- 1Fisheries College, Key Laboratory of Healthy Mariculture for the East China Sea, Ministry of Agriculture, Jimei University, Xiamen, China
- 2College of Food and Biological Engineering, Jimei University, Xiamen, China
Pseudomonas plecoglossicida is an aerobic Gram-negative bacterium, which is the pathogen of “Visceral white spot disease” in large yellow croaker. P. plecoglossicida is a temperature-dependent bacterial pathogen in fish, which not only reduces the yield of large yellow croaker but also causes continuous transmission of the disease, seriously endangering the healthy development of fisheries. In this study, a mutant strain of fusA was constructed using homologous recombination technology. The results showed that knockout of P. plecoglossicida fusA significantly affected the ability of growth, adhesion, and biofilm formation. Temperature, pH, H2O2, heavy metals, and the iron-chelating agent were used to treat the wild type of P. plecoglossicida; the results showed that the expression of fusA was significantly reduced at 4°C, 12°C, and 37°C. The expression of fusA was significantly increased at pH 4 and 5. Cu2+ has a significant inducing effect on the expression of fusA, but Pb2+ has no obvious effect; the expression of fusA was significantly upregulated under different concentrations of H2O2. The expression of the fusA gene was significantly upregulated in the 0.5~4-μmol/l iron-chelating agent. The expression level of the fusA gene was significantly upregulated after the logarithmic phase. It was suggested that fusA included in the TBDR family not only was involved in the transport of ferredoxin but also played important roles in the pathogenicity and environment adaptation of P. plecoglossicida.
1 Introduction
Pseudomonas plecoglossicida was firstly isolated from ayu (Plecoglossus altivelis) (Nishimori et al., 2000), which was a gram-negative aerobic rod-shaped bacterium responsible for the bacterial hemorrhagic ascites (Wakabayashi et al., 1996; Kuehn and Kesty, 2005; Dumetz et al., 2007; Zhang et al., 2014). At present, P. plecoglossicida has been reported to be associated with diseases in a variety of marine fish such as rainbow trout (Oncorhynchus mykiss), large yellow croaker (Pseudosciaena crocea), and orange spotted grouper (Epinephelus coioides) (Lin et al., 2021). Previous studies have shown that P. plecoglossicida is used to degrade industrial waste that pollutes the environment. For example, P. plecoglossicida TED35 is used to degrade tobacco waste containing the alkaloid nicotine (Raman et al., 2014) and P. plecoglossicida NyZ12 uses cyclohexane (CHAM) as a source of carbon and nitrogen to degrade cyclohexane (Li et al., 2015). Because P. plecoglossicida mainly infects fish’s kidney, spleen, and other internal organs, with white nodules on the surface as the main symptom and a very high mortality rate, it is therefore called “Visceral white spot disease” (Tao et al., 2016). Artificial infection with P. plecoglossicida caused “Visceral white spot disease” in the internal organs of P. crocea and E. coioides at 16°C–19°C but not at 7°C–12°C and 24°C–28°C (Sun et al., 2018). With previous transcriptomic analysis, it was also confirmed that P. plecoglossicida was a temperature-dependent pathogenic bacteria (Zhang et al., 2018a; Jiao et al., 2021).
The large yellow croaker is mainly distributed in Southeast China. It is one of the most important economically maricultured fish species in China, and its yield has been the first in China for many years (Chen et al., 2008; Liu and de Mitcheson, 2008; Wu et al., 2015). In the process of sea cage culture of large yellow croaker, “Visceral white spot disease” caused by P. plecoglossicida is one of the diseases with the highest mortality, causing huge aquatic economic losses to southeast coastal areas such as Fujian and Zhejiang (, Watts et al., 2001; Zhang et al., 2007; Martins et al., 2011; Hu et al., 2014; Lin et al., 2021). Therefore, it is of great significance to study the virulence mechanism of P. plecoglossicida.
Iron is an indispensable nutrient element for all living organisms in the world, and competing for scarce nutrients is a “required course” for most microorganisms (Lim, 2010). In Gram-negative bacteria, the outer membrane receptors of the TonB-dependent receptor (TBDR) family perform their functions by binding microorganisms with high affinity to remove iron carriers and iron-containing host proteins such as lactoferrin, transferrin, and hemoglobin (Schaible and Kaufmann, 2004; Fujita et al., 2020). The transport from nucleus to periplasm also depends on TBDRs. TBDRs work with the nucleus through a highly specialized extracellular matrix structure, with an external loop of a 22-stranded transmembrane channel β-barrel. After these initial interactions, the pipeline provides a conduit through the outer membrane for iron or the iron carrier complex (Pollet et al., 2021). TBDRs are known to play an important role in the pathogenesis of host infection (Cornelissen et al., 1998; Noinaj et al., 2012; Ollerton et al., 2014). fusA is a newly discovered member of the TonB-dependent receptor family, which is involved in virulence regulation at the transcriptional level of some pathogenic bacteria. fusA was found to be used to obtain iron from plant ferridoxins in plant pathogenic Pectobacterium spp (Díaz-Sánchez et al., 2012). Violeta Díaz-Sánchez et al. found that the fusA gene was related to the pathogenic function of Fusarium fujikuroi. Nore´n found that the fusA gene was important for bacterial protein synthesis and was associated with drug resistance of Clostridium difficile (Noreín et al., 2007). In another study, the resistance of Corynebacterium glutamicum and Brevibacterium flavum to fusidic acid was related to fusA gene mutation, and all clones containing fusA gene mutation produced 10% more lysine than their parents (Tokmakova et al., 2017). In addition, through dual RNA-seq (Luo et al., 2019), we found that fusA might be a virulence gene that played an important role in the process of P. plecoglossicida infection, but the specific mechanism is unclear. Therefore, it is of far-reaching significance to explore the function of fusA and its possibility as a target for slowing down “visceral white spot disease” or becoming a live attenuated vaccine.
In order to better understand the function of fusA in P. plecoglossicida, we constructed the fusA knockout strain and investigated the effects of the fusA mutant on the ability of growth, adhesion, biofilm formation, and environment adaptation of P. plecoglossicida.
2 Materials and Methods
2.1 Bacterial Strains and Culture Conditions
The pathogenic strain isolated from the spleen of large yellow croaker with visceral white spot disease was later confirmed as P. plecoglossicida (NZBD9) (Huang et al., 2018; Zhong et al., 2021). The strain was cultured in LB medium at 220 rpm at 28°C (He et al., 2020). Escherichia coli DH5α was obtained from TransGen Biotech (Beijing, China) and cultured in LB medium (37°C, 220 rpm). DH5α pCM 130 and DH5α pKD 46 were preserved in the laboratory.
2.2 Sequence Alignment Analysis, Phylogenetic Tree Construction, and Protein Structure Prediction
The amino acid sequence of the fusA gene of P. plecoglossicida was compared with Escherichia coli str. K-12 substr. MG1655 (a), Staphylococcus argenteus (strain: MSHR1132) (b), Vibrio alginolyticus (strain: FDAARGOS_97) (c), Pseudomonas lactis (strain: SS101) (d), Listeria seeligeri serovar 1/2b str. SLCC3954 (e), Pseudomonas plecoglossicida (strain: NZBD9) (f), Pseudomonas syringae pv. tomato str. DC3000 (g), Vibrio harveyi (strain: ATCC 33843 (392 [MAV])) (h), Aeromonas hydrophila (strain: OnP3.1) (i), and Pseudomonas aeruginosa PA96 (j) by ClustalW and ENDscript2.x/ESPript3.x to map the sequence alignment results; the phylogenetic tree was constructed by MEGA7. The protein structure of FusA of P. plecoglossicida was predicted by I-TASSER.
2.3 Construction of Mutant Strain of P. plecoglossicida
The fusA knockout strain of P. plecoglossicida was constructed by using the red recombination system (Wang et al., 2017). According to the reference genomic sequence of P. plecoglossicida NZBD9, the upstream and downstream homologous sequences of fusA were searched, and each 20 bp before and after the gene was selected as the homologous fragments of primers, and the upstream and downstream primer sequences of the tetracycline resistance gene of plasmid pCM130 were amplified by fusion at the 3′ end of primers. After successful amplification, tetracycline-resistant gene fragments were recovered by 1% gel electrophoresis and DNA fragment recovery kit. The plasmid pKD46 was transformed into P. plecoglossicida NZBD9 by electroporation and cultured into OD600 = 0.2–0.3. The previously recovered fragment of the 10-μl resistance gene was transferred into P. plecoglossicida with pKD46 by electroporation, and L-arabinose of 10–30 mmol/l was added, so that the recombinant enzymes Exo, Bet, and Gam of pkD46 were fully expressed (Wang et al., 2017). The mutant bacteria were cultured overnight in LB plates (containing 100 μg/ml tetracycline) at 28°C. The positive colonies were validated by polymerase chain reaction (PCR) and gene sequencing.
2.4 Growth Curve of fusA Mutant
The P. plecoglossicida fusA knockout strain was cultured at 28°C (OD600 = 0.3). The wild-type strain of P. plecoglossicida was used as the control. OD600 were recorded once an hour for a total of 24 h, and growth curves of wild-type and mutant strains were compared. Three independent biological replications were performed for each data point.
2.5 Semisolid Agar Plate Motility Assay
The semisolid agar method was used to measure the motility of P. plecoglossicida (Zhang et al., 2018b). In short, the mutants and wild type of P. plecoglossicida were cultured overnight at 28°C (220 rpm) and adjusted to an OD600 of 0.3. Firstly, the sterilized toothpicks were immersed in the processed bacterial solution, and then immersed in the center of a semi-solid agar plate (LB broth + 0.5% agar), and finally incubated at 28°C for 20 h. The colony diameter was measured by the instrument, each in three independent biological replicates.
2.6 Biofilm Assay
The biofilm assay for P. plecoglossicida was carried out as described by Huang et al. (2021). Firstly, the mutants and wild-type strains of P. plecoglossicida were cultured overnight at 28°C, and then the OD600 of the culture was adjusted to 0.2. 150 μl LB was mixed with 50 μl bacterial culture medium and then incubated at 28°C for 24 h, washed with aseptic PBS for 3 times, stained with 200 μl 11% crystal violet for 15 min, then rinsed with aseptic PBS and air dried. The biofilm was dissolved with 200 μl of acetic acid (33%) and quantified by OD590. Six independent biological replications were performed for each group.
2.7 Adhesion Assay In Vitro
An adhesion assay of P. plecoglossicida was carried out in vitro by the microscope counting method (Rh et al., 2020). First, 20 μl of sterile large yellow croaker epidermis mucus was added to the center of clean glass slides, then it was spread evenly with a coverslip and incubated overnight, then fixed with 4% methanol solution at room temperature for 30 min and centrifuged to collect the mutants and wild strains of P. plecoglossicida cultured overnight, and finally resuspended in PBS. According to the value of OD560, the suspension was adjusted to 108 CFU/ml. 200 μl of bacterial solution was added to the slides and incubated at 28°C for 2 h, rinsed repeatedly with sterile PBS for 5 times, and then air dried, and fixed with 4% methanol solution at room temperature for 30 min. Finally, the slides were dyed with 1% crystal violet for 3 min and then observed with a microscope and imaged with a digital camera (magnification, ×1,000). The number of bacteria was quantified from the image using IPwin software (3 slides with 20 visual fields per slide) (Ryckaert et al., 2010; Isla et al., 2014; Zhang et al., 2018b; Huang et al., 2020a).
2.8 Hemolysis Assay
The hemolysis assay for P. plecoglossicida was carried out as described by Wei et al. (Tsou and Zhu, 2010; Xu et al., 2021). 100 μl of fresh sheep blood (Ping Rui Biotechnology Co., Ltd., Beijing, China) was centrifuged at 2,500 rpm for 5 min, and the supernatant was discarded. The red blood cells were then rinsed with 200 μl PBS three times and then resuspended with 100 μl PBS. The mixture of 5 μl of resuspended red blood cells with 245 μl of wild type or P. plecoglossicida ΔfusA culture was incubated at 37°C (150 rpm) for 1 h. Finally, the mixture was centrifuged at 5,000 rpm at room temperature for 3 min, and then 100 μl of the supernatant was used to determine the OD540.
2.9 Total RNA Extraction and Reverse Transcription
TRIzol reagent (Invitrogen, Carlsbad, CA, USA) was used for total RNA extraction from bacterial cells, as directed by the manufacturer. Reverse transcription was carried out with A Reverse Aid Mu-MLV cDNA synthesis kit (TransGen Biotech Co., Ltd., Beijing, China) from 2.0 mg total RNA, as instructed by the manufacturer (Ge et al., 2020; Huang et al., 2020a; Xu et al., 2021).
2.10 qRT-PCR
qRT-PCR adopts the SYBR Green method and refers to the TransStart Top Green qPCR SuperMix kit instructions. Table 1 shows all the sequences used in the experiment. The reaction system is 10 μl, containing 0.5 μl template, 0.25 μl forward primer (10 μM), 0.25 μl reverse primer (10 μM), and 9.5 μl 2× TransStart Top Green qPCR SuperMix, and then amplified and detected on the QuantStudio™ 6 Flex real-time fluorescent quantitative PCR system instrument. Finally, the 2-ΔΔCt calculation method was used to calculate the relative expression level of genes, while gene expression levels were normalized to 16S RNA (Feng et al., 2016; Ge et al., 2020).
2.11 Statistical Analysis
Data were presented as mean ± standard deviation (SD) and analyzed by SPSS 18.0 software (IBM, Armonk, NY, USA). Differences were compared by one-way analysis of variance (ANOVA) followed by the Dunnett’s test. p < 0.05 was considered statistically significant.
3 Results
3.1 Genetic Evolution and Protein Structure Analysis of fusA of P. plecoglossicida
Supplemental 1 shows the comparison result of the FusA amino acid sequences of P. plecoglossicida (NZBD9) and nine pathogenic bacteria. From the comparison results, it can be seen that the similarity between FusA amino acid sequences of P. plecoglossicida (NZBD9) (f) and P. aeruginosa PA96 (j) was relatively high. The phylogenetic tree analysis of P. plecoglossicida (NZBD9) and the other nine pathogenic bacteria fusA showed that P. plecoglossicida (NZBD9) and P. aeruginosa PA96 (j), P. syringae pv. tomato str. DC3000 (g), and P. lactis (strain: SS101) (d) had the closest genetic distance (Figure 1A). The protein structure was predicted by I-TASSER: among the five largest structural clusters, we selected the one with the highest confidence score, namely, C-score = 0.16 (Figure 1B). According to the highest TM score, the protein with the closest structural similarity to the predicted protein model in PDB was selected (PDB ID: 2XEX) (Figure 1C). The active site analysis also yielded the prediction result with the highest confidence (C-score = 0.60, Figure 1D). The closest P. plecoglossicida FusA protein structure model to the known protein structural model in PDB was Staphylococcus aureus. As we all know, proteins with high structural similarity often have similar function to the target. The predicted P. plecoglossicida FusA protein structure was biologically annotated by COFACTOR and COACH; the protein function and the conservation of the active site were inferred. It was found that the FusA protein function active site was highly conserved.
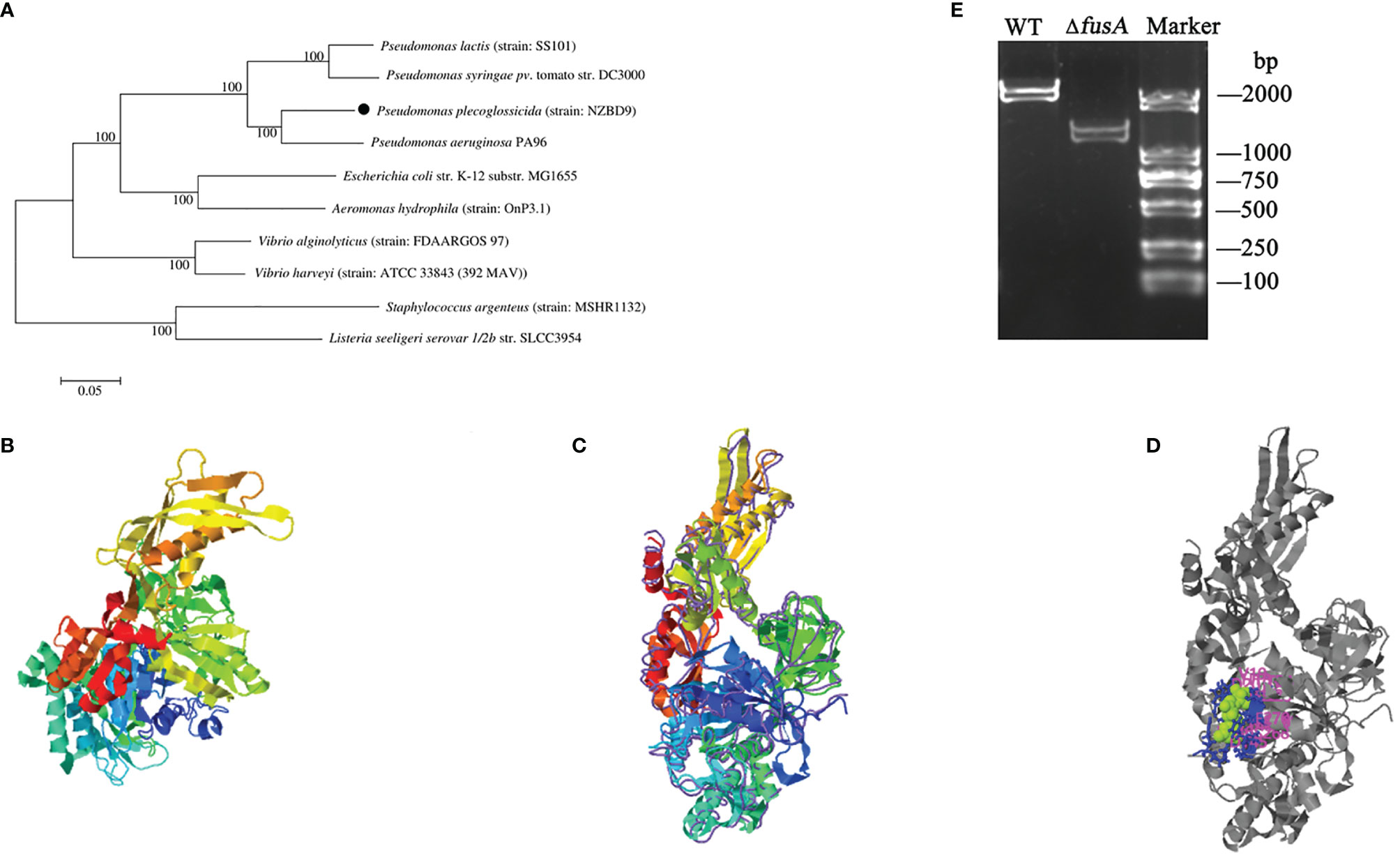
Figure 1 Phylogenetic tree and predicted protein structure of fusA of P. plecoglossicida and PCR identification of P. plecoglossicida ΔfusA. Note: (A) is the phylogenetic tree of fusA of P. plecoglossicida. MEGA7 is used to construct the adjacent tree. The self-development value is set to 1,000 times, and the self-development value ≥60% is displayed on the main nodes. The isolates in this experiment are represented by solid circle; (B) is the predicted P. plecoglossicida FusA protein structure model; (C) is the closest P. plecoglossicida FusA protein structure model to the known protein structural model in PDB; (D) is active sites of the P. plecoglossicida FusA protein structure model; (E) is the PCR identification of P. plecoglossicida ΔfusA (primers: ΔfusA-F and ΔfusA-R). Maker: DS2000 DNA marker; WT: PCR amplifications with wild-type genomic DNA (2,148 bp); ΔfusA: PCR amplifications with ΔfusA genomic DNA (1,204 bp).
3.2 Construction of the ΔfusA Mutant of P. plecoglossicida
The target fragment of fusA amplified by PCR was introduced into P. plecoglossicida NZBD9 by electrical transformation, and the fusA gene was knocked out by the λ-red recombination system. The result was confirmed by a PCR identification (Figure 1E) and DNA sequencing (data not shown), verifying that a knockout mutant of ΔfusA was successfully constructed.
3.3 The Effect of the fusA Gene on the Growth of P. plecoglossicida
In order to evaluate the difference of growth ability between ΔfusA and the wild type of P. plecoglossicida, the 24-h growth curves of wild-type P. plecoglossicida NZBD9 and ΔfusA at 28°C were compared. Through the 24-h growth curve test, the results showed that the growth curves of the two strains were significantly different, and the differences between sampling points were significant (p < 0.05) (Figure 2). The wild type and ΔfusA had no significant difference at the first 2-h adaptation period, but at the other similar time points, the OD600 of the ΔfusA growth curve was significantly lower than that of the wild type, indicating that the growth rate of the mutant strain was significantly lower than that of the wild strain. According to our results, the expression of fusA has a significant impact on the growth of P. plecoglossicida, especially in the middle to later stages of growth.
3.4 Effect of the fusA Gene on Motility of P. plecoglossicida
Flagella are a special structure of bacteria. Bacteria rely on the flagellum to achieve movement ability. The motility of bacteria has a direct impact on the chemotaxis of bacteria, which helps to move to a suitable environment and colonize the host. It is an important virulence factor of pathogenic bacteria. In order to detect whether the fusA gene affects the motility of P. plecoglossicida, the motility of the wild type and ΔfusA of P. plecoglossicida was tested. The results showed (Figure 3) that the colony diameters of the wild type and ΔfusA of P. plecoglossicida placed on semisolid agar plates for 20 h were about 8.119 ± 0.66 mm and 8.359 ± 0.49 mm. Statistical analysis showed that there was no significant difference between the two strains (p > 0.05). Therefore, it can be concluded that the fusA gene does not play a significant role in bacterial movement.
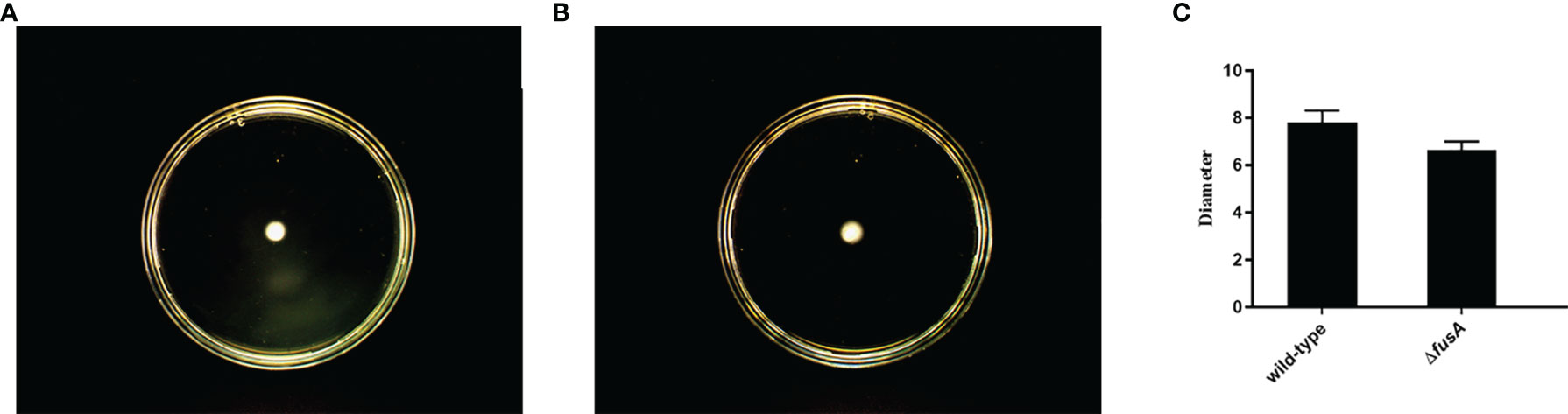
Figure 3 Determination of colony motility of wild-type and ΔfusA of P. plecoglossicida. (A) is the plate movement diagram of the wild-type of P. plecoglossicida; (B) is the plate movement diagram of the ΔfusA colony of P. plecoglossicida; (C) is the colony diameter histogram.
3.5 Effect of the fusA Gene on the Biofilm-Forming Ability of P. plecoglossicida
The bacteria with biofilm are highly resistant to antibiotics, and the biofilm can reinfect the host by releasing bacteria in the biofilm. Once the biofilm is formed, it is difficult to eradicate. In order to prove whether the fusA gene is related to biofilm formation, the biofilm formation of ΔfusA and the wild type of P. plecoglossicida was detected by OD590 nm (Figure 4). The results showed that the biofilm-forming ability of ΔfusA was lower than that of the wild type. It can be concluded that the fusA gene has a certain effect on the biofilm formation of P. plecoglossicida.
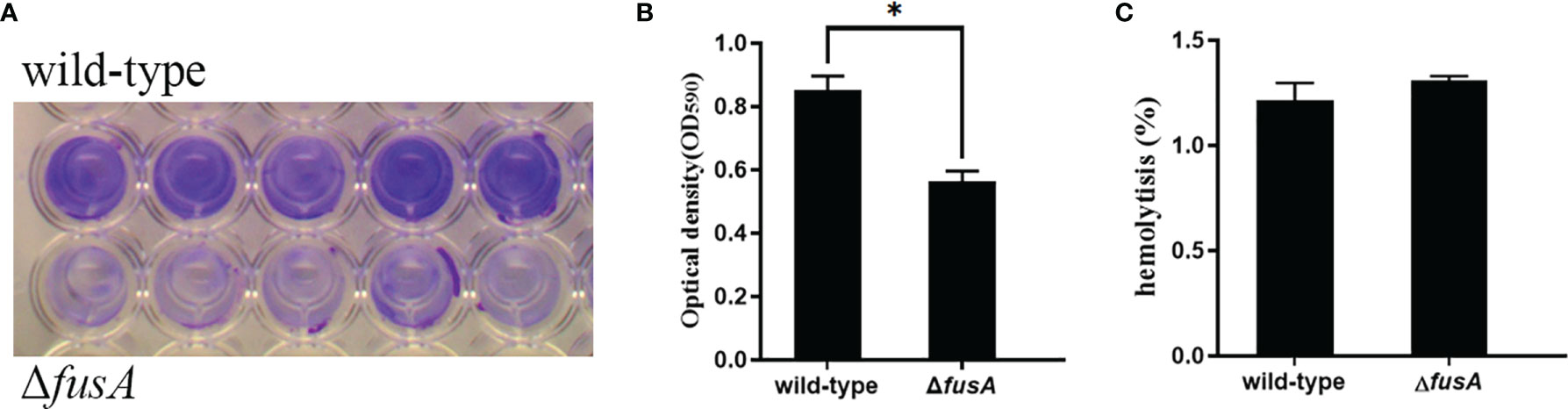
Figure 4 Determination of the biofilm formation and hemolytic ability of wild-type and ΔfusA of P. plecoglossicida. (A) is the typical figure of the biofilm formation of the wild type and ΔfusA; (B) is the biofilm formation histogram of the wild-type and ΔfusA; (C) is the hemolytic ability histogram of wild-type and ΔfusA; P < 0.05 is marked as *.
3.6 Effect of the fusA Gene on the Adhesion Ability of P. plecoglossicida
Adhesion is one of the important factors for pathogenic bacteria to invade the host. In order to verify whether the fusA gene was related to bacterial adhesion, the adhesion of the wild type and fusA mutant strains to the mucus was observed under the microscope, and the number of bacteria was quantified from the image with IPwin software (Figure 5). It could be seen from the figure that the adhesion ability of ΔfusA was significantly lower than that of the wild type, indicating that fusA was involved in the regulation of adhesion.
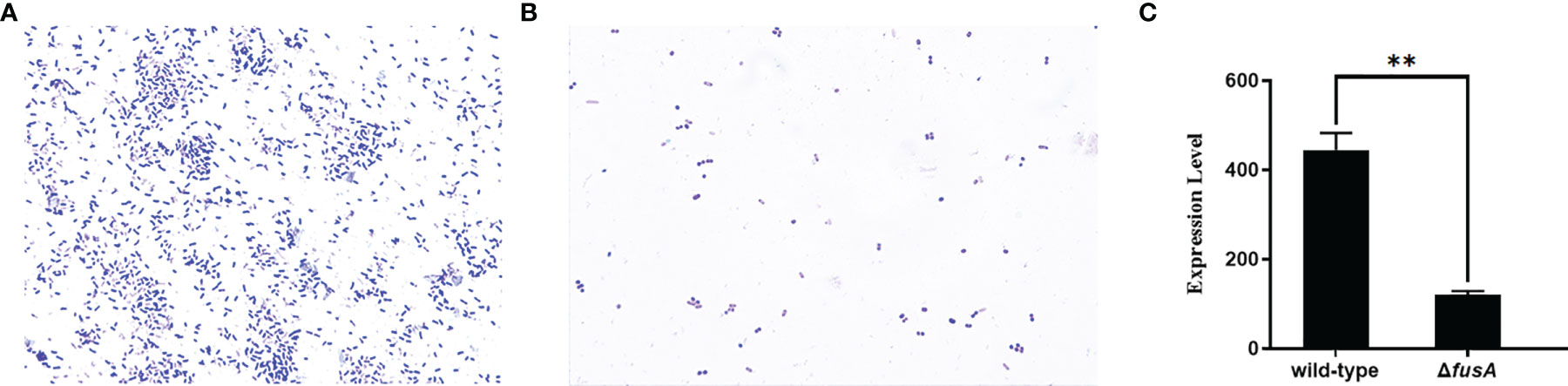
Figure 5 Determination of adhesion ability of wild type and ΔfusA of P. plecoglossicida. (A) is the typical vision of the adhesion of wild type under the optical microscope; (B) is the typical vision of the adhesion of ΔfusA under the optical microscope; (C) is the histogram of the number of bacteria in a field of the optical microscope; p < 0.01 is marked as **.
3.7 Effect of the fusA Gene on the Hemolytic Ability of P. plecoglossicida
Hemolysin is also one of the important virulence factors of bacteria. In order to verify whether the fusA gene was involved in the hemolysis mechanism of P. plecoglossicida, the hemolysis assay was carried out. The results showed (Figure 4C) that the hemolytic ability of ΔfusA was not significantly different from that of the wild type. This indicated that the fusA gene might not participate in the hemolysis process of P. plecoglossicida.
3.8 Verification of fusA Expression Under Different Stress Environments
3.8.1 The Expression Level of fusA at Different Temperatures
Temperature is one of the chief environmental factors that affect bacterial survival inside or outside their hosts, especially P. plecoglossicida which is a temperature-dependent pathogen. Therefore, qRT-RCR was used to detect the expression level of fusA at 4°C, 12°C, 18°C, 28°C, and 37°C (Figure 6A). The results showed that the expression level of fusA at 4°C, 12°C, and 37°C was significantly lower than that at 18°C and 28°C; the expression level of fusA at 18°C has no significant difference compared with that at 28°C. 18°C is the pathogenic temperature of P. plecoglossicida. It can be seen that fusA may be involved in the temperature-related virulence regulation of P. plecoglossicida.
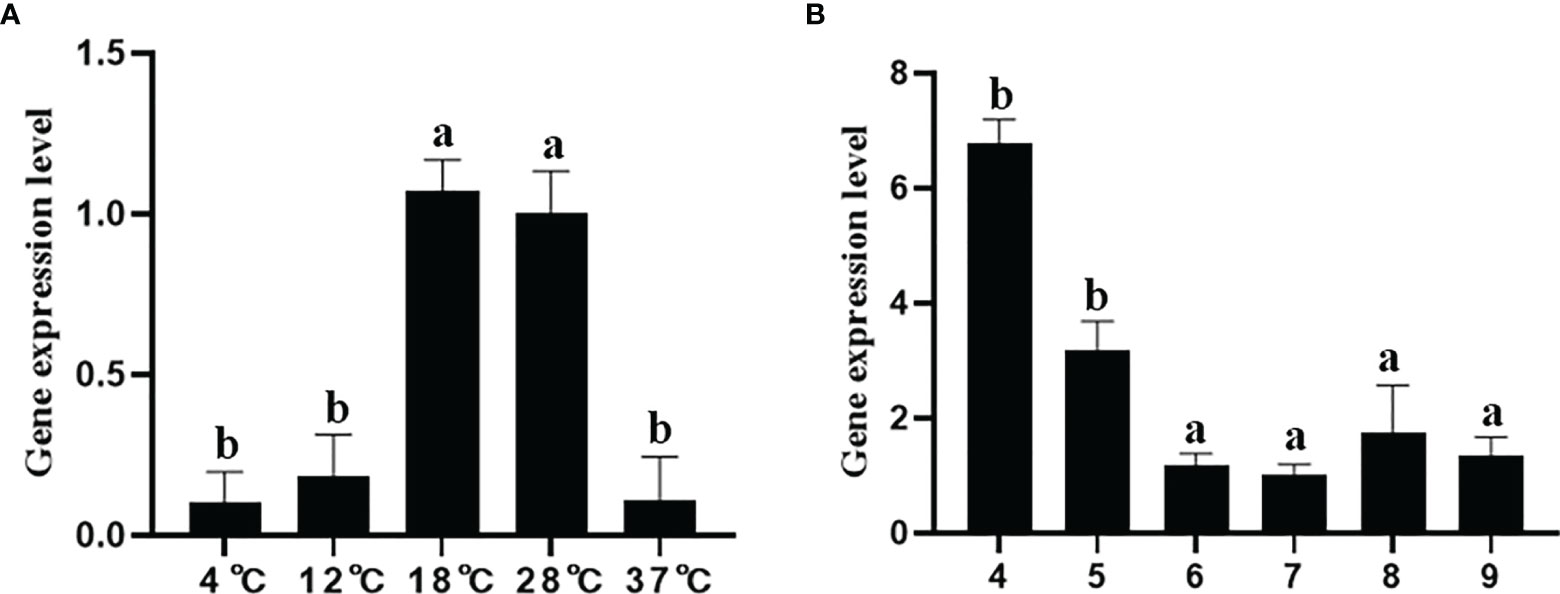
Figure 6 The expression level of the fusA gene under different temperature stresses (A) and different pH stresses (B). The means of the treatments not sharing a common letter are significantly different at p < 0.01.
3.8.2 The Expression Level of fusA at Different pH
pH is an important environmental factor that restricts the survival and growth of bacteria, which was usually used to defend the bacterial infection by hosts. Therefore, qRT-PCR was used to detect the expression of fusA at pH = 4, 5, 6, 7, 8, and 9. The results showed that (Figure 6B), compared with the pH = 7 group, the expression of fusA was significantly higher at pH = 4 and 5, and there was no significant difference between the other groups and the pH = 7 group.
3.8.3 The Expression Level of fusA Under Stresses of Heavy Metal Ion
qRT-PCR was used to detect the expression of fusA under Cu2+ and Pb2+ stress. The results show that (Figure 7A) compared with the control group, the expression of fusA increased significantly under Cu2+ stress, while under Pb2+ stress, there is no significant difference, which means that Cu2+ may have an inducing effect on the expression of fusA, while Pb2+ has no obvious effect.
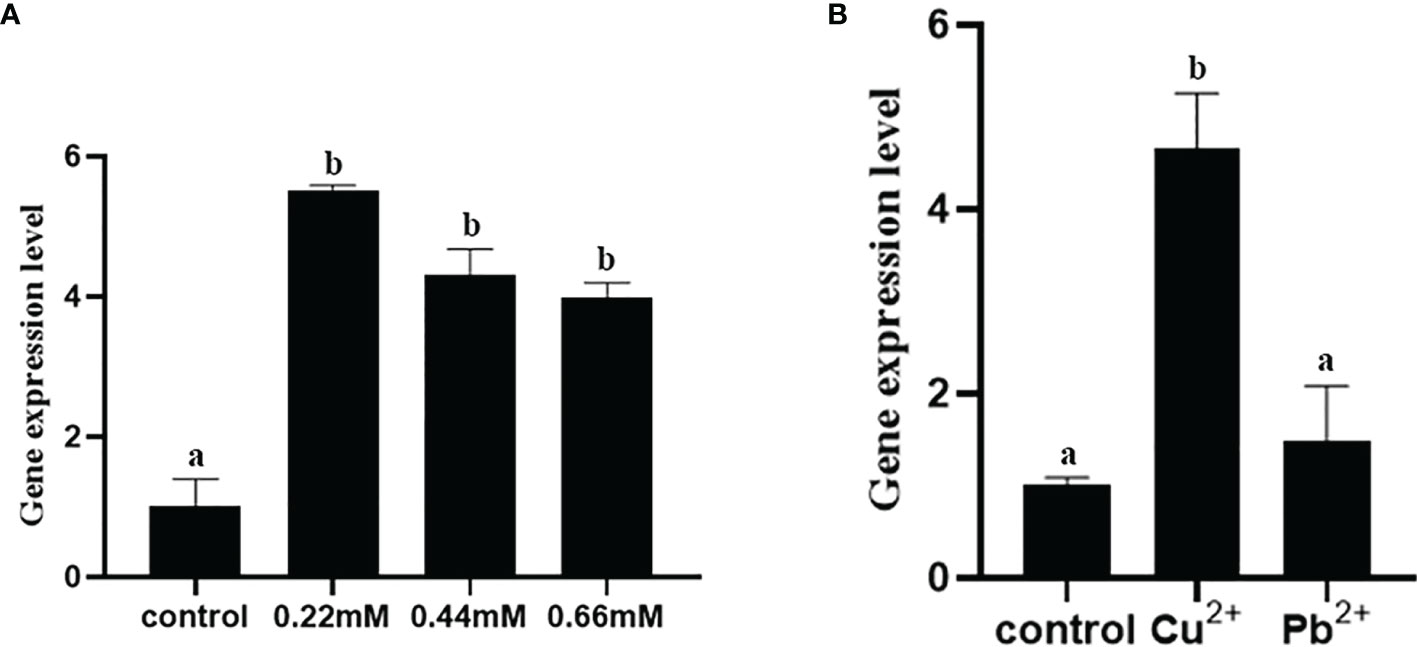
Figure 7 The expression level of fusA gene under heavy metal ion stress (A) and different concentrations of H2O2 (B). The means of the treatments not sharing a common letter are significantly different at p < 0.01.
3.8.4 The Expression Level of fusA Under Different Concentrations of H2O2
qRT-PCR was used to verify the expression of fusA at 0.22, 0.44, and 0.66 mmol/l H2O2. The results showed (Figure 7B) that the expression of fusA increased significantly under the stress of three H2O2 concentrations, but there was no significant difference in the expression of fusA among various concentrations. This indicated that H2O2 could promote the expression of fusA.
3.9 Expression Level of fusA in Iron-Poor Environment
As a member of the TBDR family, fusA is indispensable in the transport of ferredoxin. In order to verify the function of fusA in an iron-poor environment, growth assay and qRT-PCR were carried out. The results showed (Figure 8) that the growth of the ΔfusA strain in the iron-poor environment decreased significantly compared with the wild-type strain, indicating that the fusA gene might be involved in the utilization of Fe2+ by P. plecoglossicida. Meanwhile, compared with the control group, when the concentration of 2,2-bipyridine is 0.5, 1, 2, and 4 μmol/l, the fusA gene expression level was significantly upregulated, and its expression reaches the maximum value at 2 μmol/l.
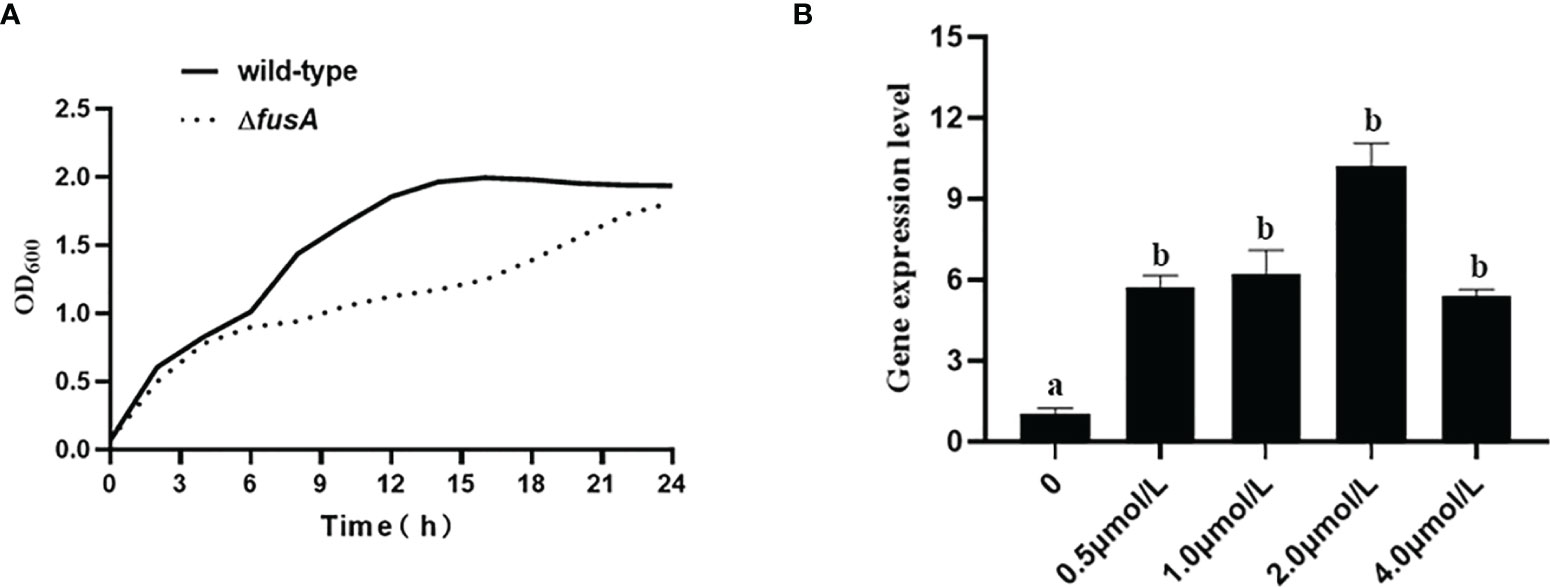
Figure 8 Determination the function of fusA in iron acquisition. (A) displays the growth ability of wild type and ΔfusA of P. plecoglossicida in the concentration of 2,2-bipyridine at 1 μmol/l; (B) is a histogram of fusA gene expression levels under the treatment of 2,2-bipyridine at 0.5, 1, 2, and 4 μmol/l, respectively. The means of the treatments not sharing a common letter are significantly different at p < 0.01.
4 Discussion
FusA amino acid sequence alignment and phylogenetic tree displayed that P. plecoglossicida has a high degree of homology with P. aeruginosa, which indicated the biological importance of FusA among Pseudomonas (Feng et al., 2016; Jones et al., 2017). Prediction of the P. plecoglossicida FusA protein structure model was carried out by I-TASSER (Yang and Zhang, 2015; Zhang et al., 2017; Zheng et al., 2021). The closest P. plecoglossicida FusA protein structure model to the known protein structural model in PDB was Staphylococcus aureus. As we all know, proteins with high structural similarity often have similar function to the target. The predicted P. plecoglossicida FusA protein structure was biologically annotated by COFACTOR and COACH; the protein function and the conservation of the active site were inferred. It was found that the FusA protein function active site was highly conserved.
All living organisms cannot do without trace metal nutrients, such as transition metals like iron (Fe), manganese (Mn), zinc (Zn), and molybdenum (Mo) (Radin et al., 2016; Radin et al., 2019a). According to proteomics or bioinformatics analysis, 30% of proteins in organisms need to interact with metal ions (Grim et al., 2017; Andreini et al., 2008; Waldron and Robinson, 2009; Waldron et al., 2009). During the infection, the pathogen’s nutrients all come from the host, and the host restricts the supply of necessary trace metals in order to resist the invasion of the pathogen (Weinberg, 2009; Kehl-Fie and Skaar, 2010), so that the pathogen is in a metal starvation state, that is, nutritional immunity (Corbin et al., 2008; Kehl-Fie and Skaar, 2010; Hood and Skaar, 2012; Huang et al., 2021). The most characteristic inhibitory response of nutritional immunity is iron (Schaible and Kaufmann, 2004; Hood and Skaar, 2012; Párraga Solórzano et al., 2019; Radin et al., 2019b).
For all living organisms, iron is an important element. Bacteria have also evolved ways to obtain iron. TBDRs are an active transporter on the outer membrane of Gram-negative bacteria (Lim, 2010; Noinaj et al., 2010; Fujita et al., 2019), which use the energy produced by the inner membrane to transport nutrients (such as sugars, etc.) through the TonB/ExbBD complex (Saier, 2000; Nikaido, 2003; Braun, 2006; Schauer et al., 2008; Jordan et al., 2013; Modrak et al., 2018). fusA is a newly discovered member of the TBDR family, which is also involved in iron transport. With the continuous study of TBDRs, it has been found that TBDR is also involved in host infection. Many laboratories have previously reported that various factors in the TBDR family are involved in virulence regulations in different bacteria. For example, in Flavobacterium psychrophilum, the exbD loci of a TonB system are required for optimal bacterial virulence (Álvarez et al., 2008), and tdrA in TBDRs is also an indispensable virulence factor in the pathogenicity of Pseudomonas fluorescens (Hu et al., 2012). According to our genomic analysis, the fusA gene existed in the P. plecoglossicida genome. In the previous dual RNA-seq of our laboratory, the fusA gene was significantly highly expressed, indicating that it may be an important virulence gene of P. plecoglossicida. In view of the importance of fusA in other bacterial pathogens, there is no indication that fusA is involved in the virulence regulation of P. plecoglossicida previously reported, and it is also not clear which pathogenic factors this gene is related to in P. plecoglossicida, so we investigated the relationship between fusA and the pathogenicity of P. plecoglossicida. In the present study, the P. plecoglossicida fusA gene knockout strain was successfully constructed, and the virulence phenotype of the mutant strain was determined.
Mucus is abundant on the surface of fish skin, gill, and intestinal wall, which provides a good adhesion environment for bacteria. Therefore, it is the first site where pathogen and host are most likely to interact (Liu et al., 2020). After the bacteria adhere to the host, they may begin to invade, and in the process, the bacteria will try to protect themselves from the host’s immune system. For example, the formation of biofilm is one of the measures to resist host immune attack (Qin et al., 2016). The results showed that there were significant differences in adhesion and bacterial growth rate between the wild type and ΔfusA of P. plecoglossicida. The absence of the fusA gene will weaken the adhesion of bacteria and the ability of tissue transmission and colonization. The TBDR family is known to be important in bacterial uptake of trace iron (Wang et al., 2016), and fusA is a member of TBDRs. In the iron-poor environment, the fusA expression level was dramatically upregulated, indicating that the fusA gene played an important role in the competition of P. plecoglossicida for the trace iron-related virulence mechanism in harsh environments. Flagella are not only a motility organ of bacteria but also one of the parameters for evaluating the virulence factors of pathogenic bacteria (Nakamura and Minamino, 2019). Relevant studies have shown that flagella are not only involved in bacterial motility but also involved in bacterial adhesion, biofilm formation, and special channel transport of virulence proteins and other pathways, which indirectly affect bacterial virulence (Feldman et al., 1998; Blocker et al., 2003; Roy et al., 2009; Duan et al., 2013; Duan et al., 2013). Although the motility and hemolytic ability of the P. plecoglossicida ΔfusA were not different from the wild type, the biofilm formation ability and adhesion ability of ΔfusA were significantly reduced. Taken together, fusA was obviously involved in the virulence regulation of P. plecoglossicida.
Bacterial virulence is usually affected by environmental factors. For example, the pathogenic temperature of “visceral white spot disease” caused by P. plecoglossicida in large yellow croaker and other commercial fish is 15°C–20°C (Zhang et al., 2018a; Kaur et al., 2019; Lv et al., 2019; Huang et al., 2020a; Hu et al., 2021; Jin et al., 2021). The results of various stress experiments showed that compared with 18°C, the expression level of fusA was significantly downregulated at 4°C, 12°C, and 37°C. This indicated that fusA may be involved in the temperature-related virulence regulation of P. plecoglossicida. Besides, the expression level was significantly upregulated under acidic conditions as pH = 4 or 5, which indicated that fusA was involved in the response to low pH. Meanwhile, the significantly high expression of fusA under Cu2+ exposure and H2O2 conditions also further indicated that fusA was important for P. plecoglossicida survival under severe conditions.
In conclusion, through fusA mutant strain construction, we found that the fusA gene was involved in a variety of pathogenic and environmental adaptation mechanisms of P. plecoglossicida. At the same time, whether fusA existed or not could promote P. plecoglossicida to compete for iron in the environment. These results further confirmed the importance of P. plecoglossicida fusA and laid a foundation for further study on the function of the gene.
Data Availability Statement
The original contributions presented in the study are included in the article/Supplementary Material. Further inquiries can be directed to the corresponding authors.
Author Contributions
All authors contributed to the article and approved the submitted version. QY and LH conceived the experiments. RH, JW, ML, JT, BW, XT, JZhou, and JZhang conducted the experiments. All authors assisted in the collection and interpretation of data. LH and QY wrote the manuscript. All authors contributed to the article and approved the submitted version.
Funding
This work was supported by grants from the major program of Science and Technology Planning of Xiamen under Contract No. 3502Z20211004 and the Fujian Engineering Research Center of Aquatic Breeding and Healthy Aquaculture, under Contract No. DF201903.
Conflict of Interest
The authors declare that the research was conducted in the absence of any commercial or financial relationships that could be construed as a potential conflict of interest.
Publisher’s Note
All claims expressed in this article are solely those of the authors and do not necessarily represent those of their affiliated organizations, or those of the publisher, the editors and the reviewers. Any product that may be evaluated in this article, or claim that may be made by its manufacturer, is not guaranteed or endorsed by the publisher.
Supplementary Material
The Supplementary Material for this article can be found online at: https://www.frontiersin.org/articles/10.3389/fcimb.2022.808800/full#supplementary-material
Supplemental Figure 1 | The comparison result of the fusA amino acid sequences of P. plecoglossicida (NZBD9) and nine pathogenic bacteria. Note: The dark background sequence indicates the same fusA amino acid sequence of P. plecoglossicida NZBD9 as the other nine pathogenic bacteria.
References
Álvarez, B., Álvarez, J., Menéndez, A., Guijarro, J. A. (2008). A Mutant in One of Two exbD Loci of a TonB System in Flavobacterium Psychrophilum Shows Attenuated Virulence and Confers Protection Against Cold Water Disease. Microbiology 154 (4), 1144–1151. doi: 10.1099/mic.0.2007/010900-0
Andreini, C., Bertini, I., Cavallaro, G., Thornton, H. (2008). Metal Ions in Biological Catalysis: From Enzyme Databases to General Principles. JBIC J. Biol. Inorg. Chem. 13 (8), 1205–1218. doi: 10.1007/s00775-008-0404-5
Blocker, A., Komoriya, K., Aizawa, S. I. (2003). Type III Secretion Systems and Bacterial Flagella: Insights Into Their Function From Structural Similarities. Proc. Natl. Acad. Sci. 100 (6), 3027–3030. doi: 10.1073/pnas.0535335100
Braun, V. (2006). Energy Transfer Between Biological Membranes. ACS Chem. Biol. 1 (6), 352–354. doi: 10.1021/cb600256k
Chen, Q., Yan, Q., Wang, K., Zhuang, Z., Wang, X., et al. (2008). Portal of Entry for Pathogenic Vibrio Alginolyticus Into Large Yellow Croaker Pseudosciaena Crocea, and Characteristics of Bacterial Adhesion to Mucus. Dis. Aquat. Org. 80, 181–188. doi: 10.3354/dao01933
Corbin, B. D., Seeley, E. H., Raab, A., Feldmann, J., Miller, M. R., Torres, V. J., et al. (2008). Metal Chelation and Inhibition of Bacterial Growth in Tissue Abscesses. Science 319 (5865), 962–965. doi: 10.1126/science.1152449
Cornelissen, C. N., Kelley, M., Hobbs, M. M., Anderson, J. E., Sparling, P. F. (1998). The Transferrin Receptor Expressed by Gonococcal Strain FA1090 Is Required for the Experimental Infection of Human Male Volunteers. Mol. Microbiol. 27 (3), 611–616. doi: 10.1046/j.1365-2958.1998.00710.x
Díaz-Sánchez, V., Avalos, J., Limón, M. C. (2012). Identification and Regulation of Fusa, the Polyketide Synthase Gene Responsible for Fusarin Production in Fusarium Fujikuroi. Appl. Environ. Microbiol. 78 (20), 7258–7266. doi: 10.1128/AEM.01552-12
Duan, Q., Zhou, M., Zhu, L., Zhu, G. (2013). Flagella and Bacterial Pathogenicity. J. Basic Microbiol. 53 (1), 1–8. doi: 10.1002/jobm.201100335
Dumetz, F., Lapatra, S. E., Duchaud, E., Claverol, S., Henaff, M. L. (2007). The Flavobacterium Psychrophilum OmpA, an Outer Membrane Glycoprotein, Induces a Humoral Response in Rainbow Trout. J. Appl. Microbiol. 103 (5), 1461–1470. doi: 10.1111/j.1365-2672.2007.03359.x
Feldman, M., Bryan, R., Rajan, S., Scheffler, L., Prince, A. (1998). Role of Flagella in Pathogenesis of Pseudomonas Aeruginosa Pulmonary Infection. Infect. Immun. 66 (1), 43–51. doi: 10.1128/IAI.66.1.43-51.1998
Feng, Y., Jonker, M. J., Moustakas, I., Bruls, S., Terkuile, B. H. (2016). Dynamics of Mutations During Development of Resistance by Pseudomonas Aeruginosa Against Five Antibiotics. Antimicrob. Agents Chemother. 60 (7), 4229–4236. doi: 10.1128/AAC.00434-16
Fujita, M., Mori, K., Hara, H., Hishiyama, S., Kamimura, N., Masai, E. A. (2019). A TonB-Dependent Receptor Constitutes the Outer Membrane Transport System for a Lignin-Derived Aromatic Compound. Commun. Biol. 2 (1), 432. doi: 10.1038/s42003-019-0676-z
Fujita, M., Sakumoto, T., Tanatani, K., Yu, H. Y., Masai, E. (2020). Iron Acquisition System of Sphingobium Sp. Strain SYK-6, a Degrader of Lignin-Derived Aromatic Compounds. Sci. Rep. 10 (1). 12177. doi: 10.1038/s41598-020-68984-2.
Ge, X. A., Fan, W. B., Lz, A., Yq, A., Lh, A., Qy, A. (2020). Integration of RNA-Seq and RNAi Provides a Novel Insight Into the Effect of pvdE Gene to the Pathogenic of Pseudomonas Plecoglossicida and on the Immune Responses of Orange-Spotted Grouper (Epinephelus Coioides). Aquaculture 529. doi: 10.1016/j.aquaculture.2020.735695
Grim, K. P., San Francisco, B., Radin, J. N., Brazel, E. B., Kelliher, J. L., Parraga Solorzano, P. K., et al. (2017). The Metallophore Staphylopine Enables Staphylococcus aureus To Compete with the Host for Zinc and Overcome Nutritional Immunity. mBio. 8 (5), e01281–e01217. doi: 10.1128/mBio.01281-17
He, R., Zhao, L., Xu, X., Zheng, W., Zhang, J., Zhang, J., et al. (2020). Aryl Hydrocarbon Receptor is Required for Immune Response in Epinephelus Coioides and Danio Rerio Infected by Pseudomonas Plecoglossicida. Fish Shellfish Immunol. 97, 564–570. doi: 10.1016/j.fsi.2019.12.084
Hood, M. I., Skaar, E. P. (2012). Nutritional Immunity: Transition Metals at the Pathogen–Host Interface. Nat. Rev. Microbiol. 10 (8), 525–537. doi: 10.1038/nrmicro2836
Huang, L., Liu, W., Jiang, Q., Zuo, Y., Yan, Q. (2018). Integration of Transcriptomic and Proteomic Approaches Reveals the Temperature-Dependent Virulence of Pseudomonas Plecoglossicida. Front. Cell. Infect. Microbiol. 8. doi: 10.3389/fcimb.2018.00207
Huang, L., Qiao, Y., Xu, W., Gong, L., He, R., Qi, W., et al. (2021). Full-Length Transcriptome: A Reliable Alternative for Single-Cell RNA-Seq Analysis in the Spleen of Teleost Without Reference Genome. Front. Immunol. 12, 737332. doi: 10.3389/fimmu.2021.737332
Huang, L., Qi, W., Zuo, Y., Alias, S. A., Xu, W. (2020a). The Immune Response of a Warm Water Fish Orange-Spotted Grouper (Epinephelus Coioides) Infected With a Typical Cold Water Bacterial Pathogen Aeromonas Salmonicida is AhR Dependent. Dev. Comp. Immunol. 113, 103779. doi: 10.1016/j.dci.2020.103779
Huang, L., Zhao, L., Qi, W., Xu, X., Yan, Q. (2020a). Temperature-Specific Expression of Cspa1 Contributes to Activation of sigX During Pathogenesis and Intracellular Survival in Pseudomonas Plecoglossicida. Aquaculture 518, 734861. doi: 10.1016/j.aquaculture.2019.734861
Huang, L., Zuo, Y., Qin, Y., Zhao, L., Lin, M., Yan, Q. (2021). The Zinc Nutritional Immunity of Epinephelus Coioides Contributes to the Importance of znuC During Pseudomonas Plecoglossicida Infection. Front. Immunol. 12, 678699. doi: 10.3389/fimmu.2021.678699
Hu, Y., Dang, W., Sun, L. (2012). A TonB-Dependent Outer Membrane Receptor of Pseudomonas Fluorescens: Virulence and Vaccine Potential. Arch. Microbiol. 194 (9), 795–802. doi: 10.1007/s00203-012-0812-3
Hu, J., Zhang, F., Xu, X., Su, Y. Q., Qin, Y. X., Ma, Y., et al. (2014). Isolation, Identification And Virulence Of The Pathogen Of White-Spots Disease In Internal Organs Of Pseudosciaena Crocea. Oceanol. Limnol. Sin. 2, 409–417.
Hu, L., Zhao, L., Zhuang, Z., Wang, X., Fu, Q., Huang, H., et al. (2021). The Effect of tonB Gene on the Virulence of Pseudomonas Plecoglossicida and the Immune Response of Epinephelus Coioides. Front. Microbiol. 12. doi: 10.3389/fmicb.2021.720967
Isla, A., Haussmann, D., Vera, T., Kausel, G., Figueroa, J. (2014). Identification of the clpB and bipA Genes and an Evaluation of Their Expression as Related to Intracellular Survival for the Bacterial Pathogen Piscirickettsia Salmonis. Veterinary. Microbiol. 173 (3-4), 390–394. doi: 10.1016/j.vetmic.2014.08.014
Jiao, J., Zhao, L., Huang, L., Qin, Y., Su, Y., Zheng, W., et al. (2021). The Contributions of fliG Gene to the Pathogenicity of Pseudomonas Plecoglossicida and Pathogen-Host Interactions With Epinephelus Coioides. Fish Shellfish Immunol. 119, 238–248. doi: 10.1016/j.fsi.2021.09.032
Jin, J., Li, Y., Huang, M., Li, S., Mao, Z. (2021). Preliminary Studies on the Different Roles of T6SSs in Pathogenicity of Pseudomonas Plecoglossicida NB2011. J. Fish Dis. 44 (11), 1669–1679. doi: 10.1111/jfd.13479
Jones, A. K., Woods, A. L., Takeoka, K. T., Shen, X., Wei, J. R., Caughlan, R. E., et al. (2017). Determinants of Antibacterial Spectrum and Resistance Potential of the Elongation Factor G Inhibitor Argyrin B in Key Gram-Negative Pathogens. Antimicrob. Agents Chemother. 61 (4), AAC.02400-16. doi: 10.1128/AAC.02400-16
Jordan, L. D., Zhou, Y., Smallwood, C. R., Lily, Y., Ritchie, K., Yip, W. T., et al. (2013). Energy-Dependent Motion of TonB in the Gram-Negative Bacterial Inner Membrane. Proc. Natl. Acad. Sci. 110 (28), 11553–11558. doi: 10.1073/pnas.1304243110
Kaur, K., Sharma, A., Capalash, N., Sharma, P. (2019). Multicopper Oxidases: Biocatalysts in Microbial Pathogenesis and Stress Management. Microbiol. Res. 222, 1–13. doi: 10.1016/j.micres.2019.02.007
Kehl-Fie, T. E., Skaar, E. P. (2010). Nutritional Immunity Beyond Iron: A Role for Manganese and Zinc. Curr. Opin. Chem. Biol. 14 (2), 218–224. doi: 10.1016/j.cbpa.2009.11.008
Kuehn, M. J., Kesty, N. C. (2005). Bacterial Outer Membrane Vesicles and the Host-Pathogen Interaction. Genes Dev. 19 (22), 2645–2655. doi: 10.1101/gad.1299905
Li, X., Li, C., Mao, L. Q., Yan, D. Z., Zhou, N. Y. (2015). Complete Genome Sequence of the Cyclohexylamine-Degrading Pseudomonas Plecoglossicida Nyz12. J. Biotechnol. 199, 29–30. doi: 10.1016/j.jbiotec.2015.02.011
Lim, B. L. (2010). TonB-Dependent Receptors in Nitrogen-Fixing Nodulating Bacteria. Microbes Environ. 25 (2), 67–74. doi: 10.1264/jsme2.ME10102
Lin, H., Zhou, Z., Zhao, J., Zhou, T., Bai, H., Ke, Q., et al. (2021). Genome-Wide Association Study Identifies Genomic Loci of Sex Determination and Gonadosomatic Index Traits in Large Yellow Croaker (Larimichthys Crocea). Mar. Biotechnol. 23 (1), 127–139. doi: 10.1007/s10126-020-10007-2
Liu, M., de Mitcheson, Y. S. (2008). Profile of a Fishery Collapse: Why Mariculture Failed to Save the Large Yellow Croaker. Fish Fish. 9 (3), 219–242. doi: 10.1111/j.1467-2979.2008.00278.x
Liu, Z., Zhao, L., Huang, L., Qin, Y., Zhang, J., Zhang, J., et al. (2020). Integration of RNA-Seq and RNAi Provides a Novel Insight Into the Immune Responses of Epinephelus Coioides to the impB Gene of Pseudomonas Plecoglossicida. Fish Shellfish Immunol. 105, 135–143. doi: 10.1016/j.fsi.2020.06.023
Luo, G., Sun, Y., Huang, L., Su, Y., Zhao, L., Qin, Y., et al. (2019). Time-Resolved Dual RNA-Seq of Tissue Uncovers Pseudomonas Plecoglossicida Key Virulence Genes in Host-Pathogen Interaction With Epinephelus Coioides. Environ. Microbiol. 22 (2), 677–693. doi: 10.1111/1462-2920.14884.
Lv, T., Dai, F., Zhuang, Q., Zhao, X., Shao, Y., Guo, M., et al. (2019). Outer Membrane Protein OmpU Is Related to Iron Balance in Vibrio Alginolyticus. Microbiol. Res. 230, 126350. doi: 10.1016/j.micres.2019.126350
Martins, M. L., Xu, D. H., Shoemaker, C. A., Klesius, P. H. (2011). Temperature Effects on Immune Response and Hematological Parameters of Channel Catfish Ictalurus Punctatus Vaccinated With Live Theronts of Ichthyophthirius Multifiliis. Fish Shellfish Immunol. 31 (6), 774–780. doi: 10.1016/j.fsi.2011.07.015
Modrak, S. K., Melin, M. E., Bowers, L. M. (2018). SucA-Dependent Uptake of Sucrose Across the Outer Membrane of Caulobacter Crescentus. J. Microbiol. 56 (9), 648–655. doi: 10.1007/s12275-018-8225-x
Nakamura, S., Minamino, T. (2019). Flagella-Driven Motility of Bacteria. Biomolecules 9 (7), 279. doi: 10.3390/biom9070279
Nikaido, H. (2003). Molecular Basis of Bacterial Outer Membrane Permeability Revisited Mol Biol Rev. 67 (4), 593–656. doi: 10.1128/MMBR.67.4.593-656.2003
Nishimori, E., Kita-Tsukamoto, K., Wakabayashi, H. (2000). Pseudomonas Plecoglossicida Sp. Nov., the Causative Agent of Bacterial Haemorrhagic Ascites of Ayu, Plecoglossus Altivelis. Int. J. Syst. Evol. Microbiol. 50 Pt 1, 83–89. doi: 10.1099/00207713-50-1-83
Noinaj, N., Easley, N. C., Oke, M., Mizuno, N., Gumbart, J., Boura, E., et al. (2012). Structural Basis for Iron Piracy by Pathogenic Neisseria. Nature 483 (7387), 53–58. doi: 10.1038/nature10823
Noinaj, N., Guillier, M., Barnard, T. J., Buchanan, S. K. (2010). TonB-Dependent Transporters: Regulation, Structure, and Function. Annu. Rev. Microbiol. 64 (1), 43–60. doi: 10.1146/annurev.micro.112408.134247
Noreín, T., A°kerlund, T., Wullt, M., Burman, L. G., Unemo, M. (2007). Mutations in fusA Associated With Posttherapy Fusidic Acid Resistance in Clostridium Difficile. Antimicrobial. Agents Chemother. 51 (5), 1840–1843. doi: 10.1128/AAC.01283-06
Ollerton, J., Erenler, H., Edwards, M., Crockett, R. (2014). Extinctions of Aculeate Pollinators in Britain and the Role of Large-Scale Agricultural Changes. Science 346 (6215), 1360–1362 . doi: 10.1126/science.1257259
Párraga Solórzano, P. K., Yao, J., Rock, C. O., Kehl-Fie, T. E. (2019). Disruption of Glycolysis by Nutritional Immunity Activates a Two-Component System That Coordinates a Metabolic and Antihost Response by Staphylococcus Aureus. mBio 10 (4), e1319–e1321. doi:10.1128/mBio.01321-19
Pollet, R. M., Martin, L. M., Koropatkin, N. M. (2021). TonB-Dependent Transporters in the Bacteroidetes: Unique Domain Structures and Potential Functions. Mol. Microbiol. 115 (3), 490–501. doi: 10.1111/mmi.14683
Qin, Y., Lin, G., Chen, W., Xu, X., Yan, Q. (2016). Flagellar Motility Is Necessary for Aeromonas Hydrophila Adhesion. Microbial. Pathogenesis 98, 160–166. doi: 10.1016/j.micpath.2016.07.006
Radin, J. N., Kelliher, J. L., Párraga Solórzano, P. K., Kehl-Fie, T. E. (2016). The Two-Component System ArlRS and Alterations in Metabolism Enable Staphylococcus Aureus to Resist Calprotectin-Induced Manganese Starvation. PloS Pathog. 12 (11), e1006040. doi: 10.1371/journal.ppat.1006040
Radin, J. N., Kelliher, J. L., Solórzano, P. K. P., Grim, K. P., Ramezanifard, R., Slauch, J. M., et al. (2019b). Metal-Independent Variants of Phosphoglycerate Mutase Promote Resistance to Nutritional Immunity and Retention of Glycolysis During Infection. PloS Pathog. 15 (7), e1007971. doi: 10.1371/journal.ppat.1007971
Radin, J. N., Zhu, J., Brazel, E. B., Kehl-Fie, T. E. (2019a). Synergy Between Nutritional Immunity and Independent Host Defenses Contributes to the Importance of the MntABC Manganese Transporter Duringstaphylococcus Aureus Infection. Infect. Immun. 87 (1). doi: 10.1128/IAI.00642-18
Raman, G., Mohan, K., Manohar, V., Sakthivel, N. (2014). Biodegradation of Nicotine by a Novel Nicotine-Degrading Bacterium, Pseudomonas Plecoglossicida TND35 and Its New Biotransformation Intermediates. Biodegradation 25 (1), 95–107. doi: 10.1007/s10532-013-9643-4
Rh, A., Yz, A., Lz, A., Ying, M. A., Qy, A., Lha, B. (2020). Copper Stress by Nutritional Immunity Activates the CusS-CusR Two-Component System That Contributes to Vibrio Alginolyticus Anti-Host Response But Affects Virulence-Related Properties - ScienceDirect. Aquaculture 532 (2020). doi: 10.1016/j.aquaculture.2020.736012.
Roy, K., Hilliard, G. M., Hamilton, D. J., Luo, J., Ostmann, M. M., Fleckenstein, J. M. (2009). Enterotoxigenic Escherichia Coli EtpA Mediates Adhesion Between Flagella and Host Cells. Nature 457 (7229), 594–598. doi: 10.1038/nature07568
Ryckaert, J., Bossier P, D., Herde, K., Diez-Fraile, A., Sorgeloos, P., Haesebrouck, F., et al. (2010). Persistence of Yersinia Ruckeri in Trout Macrophages. Fish Shellfish Immunol. 29 (4), 648–655. doi: 10.1016/j.fsi.2010.06.009
Saier, M. H. (2000). Families of Transmembrane Sugar Transport Proteins. Mol. Microbiol. 35 (4), 699–710. doi: 10.1046/j.1365-2958.2000.01759.x
Schaible, U. E., Kaufmann, S. H. E. (2004). Iron and Microbial Infection. Nat. Rev. Microbiol. 2 (12), 946–953. doi: 10.1038/nrmicro1046
Schauer, K., Rodionov, D. A., de Reuse, H. (2008). New Substrates for TonB-Dependent Transport: Do We Only See the ′Tip of the Iceberg′? Trends Biochem. Sci. 33 (7), 330–338. doi: 10.1016/j.tibs.2008.04.012
Sun, Y., Luo, G., Zhao, L., Huang, L., Qin, Y., Su, Y., et al. (2018). Integration of RNAi and RNA-Seq Reveals the Immune Responses of Epinephelus Coioides to sigX Gene of Pseudomonas Plecoglossicida. Front. Immunol. 9, 1624. doi: 10.3389/fimmu.2018.01624
Tao, Z., Zhou, T., Zhou, S., Wang, G. (2016). Temperature-Regulated Expression of Type VI Secretion Systems in Fish Pathogenpseudomonas Plecoglossicida Revealed by Comparative Secretome Analysis. FEMS Microbiol. Lett. 363 (22), w261. doi: 10.1093/femsle/fnw261
Tokmakova, I. P., Ryabchenko, L. E., Gerasimova, T. V., Kameneva, S. V., Yanenko, A. S. (2017). Mutations in the fusA Gene Encoding Elongation Factor G in the Coryneform Bacterium Lead to Increased Lysine Production. Appl. Biochem. Microbiol. 53 (8), 781–785. doi: 10.1134/S0003683817080075
Tsou, A. M., Zhu, J. (2010). Quorum Sensing Negatively Regulates Hemolysin Transcriptionally and Posttranslationally Invibrio Cholerae. Infect. Immun. 78 (1), 461–467. doi: 10.1128/IAI.00590-09
Wakabayashi, H., Sawada, K., Ninomiya, K., Nishimori, E. (1996). Bacterial Hemorrhagic Ascites of Ayu Caused by Pseudomonas sp. Fish Pathol. 31 (4), 239–240. doi: 10.3147/jsfp.31.239
Waldron, K. J., Robinson, N. J. (2009). How do Bacterial Cells Ensure That Metalloproteins Get the Correct Metal? Nat. Rev. Microbiol. 7 (1), 25–35.
Waldron, K. J., Rutherford, J. C., Ford, D., Robimson, N. J. (2009). Metalloproteins and Metal Sensing. Nature 460 (7257), 823–830. doi: 10.1038/nature08300
Wang, X., Du, Y., Hua, Y., Fu, M., Niu, C., Zhang, B., et al. (2017). The EspF N-Terminal of Enterohemorrhagic Escherichia Coli O157:H7 EDL933w Imparts Stronger Toxicity Effects on HT-29 Cells Than the C-Terminal. Front. Cell. Infect. Microbiol. 7, 410. doi: 10.3389/fcimb.2017.00410
Wang, R., Xu, H., Du, L., Chou, S. H., Liu, H., Liu, Y., et al. (2016). A TonB-Dependent Receptor Regulates Antifungal HSAF Biosynthesis in Lysobacter. Sci. Rep. 6 (1), 26881. doi: 10.1038/srep26881.
Watts, M., Munday, B. L., Burke, C. M. (2001). Immune Responses of Teleost Fish. Aust. Veterinary. J. 79 (8), 570–4. doi: 10.1111/j.1751-0813.2001.tb10753.x
Weinberg, E. D. (2009). Iron Availability and Infection. Biochim. Biophys. Acta (BBA) - Gen. Subj. 1790 (7), 600–605. doi: 10.1016/j.bbagen.2008.07.002
Wu, J., Shi, Y. H., Zhang, X. H., Li, C. H., Li, M. Y., Chen, J. (2015). Molecular Characterization of an IL-1beta Gene From the Large Yellow Croaker (Larimichthys Crocea) and its Effect on Fish Defense Against Vibrio Alginolyticus Infection. Dongwuxue Yanjiu 36 (3), 133–141.
Xu, W., Lin, W., Wang, Z., Gao, Y., Luo, Y., Grossart, H. P., et al. (2021). Disentangling the Abundance and Structure of Vibrio Communities in a Semi-Enclosed Bay With Mariculture (Dongshan Bay, Southern China). Comput. Struct. Biotechnol. J. 19, 4381–4393. doi: 10.1016/j.csbj.2021.07.040
Yang, J., Zhang, Y. (2015). I-TASSER Server: New Development for Protein Structure and Function Predictions. Nucleic Acids Res. W1), W174–W181. doi: 10.1093/nar/gkv342
Zhang, B., Luo, G., Zhao, L., Huang, L., Qin, Y., Su, Y., et al. (2018a). Integration of RNAi and RNA-Seq Uncovers the Immune Responses of Epinephelus Coioides to L321_RS19110 Gene of Pseudomonas Plecoglossicida. Fish Shellfish Immunol. 81, 121–129. doi: 10.1016/j.fsi.2018.06.051
Zhang, M., Yan, Q., Mao, L., Wang, S., Huang, L., Xu, X., et al. (2018b). KatG Plays an Important Role in Aeromonas Hydrophila Survival in Fish Macrophages and Escape for Further Infection. Gene 672, 156–164. doi: 10.1016/j.gene.2018.06.029
Zhang, C., Yu, L., Qian, R. (2007). Characterization of OmpK, GAPDH and Their Fusion OmpK-GAPDH Derived From Vibrio Harveyi Outer Membrane Proteins: Their Immunoprotective Ability Against Vibriosis in Large Yellow Croaker (Pseudosciaena Crocea). J. Appl. Microbiol. 103 (5), 1587–1599. doi: 10.1111/j.1365-2672.2007.03386.x
Zhang, C., Freddolino, P. L., Zhang, Y. (2017). COFACTOR: Improved Protein Function Prediction by Combining Structure, Sequence and Protein-Protein Interaction Information. Nucleic Acids Res. 45 (W1), W291–W299. doi: 10.1093/nar/gkx366
Zhang, J. T., Zhou, S. M., An, S. W., Chen, L., Wang, G. L. (2014). Visceral Granulomas in Farmed Large Yellow Croaker,Larimichthys Crocea(Richardson), Caused by a Bacterial Pathogen,Pseudomonas Plecoglossicida. J. Fish Dis. 37 (2), 113–121. doi: 10.1111/jfd.12075
Zheng, W., Zhang, C., Li, Y., Pearce, R., Bell, E. W., Zhang, Y. (2021). Folding non-Homologous Proteins by Coupling Deep-Learning Contact Maps With I-TASSER Assembly Simulations. Cell. Rep. Methods 1 (3), 100014. doi: 10.1016/j.crmeth.2021.100014
Zhong, Y., Qi, W., Xu, W., Zhao, L., Xiao, B., Yan, Q., et al. (2021). Insights Into Mesophilic Virulence, Antibiotic Resistant and Human Pathogenicity: A Genomics Study on the Aeromonas Salmonicida SRW-OG1 Newly Isolated From the Asian Fish Epinephelus Coioides - ScienceDirect. Aquaculture 539 (2021). doi: 10.1016/j.aquaculture.2021.736630
Keywords: visceral white spot disease, Pseudomonas plecoglossicida, virulence, fusA, environment adaptation
Citation: He R, Wang J, Lin M, Tian J, Wu B, Tan X, Zhou J, Zhang J, Yan Q and Huang L (2022) Effect of Ferredoxin Receptor FusA on the Virulence Mechanism of Pseudomonas plecoglossicida. Front. Cell. Infect. Microbiol. 12:808800. doi: 10.3389/fcimb.2022.808800
Received: 04 November 2021; Accepted: 17 February 2022;
Published: 22 March 2022.
Edited by:
Lanming Chen, Shanghai Ocean University, ChinaReviewed by:
Xiaohui Zhou, University of Connecticut, United StatesYongquan Su, Xiamen University, China
Tianjun Xu, Shanghai Ocean University, China
Copyright © 2022 He, Wang, Lin, Tian, Wu, Tan, Zhou, Zhang, Yan and Huang. This is an open-access article distributed under the terms of the Creative Commons Attribution License (CC BY). The use, distribution or reproduction in other forums is permitted, provided the original author(s) and the copyright owner(s) are credited and that the original publication in this journal is cited, in accordance with accepted academic practice. No use, distribution or reproduction is permitted which does not comply with these terms.
*Correspondence: Qingpi Yan, eWFucXBAam11LmVkdS5jbg==; Lixing Huang, bGl4aW5naHVhbmdAam11LmVkdS5jbg==
†These authors share first authorship