- 1Menzies Health Institute Queensland and School of Pharmacy and Medical Sciences, Griffith University, Southport, QLD, Australia
- 2Clem Jones Centre for Neurobiology and Stem Cell Research, Griffith University, Nathan, QLD, Australia
- 3Griffith Institute for Drug Discovery, Griffith University, Nathan, QLD, Australia
Streptococcus agalactiae causes neonatal meningitis and can also infect the adult central nervous system (CNS). S. agalactiae can cross the blood-brain barrier but may also reach the CNS via other paths. Several species of bacteria can directly invade the CNS via the olfactory and trigeminal nerves, which extend between the nasal cavity and brain and injury to the nasal epithelium can increase the risk/severity of infection. Preterm birth is associated with increased risk of S. agalactiae infection and with nasogastric tube feeding. The tubes, also used in adults, can cause nasal injuries and may be contaminated with bacteria, including S. agalactiae. We here investigated whether S. agalactiae could invade the CNS after intranasal inoculation in mice. S. agalactiae rapidly infected the olfactory nerve and brain. Methimazole-mediated model of nasal epithelial injury led to increased bacterial load in these tissues, as well as trigeminal nerve infection. S. agalactiae infected and survived intracellularly in cultured olfactory/trigeminal nerve- and brain-derived glia, resulting in cytokine production, with some differences between glial types. Furthermore, a non-capsulated S. agalactiae was used to understand the role of capsule on glial cells interaction. Interestingly, we found that the S. agalactiae capsule significantly altered cytokine and chemokine responses and affected intracellular survival in trigeminal glia. In summary, this study shows that S. agalactiae can infect the CNS via the nose-to-brain path with increased load after epithelial injury, and that the bacteria can survive in glia.
Introduction
Cranial nerves extending between the nasal cavity and the brain constitute a route by which certain microbes can invade the central nervous system (CNS). These nerves are the olfactory nerve and the intranasal branches of the trigeminal nerve. The cell bodies of sensory (primary) olfactory neurons are localized in the olfactory neuroepithelium of the nasal cavity. Their dendrites extend into the nasal epithelium, where odorant molecules are detected, and their axons reach all the way into the olfactory bulb in the brain, where they connect with second order neurons. The axons of sensory olfactory neurons together constitute the highly fasciculated olfactory nerve. The olfactory nerve is thus a direct connection between the periphery and the brain (Graziadei and Monti Graziadei, 1985). The anatomy of the trigeminal nerve is distinctly different; the cell bodies of its sensory neurons are found in the trigeminal ganglia, and there are only trigeminal axons near the nasal epithelium. The distal ends of these axons, however, are localized very close to the apical surface of the nasal epithelium (Schaefer et al., 2002). Thus, both the olfactory and the trigeminal nerves constitute potential invasion paths for infectious agents (Dando et al., 2014) (Figure 1A).
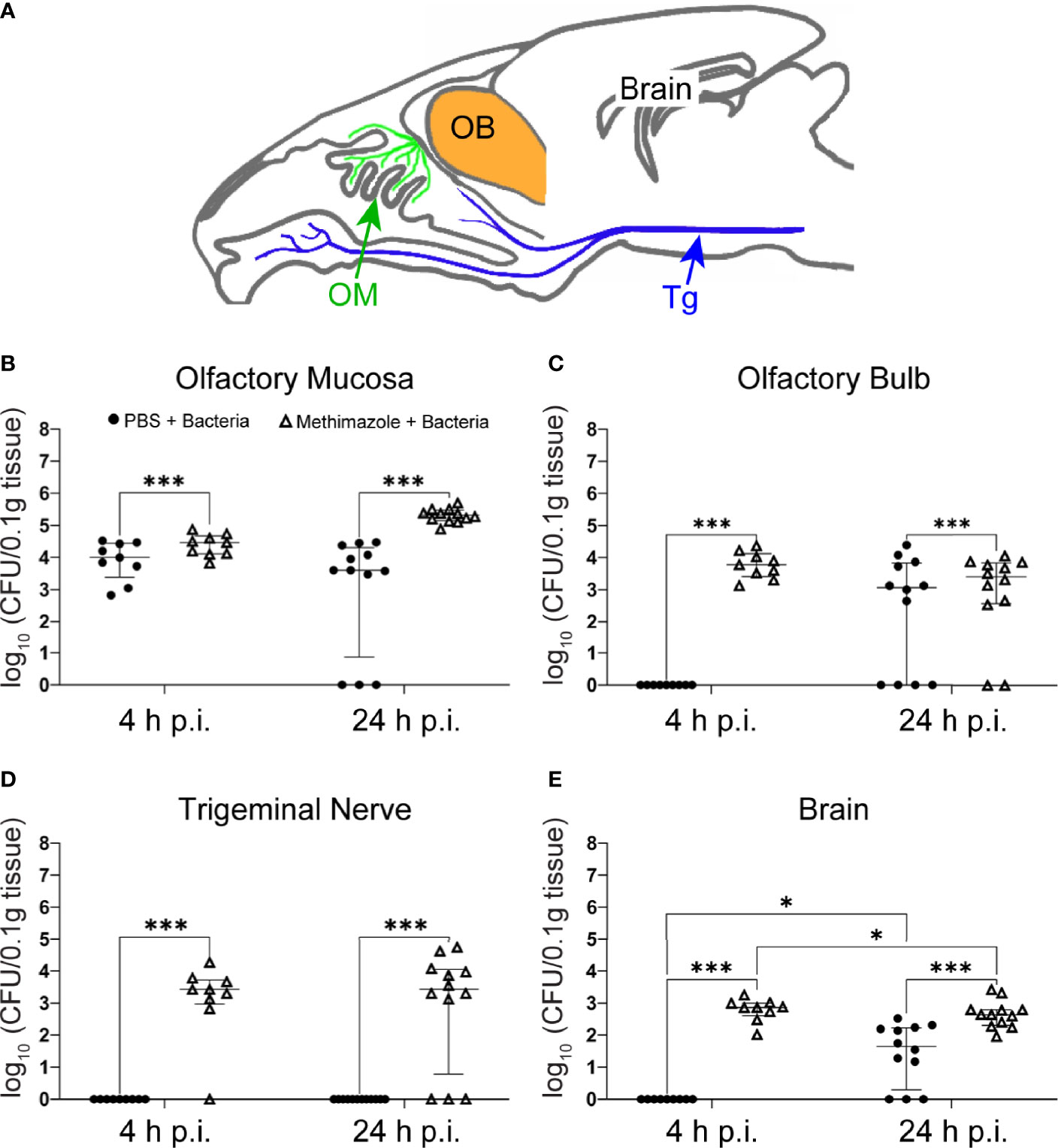
Figure 1 S. agalactiae can invade the CNS via the olfactory nerve but infects the trigeminal nerve only after epithelial injury. Mice were treated with either methimazole or vehicle (PBS), followed by intranasal inoculation with S. agalactiae (1 x 106 CFU) or vehicle (also PBS). (A) Schematic of sagittal view of mouse brain, with olfactory mucosa (OM) and olfactory nerve (green), trigeminal nerve (Tg; blue), olfactory bulb (OB; orange), and brain. Tissue of Tg was collected from region shown by blue arrow. Graphs show the amount of S. agalactiae isolated from tissues 4 h and 24 h after intranasal inoculation for the four groups, (1) PBS → PBS (data not shown as no bacteria was isolated, Supplementary Data Table 1), (2) methimazole → PBS (data not shown as no bacteria was isolated, Supplementary Data Table 1), (3) PBS → S. agalactiae and (4) methimazole → S. agalactiae. (B) olfactory mucosa, (C) olfactory bulb, (D) trigeminal nerve and (E) brain. Data are pooled results from two independent biological experiments and are shown as median and interquartile range (4 h timepoint = 9 mice per group, 24 h timepoint = 12 mice). The S. agalactiae load from the different groups were compared using two-way ANOVA with significance determined using Tukey’s multiple comparison test, *p ≤ 0.05, ***p ≤ 0. 001. For mice with zero bacterial counts, these were assigned a value of 1 in order to plot on log10 y-axis.
The nasal epithelium constitutes a physical barrier against microbial infection of underlying nerves, and exhibits powerful innate and adaptive immune system components, supported by the nasal-associated lymphoid tissue (NALT) (Jochems et al., 2019). Should microbes penetrate this layer, they encounter the glia of the olfactory and trigeminal nerves, olfactory ensheathing cells (OECs) and trigeminal Schwann cells (TgSCs), respectively. OECs and TgSCs have innate immune functions and are considered to be the key phagocytes in these nerves (Panni et al., 2013). The astrocytes of the glia limitans layer (the physical and immunological barrier between the peripheral nervous system and the CNS), where the nerves connect with the olfactory bulb/brainstem, are also innate immune cells and constitute a “third layer of defence” against invasion of the CNS by infectious agents (Nazareth et al., 2019). Thus, the cranial nerves and the CNS are well protected from microbial invasion, and only a small number of pathogens are thought to infect the CNS via these paths. Of pathogens that can use these paths, the ability to escape glial phagocytosis, and instead infect and survive inside glia, has been suggested to be a key mechanism for bacterial infection of the CNS via cranial nerves (Macedo-Ramos et al., 2011; Dando et al., 2014; Walkden et al., 2020).
Injuries to the nasal epithelium, for example caused by allergies, localized viral infections and mechanical trauma, are relatively common (Upadhyay and Holbrook, 2004) and may expose the underlying cranial nerves to infection. Experimental injuries to the nasal epithelium of mice have been shown to increase the risk of bacterial invasion of the olfactory nerve and bulb (Walkden et al., 2020). Premature infants are frequently fed with nasogastric tubes or intubated with nasal continuous positive airway pressure (nCPAP), which are associated with nasal injuries (Imbulana et al., 2018). Nasogastric feeding tubes are frequently contaminated with potentially pathogenic bacteria at the prong-mucosal interface (Petersen et al., 2016); thus, preterm infants may be at risk for contracting CNS infection via the nose-to-brain path.
Streptococcus agalactiae (group B streptococcus, GBS) is the leading cause of neonatal meningitis, and sometimes meningoencephalitis, affecting 0.5-3 infants per 1000 live births. S. agalactiae infection has high mortality and leads to permanent neurological deficits in approximately half of surviving infants (Chin and Fitzhardinge, 1985; Horváth-Puhó et al., 2021). Transmission is typically vertical (from the genouritary tract of the mother to the naso- and oropharynx of the child during delivery) (Yadeta et al., 2018), but horizontal transmission can also occur (Morinis et al., 2011). S. agalactiae infection can cause two clinical syndromes: early-onset disease (EOD) in the first week of life, or late-onset disease (LOD), occurring in infants aged one week to three months. Infants born prematurely also have three- to 30-fold increased risk of developing both EOD and LOD than full-term infants (Melin, 2011; Nanduri et al., 2019; Mynarek et al., 2021). It has been suggested that contaminated feeding tubes may be a source of transmission for S. agalactiae (Le Doare and Kampmann, 2014; Jauneikaite et al., 2018). After delivery of breast milk via nasogastric feeding tubes, preterm infants have been shown to develop LOD, sometimes with meningitis (Olver et al., 2000).
Although less commonly, S. agalactiae can also cause meningitis in adults, with increasing incidence in recent years. Nosocomial S. agalactiae disease in adults can arise from new acquisitions or from pre-existing colonization of skin and mucosal tissues, and droplet transmission has been described. S. agalactiae meningitis particularly affects people with severe underlying conditions, can be fatal or result in serious sequelae (Gupta et al., 2018). Gastric tube feeding has also led to increased amounts of S. agalactiae in the oral flora of elderly patients, suggesting tube contamination (Takeshita et al., 2011).
S. agalactiae can cross the blood-brain barrier (Maisey et al., 2008), but the mechanisms for CNS invasion have not been fully elucidated and other paths may also be involved (Dando et al., 2014). S. agalactiae can colonize the nasal epithelium in infants (Foster-Nyarko et al., 2016) and, whilst specific data regarding colonization of the nasal mucosa in adults is lacking, S. agalactiae can be cultured from the oropharynx and/or respiratory tract of ~20-25% of adults (Roloff et al., 2018). Because other commensal bacteria in the nasopharynx that cause CNS disease can reach the brain via the nose-to-brain cranial nerve path, we hypothesized that this path constitutes a potential mechanism by which S. agalactiae can infect the CNS. S. agalactiae also exhibits capacity for intracellular survival in a variety of cell types, including macrophages, neutrophils and microvascular endothelial cells (Cumley et al., 2012); thus, perhaps S. agalactiae may also survive in glia.
In the current study, we investigated whether S. agalactiae sequence type 17 (ST17); serotype III, which is epidemiologically the most relevant in neonatal (Edmond et al., 2012) and adult (Paveenkittiporn et al., 2020; Vuillemin et al., 2020) Group B streptococcal meningitis, can infect the brain via the olfactory and/or trigeminal nerves in mice. Because epithelial injury is associated with increased infection of the olfactory nerve, we also determined the effects of prior experimental injury to the nasal epithelium on S. agalactiae infection via this path. As the ability to infect glia is thought to be important for bacterial invasion of both cranial nerves and the brain, we also determined how the key glial types in the olfactory/trigeminal nerves and glia limitans layer responded to S. agalactiae.
Methods
Bacterial Strains and Culture Conditions
Wild-type (WT) S. agalactiae strain 874391, which is a serotype III isolate and a member of the hypervirulent ST17 lineage (Sullivan et al., 2017), and an isogenic ΔcpsE strain (Sullivan et al., 2016) deficient in capsule synthesis, were routinely grown at 37°C in Todd-Hewitt broth (Thermo Fisher Scientific) with shaking at 180 rpm for 16 h. Retrospective colony counts for cultures were performed by serial dilution in phosphate-buffered saline (PBS) and plating onto tryptone soya agar plates or selective media as described below.
Ethics
The experimental procedures used in the study were conducted with the approval of the Griffith University Biosafety Committee (NLRD/09/15_var7) and the Griffith University Animal Ethics Committee (MSC/08/18/AEC) in accordance with guidelines of the Australian Commonwealth Office of Gene Technology Regulator and the National Health and Medical Research Council of Australia.
Mouse Models of Infection and Epithelial Injury
S. agalactiae infection: 7- 10 weeks old female BALB/c mice (Animal Resource Centre) under anaesthesia with isofluorane (1.5-2%) were intranasally inoculated with 10 μL containing 1x106 colony-forming units (CFUs) of WT S. agalactiae suspended in PBS, or vehicle (PBS only) as described previously for other bacteria (Nazareth et al., 2021). Mice were sacrificed 4 h and 24 h post inoculation by rising CO2 asphyxiation and tissues (olfactory mucosa, olfactory bulb, brain, trigeminal nerve (from within the cranial cavity), lungs, liver and blood) were collected. Tissues were homogenized using a TissueLyzer (QIAGEN) using 5 mm tungsten carbide beads and homogenates were suspended in 1 mL PBS for colony count assays by plating serial dilutions on CHROMID Strepto B agar (Biomerieux Cat number: 43461) or fixed for use in immunohistochemistry assays according to our established protocols (Nazareth et al., 2021).
For S. agalactiae infection post methimazole-induced epithelial injury, 7-10 weeks old female BALB/c mice (Animal Resource Centre) under anaesthesia with isofluorane (1.5-2%) were injected with methimazole (Sigma-Aldrich, 50 mg/kg, 10 mg/ml in PBS) or vehicle (PBS) using intraperitoneal injection according to our published protocol (Walkden et al., 2020). The administration of methimazole causes tissue-specific death of olfactory neurons and supporting cells secondary to degeneration of the olfactory epithelium in rodents which constitutes a more robust model of olfactory nerve injury than that caused by other chemicals (Herbert et al., 2012). Three days later when neuroepithelial damage peaks, animals were intranasally inoculated with WT S. agalactiae or vehicle as described above. Mice were sacrificed 4 h and 24 h post inoculation and used for colony count or immunohistochemistry assays as described above.
Immunohistochemistry
Following sacrifice, heads of mice were fixed in 4% paraformaldehyde (PFA) overnight at 4°C, then decalcified using 20% ethylenediaminetetraacetic acid (EDTA) for 4 weeks and embedded in optimal cutting temperature (OCT) medium (ProSciTech) for sectioning. Tissue sections (50 µm cryostat sections in sagittal planes) were blocked with 2% bovine serum albumin (BSA) in PBS with 0.3% Triton-X100 solution for 60 min at room temperature. Sections were then incubated with rabbit anti-S. agalactiae (primary antibody; Abcam, Ab53584, 1:400), diluted in PBS-Triton-X100 overnight at 4°C, then washed and incubated with secondary antibody donkey anti-rabbit IgG (highly cross-adsorbed and conjugated to Alexa Fluor 488; 1:500; Thermo Fisher Scientific, A21206) at room temperature for 1 h, washed and stained with 4’,6-diamidino-2-phenylindole (DAPI, 1:5000 Thermo Fisher Scientific, D1306).
Culture of Primary Glia
Primary glia cultures were obtained from S100β-DsRed transgenic mice (Windus et al., 2007), in which all glia express the bright red protein DsRed, as previously described (Nazareth et al., 2020). Briefly, the olfactory bulb and trigeminal ganglia were dissected out for preparations of OECs and TgSCs, respectively, from postnatal day 7 pups. Tissue explants were plated into 24-well plates, with wells pre-coated with Matrigel basement membrane matrix (Corning Matrigel Basement Membrane Matrix, FAL354234). The explants were maintained in glial medium, constituting of Dulbecco’s Modifed Eagle Medium (DMEM) (Gibco) with 10% fetal bovine serum (FBS), 50 μg/mL gentamycin (Gibco), 200 μM L-glutamine (Gibco) and G5 supplement diluted as per manufacturer’s instructions (Gibco). This established method yields 80% DsRed-positive cells which are also positive for glial markers (the p75 neurotrophin receptor, s100) (Nazareth et al., 2020).
Primary astrocytes with approximately 70-80% purity were obtained from the cortices of postnatal day 3 pups following a previous published protocol (Schildge et al., 2013). The cortices were dissected out and cut into small pieces in glial medium. The cortical cell suspension was then plated in a poly-D-lysine hydrobromide (Sigma-Aldrich P6407)-coated T75 flask. Following 7 to 8 days (90% confluency), astrocytes were separated from microglia and oligodendrocyte precursor cells by shaking the flask on an orbital shaker as previously described (Schildge et al., 2013). All the glia were cultured to 80% confluency after which they were trypsinized (using Gibco TrypLE Express, 1X) and used for experiments.
In Vitro Infection of Primary Glia
To analyse the interactions of WT S. agalactiae and the capsule-deficient ΔcpsE mutant with glia, we exposed cultured primary OECs, TgSCs and astrocytes to the bacteria (n = 3 biological and 3 technical replicates for each condition/cell type). Methods for the routine culture and cell infection of cells were performed as previously described (Nazareth et al., 2021) with the following modifications: glia were seeded in 96-well plates at 6000 cells per well and incubated at 37°C in 5% CO2 until approximately 80% confluence was achieved. Glial cell monolayers were then infected with S. agalactiae at a multiplicity of infection (MOI) of 100 bacteria per cell (or, for control, medium alone was added to the cultures) for 1 h. The plate was then incubated at 37°C in 5% CO2. Bacteria were initially allowed to attach to glia for 1 h, before the infecting inoculum was aspirated and unattached bacteria was removed by washing the well three times with PBS. Then, adherent bacteria were enumerated by colony counts on Todd-Hewitt agar.
To determine the concentration of intracellular bacteria, antibiotic protection assays were utilised (Dando et al., 2016). Adherent extracellular bacteria were removed by the addition of glial medium containing antibiotics (penicillin 250 U/mL and streptomycin 250 U/mL with gentamycin 50 µg/mL, Gibco) and the number of intracellular bacteria was determined at 2 h and 24 h post infection.
Immunocytochemistry
Glial cells were seeded onto 96-well plates at 6000 cells per well and the following day were infected with WT or ΔcpsE S. agalactiae, or medium alone (control). Infected monolayers at various times were fixed with 4% PFA for 10 min, rinsed with PBS, followed by blocking/permeabilising solution (3% BSA in PBS with 0.3% Triton X-100) for 30 min at room temperature on a shaker.
Rabbit anti-S. agalactiae (Abcam, Ab53584; 1:400) or goat anti-glial fibrillary acidic protein (GFAP) (Abcam, ab53554; 1:400) was added to the fixed cells which were kept at 4°C overnight on a rocking shaker. The following day, plates were washed with PBS and secondary antibodies were added. These were donkey anti-rabbit IgG (Alexa Fluor 488; 1:500; Thermo Fisher Scientific, A21206), or donkey anti-goat IgG (Alexa Fluor 647; 1:500; Abcam, ab150135). Cell nuclei were stained with 4’,6-diamidino-2-phenylindole (DAPI,1:5000 Thermo Fisher Scientific, D1306). To assess cytotoxicity of glial cells after infection, live cell nuclei were stained with Hoechst 33342 (1:1000, Thermo Fisher Scientific, H1399) and dead cells were stained with DRAQ7 Dye (1:500; Thermo Fisher Scientific, D15106).
For glial mediated phagocytosis of bacteria in lysosomes, rat anti-lysosomal membrane protein 2 marker antibody (LAMP2) [GL2A7] (1:800, Abcam, ab13524) and chicken anti-glial fibrillary acidic protein (GFAP) (Abcam, ab4674; 1:800) was used with the rabbit anti-S. agalactiae (Abcam, Ab53584; 1:400) at 4°C overnight on a rocking shaker. Combinations of appropriate secondary antibodies were used: goat anti-chicken IgY H&L 647 (1:1000, Abcam, ab150171) plus goat anti-rat IgG H&L (Alexa Fluor 488) (1:500, Abcam, ab150157) and goat anti-rabbit IgG H&L (Alexa Fluor 594) preadsorbed (1:500, Abcam, ab150084); with Hoechst 33342 (1:5000, Thermo Fisher Scientific, H1399) to stain nuclei.
Imaging
All high magnification images were collected using an Olympus FV3000 confocal microscope and panels show confocal images of maximum projection of z-stacks. Images were colour-balanced using Adobe Photoshop CS5 (Adobe Systems Incorporated) with the figures being compiled in Adobe Illustrator CS5 (Adobe Systems Incorporated). 3D reconstruction of z-stacks were generated and videos were compiled using Imaris 9.5.1 software.
Cytokine and Chemokine Assays
The levels of cytokines were determined in the cell culture supernatants at 24 h post inoculation with Bio-Plex Pro Mouse Cytokine 23-plex assays (Bio-Rad, Gladesville, NSW, Australia). Cell culture supernatants were collected and centrifuged at 1,000 × g for 15 min at 4°C. Samples were then stored at −80°C until analysis. Thawed samples were then assayed according to the manufacturer’s instructions. The plates were read on a BioPlex 200 Luminex bead array reader (Bio-Rad) and data were acquired with Bio-Plex Manager Software (version 5.0; Bio-Rad).
Data Analysis
Statistical testing and graphical analysis were conducted using GraphPad Prism version 8.0. Colony count data from organ lysates and from cell lysates were examined by Kruskal-Wallis Test followed by Dunn’s multiple comparison post-tests. Cytokine and chemokine expression data were compared using two-way analysis of variance (ANOVA) followed by Tukey’s multiple comparison post-hoc tests.
Results
S. agalactiae Can Invade the CNS via the Olfactory Nerve
To determine whether S. agalactiae could invade the CNS via the olfactory nerve and/or the intranasal branches of the trigeminal nerve, we intranasally inoculated mice with S. agalactiae (106 bacteria in 10 μL) and determined whether live S. agalactiae bacteria could be isolated from mucosal, nerve and CNS tissues. Control inoculations contained PBS only (vehicle). At 4 h and 24 h post inoculation, the mice were sacrificed and homogenates of the olfactory mucosa, olfactory bulb, trigeminal nerve, brain (beyond the olfactory bulb; Figure 1A), lungs, liver and blood (Supplementary Data, Table 1) were analysed for S. agalactiae load. For the control group (PBS → PBS), no bacteria were isolated from any of the tissues (olfactory mucosa, olfactory bulb, trigeminal nerve, brain, lungs, liver and blood; Supplementary Data, Table 1 and Figures 1B–E). For the S. agalactiae infected group at 4 h, median tissue load was 4 log10 CFU/0.1g tissue (interquartile range (IQR) 3.3-4.4) in olfactory mucosa and no bacteria were present in all the other organs tested (Figures 1B–E). For the S. agalactiae infected group at 24 h post infection, median tissue loads were 3.6 log10 CFU/0.1g tissue (IQR 0.8-4.3) in olfactory mucosa, 3 log10 CFU/0.1g tissue (IQR 0-3.8) in the olfactory bulb, and 1.6 log10 CFU/0.1g tissue (IQR 0.2-2.2) in the brain (Figures 1B–E). Interestingly, however, no S. agalactiae bacteria were isolated from the trigeminal nerve, lungs, liver, and blood 4 h and 24 h post infection (Figure 1D; Supplementary Data Table 1).
Injury to the Nasal Epithelium Exacerbates Infection via the Olfactory Nerve Route and Results in Infection of the Trigeminal Nerve
As injury to the olfactory epithelium has been shown to increase the amount of bacteria infecting the olfactory nerve/bulb (Walkden et al., 2020), in parallel assays to those described above we pre-injured the olfactory neuroepithelium with our well-established method using methimazole (Walkden et al., 2020), which causes death of primary olfactory neurons and damage to the olfactory epithelium. Three days later, we inoculated the mice intranasally with S. agalactiae. At this time, death of olfactory neurons is at its peak (Nazareth et al., 2015) and methimazole has been cleared, limiting potential unknown side-effects of methimazole (Xie et al., 2011). Epithelial injury significantly increased the bacterial load isolated from the olfactory mucosa, and brain (Figures 1B, C, E) at 4 h and 24 h, compared to parallel infection without injury. This also resulted in recovery of S. agalactiae from the trigeminal nerve with median 3.4 log10 CFU/0.1g tissue (IQR 2.9-3.7) at 4 h and median 3.4 log10 CFU/0.1g tissue (IQR 0.7-4) at 24 h post infection (Figure 1D). Also, no S. agalactiae bacteria were isolated from the lungs, liver, and blood 4 h and 24 h post infection. Thus, S. agalactiae can infect the CNS via the olfactory nerve, and epithelial injury resulted in significantly elevated numbers of invading bacteria in the brain and olfactory bulb. Epithelial injury also exposes the trigeminal nerve for S. agalactiae infection.
To further examine the dynamics of S. agalactiae invasion via the CNS, we immunolabelled tissue sections from the olfactory epithelium, bulb and brain for S. agalactiae. In the control uninfected animals (PBS → PBS), the olfactory epithelium was an intact layer of dense cells (Figure 2A). After methimazole treatment alone (methimazole → PBS), the epithelial layer sloughed off into the nasal cavity (Figure 2B). When S. agalactiae bacteria were intranasally inoculated after methimazole injury (methimazole → S. agalactiae), bacteria were readily detected within the remaining tissue of the olfactory epithelium as well as within the exudate in the nasal cavity (Figures 2D–F). We could not detect S. agalactiae from the olfactory mucosa of uninjured mice (Figure 2C), but, consistent with a ~100-fold reduction in the number of culturable bacteria from the uninjured vs the injured group (3.6 vs 5.3 log10 CFU/0.1g tissue, respectively), this is likely due to sparse distribution of bacteria within the tissue. In the other tissues analysed, we could not clearly distinguish S. agalactiae cocci from background immunolabelling and thus, we only show immunolabelling data for the olfactory epithelium.
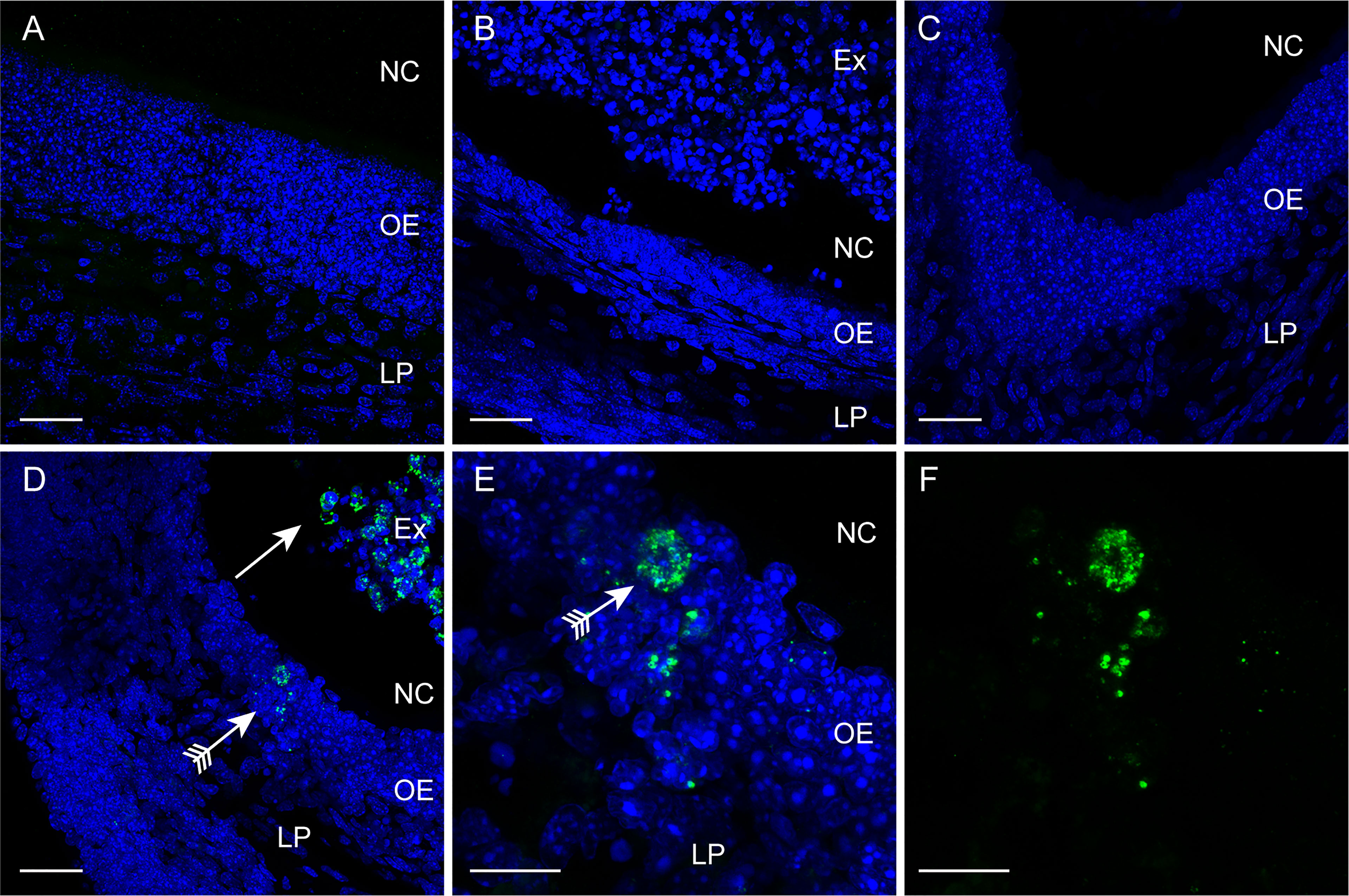
Figure 2 Immunolabelling for S. agalactiae in the olfactory mucosa. Panels show confocal microscopy images of the olfactory mucosa with immunolabelling for S. agalactiae (green), and nuclei stained with DAPI (blue) 24 h post intranasal inoculation with the bacteria or vehicle. (A) Control, vehicle-only (PBS → PBS) treatment. The olfactory epithelium (OE) and lamina propria (LP) constitute the olfactory mucosa. NC: nasal cavity. (B) Methimazole treatment only (methimazole → PBS). The OE has been degraded and is sloughing off as an exudate (Ex) into the NC. (C) Intranasal inoculation with S. agalactiae (PBS → S. agalactiae). No S. agalactiae bacteria were detected within the OE. (D) Methimazole treatment followed by intranasal S. agalactiae inoculation (methimazole → S. agalactiae). S. agalactiae bacteria were detected in the Ex (arrow) and in the OE (arrow with tail). (E, F) Higher magnification view of the OE shown in (D); (F) S. agalactiae immunolabelling alone. Scale bar: 30 μm in (A–D); 10 μm in (E, F).
S. agalactiae Can Invade and Survive Inside Glia
Glia (OECs and TgSCs) are the key phagocytes in the olfactory and trigeminal nerves, respectively, whilst astrocytes (CNS glia) in the glia limitans layer between the nerves and the CNS constitute another layer of defence against pathogens. As the capability to infect these glia is thought to constitute a key mechanism for CNS invasion, we therefore determined the susceptibility of these glia to S. agalactiae infection. Using antibiotic protection assays, we compared the adhesion and invasion capacity of S. agalactiae between primary OECs, TgSCs and astrocytes. To investigate bacterial adhesion to the cells, we exposed the cells to S. agalactiae at a multiplicity of infection of 100 bacteria per cell (MOI 100) for 1 h and determined the number of bacteria that had attached to the cells. To determine whether S. agalactiae could invade and survive within OECs, TgSCs and astrocytes, an antibiotic cocktail was added to the medium to kill extracellular bacteria. Cells were then incubated for a further 2 h or 24 h and the number of intracellular bacteria was determined for the different cell types by lysing monolayers at these timepoints. We found that S. agalactiae adhered to the cells within 1 h (Figure 3A), and bacteria could be recovered from all glia at 2 h post antibiotic addition, representing invasive S. agalactiae (Figure 3B). The frequency of invasion (% of adhered bacteria recovered at 2 h post antibiotics) was similar for the three glial types (Supplementary Figures 1A, B). After 24 h, however, bacteria were only recovered from OECs and astrocytes, and not in TgSCs (Figure 3C and Supplementary Figure 1C) suggesting that S. agalactiae was eliminated from TgSCs at 24h post inoculation. To assess the cytotoxicity of S. agalactiae on glial cells, percentage of dead cell count and total cell count was performed at 1 h and 24 h post infection. No significant cytotoxicity was observed in glial cells (OECs, TgSCs and astrocytes) post infection (Supplementary Figure 2). Thus, the trends observed in the results are due to glial responses to bacteria and not due to cell death occurring post infection. To resolve the interactions of S. agalactiae with OECs, TgSCs and astrocytes at the cellular level, we fixed and imaged infected cells at 1 h, 2 h and 24 h time-points. The glia was isolated from S100β-DsRed mice, in which the S100 promoter drives the expression of the fluorescent protein DsRed in glia, allowing easy visualisation of the cells. The expression of DsRed is lower in astrocytes than in the other glia; therefore, the identity of these cells was verified using immunolabelling for glial fibrillary associated protein (GFAP), a well-established astrocyte marker (Schildge et al., 2013) (Figures 4C, F, I, L). S. agalactiae immunolabelling showed that bacteria were attached to cells at 1 h (Figures 4D–F) and were found inside cells at 2 and 24 h post exposure (Figures 4G–L). Although bacterial counts were below the detection limit from TgSCs at 24 h post inoculation (Figure 3C), we could occasionally find S. agalactiae cocci in some TgSCs using imaging (Figure 4K). We observed that some of the cells were bi- or multinucleated, or had atypical nuclei (Figures 4I, K, L).
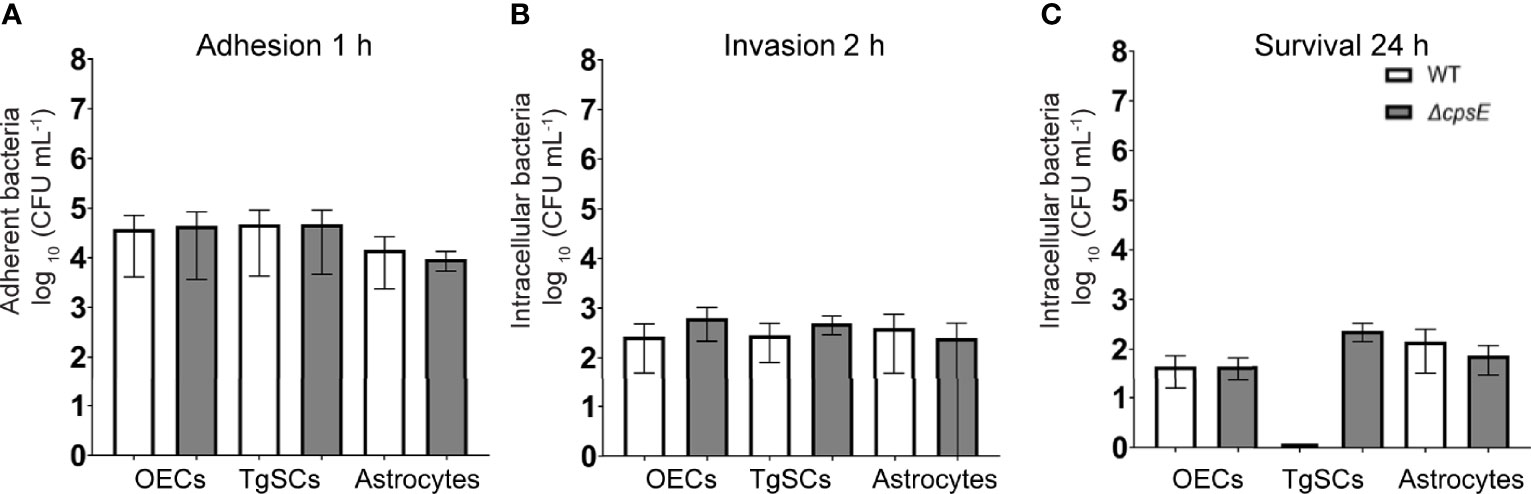
Figure 3 Comparison of adhesion/invasion of glia by S. agalactiae. (A) Cell monolayers were inoculated with WT or ΔcpsE bacteria for 1 h (MOI 100), followed by colony counts to determine the number of adherent bacteria. (B, C) After 1 h, penicillin, streptomycin and gentamicin were added to the medium to kill extracellular bacteria, and at 2 h (B) or 24 h (C) cells were lysed to recover intracellular bacteria and colony counts were performed. Data shows mean ± SEM of three independent experiments with n = 3 technical replicates. Data were compared between glial types using two-way ANOVA with Tukey’s multiple comparison test; no significant differences were detected. CFU/mL values of zero were assigned a value of 1 to enable display on log10 axes (in graph (C), TgSCs WT value is zero).
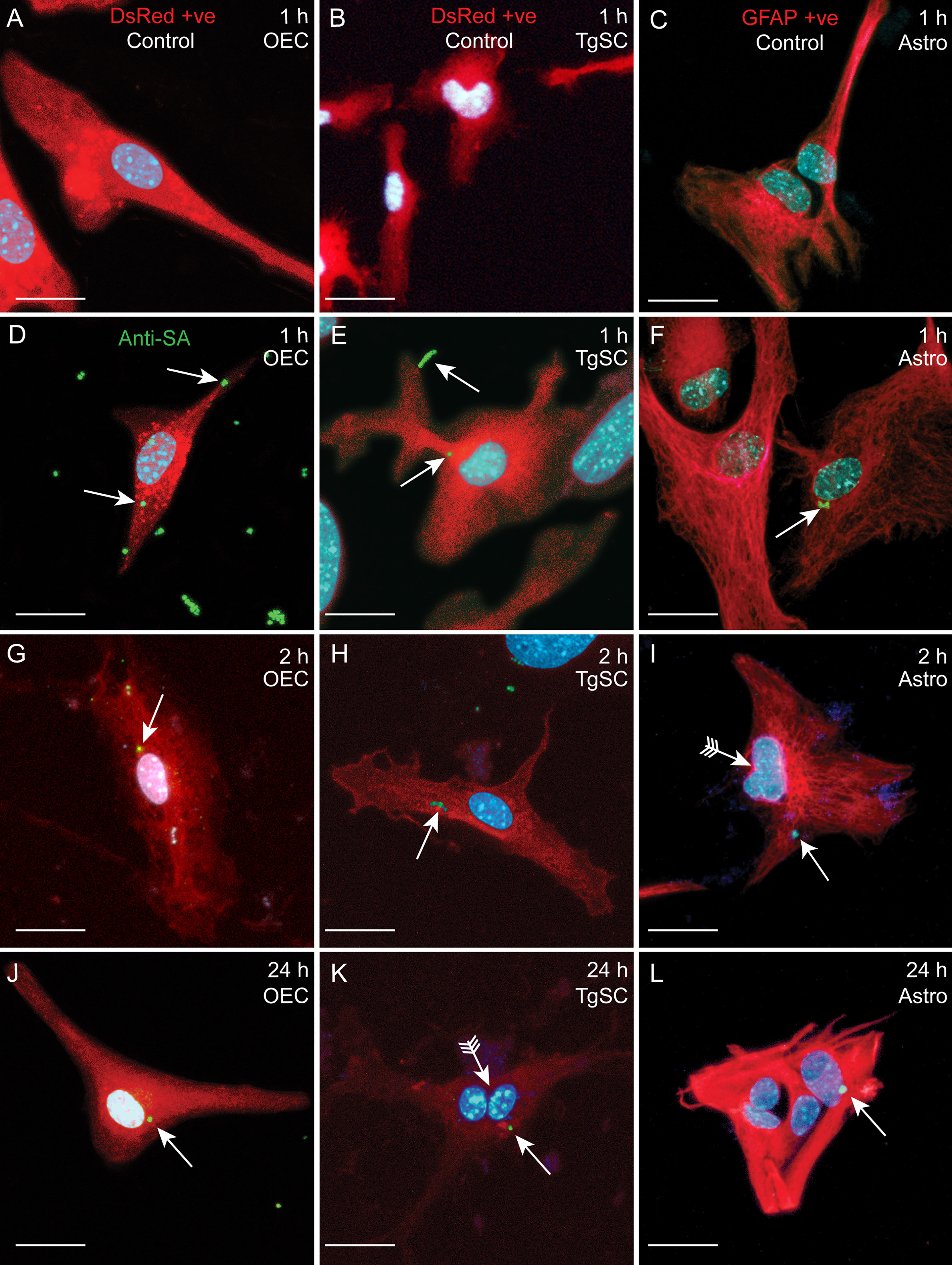
Figure 4 Confocal images showing attachment and internalisation of S. agalactiae in glia (OECs, TgSCs and astrocytes). (A–C) Control cells (without S. agalactiae). OECs and TgSCs were visualised by DsRed expression (red), whilst astrocytes were immunolabelled for GFAP (red). Nuclei are stained with DAPI (blue). (D–L) Representative images of OECs, TgSCs and astrocytes following inoculation with S. agalactiae (anti-S. agalactiae antibody (anti-SA); green, arrow). After 1 h of exposure to S. agalactiae (D–F), extracellular bacteria were removed by washing and addition of antibiotic in the medium. S. agalactiae were detected in the glia at 2 h (G–I) and at 24 h post infection (J–L). Some cells exhibited atypical nuclei or binucleation (arrows with tail). Scale bar: 20 μm.
The S. agalactiae Capsule Contributes to Invasion and Survival Within Trigeminal Schwann Cells
Lack of S. agalactiae polysaccharide capsule may alter colonization and cellular responses to the bacteria (Alkuwaity et al., 2012; Sullivan et al., 2016). Therefore, in parallel assays, we compared glial responses to an isogenic S. agalactiae mutant in cpsE (ΔcpsE) that is devoid of capsule (Sullivan et al., 2016). We found that colony counts of adhesion, invasion and intracellular survival for the ΔcpsE strain were similar to WT for OECs, TgSCs and astrocytes (Figures 3A–C); while the two-way ANOVA analysis did not reveal significant differences between any of the treatment groups, for the 24 h intracellular survival in TgSCs there was a p value of 0.08 for the comparison between ΔcpsE strain and WT. Interestingly, with a comparison of percentage of bacteria load to initial inoculum, there was significantly more ΔcpsE S. agalactiae recovered from TgSCs at 24 h compared to WT (p ≤ 0.001, Supplementary Figure 1C). In addition, ΔcpsE bacteria also had significantly higher capacity for intracellular survival in TgSCs than in astrocytes (both WT and ΔcpsE, p≤ 0.01) and also compared to OECs (WT only, ≤ 0.01) (Supplementary Figure 1C). Imaging confirmed the ΔcpsE strain could adhere to, invade and survive inside all three glial types (Figure 5).
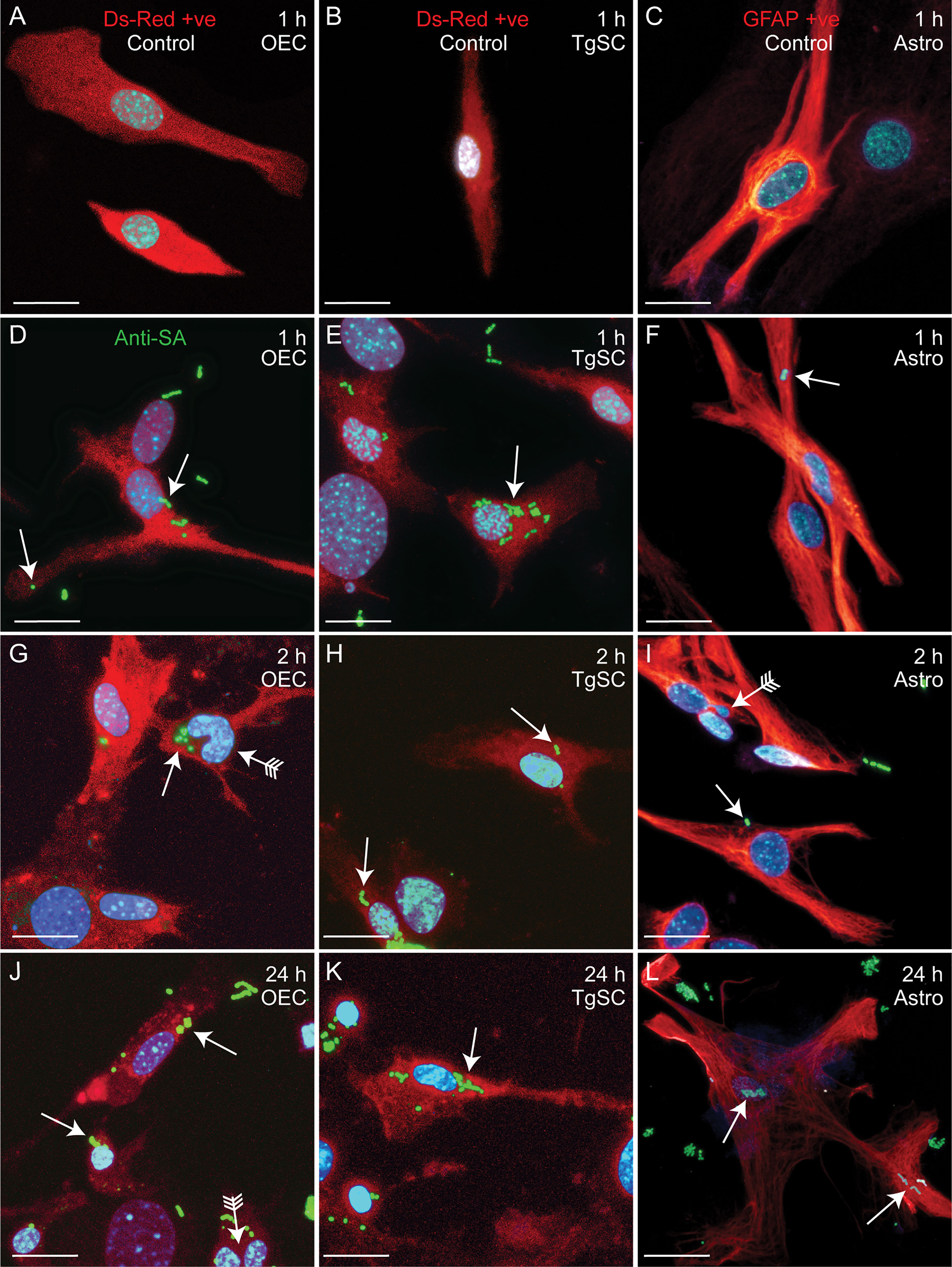
Figure 5 Confocal images showing attachment and internalisation of ΔcpsE S. agalactiae in the three types of glia. (A–C) Control cells (no S. agalactiae). Colours and immunolabelling/staining are consistent with Figure 4 (red: glia, blue: nuclei, green: ΔcpsE). (D–F) Representative images of OECs, TgSCs and astrocytes 1 h after inoculation with ΔcpsE (green, arrow). (G–L) ΔcpsE were detected in glia at 2 h (G–I) and at 24 h post inoculation (J–L). Some cells exhibited atypical nuclei or binucleation (arrows with tail). Scale bar: 20 μm.
Phagocytosis of S. agalactiae in Phagolysosomes in Glial Cells
To determine the fate of S. agalactiae after internalisation within the glia, we immunolabelled the cells at 24 h post infection for lysosome-associated membrane protein 2 (LAMP-2). LAMP-2 is the major protein component of the lysosome membrane which is required for the fusion between the late phagosome and lysosomes as well as acidification of the lysosomal lumen that helps in degradation of the internalized foreign particles such as bacteria (Eskelinen et al., 2002; Huynh et al., 2007). We observed that all glial cells (OECs, TgSCs and astrocytes) showed well-defined LAMP2-positive lysosomes around both WT and ΔcpsE S. agalactiae (Figures 6, 7) demonstrating that the bacteria were internalized or colocalized inside lysosomes after infection. To support the above statement 3D reconstruction and videos were generated which showed co-localisation of bacteria and LAMP within the cells (Supplementary Video 1 (OECs), Video 2 (TgSCs) and Video 3 (astrocytes).
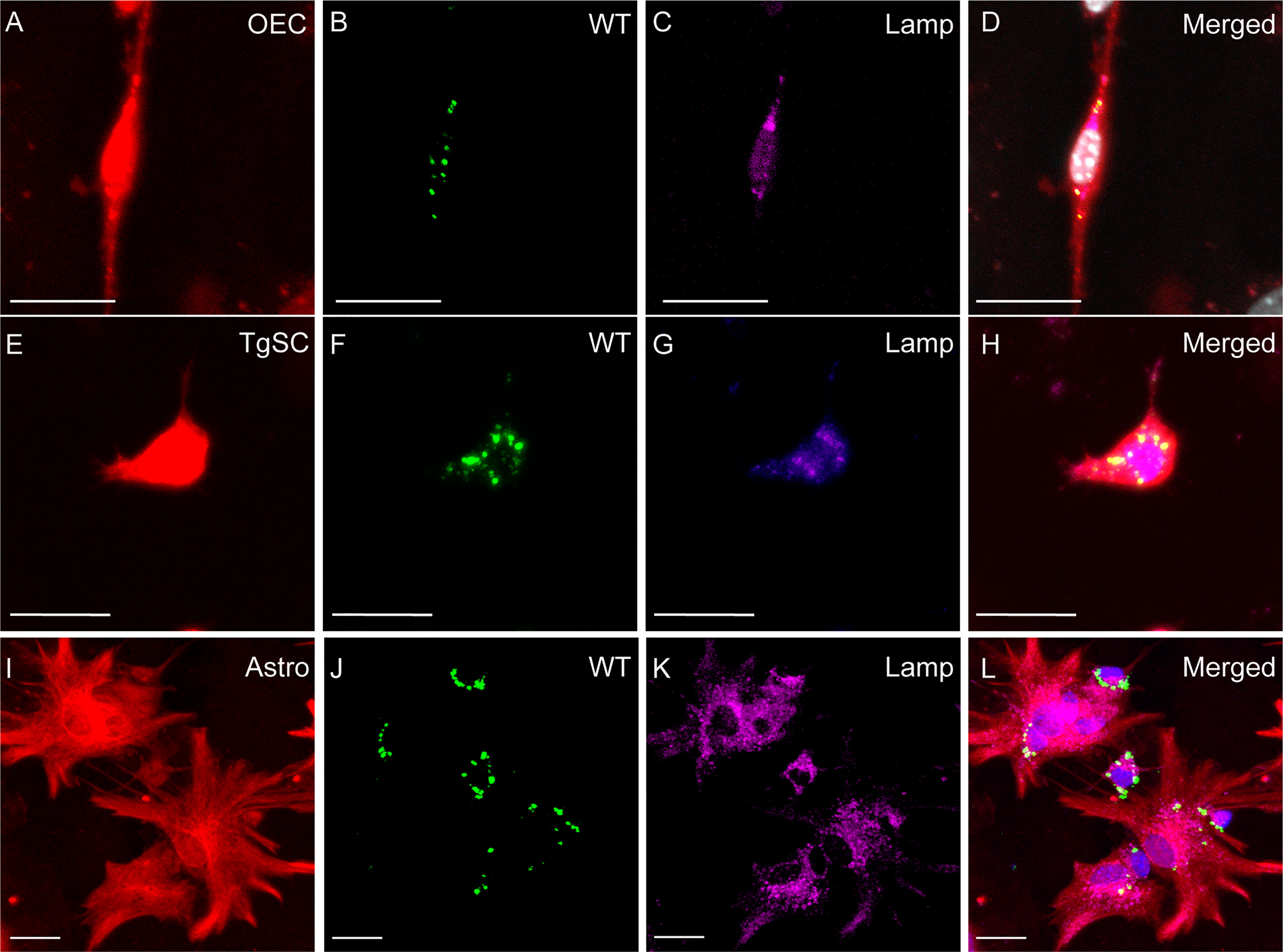
Figure 6 Phagocytosis of S. agalactiae in phagolysosomes in glial cells (OECs, TgSCs and astrocytes) 24 h post infection. Panels show primary cultures of OECs (A–D), TgSCs (E–H) and astrocytes (I–L) from S100β-DsRed mice. OEC and TgSCs were visualised by DsRed expression (red), whilst astrocytes were immunolabelled for GFAP (red). Nuclei are stained with Hoechst. (B, F, J) shows S. agalactiae within cells, (C, G, K) LAMP-2 immunolabelling for lysosomes and (D, H, L) S. agalactiae colocalizes with LAMP-2 showing the bacteria is internalized in phagolysosomes. Scale bar: 25 μm.
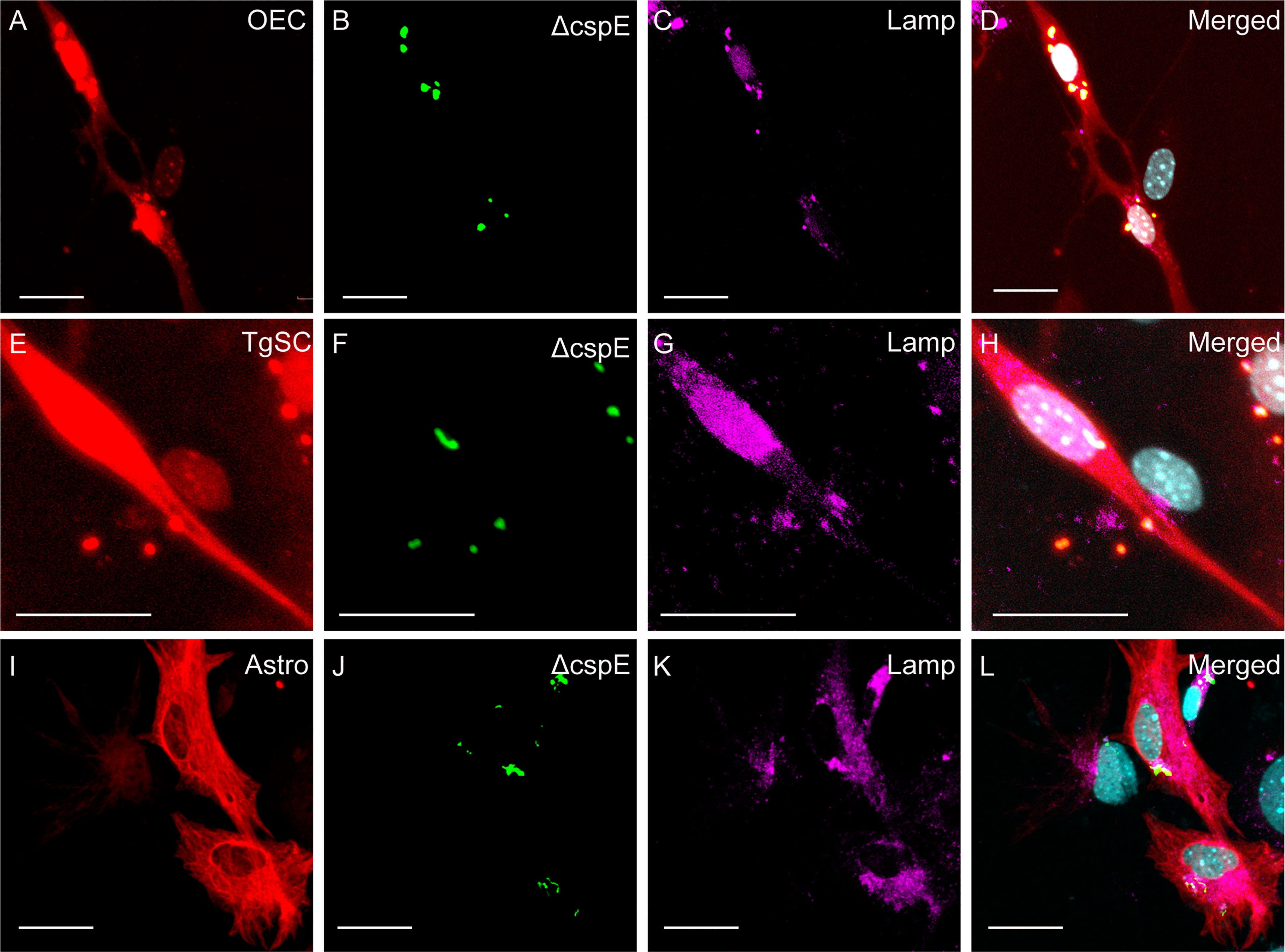
Figure 7 Phagocytosis of ΔcpsE S. agalactiae in phagolysosomes in glial cells (OECs, TgSCs and astrocytes) 24 h post infection. Panels show primary cultures of OECs (A–D), TgSCs (E–H) and astrocytes (I–L) from S100β-DsRed mice. OEC and TgSCs were visualised by DsRed expression (red), whilst astrocytes were immunolabelled for GFAP (red). Nuclei are stained with Hoechst. (B, F, J) shows S. agalactiae within cells, (C, G, K) LAMP-2 immunolabelling for lysosomes, and (D, H, L) S. agalactiae colocalizes with LAMP-2 showing the bacteria is internalized in phagolysosomes. Scale bar: 25 μm.
Glia Respond to S. agalactiae by Secreting Cytokines and Chemokines
To gain an understanding of the innate immune responses of the different glia to S. agalactiae, we also analysed secretion of cytokines and chemokines. As the S. agalactiae capsule has previously been found to alter cytokine production by other cell types (Lemire et al., 2012b), we also assessed whether the capsule affected secretion of cyto-/chemokines. We inoculated the cells with both S. agalactiae WT (white bars) and ΔcpsE strain (grey bars) and cyto/chemokine levels were analysed at 24 h post inoculation.
Cytokines
Infection of all the glial types with S. agalactiae WT and ΔcpsE strain resulted in the production of numerous pro-inflammatory cytokines (Figure 8), anti-inflammatory cytokines (Figure 9), and chemokines (Figure 10) at 24 h post-inoculation (all at significantly higher levels than non-infected control glia). The glial cytokine response included pro-inflammatory cytokines previously reported to be associated with S. agalactiae infection (Patras and Nizet, 2018), such as interleukin 1β (IL-1β), interleukin 17 (IL-17), interferon γ (IFN-γ) and tumour necrosis factor α (TNF-α). The glia also responded to both the S. agalactiae WT and ΔcpsE strain with secretion of the anti-inflammatory (regulatory) cytokines interleukin 4 (IL-4), interleukin 5 (IL-5), interleukin 6 (IL-6), interleukin 10 (IL-10) and interleukin 13 (IL-13) at 24 h post inoculation (Figure 9). Overall, S. agalactiae-infected OECs also produced higher levels of both pro- and anti-inflammatory cytokines than TgSCs and astrocytes (Figures 8, 9).
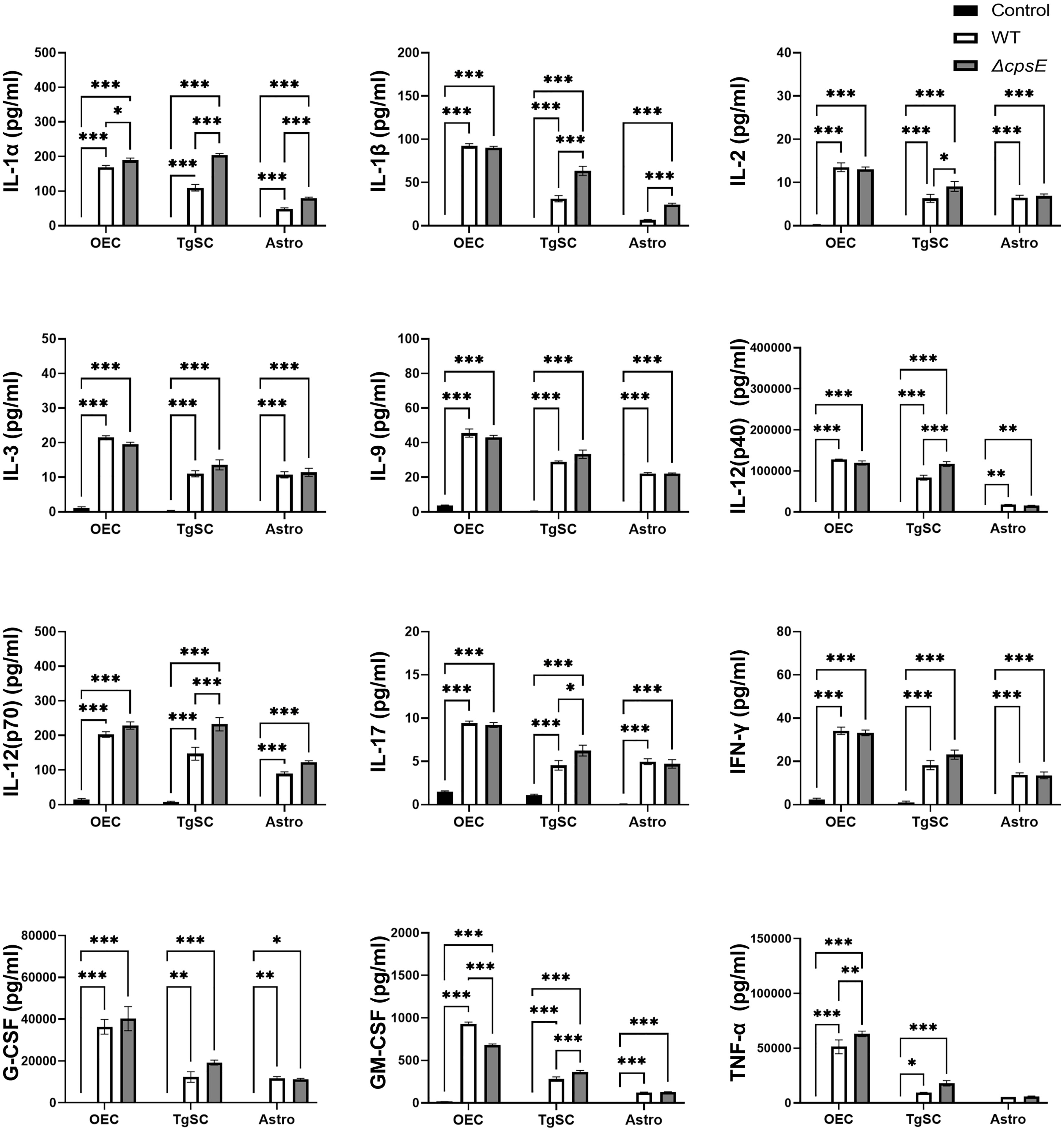
Figure 8 Pro-inflammatory cytokine responses in glia after inoculation with wild-type and capsule-deficient S. agalactiae. OECs, TgSCs and astrocytes were inoculated with either WT or ΔcpsE (MOI 100:1) after which the cytokine levels in the medium were analysed at 24 h post inoculation. Data show mean ± SEM, *p ≤ 0.05, **p ≤ 0.01, ***p ≤ 0.001 (two-way ANOVA with Tukey’s multiple comparison test, n = 3 technical replicates x 100,000 cells/well).
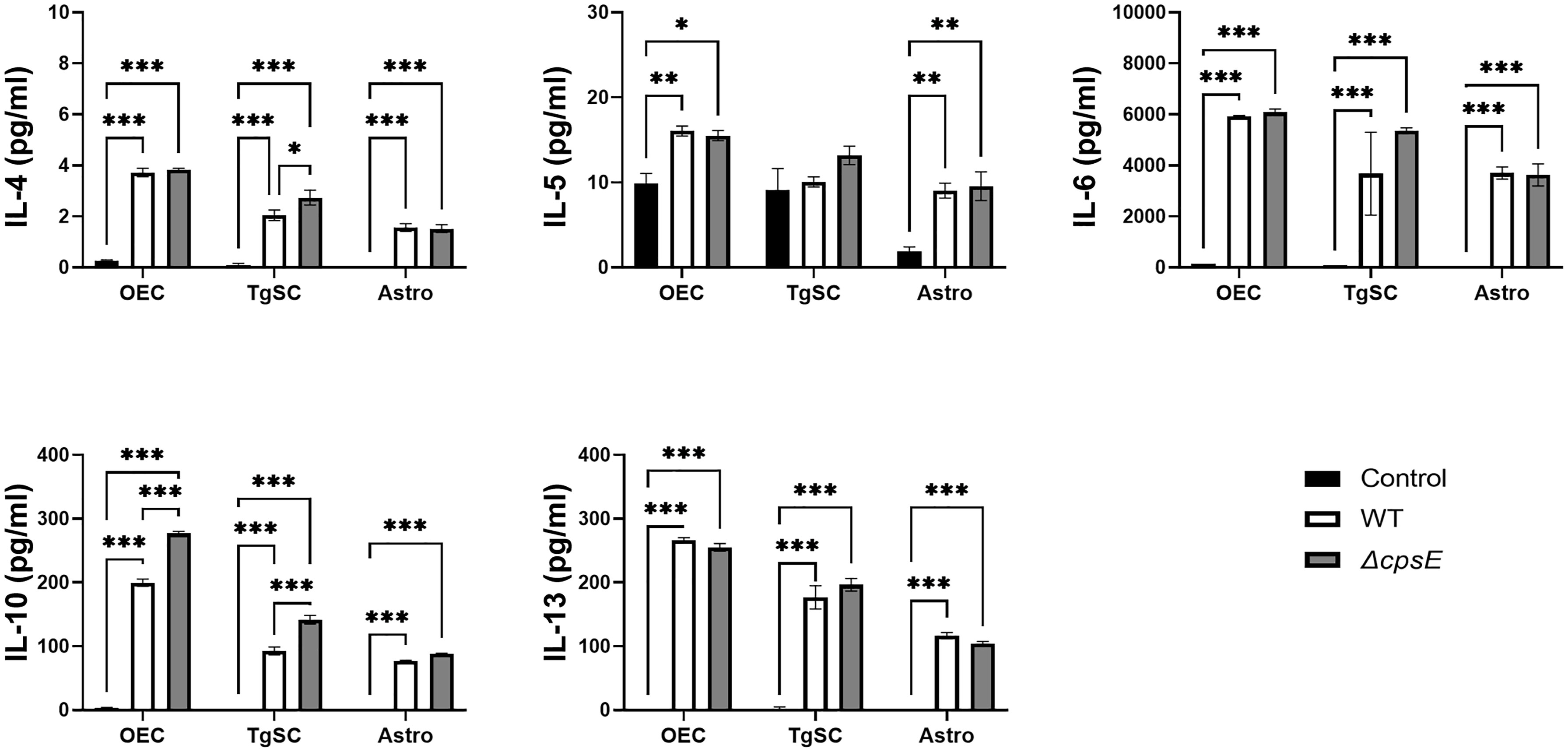
Figure 9 Anti-inflammatory cytokine responses in glia after inoculation with wild-type and capsule-deficient S. agalactiae. OECs, TgSCs and astrocytes were inoculated with either WT or ΔcpsE (MOI 100:1) after which the cytokine levels in the medium were analysed at 24 h post inoculation. Data show mean ± SEM, *p ≤ 0.05, **p ≤ 0.01, ***p ≤ 0.001 (two-way ANOVA with Tukey’s multiple comparison test, n = 3 technical replicates x 100,000 cells/well).
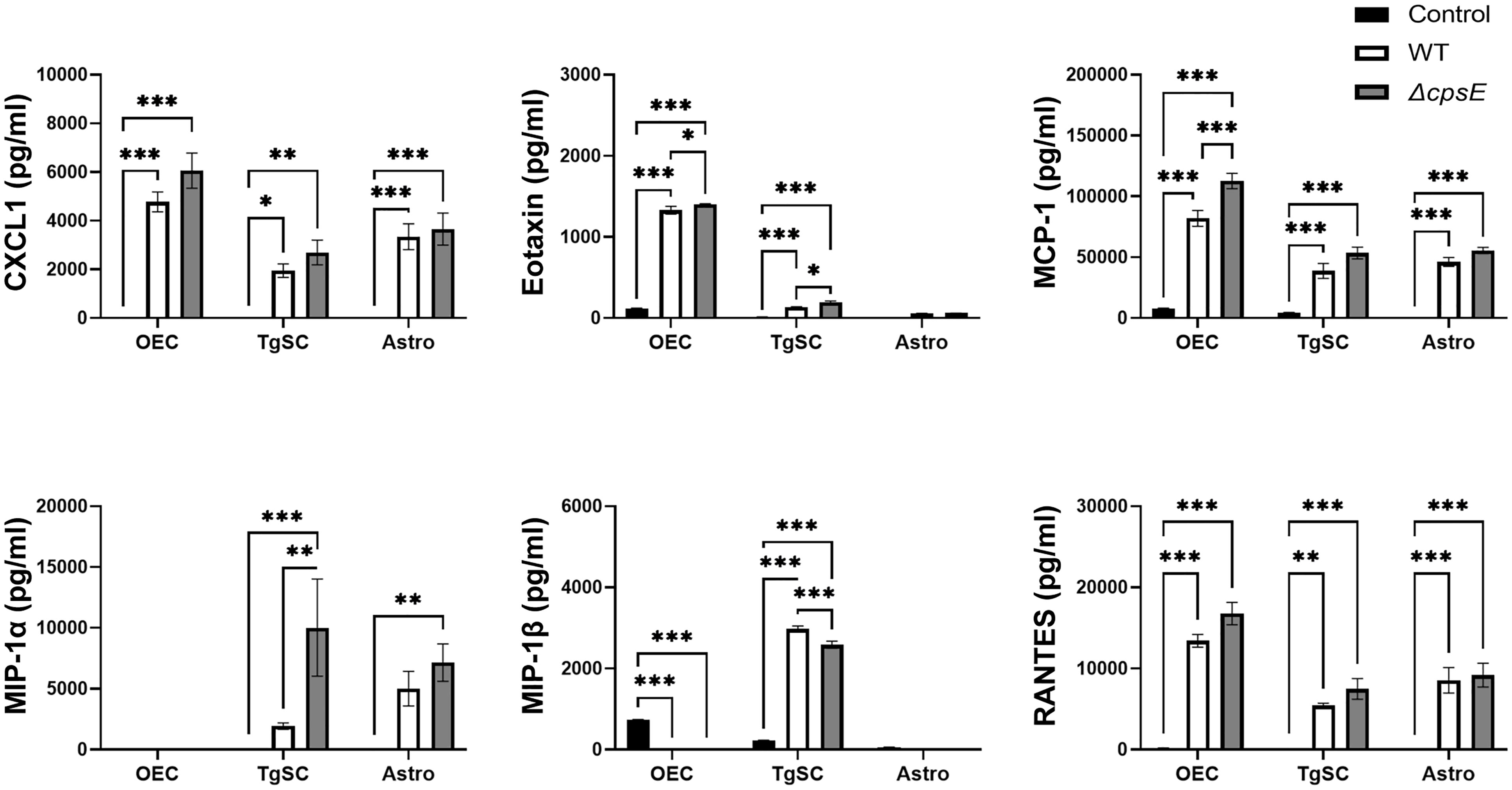
Figure 10 Chemokine responses in glia after inoculation with wild-type and capsule-deficient S. agalactiae. OECs, TgSCs and astrocytes were inoculated with either WT or ΔcpsE (MOI 100:1) after which the chemokine levels in the medium were analysed at 24 h post inoculation. Data show mean ± SEM, *p ≤ 0.05, **p ≤ 0.01, ***p ≤ 0.001 (two-way ANOVA with Tukey’s multiple comparison test, n = 3 technical replicates x 100,000 cells/well).
The glia exhibited differential cytokine responses to the wild-type and capsule-deficient S. agalactiae strains. All three glia produced significantly higher levels of IL-1α (Figure 8A), while OECs and TgSCs produced significantly higher levels of IL-10 and only TgSCs produced significantly higher levels of IL-12p70 in response to the ΔcpsE strain than to the WT strain (Figures 8, 9). For TgSCs in particular, the ΔcpsE strain was generally associated with secretion of higher levels of cytokines than the WT strain (with individual variations depending on cytokine and/or cell type; Figures 8, 9). In response to ΔcpsE strain, TgSCs secreted significantly more IL-2, IL-12p40, IL-12p70, IL-17 than in response to WT. OECs, in contrast, secreted significantly lower levels of GM-CSF after exposure to the capsule-deficient strain than to the wild-type strain (Figure 8K).
Chemokines
The glia also responded to S. agalactiae with the secretion of the chemokines C-X-C motif ligand 1 (CXCL-1, also known as Gro/KC or Groα), eotaxin, monocyte chemoattractant protein 1 (MCP-1), macrophage inflammatory proteins (MIP-1α and MIP-1β, also known as CCL3 and CCL4) and regulated upon activation-normal T cell expressed and presumably secreted (RANTES) (Figure 10). At 24 h post inoculation, OECs secreted higher levels of CXCL-1, eotaxin, MCP-1 and RANTES than the other cell types (Figure 10).
Some differences in secretion of chemokines were found between cells that had been inoculated with the WT strain versus the ΔcpsE strain. At 24 h post inoculation, all three glial types secreted higher levels of MCP-1 in response to the capsule-deficient strain than to the wild-type strain, although only OECs had significantly higher levels. TgSCs also secreted more MIP-1α in response to the S. agalactiae ΔcpsE strain than to the wild-type strain (Figure 10).
Discussion
This study demonstrates that S. agalactiae can rapidly (within 24 h) infect the CNS via the olfactory nerve in mice, and that injury to the nasal epithelium exacerbates infection via this route. We also found that injury, often associated with nasal infections or mechanical trauma in humans, exposes the trigeminal nerve to infection. In cultured cells, we showed (1) that S. agalactiae can infect and survive inside glia (primary mouse OECs, TgSCs and astrocytes), (2) resulting in glial-mediated secretion of cytokines and chemokines, and (3) that the S. agalactiae capsule deficiency plays a major role in cellular interactions with TgSCs and cytokine/chemokine secretion. Together, these findings show that cranial nerves extending between the nasal cavity and the brain constitute an alternative path by which S. agalactiae can infect the CNS, which may explain why meningitis can occur in patients despite negative blood cultures (Garges et al., 2006). The outcomes also suggest that intracellular survival in glia constitutes a mechanism behind the capacity for invading the brain via these routes.
The olfactory nerve has long been hypothesized to be a potential invasion route for pathogens causing bacterial meningitis (Filippidis and Fountas, 2009). Rapid infection of the meninges/CNS via this route has been demonstrated for Neisseria meningitidis (Sjolinder and Jonsson, 2010) and Streptococcus pneumoniae (van Ginkel et al., 2003), even in the absence of blood infection. Chlamydia pneumoniae, which is predominantly a respiratory pathogen, is also considered to infect the CNS via this path (Balin et al., 2018) and Treponema species (Riviere et al., 2002) appear to primarily reach the CNS via the trigeminal nerve. Chlamydia muridarum (Nazareth et al., 2021) (Chlamydia commonly used to model rodent chlamydia lung infection), Burkholderia pseudomallei (St John et al., 2014; Dando et al., 2016) and Listeria monocytogenes have also been shown to infect the CNS via both the olfactory and the trigeminal nerve routes in mice, with circumstantial evidence of trigeminal nerve/brainstem involvement in humans for the latter two (Drevets and Bronze, 2008; Pagelow et al., 2018). Certain viruses such as herpes simplex type 1 (HSV1) (Shivkumar et al., 2013; Doll et al., 2019) and SARS-CoV2 (Brouwer et al., 2020) are also considered capable of invading the brain via the olfactory and/or trigeminal nerve routes. The majority of these infectious agents can invade the CNS via the nerves rapidly (within 24-48 h) (Dando et al., 2014).
Infection of the olfactory and/or trigeminal nerve by B. pseudomallei (St John et al., 2014) and N. meningitidis (Sjolinder and Jonsson, 2010) are associated with patchy injury to the nasal epithelium. In the current study, we did not observe epithelial damage when mice were infected with S. agalactiae alone; despite this, the bacteria were able to enter the olfactory nerve. This is in alignment with previous studies of olfactory nerve infection by S. pneumoniae (van Ginkel et al., 2003), but in contrast to studies on S. aureus, which requires epithelial injury to enter the nerve. From the trigeminal nerve, however, no bacteria were isolated without prior epithelial injury. In contrast, when the olfactory epithelium was injured, we found that S. agalactiae entered the trigeminal nerve. This finding suggests that perhaps other cell types restricted to the olfactory neuroepithelium are involved in the mechanism by which S. agalactiae enters the olfactory nerve in the absence of injury.
The capacity for intracellular survival in glia has been suggested as a key mechanism by which bacteria can infect the olfactory and trigeminal nerves. Intracellular survival in OECs and/or TgSCs has previously been shown for S. pneumoniae (Macedo-Ramos et al., 2014), C. muridarum (Nazareth et al., 2021), B. pseudomallei (Walkden et al., 2020), and N. meningitidis (Delbaz et al., 2020). The current study demonstrates that S. agalactiae can survive inside cultured OECs and astrocytes for at least 24 h. The ability to resist killing and to survive in OECs is likely important for olfactory nerve infection, and in astrocytes, for traversing the glia limitans layer and for entry into the CNS (Ruiz-Mendoza et al., 2016). Whilst intracellular survival in OECs has not been previously investigated for S. agalactiae, one previous study has shown that S. agalactiae (including serotype III) can survive in astrocytes for at least 12 h (Stoner et al., 2015), which is in accordance with our findings. We found that S. agalactiae is attenuated for intracellular survival in TgSCs compared to other glial types. This finding was surprising, given the fact that OECs have been shown to mount a much more powerful immune response to Escherichia coli and pathogen-associated molecular patterns (PAMPs) (Vincent et al., 2007), as well as exhibit higher levels of immune- and inflammatory-related factors on the transcriptional level (Vincent et al., 2005) than Schwann cells. It is important to note that these previous investigations used Schwann cells from the sciatic nerve, and not the trigeminal nerve; TgSCs may exhibit differential immune responses than other Schwann cells due to their anatomical location near the nasal cavity (Choudhury et al., 2021). A recent study, however, has shown that OECs are better protected against intracellular survival of C. muridarum (Nazareth et al., 2021), and are more efficient phagocytes of necrotic cellular debris, than TgSCs (Nazareth et al., 2020). It is thus possible that OECs and TgSCs may respond differently to distinct phagocytic targets and while colocalisation of LAMP2 with the internalised bacteria appeared similar across the different glial types, further investigations may reveal mechanisms that modulate intracellular survival or destruction of the bacteria in the different glia. Whilst the intact nasal epithelium is likely to be the key to protecting the trigeminal nerve from infection, the low capacity for survival in TgSCs may also contribute to the lack of trigeminal nerve S. agalactiae infection.
Glial activation is one of the first hallmarks of nervous system infection and neuroinflammation, characterized by cytokine and chemokine release (Refolo and Stefanova, 2019). These factors are protective in that they stimulate clearance of infectious agents and cell debris, with some factors also directly promoting tissue repair. However, if inflammation is excessive and long-lasting, it can lead to neurotoxicity and tissue damage. The host inflammatory response to S. agalactiae contributes significantly to the pathogenesis of meningitis and CNS injury (Patras and Nizet, 2018). We here showed that S. agalactiae infection resulted in the production of many pro- and anti-inflammatory cytokines and chemokines by glia.
All three glial types responded with secretion of the pro-inflammatory cytokines IL-12, TNF-α, IL-1β, IL-17 and IFN-γ, which are also secreted at high levels in mouse models of S. agalactiae sepsis (Patras and Nizet, 2018). IL-12, TNF-α and IL-1β are key mediators of for the initial inflammation cascade in response to bacteria (Wennekamp and Henneke, 2008), including S. agalactiae (Patras and Nizet, 2018). IL-12, in particular, has key roles in combatting S. agalactiae infections (Patras and Nizet, 2018), including urinary tract infection (Sullivan et al., 2016) and sepsis (Landwehr-Kenzel and Henneke, 2014). High levels of TNF-α can cause damage to the brain tissue, but peripheral glia produce pituitary adenylate cyclase activating peptide (PACAP) (Hegg et al., 2003), which may protect against TNF-α-mediated damage (Kanekar et al., 2010). Production of pro-inflammatory cytokines was overall highest in OECs, followed by TgSCs and then astrocytes. Secretion of these factors may be important for OECs and TgSCs (peripheral glia) in preventing microbial invasion of the brain via peripheral nerves.
The pro-inflammatory glial responses were counter-balanced by the generation of the anti-inflammatory cytokines IL-6 and IL-10. IL-6 is important for regeneration/cell survival, whereas IL-10 overall limits inflammation (Landwehr-Kenzel and Henneke, 2014). Astrocytes induce transcription of IL-6 in response to S. agalactiae infection (Stoner et al., 2015), which is considered important for prevention of S. agalactiae-induced damage to the CNS tissue (Landwehr-Kenzel and Henneke, 2014). OECs and TgSCs have previously been shown to produce IL-12 and IL-6 in response to both Gram-negative and Gram-positive bacteria (Dando et al., 2016; Choudhury et al., 2021; Nazareth et al., 2021). Thus, secretion of IL-12 and IL-6 may be key mediators of the peripheral glial responses to a wide range of infectious agents. In the current study, we found that the glia also responded to S. agalactiae with secretion of several chemokines (usually secreted secondary to pro-inflammatory cytokines) that mediate recruitment of leukocytes (Graves and Jiang, 1995) and have important roles in neuroinflammation and neural repair (Miller et al., 2008).
S. agalactiae produces a polysaccharide capsule, which protects against opsonisation and, thus, opsonophagocytosis by several cell types, as well as suppresses oxidative burst and subsequent release of proteases by neutrophils (Carlin et al., 2009). S. agalactiae are classified into 10 different serotypes based on capsule composition (Afshar et al., 2011), however, 0.5% of clinical S. agalactiae isolates do not react with any diagnostic anti-capsular serum (Ippolito et al., 2010), and are considered non-typeable (NT). NT strains may either completely or in part lack the capsule. Human clinical isolates completely lacking the entire capsular locus have been identified using PCR (Creti et al., 2012). In a murine model of urinary tract infection, lack of the S. agalactiae type III capsule was shown to enhance bladder colonization (Sullivan et al., 2016).
In the current study, we showed that the capsule did not affect adhesion and intracellular survival by S. agalactiae in OECs and astrocytes. These findings suggest that capsule-independent mechanisms mediate attachment and internalization of S. agalactiae in these cells, as has previously been shown for dendritic cells in which both capsule-dependent and independent internalization mechanisms have been described (Lemire et al., 2012a). Regarding astrocytes, this finding contradicts a previous study, which showed that presence of the capsule impaired intracellular survival of S. agalactiae, however the study used S. agalactiae serotype I and not III (Alkuwaity et al., 2012). In TgSCs, however, intracellular survival of wild-type S. agalactiae was negligible, whereas the capsule-deficient mutant exhibited significant intracellular survival (in fact, higher than in astrocytes); thus, the capsule appeared to repress intracellular survival in TgSCs, however the mechanism is yet to be determined. It is worth noting that our assays used heat-inactivated bovine serum (inactivated complement system) in the medium; this would reduce S. agalactiae capsule interaction with the opsonizing effects of the complement system (Maisey et al., 2008; Patras and Nizet, 2018).
We found that the capsule also moderately affected the production of cyto/chemokines. Overall, the presence of the capsule repressed the production of multiple cyto/chemokines by the glia, but enhanced secretion of some specific ones by OECs. Overall, the capsule promoted secretion of some chemokines by OECs, whilst having the opposite effects on TgSCs and astrocytes. Thus, the interrelationship between cytokine production and intracellular survival remains unclear.
The study has several limitations, which are important to address in future investigations of how S. agalactiae invade the CNS via cranial nerves and infect glia. Long-term in vivo studies are required to determine S. agalactiae survival in the nerves and CNS, and whether S. agalactiae causes associated neuropathologies as has been shown for C. pneumoniae and HSV-1 (Balin et al., 2018) for example. The cell cultures had purities of 70-80% and thus the cytokine responses reflect the total cell population and not just the target glial cells; organoid type 3D cultures in future may reveal more in vivo like responses. Further investigations focussing on the potential for S. agalactiae to cause morphological changes, such as nuclear abnormalities, are also needed.
Conclusion
In the current study, S. agalactiae was shown to invade the CNS via the olfactory nerve in mice after intranasal inoculation. We also showed that epithelial injury was associated with increased infection of the olfactory nerve and also led to S. agalactiae infection of the trigeminal nerve. Finally, we showed that S. agalactiae also infected and survived intracellularly in the glia of both the olfactory and the trigeminal nerves and CNS. Importantly, this ability to infect glia may also constitute a key reason for the bacterial invasion of these cranial nerves and the brain.
Data Availability Statement
The original contributions presented in the study are included in the article/Supplementary Material. Further inquiries can be directed to the corresponding author.
Ethics Statement
All procedures were approved by the Griffith University Biosafety Committee (NLRD/09/15_var7) and the Griffith University Animal Ethics Committee (MSC/08/18/AEC) in accordance with guidelines of the Australian Commonwealth Office of Gene Technology Regulator and the National Health and Medical Research Council of Australia.
Author Contributions
Experiments were conducted by AC, AD, IC, TE, MS, and CG. Figures were prepared by AC, AD, JS, and JE. All authors analysed the data. AC, AD, and JE wrote the main manuscript and all authors reviewed and edited the manuscript. JE and GU provided the overall supervision of the project. All authors contributed to the article and approved the submitted version.
Funding
This study was supported by a Menzies Health Institute Queensland Capacity Grant (Griffith University) to JE, MS, and GU and a Clem Jones Foundation grant to JE and JS. The funders had no role in study design, data collection and interpretation, or the decision to submit the work for publication.
Conflict of Interest
The authors declare that the research was conducted in the absence of any commercial or financial relationships that could be construed as a potential conflict of interest.
Publisher’s Note
All claims expressed in this article are solely those of the authors and do not necessarily represent those of their affiliated organizations, or those of the publisher, the editors and the reviewers. Any product that may be evaluated in this article, or claim that may be made by its manufacturer, is not guaranteed or endorsed by the publisher.
Supplementary Material
The Supplementary Material for this article can be found online at: https://www.frontiersin.org/articles/10.3389/fcimb.2022.793416/full#supplementary-material
References
Afshar, B., Broughton, K., Creti, R., Decheva, A., Hufnagel, M., Kriz, P., et al. (2011). International External Quality Assurance for Laboratory Identification and Typing of Streptococcus Agalactiae (Group B Streptococci). J. Clin. Microbiol. 49 (4), 1475–1482. doi: 10.1128/JCM.02365-10
Alkuwaity, K., Taylor, A., Heckels, J. E., Doran, K. S., Christodoulides, M. (2012). Group B Streptococcus Interactions With Human Meningeal Cells and Astrocytes. Vitro PloS One 7 (8), e42660. doi: 10.1371/journal.pone.0042660
Balin, B. J., Hammond, C. J., Little, C. S., Hingley, S. T., Al-Atrache, Z., Appelt, D. M., et al. (2018). Chlamydia Pneumoniae: An Etiologic Agent for Late-Onset Dementia. Front. Aging Neurosci. 10, 302. doi: 10.3389/fnagi.2018.00302
Brouwer, M. C., Ascione, T., Pagliano, P. (2020). Neurologic Aspects of Covid-19: A Concise Review. Infez Med. 28 (suppl 1), 42–45.
Carlin, A. F., Uchiyama, S., Chang, Y. C., Lewis, A. L., Nizet, V., Varki, A. (2009). Molecular Mimicry of Host Sialylated Glycans Allows a Bacterial Pathogen to Engage Neutrophil Siglec-9 and Dampen the Innate Immune Response. Blood 113 (14), 3333–3336. doi: 10.1182/blood-2008-11-187302
Chin, K. C., Fitzhardinge, P. M. (1985). Sequelae of Early-Onset Group B Hemolytic Streptococcal Neonatal Meningitis. J. Pediatr. 106 (5), 819–822. doi: 10.1016/S0022-3476(85)80365-6
Choudhury, I. N., Chacko, A., Delbaz, A., Chen, M., Basu, S., St John, J. A., et al. (2021). Antimicrobial Responses of Peripheral and Central Nervous System Glia Against Staphylococcus Aureus. Sci. Rep. 11 (1), 10722. doi: 10.1038/s41598-021-90252-0
Creti, R., Imperi, M., Pataracchia, M., Alfarone, G., Recchia, S., Baldassarri, L. (2012). Identification and Molecular Characterization of a S. Agalactiae Strain Lacking the Capsular Locus. Eur. J. Clin. Microbiol. Infect. Dis. 31 (3), 233–235. doi: 10.1007/s10096-011-1298-7
Cumley, N. J., Smith, L. M., Anthony, M., May, R. C. (2012). The CovS/CovR Acid Response Regulator Is Required for Intracellular Survival of Group B Streptococcus in Macrophages. Infect. Immun. 80 (5), 1650–1661. doi: 10.1128/IAI.05443-11
Dando, S. J., Ipe, D. S., Batzloff, M., Sullivan, M. J., Crossman, D. K., Crowley, M., et al. (2016). Burkholderia Pseudomallei Capsule Exacerbates Respiratory Melioidosis But Does Not Afford Protection Against Antimicrobial Signaling or Bacterial Killing in Human Olfactory Ensheathing Cells. Infect. Immun. 84 (7), 1941–1956. doi: 10.1128/IAI.01546-15
Dando, S. J., Mackay-Sim, A., Norton, R., Currie, B. J., St John, J. A., Ekberg, J. A., et al. (2014). Pathogens Penetrating the Central Nervous System: Infection Pathways and the Cellular and Molecular Mechanisms of Invasion. Clin. Microbiol. Rev. 27 (4), 691–726. doi: 10.1128/CMR.00118-13
Delbaz, A., Chen, M., Jen, F. E., Schulz, B. L., Gorse, A. D., Jennings, M. P., et al. (2020). Neisseria Meningitidis Induces Pathology-Associated Cellular and Molecular Changes in Trigeminal Schwann Cells. Infect. Immun. 88 (4), e00955–19. doi: 10.1128/IAI.00955-19
Doll, J. R., Thompson, R. L., Sawtell, N. M. (2019). Infectious Herpes Simplex Virus in the Brain Stem Is Correlated With Reactivation in the Trigeminal Ganglia. J. Virol. 93 (8), e02209–e02218. doi: 10.1128/JVI.02209-18
Drevets, D. A., Bronze, M. S. (2008). Listeria Monocytogenes : Epidemiology, Human Disease, and Mechanisms of Brain Invasion. FEMS Immunol. Med. Microbiol. 53 (2), 151–165. doi: 10.1111/j.1574-695X.2008.00404.x
Edmond, K. M., Kortsalioudaki, C., Scott, S., Schrag, S. J., Zaidi, A. K., Cousens, S., et al. (2012). Group B Streptococcal Disease in Infants Aged Younger Than 3 Months: Systematic Review and Meta-Analysis. Lancet 379 (9815), 547–556. doi: 10.1016/S0140-6736(11)61651-6
Eskelinen, E.-L., Illert, A. L., Tanaka, Y., Schwarzmann, G., Blanz, J., Figura, K. V., et al. (2002). Role of LAMP-2 in Lysosome Biogenesis and Autophagy. Mol. Biol. Cell 13 (9), 3355–3368. doi: 10.1091/mbc.e02-02-0114
Filippidis, A., Fountas, K. N. (2009). Nasal Lymphatics as a Novel Invasion and Dissemination Route of Bacterial Meningitis. Med. Hypotheses 72 (6), 694–697. doi: 10.1016/j.mehy.2008.10.031
Foster-Nyarko, E., Kwambana, B., Aderonke, O., Ceesay, F., Jarju, S., Bojang, A., et al. (2016). Associations Between Nasopharyngeal Carriage of Group B Streptococcus and Other Respiratory Pathogens During Early Infancy. BMC Microbiol. 16, 97. doi: 10.1186/s12866-016-0714-7
Garges, H. P., Moody, M. A., Cotten, C. M., Smith, P. B., Tiffany, K. F., Lenfestey, R., et al. (2006). Neonatal Meningitis: What Is the Correlation Among Cerebrospinal Fluid Cultures, Blood Cultures, and Cerebrospinal Fluid Parameters? Pediatrics 117 (4), 1094–1100. doi: 10.1542/peds.2005-1132
Graves, D. T., Jiang, Y. (1995). Chemokines, a Family of Chemotactic Cytokines. Crit. Rev. Oral. Biol. Med. 6 (2), 109–118. doi: 10.1177/10454411950060020101
Graziadei, P. P., Monti Graziadei, G. A. (1985). Neurogenesis and Plasticity of the Olfactory Sensory Neurons. Ann. N Y Acad. Sci. 457, 127–142. doi: 10.1111/j.1749-6632.1985.tb20802.x
Gupta, R., Maraiyesa, T., Conry, B. (2018). Bilateral Haemorrhagic Basal Ganglia Infarction Associated With Early-Onset Group B Streptococcus Meningitis. BMJ Case Rep. 2018, bcr-2017-223085. doi: 10.1136/bcr-2017-223085
Hegg, C. C., Au, E., Roskams, A. J., Lucero, M. T. (2003). PACAP Is Present in the Olfactory System and Evokes Calcium Transients in Olfactory Receptor Neurons. J. Neurophysiol. 90 (4), 2711–2719. doi: 10.1152/jn.00288.2003
Herbert, R. P., Harris, J., Chong, K. P., Chapman, J., West, A. K., Chuah, M. I. (2012). Cytokines and Olfactory Bulb Microglia in Response to Bacterial Challenge in the Compromised Primary Olfactory Pathway. J. Neuroinflamm. 9, 109. doi: 10.1186/1742-2094-9-109
Horváth-Puhó, E., van Kassel, M. N., Gonçalves, B. P., de Gier, B., Procter, S. R., Paul, P., et al. (2021). Mortality, Neurodevelopmental Impairments, and Economic Outcomes After Invasive Group B Streptococcal Disease in Early Infancy in Denmark and the Netherlands: A National Matched Cohort Study. Lancet Child Adolesc. Health 5 (6), 398–407. doi: 10.1016/S2352-4642(21)00022-5
Huynh, K. K., Eskelinen, El, Scott, C. C., Malevanets, A., Saftig, P., Grinstein, S., et al. (2007). LAMP Proteins Are Required for Fusion of Lysosomes With Phagosomes. EMBO J. 26 (2), 313–324. doi: 10.1038/sj.emboj.7601511
Imbulana, D. I., Owen, L. S., Dawson, J. A., Davis, P. G., Manley, B. J. (2018). The Pronose Study: A Randomised Controlled Trial of a Nasal Barrier Dressing to Reduce Injury in Preterm Infants Receiving Binasal Non-Invasive Respiratory Support. J. Paediatrics Child Health 54 (S1), 82–83. doi: 10.1111/jpc.13882_219
Ippolito, D. L., James, W. A., Tinnemore, D., Huang, R. R., Dehart, M. J., Williams, J., et al. (2010). Group B Streptococcus Serotype Prevalence in Reproductive-Age Women at a Tertiary Care Military Medical Center Relative to Global Serotype Distribution. BMC Infect. Dis. 10, 336. doi: 10.1186/1471-2334-10-336
Jauneikaite, E., Kapatai, G., Davies, F., Gozar, I., Coelho, J., Bamford, K. B., et al. (2018). Serial Clustering of Late Onset Group B Streptococcal Infections in the Neonatal Unit - a Genomic Re-Evaluation of Causality. Clin. Infect. Dis. 67 (6), 854–860. doi: 10.1093/cid/ciy174
Jochems, S. P., de Ruiter, K., Solorzano, C., Voskamp, A., Mitsi, E., Nikolaou, E., et al. (2019). Innate and Adaptive Nasal Mucosal Immune Responses Following Experimental Human Pneumococcal Colonization. J. Clin. Invest. 130, 4523–4538. doi: 10.1172/JCI128865
Kanekar, S., Gandham, M., Lucero, M. T. (2010). PACAP Protects Against Tnfα-Induced Cell Death in Olfactory Epithelium and Olfactory Placodal Cell Lines. Mol. Cell Neurosci. 45 (4), 345–354. doi: 10.1016/j.mcn.2010.07.007
Landwehr-Kenzel, S., Henneke, P. (2014). Interaction of Streptococcus Agalactiae and Cellular Innate Immunity in Colonization and Disease. Front. Immunol. 5 (519). doi: 10.3389/fimmu.2014.00519
Le Doare, K., Kampmann, B. (2014). Breast Milk and Group B Streptococcal Infection: Vector of Transmission or Vehicle for Protection? Vaccine 32 (26), 3128–3132. doi: 10.1016/j.vaccine.2014.04.020
Lemire, P., Houde, M., Lecours, M.-P., Fittipaldi, N., Segura, M. (2012a). Role of Capsular Polysaccharide in Group B Streptococccus Interactions With Dendritic Cells. Microbes Infect 14 (12), 1064–1076. doi: 10.1016/j.micinf.2012.05.015
Lemire, P., Houde, M., Segura, M. (2012b). Encapsulated Group B Streptococcus Modulates Dendritic Cell Functions via Lipid Rafts and Clathrin-Mediated Endocytosis. Cell. Microbiol. 14 (11), 1707–1719. doi: 10.1111/j.1462-5822.2012.01830.x
Macedo-Ramos, H., Batista, A. F., Carrier-Ruiz, A., Alves, L., Allodi, S., Ribeiro-Resende, V. T., et al. (2014). Evidence of Involvement of the Mannose Receptor in the Internalization of Streptococcus Pneumoniae by Schwann Cells. BMC Microbiol. 14, 211. doi: 10.1186/s12866-014-0211-9
Macedo-Ramos, H., Campos, F. S., Carvalho, L. A., Ramos, I. B., Teixeira, L. M., De Souza, W., et al. (2011). Olfactory Ensheathing Cells as Putative Host Cells for Streptococcus Pneumoniae: Evidence of Bacterial Invasion via Mannose Receptor-Mediated Endocytosis. Neurosci. Res. 69 (4), 308–313. doi: 10.1016/j.neures.2010.12.015
Maisey, H. C., Doran, K. S., Nizet, V. (2008). Recent Advances in Understanding the Molecular Basis of Group B Streptococcus Virulence. Expert Rev. Mol. Med. 10, e27. doi: 10.1017/S1462399408000811
Melin, P. (2011). Neonatal Group B Streptococcal Disease: From Pathogenesis to Preventive Strategies. Clin. Microbiol. Infect. 17 (9), 1294–1303. doi: 10.1111/j.1469-0691.2011.03576.x
Miller, R. J., Rostene, W., Apartis, E., Banisadr, G., Biber, K., Milligan, E. D., et al. (2008). Chemokine Action in the Nervous System. J. Neurosci. 28 (46), 11792–11795. doi: 10.1523/JNEUROSCI.3588-08.2008
Morinis, J., Shah, J., Murthy, P., Fulford, M. (2011). Horizontal Transmission of Group B Streptococcus in a Neonatal Intensive Care Unit. Paediatr. Child Health 16 (6), e48–e50. doi: 10.1093/pch/16.6.e48
Mynarek, M., Bjellmo, S., Lydersen, S., Afset, J. E., Andersen, G. L., Vik, T. (2021). Incidence of Invasive Group B Streptococcal Infection and the Risk of Infant Death and Cerebral Palsy: A Norwegian Cohort Study. Pediatr. Res. 89 (6), 1541–1548. doi: 10.1038/s41390-020-1092-2
Nanduri, S. A., Petit, S., Smelser, C., Apostol, M., Alden, N. B., Harrison, L. H., et al. (2019). Epidemiology of Invasive Early-Onset and Late-Onset Group B Streptococcal Disease in the United Stateto 2015: Multistate Laboratory and Population-Based Surveillance. JAMA Pediatr. 173 (3), 224–233. doi: 10.1001/jamapediatrics.2018.4826
Nazareth, L., Chen, M., Shelper, T., Shah, M., Tello Velasquez, J., Walkden, H., et al. (2019). Novel Insights Into the Glia Limitans of the Olfactory Nervous System. J. Comp. Neurol. 527 (7), 1228–1244. doi: 10.1002/cne.24618
Nazareth, L., Lineburg, K. E., Chuah, M. I., Tello Velasquez, J., Chehrehasa, F., St John, J. A., et al. (2015). Olfactory Ensheathing Cells Are the Main Phagocytic Cells That Remove Axon Debris During Early Development of the Olfactory System. J. Comp. Neurol. 523 (3), 479–494. doi: 10.1002/cne.23694
Nazareth, L., Shelper, T. B., Chacko, A., Basu, S., Delbaz, A., Lee, J. Y. P., et al. (2020). Key Differences Between Olfactory Ensheathing Cells and Schwann Cells Regarding Phagocytosis of Necrotic Cells: Implications for Transplantation Therapies. Sci. Rep. 10 (1), 18936. doi: 10.1038/s41598-020-75850-8
Nazareth, L., Walkden, H., Chacko, A., Delbaz, A., Shelper, T., Armitage, C. W., et al. (2021). Chlamydia Muridarum Can Invade the Central Nervous System via the Olfactory and Trigeminal Nerves and Infect Peripheral Nerve Glial Cells. Front. Cell. Infect Microbiol. 10 (819). doi: 10.3389/fcimb.2020.607779
Olver, W. J., Bond, D. W., Boswell, T. C., Watkin, S. L. (2000). Neonatal Group B Streptococcal Disease Associated With Infected Breast Milk. Arch. Dis. Child Fetal Neonatal Ed 83 (1), F48–F49. doi: 10.1136/fn.83.1.F48
Pagelow, D., Chhatbar, C., Beineke, A., Liu, X., Nerlich, A., van Vorst, K., et al. (2018). The Olfactory Epithelium as a Port of Entry in Neonatal Neurolisteriosis. Nat. Commun. 9 (1), 4269. doi: 10.1038/s41467-018-06668-2
Panni, P., Ferguson, I. A., Beacham, I., Mackay-Sim, A., Ekberg, J. A. K., St John, J. A. (2013). Phagocytosis of Bacteria by Olfactory Ensheathing Cells and Schwann Cells. Neurosci. Lett. 539, 65–70. doi: 10.1016/j.neulet.2013.01.052
Patras, K. A., Nizet, V. (2018). Group B Streptococcal Maternal Colonization and Neonatal Disease: Molecular Mechanisms and Preventative Approaches. Front. Pediatr. 6 (27). doi: 10.3389/fped.2018.00027
Paveenkittiporn, W., Ungcharoen, R., Kerdsin, A. (2020). Streptococcus Agalactiae Infections and Clinical Relevance in Adults, Thailand. Diagn. Microbiol. Infect. Dis. 97 (1), 115005. doi: 10.1016/j.diagmicrobio.2020.115005
Petersen, S. M., Greisen, G., Krogfelt, K. A. (2016). Nasogastric Feeding Tubes From a Neonatal Department Yield High Concentrations of Potentially Pathogenic Bacteria- Even 1 D After Insertion. Pediatr. Res. 80 (3), 395–400. doi: 10.1038/pr.2016.86
Refolo, V., Stefanova, N. (2019). Neuroinflammation and Glial Phenotypic Changes in Alpha-Synucleinopathies. Front. Cell. Neurosci. 13 (263). doi: 10.3389/fncel.2019.00263
Riviere, G. R., Riviere, K. H., Smith, K. S. (2002). Molecular and Immunological Evidence of Oral Treponema in the Human Brain and Their Association With Alzheimer's Disease. Oral. Microbiol. Immunol. 17 (2), 113–118. doi: 10.1046/j.0902-0055.2001.00100.x
Roloff, K., Stepanyan, G., Valenzuela, G. (2018). Prevalence of Oropharyngeal Group B Streptococcus Colonization in Mothers, Family, and Health Care Providers. PloS One 13 (9), e0204617. doi: 10.1371/journal.pone.0204617
Ruiz-Mendoza, S., Macedo-Ramos, H., Santos, F. A., Quadros-de-Souza, L. C., Paiva, M. M., Pinto, T. C., et al. (2016). Streptococcus Pneumoniae Infection Regulates Expression of Neurotrophic Factors in the Olfactory Bulb and Cultured Olfactory Ensheathing Cells. Neuroscience 317, 149–161. doi: 10.1016/j.neuroscience.2016.01.016
Schaefer, M. L., Bottger, B., Silver, W. L., Finger, T. E. (2002). Trigeminal Collaterals in the Nasal Epithelium and Olfactory Bulb: A Potential Route for Direct Modulation of Olfactory Information by Trigeminal Stimuli. J. Comp. Neurol. 444 (3), 221–226. doi: 10.1002/cne.10143
Schildge, S., Bohrer, C., Beck, K., Schachtrup, C. (2013). Isolation and Culture of Mouse Cortical Astrocytes. J. Vis. Exp. 71, 50079. doi: 10.3791/50079
Shivkumar, M., Milho, R., May, J. S., Nicoll, M. P., Efstathiou, S., Stevenson, P. G. (2013). HSV 1 Targets the Murine Olfactory Neuroepithelium for Host Entry. J. Virol. 87 (19), 10477–10488. doi: 10.1128/JVI.01748-13
Sjolinder, H., Jonsson, A. B. (2010). Olfactory Nerve–a Novel Invasion Route of Neisseria Meningitidis to Reach the Meninges. PloS One 5 (11), e14034. doi: 10.1371/journal.pone.0014034
St John, J. A., Ekberg, J. A., Dando, S. J., Meedeniya, A. C., Horton, R. E., Batzloff, M., et al. (2014). Burkholderia Pseudomallei Penetrates the Brain via Destruction of the Olfactory and Trigeminal Nerves: Implications for the Pathogenesis of Neurological Melioidosis. MBio 5 (2), e00025. doi: 10.1128/mBio.00025-14
Stoner, T. D., Weston, T. A., Trejo, J., Doran, K. S. (2015). Group B Streptococcal Infection and Activation of Human Astrocytes. PloS One 10 (6), e0128431. doi: 10.1371/journal.pone.0128431
Sullivan, M. J., Forde, B. M., Prince, D. W., Ipe, D. S., Ben Zakour, N. L., Davies, M. R., et al. (2017). Complete Genome Sequence of Serotype III Streptococcus Agalactiae Sequence Type 17 Strain 874391. Genome Announc 5 (42), e01107–17. doi: 10.1128/genomeA.01107-17
Sullivan, M. J., Leclercq, S. Y., Ipe, D. S., Carey, A. J., Smith, J. P., Voller, N., et al. (2016). Effect of the Streptococcus Agalactiae Virulence Regulator CovR on the Pathogenesis of Urinary Tract Infection. J. Infect. Dis. 215 (3), 475–483. doi: 10.1093/infdis/jiw589
Takeshita, T., Yasui, M., Tomioka, M., Nakano, Y., Shimazaki, Y., Yamashita, Y. (2011). Enteral Tube Feeding Alters the Oral Indigenous Microbiota in Elderly Adults. Appl. Environ. Microbiol. 77 (19), 6739–6745. doi: 10.1128/AEM.00651-11
Upadhyay, U. D., Holbrook, E. H. (2004). Olfactory Loss as a Result of Toxic Exposure. Otolaryngol Clin. North Am. 37 (6), 1185–1207. doi: 10.1016/j.otc.2004.05.003
van Ginkel, F. W., McGhee, J. R., Watt, J. M., Campos-Torres, A., Parish, L. A., Briles, D. E. (2003). Pneumococcal Carriage Results in Ganglioside-Mediated Olfactory Tissue Infection. Proc. Natl. Acad. Sci. U.S.A. 100 (24), 14363–14367. doi: 10.1073/pnas.2235844100
Vincent, A. J., Choi-Lundberg, D. L., Harris, J. A., West, A. K., Chuah, M. I. (2007). Bacteria and PAMPs Activate Nuclear Factor kappaB and Gro Production in a Subset of Olfactory Ensheathing Cells and Astrocytes But Not in Schwann Cells. Glia 55 (9), 905–916. doi: 10.1002/glia.20512
Vincent, A. J., Taylor, J. M., Choi-Lundberg, D. L., West, A. K., Chuah, M. I. (2005). Genetic Expression Profile of Olfactory Ensheathing Cells is Distinct From That of Schwann Cells and Astrocytes. Glia 51 (2), 132–147. doi: 10.1002/glia.20195
Vuillemin, X., Hays, C., Plainvert, C., Dmytruk, N., Louis, M., Touak, G., et al. (2020). Invasive Group B Streptococcus Infections in Non-Pregnant Adults: A Retrospective Study, Franc-2019. Clin. Microbiol. Infect. 27 (1), 129.e1–129.e4. doi: 10.1016/j.cmi.2020.09.037
Walkden, H., Delbaz, A., Nazareth, L., Batzloff, M., Shelper, T., Beacham, I. R., et al. (2020). Burkholderia Pseudomallei Invades the Olfactory Nerve and Bulb After Epithelial Injury in Mice and Causes the Formation of Multinucleated Giant Glial Cells In Vitro. PloS Negl. Trop. Dis. 14 (1), e0008017. doi: 10.1371/journal.pntd.0008017
Wennekamp, J., Henneke, P. (2008). Induction and Termination of Inflammatory Signaling in Group B Streptococcal Sepsis. Immunol. Rev. 225, 114–127. doi: 10.1111/j.1600-065X.2008.00673.x
Windus, L. C., Claxton, C., Allen, C. L., Key, B., St John, J. A. (2007). Motile Membrane Protrusions Regulate Cell-Cell Adhesion and Migration of Olfactory Ensheathing Glia. Glia 55 (16), 1708–1719. doi: 10.1002/glia.20586
Xie, F., Zhou, X., Genter, M. B., Behr, M., Gu, J., Ding, X. (2011). The Tissue-Specific Toxicity of Methimazole in the Mouse Olfactory Mucosa Is Partly Mediated Through Target-Tissue Metabolic Activation by CYP2A5. Drug Metab. Disp: Biol. Fate Chemicals 39 (6), 947–951. doi: 10.1124/dmd.110.037895
Keywords: Streptococcus agalactiae, peripheral nerve, bacteria, olfactory ensheathing cell, Schwann cell, astrocyte, central nervous system
Citation: Chacko A, Delbaz A, Choudhury IN, Eindorf T, Shah M, Godfrey C, Sullivan MJ, St John JA, Ulett GC and Ekberg JAK (2022) Streptococcus agalactiae Infects Glial Cells and Invades the Central Nervous System via the Olfactory and Trigeminal Nerves. Front. Cell. Infect. Microbiol. 12:793416. doi: 10.3389/fcimb.2022.793416
Received: 12 October 2021; Accepted: 07 February 2022;
Published: 24 February 2022.
Edited by:
Francois Vandenesch, Université de Lyon, FranceReviewed by:
Asmaa Tazi, INSERM U1016 Institut Cochin, FranceSandrine Bourdoulous, INSERM U1016 Institut Cochin, France
Copyright © 2022 Chacko, Delbaz, Choudhury, Eindorf, Shah, Godfrey, Sullivan, St John, Ulett and Ekberg. This is an open-access article distributed under the terms of the Creative Commons Attribution License (CC BY). The use, distribution or reproduction in other forums is permitted, provided the original author(s) and the copyright owner(s) are credited and that the original publication in this journal is cited, in accordance with accepted academic practice. No use, distribution or reproduction is permitted which does not comply with these terms.
*Correspondence: Jenny A. K. Ekberg, ai5la2JlcmdAZ3JpZmZpdGguZWR1LmF1
†These authors share first authorship
‡These authors have contributed equally to this work and share senior authorship