H. (1999). Designing Blood-Stage Vaccines Against Babesia Bovis and B. Bigemina. Parasitol. Today 15 (7), 275–281. doi: 10.1016/s0169-4758(99)01471-4
Chen, Q., Barragan, A., Fernandez, V., Sundstrom, A., Schlichtherle, M., Sahlen, A., et al. (1998). Identification of Plasmodium Falciparum Erythrocyte Membrane Protein 1 (PfEMP1) as the Rosetting Ligand of the Malaria Parasite P-Falciparum. J. Exp. Med. 187 (1), 15–23. doi: 10.1084/jem.187.1.15
de Ramon, C., Cid, J., Rodriguez-Tajes, S., Alvarez-Martinez, M. J., Valls, M. E., Fernandez, J., et al. (2016). Severe Babesia Microti Infection in an American Immunocompetent Patient Diagnosed in Spain. Transf. Apheresis. Sci. 55 (2), 243–244. doi: 10.1016/j.transci.2016.07.021
Ditgen, D., Anandarajah, E. M., Hansmann, J., Winter, D., Schramm, G., Erttmann, K. D., et al. (2016). Multifunctional Thioredoxin-Like Protein From the Gastrointestinal Parasitic Nematodes Strongyloides Ratti and Trichuris Suis Affects Mucosal Homeostasis. J. Parasitol. Res. 2016, 8421597 doi: 10.1155/2016/8421597
Falagas, M. E., Klempner, M. S. (1996). Babesiosis in Patients With AIDS: A Chronic Infection Presenting as Fever of Unknown Origin. Clin. Infect. Dis. 22 (5), 809–812. doi: 10.1093/clinids/22.5.809
Flick, K., Ahuja, S., Chene, A., Bejarano, M. T., Chen, Q. J. (2004). Optimized Expression of Plasmodium Falciparum Erythrocyte Membrane Protein I Domains in Escherichia Coli. Malaria J. 15 (3), 50. doi: 10.1186/1475-2875-3-50
Florin-Christensen, M., Suarez, C. E., Hines, S. A., Palmer, G. H., Brown, W. C., McElwain, T. F. (2002). The Babesia Bovis Merozoite Surface Antigen 2 Locus Contains Four Tandemly Arranged and Expressed Genes Encoding Immunologically Distinct Proteins. Infect. Immun. 70 (7), 3566–3575. doi: 10.1128/Iai.70.7.3566-3575.2002
Gorenflot, A., Moubri, K., Precigout, E., Carcy, B., Schetters, T. P. M. (1998). Human Babesiosis. Ann. Trop. Med. Parasitol. 92 (4), 489–501. doi: 10.1080/00034989859465
Gray, J., Zintl, A., Hildebrandt, A., Hunfeld, K. P., Weiss, L. (2010). Zoonotic Babesiosis Overview of the Disease and Novel Aspects of Pathogen Identity. Ticks. Tick-Borne. Dis. 1 (1), 3–10. doi: 10.1016/j.ttbdis.2009.11.003
Hatcher, J. C., Greenberg, P. D., Antique, J., Jimenez-Lucho, V. E. (2001). Severe Babesiosis in Long Island: Review of 34 Cases and Their Complications. Clin. Infect. Dis. 32 (8), 1117–1125. doi: 10.1086/319742
Holzerova, E., Danhauser, K., Haack, T. B., Kremer, L. S., Melcher, M., Ingold, I., et al. (2016). Human Thioredoxin 2 Deficiency Impairs Mitochondrial Redox Homeostasis and Causes Early-Onset Neurodegeneration. Brain 139, 346–354. doi: 10.1093/brain/awv350
Huang, J. W., Xiong, K., Zhang, H. S., Zhao, Y. Z., Cao, J., Gong, H. Y., et al. (2018a). Molecular Characterization of Babesia Microti Thioredoxin (BmTrx2) and Its Expression Patterns Induced by Antiprotozoal Drugs. Parasit. Vectors 11 (1), 38. doi: 10.1186/s13071-018-2619-9
Huang, J. W., Xiong, K., Zhang, H. S., Zhao, Y. Z., Cao, J., Zhou, Y. Z., et al. (2018b). Babesia Microti Thioredoxin 3 is an Effective Antioxidant and Involved in the Response to Antiprotozoal Drugs. Ticks. Tick-Borne. Dis. 9 (3), 645–653. doi: 10.1016/j.ttbdis.2018.01.021
Jacquet, A., Coulon, L., De Neve, J., Daminet, V., Haumont, M., Garcia, L., et al. (2001). The Surface Antigen SAG3 Mediates the Attachment of Toxoplasma Gondii to Cell-Surface Proteoglycans. Mol. Biochem. Parasitol. 116 (1), 35–44. doi: 10.1016/S0166-6851(01)00297-3
Jaijyan, D. K., Govindasamy, K., Singh, J., Bhattacharya, S., Singh, A. P. (2020). Establishment of a Stable Transfection Method in Babesia Microti and Identification of a Novel Bidirectional Promoter of Babesia Microti. Sci. Rep. 10, 15614. doi: 10.1038/s41598-020-72489-3
Jortzik, E., Becker, K. (2012). Thioredoxin and Glutathione Systems in Plasmodium Falciparum. Int. J. Med. Microbiol. 302 (4-5), 187–194. doi: 10.1016/j.ijmm.2012.07.007
Ju, Y., Wu, L., Yang, G. (2015). Thioredoxin 1 Regulation of Protein S-Desulfhydration. Biochem. Biophys. Rep. 30 (5), 27–34. doi: 10.1016/j.bbrep.2015.11.012
Kim, J. Y., Cho, S. H., Joo, H. N., Tsuji, M., Cho, S. R., Park, I. J., et al. (2007). First Case of Human Babesiosis in Korea: Detection and Characterization of a Novel Type of Babesia Sp (KO1) Similar to Ovine Babesia. J. Clin. Microbiol. 45 (6), 2084–2087. doi: 10.1128/Jcm.01334-06
King, B. C., Nowakowska, J., Karsten, C. M., Kohl, J., Renstrom, E., Blom, A. M. (2012). Truncated and Full-Length Thioredoxin-1 Have Opposing Activating and Inhibitory Properties for Human Complement With Relevance to Endothelial Surfaces. J. Immunol. 188 (8), 4103–4112. doi: 10.4049/jimmunol.1101295
Kletsova, E. A., Spitzer, E. D., Fries, B. C., Marcos, L. A. (2017). Babesiosis in Long Island: Review of 62 Cases Focusing on Treatment With Azithromycin and Atovaquone. Ann. Clin. Microbiol. Antimicrob. 16 (1), 26. doi: 10.1186/s12941-017-0198-9
Krause, P. J., Gewurz, B. E., Hill, D., Marty, F. M., Vannier, E., Foppa, I. M., et al. (2008). Persistent and Relapsing Babesiosis in Immunocompromised Patients. Clin. Infect. Dis. 46 (3), 370–376. doi: 10.1086/525852
Krause, P. J., Lepore, T., Sikand, V. K., Gadbaw, J. J., Burke, G., Telford, S. R., et al. (2000). Atovaquone and Azithromycin for the Treatment of Babesiosis. New Engl. J. Med. 343 (20), 1454–1458. doi: 10.1056/Nejm200011163432004
Krause, P. J., McKay, K., Gadbaw, J., Christianson, D., Closter, L., Lepore, T., et al. (2003). Increasing Health Burden of Human Babesiosis in Endemic Sites. Am. J. Trop. Med. Hyg. 68 (4), 431–436. doi: 10.4269/ajtmh.2003.68.431
Kumar, S., Yokoyama, N., Kim, J. Y., Huang, X. H., Inoue, N., Xuan, X. N., et al. (2004). Expression of Babesia Equi EMA-1 and EMA-2 During Merozoite Developmental Stages in Erythrocyte and Their Interaction With Erythrocytic Membrane Skeleton. Mol. Biochem. Parasitol. 133 (2), 221–227. doi: 10.1016/j.molbiopara.2003.10.010
Li, M. X., Ao, Y. S. Q., Guo, J. Y., Nie, Z., Liu, Q., Yu, L., et al. (2020). Surface Antigen 1 Is a Crucial Secreted Protein That Mediates Babesia Microti Invasion Into Host Cells. Front. Microbiol. 10. doi: 10.3389/fmicb.2019.03046
Lin, J. S., Wilson, M. A. (2011). Escherichia Coli Thioredoxin-Like Protein YbbN Contains an Atypical Tetratricopeptide Repeat Motif and Is a Negative Regulator of GroEL. J. Biol. Chem. 286 (22), 19459–19469. doi: 10.1074/jbc.M111.238741
Liu, M., Ji, S., Rizk, M. A., Adjou, M. P. F., Galon, E. M., Li, J., et al. (2020). Transient Transfection of the Zoonotic Parasite Babesia Microti. Pathogens 9 (2), 108. doi: 10.3390/pathogens9020108
Lu, Y., Cai, Y., Chen, S., Chen, J., Guo, J., Chen, M. (2012). Establishment of the Experimental Animal Model of Babesia Microti. Zhongguo. Ji. Sheng. Chong. Xue. Yu. Ji. Sheng. Chong. Bing. Za. Zhi. 30 (6), 423–427.
Lu, J., Holmgren, A. (2014). The Thioredoxin Antioxidant System. Free Radic. Biol. Med. 66, 75–87. doi: 10.1016/j.freeradbiomed.2013.07.036
Mahmood, D. F. D., Abderrazak, A., Couchie, D., Lunov, O., Diderot, V., Syrovets, T., et al. (2013). Truncated Thioredoxin (Trx-80) Promotes Pro-Inflammatory Macrophages of the M1 Phenotype and Enhances Atherosclerosis. J. Cell. Physiol. 228 (7), 1577–1583. doi: 10.1002/jcp.24319
Matthias, L. J., Yam, P. T. W., Jiang, X. M., Vandegraaff, N., Li, P., Poumbourios, P., et al. (2002). Disulfide Exchange in Domain 2 of CD4 is Required for Entry of HIV-I. Nat. Immunol. 3 (8), 727–732. doi: 10.1038/ni815
Peng, X. X., Zhang, M. Q. Z., Conserva, F., Hosny, G., Selivanova, G., Bykov, V. J. N., et al. (2017). APR-246/PRIMA-1(MET) Inhibits Thioredoxin Reductase 1 and Converts the Enzyme to a Dedicated NADPH Oxidase. Cell Death Dis. 8 (4), e2751. doi: 10.1038/cddis.2016.137
Petersen, T. N., Brunak, S., von Heijne, G., Nielsen, H. (2011). SignalP 4.0: Discriminating Signal Peptides From Transmembrane Regions. Nat. Methods 8 (10), 785–786. doi: 10.1038/nmeth.1701
Quick, R. E., Herwaldt, B. L., Thomford, J. W., Garnett, M. E., Eberhard, M. L., Wilson, M., et al. (1993). Babesiosis in Washington-State - a New Species of Babesia. Ann. Internal Med. 119 (4), 284–290. doi: 10.7326/0003-4819-119-4-199308150-00006
Rubartelli, A., Bajetto, A., Allavena, G., Wollman, E., Sitia, R. (1992). Secretion of Thioredoxin by Normal and Neoplastic-Cells Through a Leaderless Secretory Pathway. J. Biol. Chem. 267 (34), 24161–24164. doi: 10.1016/S0021-9258(18)35742-9
Ruijter, J. M., Ramakers, C., Hoogaars, W. M., Karlen, Y., Bakker, O., van den Hoff, M. J., et al. (2009) Amplification Efficiency: Linking Baseline and Bias in the Analysis of 597 Quantitative PCR Data. Nucleic Acids Res. 37 (6), e45. doi: 10.1093/nar/gkp045
Schetters, T. P., Strydom, T., Crafford, D., Kleuskens, J. A., Crommert, J. V. D., Vermeulen, A. N. (2007). Immunity Against Babesia Rossi Infection in Dogs Vaccinated With Antigens From Culture Supernatants. Vet. Parasitol. 144 (1-2), 10–19. doi: 10.1016/j.vetpar.2006.09.026
Schnittger, L., Rodriguez, A. E., Florin-Christensen, M., Morrison, D. A. (2012). Babesia: A World Emerging. Infect. Genet. Evol. 12 (8), 1788–1809. doi: 10.1016/j.meegid.2012.07.004
Schofield, L., Grau, G. E. (2005). Immunological Processes in Malaria Pathogenesis. Nat. Rev. Immunol. 5 (9), 14. doi: 10.1038/nri1686
Senanayake, S. N., Paparini, A., Latimer, M., Andriolo, K., Dasilva, A. J., Wilson, H., et al. (2012). First Report of Human Babesiosis in Australia. Med. J. Aust. 196 (5), 350–352. doi: 10.5694/mja11.11378
Smith, D. B., Johnson, K. S. (1988). Single-Step Purification of Polypeptides Expressed in Escherichia-Coli as Fusions With Glutathione S-Transferase. Gene 67 (1), 31–40. doi: 10.1016/0378-1119(88)90005-4
Stowell, C. P., Gelfand, J. A., Shepard, J. A., Kratz, A. (2007). Case Records of the Massachusetts General Hospital. Case 17-2007. A 25-Year-Old Woman With Relapsing Fevers and Recent Onset of Dyspnea. N. Engl. J. Med. 356, (22). doi: 10.1056/NEJMcpc079011
Tagaya, Y., Maeda, Y., Mitsui, A., Kondo, N., Matsui, H., Hamuro, J., et al. (1989). Atl-Derived Factor (Adf), an Il-2 Receptor Tac Inducer Homologous to Thioredoxin - Possible Involvement of Dithiol-Reduction in the Il-2 Receptor Induction. EMBO J. 8 (3), 757–764. doi: 10.1002/j.1460-2075.1989.tb03436.x
Tagaya, Y., Wakasugi, H., Masutani, H., Nakamura, H., Iwata, S., Mitsui, A., et al. (1990). Role of Atl-Derived Factor (Adf) in the Normal and Abnormal Cellular Activation - Involvement of Dithiol Related Reduction. Mol. Immunol. 27 (12), 1279–1289. doi: 10.1016/0161-5890(90)90032-u
Vannier, E., Gewurz, B. E., Krause, P. J. (2008). Human Babesiosis. Infect. Dis. Clinics North America 22 (3), 469–488. doi: 10.1016/j.idc.2008.03.010
Vannier, E., Krause, P. J. (2012). Human Babesiosis. New Engl. J. Med. 366 (25), 2397–2407. doi: 10.1056/NEJMra1202018
Vogt, A. M., Winter, G., Wahlgren, M., Spillmann, D. (2004). Heparan Sulphate Identified on Human Erythrocytes: A Plasmodium Falciparum Receptor. Biochem. J. 381, 593–597. doi: 10.1042/Bj20040762
Wakasugi, N., Tagaya, Y., Wakasugi, H., Mitsui, A., Maeda, M., Yodoi, J., et al. (1990). Adult T-Cell Leukemia-Derived Factor Thioredoxin, Produced by Both Human T-Lymphotropic Virus Type-I and Epstein-Barr Virus-Transformed Lymphocytes, Acts as an Autocrine Growth-Factor and Synergizes With Interleukin-1 and Interleukin-2. Proc. Natl. Acad. Sci. U. S. A. 87 (21), 8282–8286. doi: 10.1073/pnas.87.21.8282
Wang, W., Huang, P., Jiang, N., Lu, H. J., Zhang, D. C., Wang, D. W., et al. (2018). A Thioredoxin Homologous Protein of Plasmodium Falciparum Participates in Erythrocyte Invasion. Infect. Immun. 86 (8), e00289–e00218. doi: 10.1128/IAI.00289-18
Wang, H., Wang, Y., Huang, J., Xu, B., Chen, J., Dai, J., et al. (2020). Babesia Microti Protein BmSP44 Is a Novel Protective Antigen in a Mouse Model of Babesiosis. Front. Immunol. 7 (11). doi: 10.3389/fimmu.2020.01437
Wedmann, R., Onderka, C., Wei, S. W., Szijarto, I. A., Miljkovic, J. L., Mitrovic, A., et al. (2016). Improved Tag-Switch Method Reveals That Thioredoxin Acts as Depersulfidase and Controls the Intracellular Levels of Protein Persulfidation. Chem. Sci. 7 (5), 3414–3426. doi: 10.1039/c5sc04818d
Wormser, G. P., Prasad, A., Neuhaus, E., Joshi, S., Nowakowski, J., Nelson, J., et al. (2010). Emergence of Resistance to Azithromycin-Atovaquone in Immunocompromised Patients With Babesia Microti Infection. Clin. Infect. Dis. 50 (3), 381–386. doi: 10.1086/649859
Yang, D. H., Liu, X. H., Xu, W. T., Gu, Z. Y., Yang, C. T., Zhang, L. Z., et al. (2019). The Edwardsiella Piscicida Thioredoxin-Like Protein Inhibits ASK1-MAPKs Signaling Cascades to Promote Pathogenesis During Infection. PloS Pathog. 15 (7), e1007917. doi: 10.1371/journal.ppat.1007917
Yokoyama, N., Bork, S., Nishisaka, M., Hirata, H., Matsuo, T., Inoue, N., et al. (2003). Roles of the Maltese Cross Form in the Development of Parasitemia and Protection Against Babesia Microti Infection in Mice. Infect. Immun. 71 (1), 411–417. doi: 10.1128/Iai.71.1.411-417.2003
Yokoyama, N., Suthisak, B., Hirata, H., Matsuo, T., Inoue, N., Sugimoto, C., et al. (2002). Cellular Localization of Babesia Bovis Merozoite Rhoptry-Associated Protein 1 and Its Erythrocyte-Binding Activity. Infect. Immun. 70 (10), 5822–5826. doi: 10.1128/Iai.70.10.5822-5826.2002
Zhang, Y., Jiang, N., Lu, H. J., Hou, N., Piao, X. Y., Cai, P. F., et al. (2013). Proteomic Analysis of Plasmodium Falciparum Schizonts Reveals Heparin-Binding Merozoite Proteins. J. Proteome Res. 12 (5), 2185–2193. doi: 10.1021/pr400038j
Keywords: Babesia microti, immunoprotection, merozoite, pathogenicity, thioredoxin-like protein
Citation: Piao X, Ma Y, Liu S, Hou N and Chen Q (2022) A Novel Thioredoxin-Like Protein of Babesia microti Involved in Parasite Pathogenicity. Front. Cell. Infect. Microbiol. 12:826818. doi: 10.3389/fcimb.2022.826818
Received: 01 December 2021; Accepted: 26 January 2022;
Published: 17 February 2022.
Edited by:
Lan He, Huazhong Agricultural University, ChinaCopyright © 2022 Piao, Ma, Liu, Hou and Chen. This is an open-access article distributed under the terms of the Creative Commons Attribution License (CC BY). The use, distribution or reproduction in other forums is permitted, provided the original author(s) and the copyright owner(s) are credited and that the original publication in this journal is cited, in accordance with accepted academic practice. No use, distribution or reproduction is permitted which does not comply with these terms.
*Correspondence: Nan Hou, aG91bmFuQGlwYmNhbXMuYWMuY24=; Qijun Chen, cWlqdW5jaGVuNzU5QHN5YXUuZWR1LmNu
†These authors have contributed equally to this work and share first authorship
Disclaimer: All claims expressed in this article are solely those of the authors and do not necessarily represent those of their affiliated organizations, or those of the publisher, the editors and the reviewers. Any product that may be evaluated in this article or claim that may be made by its manufacturer is not guaranteed or endorsed by the publisher.
Research integrity at Frontiers
Learn more about the work of our research integrity team to safeguard the quality of each article we publish.