- 1Department of Research, Shanghai University of Medicine and Health Sciences Affiliated Zhoupu Hospital; The College of Medical Technology, Shanghai University of Medicine and Health Sciences, Shanghai, China
- 2Key Laboratory of Medical Science and Laboratory Medicine, School of Medicine, Jiangsu University, Zhenjiang, China
- 3School of Pharmacy, Shanghai University of Medicine and Health Sciences, Shanghai, China
- 4Human and Animal Health Unit, Department of Epidemiology and Public Health, Swiss Tropical and Public Health Institute, Basel, Switzerland
The liver is directly connected to the intestines through the portal vein, which enables the gut microbiota and gut-derived products to influence liver health. There is accumulating evidence of decreased gut flora diversity and alcohol sensitivity in patients with various chronic liver diseases, including non-alcoholic/alcoholic liver disease, chronic hepatitis virus infection, primary sclerosing cholangitis and liver cirrhosis. Increased intestinal mucosal permeability and decline in barrier function were also found in these patients. Followed by bacteria translocation and endotoxin uptake, these will lead to systemic inflammation. Specific microbiota and microbiota-derived metabolites are altered in various chronic liver diseases studies, but the complex interaction between the gut microbiota and liver is missing. This review article discussed the bidirectional relationship between the gut and the liver, and explained the mechanisms of how the gut microbiota ecosystem alteration affects the pathogenesis of chronic liver diseases. We presented gut-microbiota targeted interventions that could be the new promising method to manage chronic liver diseases.
Introduction
Liver disease accounts for approximately 2 million deaths per year worldwide, liver disease is currently the 11th leading cause of mortality worldwide (Asrani et al., 2019), and it caused 4.6% of deaths in the Asia-Pacific region in 2015, compared with 2.7% of the USA (Younossi et al., 2020) and 2.1% in Europe (Sarin et al., 2020). Chronic liver disease includes chronic viral infection, alcoholic and non-alcoholic fatty liver diseases (ALD/NAFLD), non-alcoholic steatohepatitis (NASH), primary biliary cholangitis, cirrhosis, and hepatic encephalopathy (HE). Metabolic toxic, autoimmune, viral, and genetic disorders are clinical risk factors for chronic liver diseases (Rinella, 2015). Nutrition is also an vital factor for chronic liver disease (Johnson et al., 2013). For example, many patients who suffer from advanced liver disease have malnutrition problems as well. Malnutrition causes changes in the gut microbiota, leading to dysbiosis, and bacterial and/or pathogen-derived factors may be transferred from the gut to the liver. The gut microbiota contributes to the progression of chronic liver disease as well as hepatocellular carcinoma. The intestinal permeability changes are associated with gut microbiota (GM) involved in developing chronic liver disease (Milosevic et al., 2019). The gut microbiota closely interacts with a variety of immune cells in the liver (macrophages, Natural killer T cells, γδ T cells) (Yang et al., 2021). The pro-inflammatory factors generated from gut microbiota such as peptidoglycan and lipopolysaccharide (LTA) (Lebeer et al., 2010) induce excessive activation of immune cells and thus may aggravate the liver injury, inflammation, which worsen the chronic liver disease (Wheeler, 2003). On the contrary, metabolites from gut bacteria such as tryptophan metabolites, acetic acid, butyric acid, bile acids, phenolic compounds, and carotenoids may restore oxidative damage, inflammatory responses and lipogenesis in the liver tissue (Molinero et al., 2019). Many dietary components have the hepatoprotective function, the components could interact with gut microbiota and gut-derived metabolites, and improve intestinal mucosal immunity (Li et al., 2019), increase the beneficial bacteria composition, and reduce toxic metabolites release (Chen et al., 2014). Some natural products such as flaxseed oil (Zhang et al., 2017), brown algae Lessonia nigrescens (Zhao et al., 2017), and the herbal medicine Qushi Huayu decoction can revert gut dysbiosis and improve liver disease symptoms (Feng et al., 2017).
There is a close connection between the intestine and the liver through the gut microbiota and their metabolites, but the mechanism of how gut microbiota affect chronic liver diseases is still not clear. In this review article, we discussed in detail how the intestine and the liver communicate with each other through bile acid circulation and choline metabolites, elaborated the mechanism of the gut microbiota change that affects chronic liver disease. All relevant articles until May 2021 were included. The articles were searched through PubMed. We searched using terms including “gut microbiota”, “gut dysbiosis”, “liver” and “steatosis” as well as “non-alcoholic fatty liver disease”, “steatohepatitis”, “diets”, “probiotics” and “primary sclerosing cholangitis”. Over 100 articles were included, based on great deal of research works, we give a new promising approach that alters the structure of gut microbiota that could be used to manage the chronic liver disease.
Bi-Direction Communication Between the Gut and the Liver
The Association Between the Disorder of Intestinal Barrier Function and Liver Disease
Since the intestine and the liver have a close connection in anatomy and physiology, intestinal permeability and intestinal microbiota are closely connected to chronic liver disease (Chopyk and Grakoui, 2020). The rise in the incidence of liver diseases is a positive association with gastrointestinal and immune disorders (Brenner et al., 2015; Fukui, 2019). The intestinal barrier consists of physical, immune, and microbial components. The physical barrier is related to epithelium and mucus elements (Spadoni et al., 2017). A thick layer of mucus covered the whole intestinal epithelium, which contains mucin (MUCs), a highly glycosylated glycoprotein produced by goblet cells (Cornick et al., 2015). MUCs contain two types: the secreted mucins (MUC2) and membrane-bound mucins (MUC1, MUC3, MUC4) (Johansson et al., 2011). Mucus can act as a physical barrier to prevent pathogens invasion, they also can be used as a carbohydrates source for symbiotic bacteria. The most important function of mucus is immunity activity. Mucus contains many immunomodulatory molecules, such as anti-microbial peptides and immunoglobulins (Birchenough and Johansson, 2020). The thickness of the mucus layer also affects the survival and proliferation of bacteria (Schroeder, 2019). Bacteria also can affect mucus development in the intestine. In the first few weeks of germ-free mice experiencing bacterial colonization, the levels of IgA in the mucus were found to have a peak. The composition of bacteria in the intestine also has a significant alteration: the number of the Bacteroidetes increased while the Firmicutes reduced (Johansson et al., 2015). After five weeks of bacteria colonization, the mucus quality and microbiota composition of germ-free mice returned similar to that of wild-type mice, which indicates mucus could maintain the small intestine homeostasis.
Furthermore, pathogen recognition receptor toll-like receptors (TLRs) are important mediators between microorganisms and host. They play important role in mucus formation (Rakoff-Nahoum et al., 2004). Commensal microbes can provide continuous stimulation of TLRs on enterocytes to increase MUCs and anti-microbial peptides production, which maintain the tight junction of the epithelial barrier (Mukherjee and Hooper, 2015). Intestinal dysbiosis could increase intestinal permeability and disrupt the tight junction between intestinal epithelial cells. Microbial-derived metabolites, such as β-glucan, endotoxins (lipopolysaccharide, LPS) and bacterial viral RNAs, these molecules we called pathogen-associated molecular patterns (PAMPs). PAMPs can disturb the gut-liver axis, cause gut dysfunction and intestinal dysbiosis, which will cause intestinal permeability to increase in liver cirrhosis and small intestinal bacterial overgrowth (Anand et al., 2016; Fukui, 2021). Through the portal vein, blood flows from the intestine to the liver, PAMPs activate TLR4 on liver macrophages and hepatic immunity cells (Uesugi et al., 2001). The TLR4 signal in liver resident macrophages triggers a downstream inflammatory reaction to mediate the activation of TNF-α and IL-8 (Seki and Schnabl, 2012). The TLR4 signal also promotes fibrosis in hepatosplenic cells by downregulating the membrane-binding inhibitor homologous BAMBI (Lebeaupin et al., 2015), ultimately causing hepatic injury (Anand et al., 2016) (Figure 1).
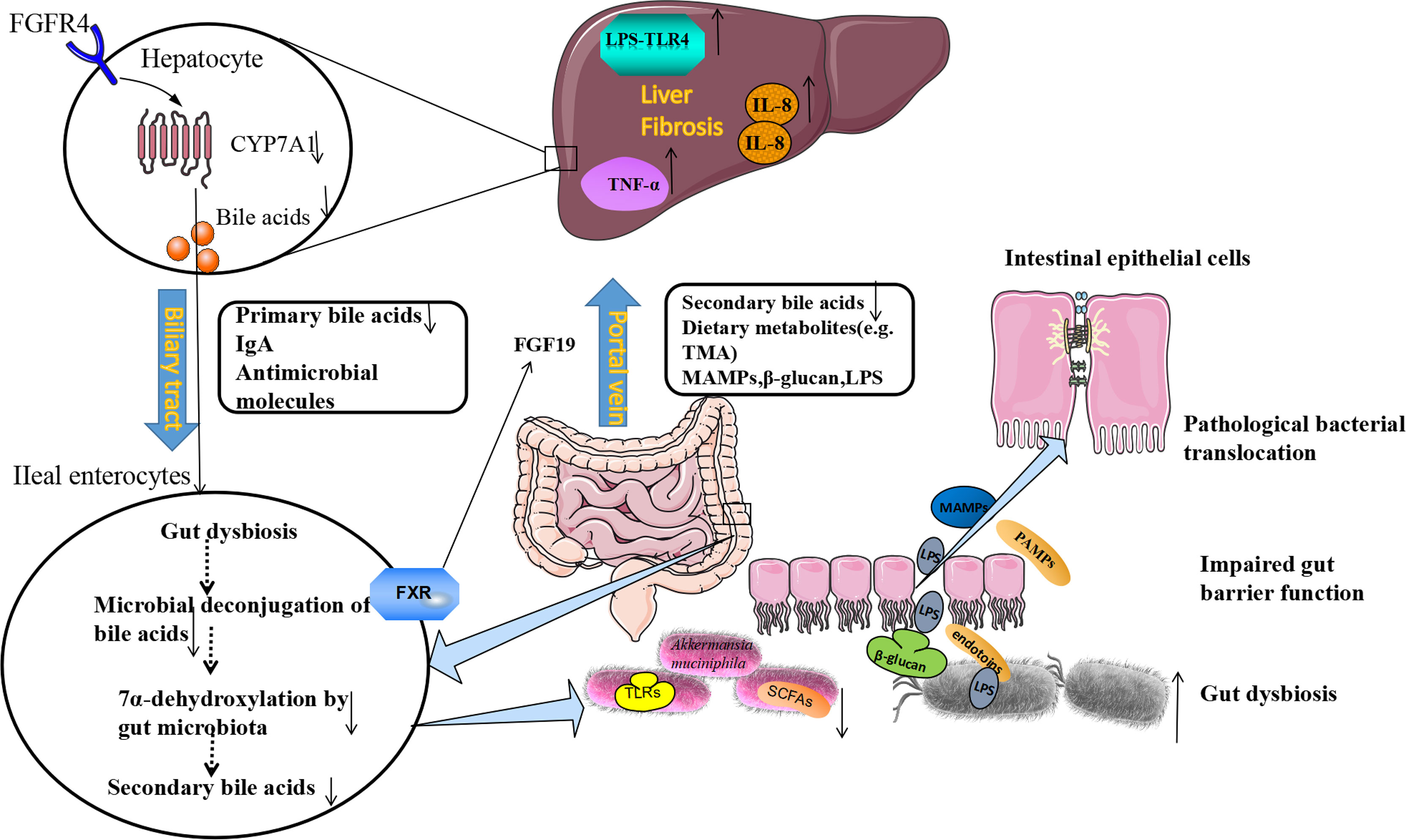
Figure 1 The Etiological Mechanism of bi-direction circulation between the intestine and the liver. The liver communicates with the gut through the portal veins, bile duct, and circulation system. Under pathological conditions of the liver, the gut microbiota dysbiosis could be found. The beneficial bacteria such as Akkermansia muciniphila decreased, and pathogenic bacteria such as Enterobacteriaceae increased. Following the production of SCFAs (butyrate) decreased, the production of antimicrobial molecules (IgA) reduced, tight junction integrity was disrupted, and intestinal permeability increased. LPS, β-glucan, and MAMPs moved from the gut lumen into the portal circulation, and translocate to the liver through portal veins. LPS activates pathogen recognition receptor TLR4 on liver resident macrophages Kupffer cells and hepatic stellate cells. The TLR4 signal in Kupffer cells triggers a downstream inflammatory cascade reaction to mediate the activation of TNF-α and IL-8. The TLR4 signal also promotes fibrosis in hepatocytes. On the other hand, fibrosis alters bile acid homeostasis and contributes to gut dysbiosis. Gut bacteria can express bile salt hydrolases to deconjugate primary bile acids. The 7α-dehydroxylase expressed by other bacteria can convert primary bile acids to secondary bile acids. In fibrosis, these bacteria decreased and reduced the conversion. Bile acids produced from the gut could bind to FXR, and produce FGF19, which reach the liver through the portal vein and down-regulates the synthesis of primary bile acids in hepatocytes by inhibiting cholesterol 7α-monooxygenase (CYP7A1) expression, further contributing to gut dysbiosis. lgA, immunoglobulin A; FXR, farnesoid X receptor; MAMPs, microbial-associated molecular patterns; SCFAs, short-chain free acids; LPS, lipopolysaccharide; TLR4, toll-like receptor 4; FGF19, fibroblast growth factor 19.
Intestine and Liver Communicates Through Bile Acids Enterohepatic Circulation
The liver communicates with the gut through the portal veins, bile duct, and circulation system (Stärkel and Schnabl, 2016). In the gut, the microbiota can metabolize the substance from diet and host metabolism, the gut microbiota metabolites can be translocated to the liver and affect liver functions (Tripathi et al., 2018). The liver can also release bioactive mediators to affect the gut system through the biliary tract and the circulation system, the most common mediators are bile acids (BAs) (Chiang, 2013). Bile acids are synthesized by the pericentral hepatocytes through the conversion of cholesterol, and primary BAs are conjugated with taurine and glycine in a ratio of 1:3 in humans which are released in the biliary tract (Wahlström et al., 2016). The enterohepatic circulation of BAs plays an important physiological role in the absorption of dietary nutrients, lipids, toxic metabolites, and exogenous substances excreted with bile digestion (Wahlström et al., 2016). After entering the intestinal tract, about 95% of the BAs are actively reabsorbed into epithelial cells of the terminal ileum and transported back to the liver through ileal bile acid transporter protein (IBAT). About 5% of BAs are metabolized by the gut microbiota to secondary bile acids, which increases the diversity and hydrophobicity of bile acids, and is beneficial for the excretion of bile acids (Arab et al., 2017). The lipid digestion needs a large number of bile acids, the liver can recycle and recover the BAs through enterohepatic circulation, which can reuse limited bile acids as well as exchange metabolites between the intestine and the liver (Arab et al., 2017).
By activating specific receptors expressed in different cell types can trigger the physiological action of BAs, and then the metabolism of organisms is regulated. FXR is the most important receptor involved in regulating the intestinal-hepatic circulation of BAs and BAs biosynthesis, and it is expressed in the liver, intestine, kidney, and other tissues (Zarrinpar and Loomba, 2012). FXR receptor is also an inhibitor of BAs synthesis in the liver, which regulates BAs metabolism in enterohepatic circulation and can effectively control BAs content in the liver and intestine. In the intestines, BAs stimulates the FXR receptor, activates the FXR-FGF15/19 signaling pathway, mediates the expression of fibroblast growth factors (Fibroblast Growth Factor, FGF15/19) in the epithelial cells of the intestine, and thus affects the secretion of the FGFR4 receptor (Fibroblast Growth Factor Receptors 4) (Copple and Li, 2016). FGF15/19 reaches the liver and down-regulates the synthesis of BAs in hepatocytes by inhibiting cholesterol 7α-monooxygenase (CYP7A1) (Sinal et al., 2000), forming a feedback system that regulates BAs production, as shown in Figure 1 (Broeders et al., 2015). This provides feedback that if sufficient bile acids are absorbed in the ileum, new bile acids synthesis in the liver is inhibited.
BAs regulates the ecosystem of gut microbiota and the interaction between the BAs and GM is a two-way direction (Ridlon et al., 2016). Studies have shown that bile ducts blockages prevent bile from flowing into the intestines, which will cause the small intestinal bacterial overgrowth and translocation, and this could be reversed by BAs administration. BAs binding to FXR could induce antimicrobial peptides (AMPS) production, which has a function in inhibiting the intestinal microbial overgrowth and intestinal barrier dysfunction (Jiang et al., 2015). Intestinal gut microbiota dysbiosis disrupts the balance between primary and secondary BAs (Ridlon et al., 2014). The imbalance between BAs and intestinal bacteria leads to a series of host immune responses related to liver disease progression, bile acids regulate specific host metabolic pathways and modulate the inflammatory responses through FXR and G protein-coupled bile acid receptor 1 (Marialena et al., 2016). Gut microbes can also use bile acids to regulate the aggregation of NKT cells in mouse livers (Ma et al., 2018).
Choline Metabolites Affect Liver Diseases
Choline, especially phosphatidylcholine, plays an important role in transporting lipids of the liver. In the absence of choline, fat accumulates in the liver, which results in NAFLD. It is worth noting that rodents fed with a choline-deficient diet are often being used as models of non-alcoholic steatohepatitis (NASH) (Valor et al., 2019). Choline is not only important for liver function but also protects brain memory, enhances the brain to concentrate, improves motor ability, and delays the aging of the brain (Derbyshire and Obeid, 2020). Choline can be converted to phosphatidylcholine (lecithin) by the host to assist the liver in excreting very-low-density lipoprotein (VLDL) particles (Mehedint and Zeisel, 2013). This process prevents triglycerides accumulation in the liver. However, gut microbiota such as Firmicutes and Proteobacteria can convert choline into trimethylamine (TMA); TMA can be transferred and converted to harmful metabolite trimethylamine N-oxide (TMAO) in the liver (Coutinho-Wolino et al., 2021). The importance of methylamine is increasingly recognized, involving the liver, heart metabolism, and neurological disorders (Chhibber-Goel et al., 2016). Increased systemic circulation of TMAO is accompanied by a decreased level of phosphatidylcholine produced by the host (Del Rio et al., 2017). This imbalance is characteristic of intestinal disorders of patients. In human and experimental NAFLD models, TMAO is associated with liver injury due to increased triglyceride accumulation/hepatic steatosis (Gogiashvili et al., 2016; Sherriff et al., 2016; Del Rio et al., 2017).
Free Fatty Acids and Their Roles on Liver Disease
Free fatty acids include short-chain fatty acids (SCFAs) and long-chain fatty acids (LCFAs). SCFAs mainly include acetate, propionate, and butyrate, which are produced during the bacterial fermentation of dietary fibers (Bergman, 1990). Butyrate could be used as the energy source of intestinal cells and helps to maintain the intestinal barrier functions (Hamer et al., 2010). Alcohol-induced liver injury is characterized by butyrate and propionate decreased, the level of acetate increased (Peng et al., 2014). Butyrate enhances the immune barrier of the intestinal mucosa, which prevents bacteria and their metabolites from entering the bloodstream and reducing the inflammatory response. Butyrate supplementation in glyceryl tributyl ester can reduce intestinal permeability disrupted by alcohol (Cresci et al., 2017). However, the mechanism through which tributyl glycerol protects the intestinal barrier remains unknown. LCFAs were also found closely connected with alcohol-induced liver injury. In the mice fed with an alcohol diet, the production of C15:0 and C17:0 was significantly decreased compared to the control fed with an isocaloric diet (Shi et al., 2015). In general, the level of total saturated LCFAs is positively correlated with the lumen abundance of Lactobacilli (known metabolizers of saturated LCFAs). Saturated LCFA can promote colonic motility in rats and increase stool frequency (Zhao et al., 2018). Lactobacillus rhamnosus administration can increase the levels of luminal LCFA, and enhance its probiotic effects (Shi et al., 2015).
Specific Gut Microbiota Altered in Different Types of Chronic Liver Diseases
Alterations of gut microbiota compositions in different types of chronic liver diseases were summarized in Table 1.
Non-Alcoholic Fatty Liver Disease
NAFLD is characterized by fat deposits in the liver and lipid metabolism disorders without significant alcohol consumption (Angulo, 2002). NAFLD can develop into non-alcoholic steatohepatitis (NASH), fibrosis, cirrhosis, and even hepatocellular carcinoma (HCC) (Sun et al., 2015). NAFLD has been considered as a multidirectional relationship with metabolic syndrome (Godoy-Matos et al., 2020). The prevalence of NAFLD ranges from 25% to 45%, and NAFLD is the most common cause of liver damage worldwide (Bellentani et al., 2010). According to GHE data in 2015, NAFLD accounted for 11.7% of all deaths from liver cirrhosis and other chronic liver diseases (Kuruvilla et al., 2016). Another study found that the prevalence of NAFLD was 46%, and biopsy confirmed the prevalence of NASH was as high as 12% (Williams et al., 2011). Lifestyles and dietary habits are closely related to the prevalence of NAFLD. There is increasing evidence that NAFLD patients also have gut microbiota dysbiosis problems. A few microbiotas could be used as biomarkers to distinguish healthy individuals from patients with NAFLD, NASH, or cirrhosis (Aron-Wisnewsky et al., 2020). Interestingly, it is the degree of cirrhosis, but not the NASH presence, which is related to intestinal leakage and intestinal bacterial overgrowth (Miele et al., 2009). The abundance of Bacteroides increased significantly and Firmicutes decreased in NASH groups when compared with the healthy control. Proteobacteria, Enterobacteriaceae, and Escherichia were significantly elevated in NASH. The possible mechanism of NASH is the intestinal alcohol-producing bacteria (e.g. Escherichia coli) increased, more alcohol could provide a continuous source of ROS to the liver, leading to liver inflammation. Recently, the high-alcohol-producing Klebsiella Pneumoniae (HiAlc Kpn) was found in the gut of NASH patients. About 60% of NAFLD patients in a Chinese cohort study are associated with HiAlc Kpn. The NAFLD mouse model induced by HiAlc Kpn was also successfully established. Through germ-free mice, fecal bacteria transplantation, bacteriophage, and antibiotic treatment experiments, it was proved that HiAlc Kpn in intestinal flora was a new cause of NAFLD liver disease, and NAFLD’s etiological theory of “endogenous alcoholic fatty liver disease” was put forward. HiAlc Kpn with high ethanol production colonized the intestinal tract and produced a large amount of ethanol, which was transferred into the liver through the portal system, causing dysfunction of the liver (Yuan et al., 2019). It is reported that the mice deficient in junctional adhesion molecule A (JAMA) suffered more severe fatty liver and steatohepatitis than control mice when treated mice with a high saturated fat diet. Antibiotics or sevelamer hydrochloride treatment can reduce the severity of fatty liver and steatohepatitis in JAMA deficient mice (Rahman et al., 2016).
Alcoholic Liver Disease
Alcohol-related liver disease (ALD) accounts for 0.9% of the total deaths worldwide, accounting for 47.9% of the incidence rate of cirrhosis (Pda et al., 2019). Drinking alcohol can increase the imbalance of intestinal bacteria and fungi, leading to the development of disease susceptibility, intestinal barrier function loss, and liver injury (Bajaj, 2019). Cho et al. reported that in ethanol-fed rodents, intestinal cell apoptosis increased with the degradation of tight junction protein (Cho et al., 2018). In addition, the levels of bacterial endotoxins in the blood of alcoholics, alcoholic hepatitis, and liver cirrhosis patients were higher than those of healthy people (Fukui et al., 1991). The gut-derived endotoxins contribute to increased intestinal permeability and alcohol-induced tissue injury (Schnabl and Brenner, 2014). Alcohol could disrupt the tight junctions of epithelial cells, which cause endotoxins translocation and the pathogenesis of ALD. The anti-microbial peptide could be used to treat ALD. When feeding the mice with alcohol, the anti-microbial peptide REG3G expression in the intestinal tissues was reduced (Wang et al., 2016). In addition, when the mice were gavaged with the engineered bacteria which overexpress interleukin 22 (IL22) to induce REG3G expression, the severity of the alcohol-induced liver injury can be reduced (Hendrikx et al., 2019).
The amount of Bacteroidetes and Firmicutes was reduced, while the Proteobacteria was increased in the colon samples of ALD patients (Mutlu et al., 2012). However, a higher abundance of Streptococci, Bifidobacteria, Enterobacteria, and decreased anti-inflammatory Clostridium leptum or Faecalibacterium prausnitziithan were found in feces of patients with severe alcohol-associated hepatitis than controls (Llopis et al., 2016). The dysbiosis of intestinal flora was observed in alcoholics, Enterobacteriaceae significantly increased and Bacteroidetes and Lactobacillus reduced (Mutlu et al., 2012). Either alcohol withdrawal or probiotic oral supplementation could reduce alcohol-induced dysbiosis (Leclercq et al., 2014). Among alcohol-related liver diseases, alcoholic hepatitis has high mortality almost 20% (Thursz et al., 2015). The gut microbiota composition between patients with severe alcoholic hepatitis (AH) and alcoholic patients without alcoholic hepatitis (non-AH) are significantly different. To study the GM function on the pathology of AH. GM was collected from AH and non-AH groups and transplanted into germ-free mice separately. In the following five weeks, mice were fed an alcohol-containing diet. The non-AH donor GM received group gained more weight than the severe AH donor group. Liver inflammation was more severe in the severe AH donor group (Jefferies et al., 2018). By gut microbiota sequence analysis, in both groups, the abundance of Bacteroides was dominant, but in severe AH mice, the number was more marked. Butyricimonas, Bilophila, Clostridium cluster XIVa and Alistipes were also more significantly abundant in the severe AH mice. Large amount of Parasutterella excrementihominis was found in non-AH mouse microbiota, but almost absent in severe AH donors, which suggest the bacteria may have a protective effect (Llopis et al., 2016).
Hepatitis Virus Infection
Hepatitis virus infections include chronic hepatitis B (HBV) and chronic hepatitis C (HCV) infection. The gut microbiome is involved in the progression of hepatitis virus-related liver disease. Gut microbiota alterations were significantly associated with liver disease progression. Compared to healthy controls, patients with HBV has a higher abundance of Veillonella, Fusobacteria, and Haemophilus, lower amount Prevotella and Phascolarctobacterium (Chen et al., 2020) Recent research showed that when comparing the stage 4 HCV patients to healthy controls, the amount of Bacteroidetes increased and Firmicutes has slightly decreased (at the phylum level), the abundance of Prevotella, Acinetobacter, Phascolarctobacterium, Veillonella, and Faecalibacterium increased, the genus of Ruminococcus, Clostridium and Bifidobacterium decreased (Aly et al., 2016).
Primary Sclerosing Cholangitis (PSC)
Primary sclerosing cholangitis is a chronic bile duct disease characterized by inflammation and bile ducts fibrosis, which leads to impaired bile formation or flow. Most patients eventually with a high rate of cirrhosis, portal hypertension, and liver dysfunction (Lazaridis and Larusso, 2016). Currently, there is no effective medical treatment for PSC, and liver transplantation is the only effective option with high cost. Recently, a lot of research has focused on the role of gut microbiota in PSC (Little et al., 2020). Sabino et al. found intestinal microbiota diversity of PSC was decreased, the number of Enterococcus, Fusobacterium, and Lactobacillus significantly increased (Sabino et al., 2016). Another study also found the bacterial diversity was reduced and the mucosa-associated bacteria (an uncultured Clostridiales II) was under- represented (Rossen et al., 2015). A further study concluded the number of Blautia and Barnesiellaceae were increased in the mucosal samples of PSC (Torres et al., 2016). The abundance of Veillonella has been proved to be associated with PSC (Iwasawa et al., 2017). Veilonella contains genes that can encode amine oxidase, this molecule has important effects on the liver. In various studies, Veilonella appears to also have pro-inflammatory features (Van den Bogert et al., 2014). A UK study showed that the increase of Escherichia, Megasphera, Lachnospiraceae and reduction in Roseburia and Prevotella, Bacteroides was almost disappeared in PSC patients (Quraishi et al., 2017). Rühlemann et al. demonstrated eight taxa enriched in PSC compared to healthy controls. An increase of Proteobacteria and Parabacteroides were newly identified in PSC, and they are bile-tolerant taxons, which are important in cholesterol and bile acid metabolism (Rühlemann et al., 2019). Patients with PSC have a signature characterized by several genera decrease and certain genera increase, these genera could be the biomarkers for PSC and can highly predict PSC (Table 1).
Cirrhosis
Cirrhosis is characterized by hepatocyte loss, fibrous scar thickening, and regenerative nodules (Bhat et al., 2016). NAFLD, ALD, primary sclerosing cholangitis, and hepatitis can progress to cirrhosis (Tsochatzis et al., 2014). Specific bacteria enrichment or decrease were correlated with the severity of cirrhosis (Qin et al., 2014). Spontaneous bacterial peritonitis or worsened liver dysfunction was often found in cirrhosis patients. Quin and colleagues assessed the most extensive metagenome communities of cirrhosis (Qin et al., 2014). They observed severe ecological dysbiosis, and over fifty percent of patients’ abundant taxonomically species were from oral, suggesting that the oral microbiome contributes to cirrhosis processes and severity. Many studies have proved that the fecal microbial community has significant alterations in cirrhosis patients (Bajaj and Khoruts, 2020; Zheng et al., 2020; Trebicka et al., 2021). The abundance of Fusobacteria and Proteobacteria increased whereas Bacteroidetes was significantly reduced in the cirrhosis patients (Chen et al., 2011). Another study showed Enterobacteriaceae and Enterococcus were significantly increased, the ratio of Bifidobacterium and Enterobacteriaceae were reduced in cirrhotic patients. The Bifidobacterium/Enterobacteriaceae (B/E) reflects the microbial colonization resistance in the intestine.Plasma endotoxin, IL-6, and fecal secretory IgA were negatively correlated with Bacteroides-Prevotella group and Eubacteria group. The plasma concentration of endotoxin and IL-6 significantly increased in cirrhotic patients (Liu et al., 2012). Bajaj et al. confirmed the association between GM and liver cirrhosis, which showed that GM diversity and symbiosis significantly improved in patients with severe liver cirrhosis after liver transplantation (Bajaj et al., 2017). However, Bacteroidetes, Lachnospiraceae, and Ruminococcaceae were reduced while Enterobacteriaceae, Alcaligenaceae, Streptococcaceae, Veillonellaceae, and Fusobacteriaceae were increased in cirrhosis patients when comparison with healthy controls (Bajaj et al., 2014b; Trebicka et al., 2021). These findings provide a new approach for the management of liver cirrhosis with an emphasis on gut microbiota regulation.
Hepatic Encephalopathy (HE)
Hepatic encephalopathy (HE), is a reversible neuropsychiatric impairment caused by impaired liver function. Studies have shown that the decrease of blood hypoxia peristalsis can cause intestinal flora imbalance, thus increasing the release of intestinal ammonia and endotoxin, and inducing hepatic encephalopathy. However, the types of intestinal microbiota involved in this process are not clear. Intestinal dysbiosis, inflammation, and oxidative stress could be observed in the pathogenesis process of HE (Rai et al., 2015). The most possible pathology of HE is the damaged liver cannot convert intestinal nitrogenous toxins into non-toxic substances and excrete them from the body. Other neurotoxins include methionine derivatives such as thiols, phenols and fatty acids, they may act synergistically with ammonia. Food and gut-derived substances such as pseudoneurotransmitters such as octopamine, benzodiazepine ligands and GABA, are elevated in HE and have been blamed for interfering with neurotransmission. However, their significance in HE pathogenesis is unclear. The excess ammonia in the intestine is transferred to the brain and causes brain damage. The urease-producing bacteria which can produce ammonia and endotoxins such as Proteus and Klebsiella are increased in the intestine (Milosevic et al., 2019), intestinal permeability also are increased and cause bacterial translocation, increase inflammatory response (Bajaj, 2014). Fecalibacterium, Blautia, Roseburia, and Dorea were associated with decreased inflammation and good cognition in both HE/non-HE. In contrast, genera over-represented in HE (Megasphaera, Enterococcus, and Burkholderia) were linked to poor cognition and inflammation (Bajaj et al., 2012). When compared to cirrhotic patients without HE, a higher proportion of Veillonellaceae was observed in HE, this bacteria was associated with increased inflammatory cytokines (IL-6, TNF-α, IL-2, and IL-13) and poor cognition. The enrichment of the Alcaligenaceae family is related to poor cognitive ability. Proteobacteria is an opportunistic pathogen that can degrade urea to ammonia, and this gives a reasonable explanation for cognitive loss (Bajaj, 2014). Therefore, it has been assumed that identifying specific fecal microbial characteristics could be used to predict the absence of minimal HE, instead of cognitive testing (Bajaj et al., 2019). These findings suggest that the microbiome composition of HE patients is closely related to cognition and inflammation.
Hepatocellular Carcinoma (HCC)
Hepatocellular carcinoma (HCC) is the third leading cause of high cancer mortality. Cirrhosis and viral hepatitis may progress to HCC (Cabrera and Nelson, 2010). It is predicted that more than one million individuals will be affected by liver cancer by 2025, HCC accounts for ~90% of liver cancer cases (Llovet et al., 2021). Experiments have provided evidence that gut microbiota plays a role in hepatocarcinogenesis (Yu and Schwabe, 2017). Dipito et al. found that TLR4 deletion can reduce the number and size of HCC, but cannot prevent the incidence of tumors (Dapito et al., 2012). Thus, the TLR4-LPS pathway is not required for HCC initiation but HCC promotion. When the gut barrier was disrupted, LPS from the gut promotes hepatocarcinogenesis through hepatic stellate cells, hepatocyte-tumor compartment, and Kupffer cells (Gupta et al., 2019). Another critical mechanism about the LPS–TLR4 axis promotes HCC formation is through NFκBmediated pathway. Activated TLR-4 further triggers inflammatory signaling pathways for NFκB that induce the production and release of inflammatory cytokines IL-1β and IL-18 (Yu et al., 2010). Moreover, LPS activates TLR4 in HCC cells can enhance their potential invasive ability and induce the transition of epithelial-mesenchymal (Yu and Schwabe, 2017).
Until now, studies conducted on the gut microbiome and different underlying liver cirrhosis, showing that at least some microbial changes have the same features to different aetiologies. Secretion of bile reduced and changes in intestinal secretion of anti-microbial peptides and IgA at end-stage of liver disease. The common alteration of gut microbiota composition in patients with liver cirrhosis includes enrichment of Veillonella or Streptococcus and decrease of order Clostridiales (Bajaj et al., 2014a). In addition, Escherichia coli were significantly increased in fecal samples of HCC patients compared to cirrhosis patients without HCC, indicating that hepatocarcinogenesis may attribute to the overgrowth of E. coli (Grt et al., 2016). The most common bacteria isolated from tongue swabs of patients with HCC are Oribacterium and Fusobacterium. On the other hand, decreases of Lactobacillus, Bifidobacterium and Enterococcus were observed in stool samples of HCC patients (Zhang et al., 2012). It was also reported deoxycholic acid (DCA) accelerates the HCC development in obese mice by inducing the senescence-related secretory phenotype (SASP) in HSCs (Yoshimoto et al., 2013). In addition to synergy with DCA, lipoteichoic acid enters the liver through TLR2 and up-regulates the expression of SASP and COX-2 in senescent HSCs (Loo et al., 2017). Both gut microbiome and their metabolites play a vital role in the process of HCC, microbiome-targeted therapeutic modalities for HCC aroused great interest from scholars, and we will discuss possible approaches in detail in the next paragraph.
Targeting Gut-Microbiota to Manage Chronic Liver Disease
Gut microbiota-related strategies to manage chronic liver disease were summarized in Table 2.
Diet
Many studies focus on dietary modification to optimize the structure of gut microbiota. Higher intakes of branched-chain amino acids (BCAAs, leucine, isoleucine and valine) and vegetable proteins have shown benefits in cirrhosis patients (Fukushima et al., 2003). Serum BCAAs normalization can promote protein synthesis, reduce nitrogen-containing products, and prevent pseudo-neurotransmitters formation, which are important for neurotransmitters’ development. BCAA-enriched diet can reduce the progression of liver failure in patients with advanced cirrhosis, which can reduce the severity and frequency of HE, and improve quality of life.
Vitamin and/or mineral deficiencies can occur in chronic liver disease. Thiamine supplementation should be considered in severely alcoholic patients (Satish and Shaik, 2021). Fat-soluble vitamin supplementation should be considered in conditions of cholestatic (Atarashi et al., 2017). Vitamin K supplementation is only to be considered at high risk of a hemorrhagic situation (Atarashi et al., 2017). Zinc and magnesium supplementation can indirectly improve nutrient intake and nutritional status (Garrett-Laster et al., 1984). Calcium and vitamin D supplementation could be considered in patients with cholestasis and osteoporosis (Francis, 2006). SCFAs regulate liver metabolism and immune function by inhibiting histone deacetylase or activating G-protein coupled receptors, including GPR41, GPR43, GPR109a, and OLFR78. In addition, acetate and propionate have anti-inflammatory properties and inhibit hepatic lipogenesis and lipid accumulation (Sahuri-Arisoylu et al., 2016). Butyrate can modulate the GM composition, increase the expression of glucagon-like peptide-1 receptors (GLP-1R), reduce inflammatory signals and liver oxide damage, ultimately attenuate steatohepatitis (Jin et al., 2015).
Indole can enhance epithelial cells’ tight junction, attenuate indicators of inflammation and intestinal injury. Recently, Beaumont et al. have proved that after feeding the mice with indole, the expression of tight junction protein was up-regulated, and the mice display resistance to liver inflammation (Beaumont et al., 2018). Choline deficiency in diet could lead to reversible hepatic steatosis. Choline has a function in removing fat from hepatocytes (Yu et al., 2016). Metagenomic analysis of the microbial communities in the intestinal tracts of 15 women showed that increased Gammaproteobacteria and decreased Erysipelotrichi can prevent steatosis with a choline-depleted diet (Spencer et al., 2011).
Antibiotics
Antibiotics can decrease the total number of gut microbiota, eliminate bacteria with a high ability to translocate and inhibit pro-inflammatory signals arising from the gut ecosystem (Zhang et al., 2015). Rifaximin could be used to treat HE in clinical trials (Kimer et al., 2014). It may increase the number of beneficial bacteria while decreasing the number of harmful bacteria, and also improve beneficial bacteria function. Rifaximin could reduce gut-derived toxins and reduce serum pro-inflammatory cytokines levels (Ponziani et al., 2016). It may also affect gut microbiota metabolites (Bajaj, 2016). The research tested the functions of rifaximin on cirrhotic patients with minimal HE. They analyzed both the structure of gut microbiota and the metabolome of serum and urine. The results showed that after eight weeks of rifaximin treatment, cognition and endotoxemia could be improved. The composition of gut microbiota didn’t change significantly after rifaximin treatment (Kaji et al., 2017), only a slight increase of Eubacteriaceae and reduction of Veillonellaceae were found in the study (Bajaj et al., 2013). However, the unsaturated and saturated fatty acids in the serum were significantly increased after rifaximin treatment. The results showed rifaximin does not change the overall composition and diversity of gut microbiota, it modifies the metabolites of the bacteria. Rifaximin can also increase the concentration of linoleic and arachidonic acids, which have brain beneficial functions (Tang, 2014).
Probiotics
Many studies have reported that probiotics have beneficial effects on liver health. Probiotics have functions in improving nutritional stations, repairing mucosal barrier, providing short-chain acids to prevent apoptosis, improving intestinal epithelial viability (Kanauchi et al., 1999). These functions prevent the disturbance of pathogens on tight junctions. Lactobacillus GG can improve intestinal oxidative stress, intestinal leakage, and liver injury in the alcoholic steatohepatitis rat model (Forsyth et al., 2009). In patients with alcoholic cirrhosis, probiotics Lactobacillus casei Shirota may restore phagocytosis of neutrophils by changing IL-10 secretion and TLR4 expression (Stadlbauer et al., 2008). The effectiveness of probiotics on liver cirrhosis can be divided into improving liver function and preventing pathogens infection, hepatic encephalopathy, and other complications. Intake of VSL#3 daily for six months significantly reduced the risk of hospitalization for HE and improved Child-Turcotte-Pugh (CTP) and model for end-stage liver disease scores (MELD) in patients with cirrhosis (Dhiman et al., 2014). The ability of probiotics to modulate GM has therapeutic potential. There is some evidence regarding probiotic usage to treat spontaneous bacterial peritonitis (SBP) and HE. Further research in evaluating gut microbiota and appropriately selected beneficial bacterial strains as treatment modalities should be undertaken (Forsyth et al., 2009).
Prebiotics
Prebiotics are non-digestible but fermentable food substances fermented by bacteria and help the intestine peristaltic and selectively stimulate the growth of gut bacteria. Some prebiotic foods, like lactulose and pectin, seemed to be promising therapeutic agents to treat liver diseases. Lactulose is an unabsorbable disaccharide, which can acidify the intestinal cavity, inhibit urease-producing bacteria, and limit the diffusion of ammonia into the blood (Phongsamran et al., 2010). The main treatment for HE patients is to target the intestine to reduce colonic bacterial generation and reduce blood ammonia. Pectin can restore the levels of Bacteroides and prevent liver damage in rodent models (Ferrere et al., 2017). A meta-analysis of 1309 NAFLD patients showed significant reductions in BMI, liver enzymes, serum cholesterol, and triglycerides after prebiotic treatment. This suggests that prebiotics may improve NAFLD by regulating intestinal flora homeostasis (Loman et al., 2018). Fructooligosaccharide (FOS) can promote fatty acid oxidation by up-regulating peroxidase expression, inhibiting the expression of SREBP-2 in the liver, and reducing the accumulation of cholesterol (Matsumoto et al., 2017). This showed oligosaccharides could reduce bacterial overgrowth and improve alcoholic steatohepatitis by restoring the expression of partial lectin protein reg3g. Lactulose is prebiotic which can promote the growth of Bifidobacterium and Lactobacillus. Fan et al. used lactulose to treat NASH mice. The results showed that alanine aminotransferase (ALT) and other liver inflammatory indexes in the lactulose treatment group were significantly reduced compared with the non-alcoholic steatohepatitis model group (P < 0.05), which were established by given high-fat diet, but the steatosis of liver cells was not significantly improved, suggesting that lactulose has a certain role in reducing liver inflammation, but it can’t improve hepatocyte steatosis (Fan et al., 2005).
Symbiotics
Symbiotics consist of a combination of probiotics and prebiotics. Symbiotics have the function of regulating the expression of intestinal flora and related functional genes. It can reduce the colitis reaction and liver inflammatory response, reduce the level of SCFA in feces, enhance tight junction of the intestinal barrier, and improve fatty degeneration of the liver as well as insulin resistance. Meanwhile, symbiotics can reduce the degree of liver fibrosis and LPS in mice. Clinical research indicated that after six months of intervention by Bifidobacterium longum and FOS in 66 NASH patients, serum AST, LPS, and inflammatory response transmitters (HOMA-IR), fat denaturation and NASH activity index were significantly reduced (Malaguarnera et al., 2012). Symbiotic treatment could be an ideal approach to treat liver diseases since they can protect against inflammation and hepatocyte damage through probiotics and prebiotics. The mechanism of symbiotic protection has not yet been fully clarified. Potential mechanisms of symbiotic effect on liver disease include gut microbiota structure and microbiota metabolite alteration, which have anti-inflammatory and immunomodulatory effects. There are no adverse effects that have been reported with this approach.
Fecal Microbiota Transplant (FMT)
Fecal microbiota transplantation (FMT) means transplanting bacteria from the feces of healthy individuals into the recipient through a series of routes (Xu et al., 2021). FMT can restore the health of intestinal flora, further reduce the transport of endogenous ethanol, endotoxin, and other metabolites to the liver, reduce the damage of metabolic substances to the liver, and also effectively reduce the inflammatory response and the expansion of liver cells. Compared with the oral probiotics method, FMT can significantly improve intestinal bacteria disorder, and FMT is the most effective method to restore intestinal microecological balance currently.
FMT has been studied more deeply in recent years. The possible mechanism of FMT involves establishing beneficial microbes in the gut and the production of anti-microbial substances. In Ferrer’s study, they transplanted fecal bacteria from alcohol resistant mice to alcohol sensitive receptor mice and found that FMT prevented alcohol-induced intestinal disorders and fatty hepatitis in the liver to some extent. The number of Bacteroides in alcohol-sensitive mice decreased, and the number of Actinobacteria and Firmicutes increased. Alcohol-sensitive mice had fifty percent fewer Bacteroides than alcohol-resistant mice (Ferrere et al., 2017). Zhou et al. used fecal bacteria transplantation to treat NAFLD mice caused by a high-fat diet. The results showed that fecal bacteria transplantation could improve intestinal flora disorder, enhance intestinal barrier function and reduce liver steatosis (Zhou et al., 2017). At present, FMT has successfully treated Clostridium difficile infection patients in clinical and promoted the recovery of intestinal ecological balance. Simultaneously, the clinical results showed that the improvement effect was better than that of standard antibiotics. A clinical trial showed that FMT showed good safety in 10 patients with primary sclerosing cholangitis complicated with IBD, and it could effectively reduce alkaline phosphatase in some patients. Liver enzymes, fecal microflora, and fecal metabonomics were analyzed at 1, 4, 8, 12, and 24 weeks after FMT. The mean baseline level of ALP was 489U/L. FMT rarely has adverse events. The ALP level of 3 patients (30%) decreased by more than 50%; The fecal flora diversity of all patients increased after transplantation, and the successful colonization of donor flora in patients was related to the decrease of ALP (Allegretti et al., 2019).
Therefore, FMT can be used as an effective method to treat and prevent chronic liver disease. The preliminary basic research shows that FMT can restore the probiotics in the intestinal ecosystem (Craven et al., 2020; Lechner et al., 2020; Liu et al., 2021). Recently one randomized, double-blind trial was performed, patients with ALD‐related cirrhosis were randomized to receive FMT from a donor enriched in Lachnospiraceae and Ruminococcaceae or one placebo. On day 15, craving, urinary ethyl glucuronide/creatinine, cognitive and psychosocial quality of life was largely reduced in 90% of the FMT group and 30% of the placebo group (P = 0.02), while serum IL-6 and LPS-binding protein were decreased and butyrate/isobutyrate was increased in FMT group compared with baseline, but not in the placebo group (Bajaj et al., 2020). Therefore, fecal bacteria transplantation could be a valuable and safe treatment for cirrhosis. The advantage of FMT is that it can rebulid the whole dysbiotic intestinal environment, but the best optimal route of administration, the duration of treatment and the durability of reaction still need to be determined. There is an urgent need for large-scale and high-quality research in this field in order to evaluate the most effective method to achieve rebiosis.
Conclusions
The gut microbiota plays an important role in the pathogenesis of chronic liver diseases, including NAFLD, ALD, Hepatitis Virus Infection, PCS, cirrhosis, and HE. The mechanism of connection between liver diseases and the intestine is poorly understood. Intestinal permeability is closely related to chronic liver disease. The increase of intestinal permeability will lead to the release of intestinal inflammation factors and cause flora dysbiosis, which contributes to liver disease development (Fukui, 2019). The liver can communicate with the gut through bile acids circulation. The binding of BAs to FXR can induce anti-microbial peptides to produce, which can inhibit gut microbial overgrowth and improve gut barrier function. Choline assists the liver in excreting VLDL particles, preventing the accumulation of triglycerides in hepatic steatosis. Intestinal bacteria can convert choline into TMA. TMA can be transferred to the liver and converted to TMAO, which is associated with liver injury. Gut microbiota also can produce SCFAs including butyrate, propionate, acetate, etc. Butyrate can reduce intestinal permeability and subsequent liver injury induced by alcohol. Since the gut microbiota places an important role in the progress of the chronic liver disease, we believe the gut-microbiota-targeted interventions could be used to regulate the intestinal flora community and manage chronic liver diseases.
However, a comprehensive understanding of the interactions between microbes and liver diseases is still not clear. Although animal models help to elucidate many important mechanistic pathways in the etiology of liver diseases. From animal models to human models requires well-designed, large-scale clinical trials spanning multiple disease aetiologies and patient characteristics. With the increasing recognition of the role of the microbiome in the development, prognosis, and treatment of liver diseases, we emphasize the need for a focus on the microbiome to effectively address the socioeconomic burden of this type of liver disease. Furthermore, it is critical to use animal models that mimic human disease as closely as possible.
Author Contributions
JL contributed to manuscript writing. DY, XW, QZ, and LN critically reviewed the manuscript. PA critically edited the English in the manuscript. LS supervised the whole process and reviewed the manuscript. All authors contributed to the article and approved the submitted version.
Funding
This research was financially supported by the Natural Science Foundation of Shanghai (20ZR1424600), the National Natural Science Foundation of China (81773616), Shanghai Excellent Technology Leader Program (17XD1423200), Shanghai Municipal Public Health System Construction Three-Year Action Plan (2020-2022) (GWV-10.1-XK01), National Natural Science Foundation of China (82100666), National Natural Science Foundation for Young Scientists of Jiangsu Province (BK20200906), and Shanghai Education Development Project for Industry-University-Research Practice (A3-0200-21-311007-34).
Conflict of Interest
The authors declare that the research was conducted in the absence of any commercial or financial relationships that could be construed as a potential conflict of interest.
Publisher’s Note
All claims expressed in this article are solely those of the authors and do not necessarily represent those of their affiliated organizations, or those of the publisher, the editors and the reviewers. Any product that may be evaluated in this article, or claim that may be made by its manufacturer, is not guaranteed or endorsed by the publisher.
References
Allegretti, J. R., Kassam, Z., Carrellas, M., Mullish, B. H., Marchesi, J. R., Pechlivanis, A., et al. (2019). Fecal Microbiota Transplantation in Patients With Primary Sclerosing Cholangitis: A Pilot Clinical Trial. Off. J. Am. Coll. Gastroenterology| ACG 114, 1071–1079. doi: 10.14309/ajg.0000000000000115
Aly, A. M., Adel, A., El-Gendy, A. O., Essam, T. M., Aziz, R. K. (2016). Gut Microbiome Alterations in Patients With Stage 4 Hepatitis C. Gut Pathog. 8, 42. doi: 10.1186/s13099-016-0124-2
Anand, G., Zarrinpar, A., Loomba, R. (2016). Targeting Dysbiosis for the Treatment of Liver Disease. Semin. Liver Dis. 36, 037–047. doi: 10.1055/s-0035-1571276
Angulo, P. (2002). Nonalcoholic Fatty Liver Disease. N. Engl. J. Med. 346, 1221–1231. doi: 10.1056/NEJMra011775
Arab, J. P., Karpen, S. J., Dawson, P. A., Arrese, M., Trauner, M. (2017). Bile Acids and Nonalcoholic Fatty Liver Disease: Molecular Insights and Therapeutic Perspectives. Hepatology 65, 350–362. doi: 10.1002/hep.28709
Aron-Wisnewsky, J., Vigliotti, C., Witjes, J., Le, P., Holleboom, A. G., Verheij, J., et al. (2020). Gut Microbiota and Human NAFLD: Disentangling Microbial Signatures From Metabolic Disorders. Nat. Rev. Gastroenterol. Hepatol. 17, 279–297. doi: 10.1038/s41575-020-0269-9
Asrani, S. K., Devarbhavi, H., Eaton, J., Kamath, P. S. (2019). Burden of Liver Diseases in the World. J. Hepatol. 70, 151–171. doi: 10.1016/j.jhep.2018.09.014
Atarashi, K., Suda, W., Luo, C., Kawaguchi, T., Motoo, I., Narushima, S., et al. (2017). Ectopic Colonization of Oral Bacteria in the Intestine Drives TH1 Cell Induction and Inflammation. Science 358, 359–365. doi: 10.1126/science.aan4526
Bajaj, J. S. (2014). The Role of Microbiota in Hepatic Encephalopathy. Gut Microbes 5, 397–403. doi: 10.4161/gmic.28684
Bajaj, J. (2016). Review Article: Potential Mechanisms of Action of Rifaximin in the Management of Hepatic Encephalopathy and Other Complications of Cirrhosis. Alimentary Pharmacol. Ther. 43, 11–26. doi: 10.1111/apt.13435
Bajaj, J. S. (2019). Alcohol, Liver Disease and the Gut Microbiota. Nat. Rev. Gastroenterol. Hepatol. 16, 235–246. doi: 10.1038/s41575-018-0099-1
Bajaj, J. S., Cox, I. J., Betrapally, N. S., Heuman, D. M., Gillevet, P. M. (2014a). Systems Biology Analysis of Omeprazole Therapy in Cirrhosis Demonstrates Significant Shifts in Gut Microbiota Composition and Function. Am. J. Physiol. Gastrointest Liver Physiol. 307, G951. doi: 10.1152/ajpgi.00268.2014
Bajaj, J. S., Fagan, A., Sikaroodi, M., White, M. B., Sterling, R. K., Gilles, H. C., et al. (2017) 23, 907–914. Liver Transplant Modulates Gut Microbial Dysbiosis and Cognitive Function in Cirrhosis. Liver Transplantation. doi: 10.1002/lt.24754
Bajaj, J. S., Fagan, A., White, M. B., Wade, J. B., Hylemon, P. B., Heuman, D. M., et al. (2019). Specific Gut and Salivary Microbiota Patterns Are Linked With Different Cognitive Testing Strategies in Minimal Hepatic Encephalopathy. Am. J. Gastroenterol. 114, 1080. doi: 10.14309/ajg.0000000000000102
Bajaj, J. S., Gavis, E. A., Fagan, A., Wade, J. B., Thacker, L. R., Fuchs, M., et al. (2020). A Randomized Clinical Trial of Fecal Microbiota Transplant for Alcohol Use Disorder. Hepatology 73, 1688–1700. doi: 10.1002/hep.31496
Bajaj, J. S., Heuman, D. M., Hylemon, P. B., Sanyal, A. J., White, M. B., Monteith, P., et al. (2014b). Altered Profile of Human Gut Microbiome Is Associated With Cirrhosis and Its Complications. J. Hepatol. 60, 940–947. doi: 10.1016/j.jhep.2013.12.019
Bajaj, J. S., Heuman, D. M., Sanyal, A. J., Hylemon, P. B., Sterling, R. K., Stravitz, R. T., et al. (2013). Modulation of the Metabiome by Rifaximin in Patients With Cirrhosis and Minimal Hepatic Encephalopathy. PloS One 8, e60042. doi: 10.1371/journal.pone.0060042
Bajaj, J. S., Hylemon, P. B., Ridlon, J. M., Heuman, D. M., Daita, K., White, M. B., et al. (2012). Colonic Mucosal Microbiome Differs From Stool Microbiome in Cirrhosis and Hepatic Encephalopathy and Is Linked to Cognition and Inflammation. Am. J. Physiology-Gastrointestinal Liver Physiol. 303, G675–G685. doi: 10.1152/ajpgi.00152.2012
Bajaj, J. S., Hylemon, P. B., Ridlon, J. M., Heuman, D. M., Daita, K., White, M. B., et al. (2016). Colonic Mucosal Microbiome Differs From Stool Microbiome in Cirrhosis and Hepatic Encephalopathy and Is Linked to Cognition and Inflammation. Am. J. Physiol. Gastrointest. Liver Physiol. 303, 675–685. doi: 10.1152/ajpgi.00152.2012
Bajaj, J. S., Khoruts, A. (2020). Microbiota Changes and Intestinal Microbiota Transplantation in Liver Diseases and Cirrhosis. J. Hepatol. 72, 1003–1027. doi: 10.1016/j.jhep.2020.01.017
Beaumont, M., Neyrinck, A. M., Olivares, M., Rodriguez, J., de Rocca Serra, A., Roumain, M., et al. (2018). The Gut Microbiota Metabolite Indole Alleviates Liver Inflammation in Mice. FASEB J. 32, 6681–6693. doi: 10.1096/fj.201800544
Bellentani, S., Scaglioni, F., Marino, M., Bedogni, G. (2010). Epidemiology of Non-Alcoholic Fatty Liver Disease. Dig. Dis. 28, 155–161. doi: 10.1159/000282080
Bergman, E. (1990). Energy Contributions of Volatile Fatty Acids From the Gastrointestinal Tract in Various Species. Physiol. Rev. 70, 567–590. doi: 10.1152/physrev.1990.70.2.567
Bhat, M., Arendt, B. M., Bhat, V., Renner, E. L., Allard, J. P. (2016). Implication of the Intestinal Microbiome in Complications of Cirrhosis. World J. Hepatol. 8, 1128. doi: 10.4254/wjh.v8.i27.1128
Birchenough, G., Johansson, M. (2020). Forming a Mucus Barrier Along the Colon. Science 370, 402–403. doi: 10.1126/science.abe7194
Brenner, D. A., Paik, Y. H., Schnabl, B. (2015). Role of Gut Microbiota in Liver Disease. J. Clin. Gastroenterol. 49, S25. doi: 10.1097/MCG.0000000000000391
Broeders, E., Nascimento, E., Havekes, B., Brans, B., Schrauwen, P. (2015). The Bile Acid Chenodeoxycholic Acid Increases Human Brown Adipose Tissue Activity. Cell Metab. 22, 418–426. doi: 10.1016/j.cmet.2015.07.002
Cabrera, R., Nelson, D. R. (2010). The Management of Hepatocellular Carcinoma. Alimentary Pharmacol. Ther. 31, 461–476. doi: 10.1111/j.1365-2036.2009.04200.x
Chen, H. J., Kang, S. P., Lee, I. J., Lin, Y. L. (2014). Glycyrrhetinic Acid Suppressed NF-κb Activation in TNF-α-Induced Hepatocytes. J. Agric. Food Chem. 62, 618–625. doi: 10.1021/jf405352g
Chen, Z., Xie, Y., Zhou, F., Zhang, B., Wu, J., Yang, L., et al. (2020). Featured Gut Microbiomes Associated With the Progression of Chronic Hepatitis B Disease. Front. Microbiol. 11, 383. doi: 10.3389/fmicb.2020.00383
Chen, Y., Yang, F., Lu, H., Wang, B., Li, L. (2011). Characterization of Fecal Microbial Communities in Patients With Liver Cirrhosis. Hepatology 54, 562–572. doi: 10.1002/hep.24423
Chhibber-Goel, J., Gaur, A., Singhal, V., Parakh, N., Bhargava, B., Sharma, A. (2016). The Complex Metabolism of Trimethylamine in Humans: Endogenous and Exogenous Sources. Expert Rev. Mol. Med. 18, e8. doi: 10.1017/erm.2016.6
Chiang, J. (2013). Bile Acid Metabolism and Signaling. Compr. Physiol. 3, 1191–1212. doi: 10.1002/cphy.c120023
Chopyk, D. M., Grakoui, A. (2020). Contribution of the Intestinal Microbiome and Gut Barrier to Hepatic Disorders. Gastroenterology 159, 849–863. doi: 10.1053/j.gastro.2020.04.077
Cho, Y.-E., Yu, L.-R., Abdelmegeed, M. A., Yoo, S.-H., Song, B.-J. (2018). Apoptosis of Enterocytes and Nitration of Junctional Complex Proteins Promote Alcohol-Induced Gut Leakiness and Liver Injury. J. Hepatol. 69, 142–153. doi: 10.1016/j.jhep.2018.02.005
Copple, B. L., Li, T. (2016). Pharmacology of Bile Acid Receptors: Evolution of Bile Acids From Simple Detergents to Complex Signaling Molecules. Pharmacol. Res. 104, 9–21. doi: 10.1016/j.phrs.2015.12.007
Cornick, S., Tawiah, A., Chadee, K. (2015). Roles and Regulation of the Mucus Barrier in the Gut. Tissue barriers 3, e982426. doi: 10.4161/21688370.2014.982426
Coutinho-Wolino, K. S., de F Cardozo, L. F., de Oliveira Leal, V., Mafra, D., Stockler-Pinto, M. B. (2021). Can Diet Modulate Trimethylamine N-Oxide (TMAO) Production? What Do We Know So Far? Eur. J. Nutr. 60, 3567–3584. doi: 10.1007/s00394-021-02491-6
Craven, L., Rahman, A., Parvathy, S. N., Beaton, M., Silverman, J., Qumosani, K., et al. (2020). Allogenic Fecal Microbiota Transplantation in Patients With Nonalcoholic Fatty Liver Disease Improves Abnormal Small Intestinal Permeability: A Randomized Control Trial. Am. J. Gastroenterol. 115, 1055–1065. doi: 10.14309/ajg.0000000000000661
Cresci, G. A., Glueck, B., McMullen, M. R., Xin, W., Allende, D., Nagy, L. E. (2017). Prophylactic Tributyrin Treatment Mitigates Chronic-Binge Ethanol-Induced Intestinal Barrier and Liver Injury. J. Gastroenterol. Hepatol. 32, 1587–1597. doi: 10.1111/jgh.13731
Dapito, D., Mencin, A., Gwak, G. Y., Pradere, J. P., Jang, M. K., Mederacke, I., et al. (2012). Promotion of Hepatocellular Carcinoma by the Intestinal Microbiota and TLR4. Cancer Cell 21, 504–516. doi: 10.1016/j.ccr.2012.02.007
Del Rio, D., Zimetti, F., Caffarra, P., Tassotti, M., Bernini, F., Brighenti, F., et al. (2017). The Gut Microbial Metabolite Trimethylamine-N-Oxide Is Present in Human Cerebrospinal Fluid. Nutrients 9, 1053. doi: 10.3390/nu9101053
Derbyshire, E., Obeid, R. (2020). Choline, Neurological Development and Brain Function: A Systematic Review Focusing on the First 1000 Days. Nutrients 12, 1731. doi: 10.3390/nu12061731
Dhiman, R. K., Rana, B., Agrawal, S., Garg, A., Chopra, M., Thumburu, K. K., et al. (2014). Probiotic VSL# 3 Reduces Liver Disease Severity and Hospitalization in Patients With Cirrhosis: A Randomized, Controlled Trial. Gastroenterology 147, 1327–1337.e1323. doi: 10.1053/j.gastro.2014.08.031
Duarte, S. M., Stefano, J. T., Oliveira, C. P. (2019). Microbiota and Nonalcoholic Fatty Liver Disease/Nonalcoholic Steatohepatitis (NAFLD/NASH). Ann. Hepatol. 18, 416–421. doi: 10.1016/j.aohep.2019.04.006
Fan, J.-G., Xu, Z.-J., Wang, G.-L. (2005). Effect of Lactulose on Establishment of a Rat Non-Alcoholic Steatohepatitis Model. World J. Gastroenterol. WJG 11, 5053. doi: 10.3748/wjg.v11.i32.5053
Feng, Q., Liu, W., Baker, S. S., Li, H., Chen, C., Liu, Q., et al. (2017). Multi-Targeting Therapeutic Mechanisms of the Chinese Herbal Medicine QHD in the Treatment of Non-Alcoholic Fatty Liver Disease. Oncotarget 8, 27820. doi: 10.18632/oncotarget.15482
Ferrere, G., Wrzosek, L., Cailleux, F., Turpin, W., Puchois, V., Spatz, M., et al. (2017). Fecal Microbiota Manipulation Prevents Dysbiosis and Alcohol-Induced Liver Injury in Mice. J. Hepatol. 66, 806–815. doi: 10.1016/j.jhep.2016.11.008
Forsyth, C. B., Farhadi, A., Jakate, S. M., Tang, Y., Shaikh, M., Keshavarzian, A. (2009). Lactobacillus GG Treatment Ameliorates Alcohol-Induced Intestinal Oxidative Stress, Gut Leakiness, and Liver Injury in a Rat Model of Alcoholic Steatohepatitis. Alcohol 43, 163–172. doi: 10.1016/j.alcohol.2008.12.009
Francis, R. M. (2006). Calcium, Vitamin D and Involutional Osteoporosis. Curr. Opin. Clin. Nutr. Metab. Care 9, 13–17. doi: 10.1097/01.mco.0000196140.95916.3a
Fukui, H. (2019). Role of Gut Dysbiosis in Liver Diseases: What Have We Learned So Far? Diseases 7, 58. doi: 10.3390/diseases7040058
Fukui, H. (2021). Leaky Gut and Gut-Liver Axis in Liver Cirrhosis: Clinical Studies Update. Gut Liver 15, 666. doi: 10.5009/gnl20032
Fukui, H., Brauner, B., Bode, J. C., Bode, C. (1991). Plasma Endotoxin Concentrations in Patients With Alcoholic and Non-Alcoholic Liver Disease: Reevaluation With an Improved Chromogenic Assay. J. Hepatol. 12, 162–169. doi: 10.1016/0168-8278(91)90933-3
Fukushima, H., Miwa, Y., Ida, E., Kuriyama, S., Toda, K., Shimomura, Y., et al. (2003). Nocturnal Branched-Chain Amino Acid Administration Improves Protein Metabolism in Patients With Liver Cirrhosis: Comparison With Daytime Administration. J. Parenter. Enteral. Nutr. 27, 315–322. doi: 10.1177/0148607103027005315
Garrett-Laster, M., Russell, R., Jacques, P. (1984). Impairment of Taste and Olfaction in Patients With Cirrhosis: The Role of Vitamin A. Hum. Nutr. Clin. Nutr. 38, 203–214.
Godoy-Matos, A. F., Silva Júnior, W. S., Valerio, C. M. (2020). NAFLD as a Continuum: From Obesity to Metabolic Syndrome and Diabetes. Diabetol. Metab. Syndrome 12, 1–20. doi: 10.1186/s13098-020-00570-y
Gogiashvili, M., Edlund, K., Gianmoena, K., Marchan, R., Cadenas, C. (2016). Metabolic Profiling of Ob/Ob Mouse Fatty Liver Using HR-MAS 1h-NMR Combined With Gene Expression Analysis Reveals Alterations in Betaine Metabolism and the Transsulfuration Pathway. Anal. Bioanal. Chem. 409, 1–16. doi: 10.1007/s00216-016-0100-1
Grt, M., Wronka, K. M., Krasnodbski, M., Masior, Lewandowski, Z., Kosińska, I., et al. (2016). Profile of Gut Microbiota Associated With the Presence of Hepatocellular Cancer in Patients With Liver Cirrhosis. Transplant. Proc. 48, 1687–1691. doi: 10.1016/j.transproceed.2016.01.077
Gupta, H., Youn, G. S., Shin, M. J., Suk, K. T. (2019). Role of Gut Microbiota in Hepatocarcinogenesis. Microorganisms 7, 121. doi: 10.3390/microorganisms7050121
Hamer, H. M., Jonkers, D., Venema, K., Vanhoutvin, S., Brummer, R. J. (2010). Review Article: The Role of Butyrate on Colonic Function. Alimentary Pharmacol. Ther. 27, 104–119. doi: 10.1111/j.1365-2036.2007.03562.x
Hendrikx, T., Duan, Y., Wang, Y., Oh, J.-H., Alexander, L. M., Huang, W., et al. (2019). Bacteria Engineered to Produce IL-22 in Intestine Induce Expression of REG3G to Reduce Ethanol-Induced Liver Disease in Mice. Gut 68, 1504–1515. doi: 10.1136/gutjnl-2018-317232
Iwasawa, K., Suda, W., Tsunoda, T., Oikawa-Kawamoto, M., Umetsu, S., Inui, A., et al. (2017). Characterisation of the Faecal Microbiota in Japanese Patients With Paediatric-Onset Primary Sclerosing Cholangitis. Gut 66, 1344–1346. doi: 10.1136/gutjnl-2016-312533
Jefferies, M., Rauff, B., Rashid, H., Lam, T., Rafiq, S. (2018). Update on Global Epidemiology of Viral Hepatitis and Preventive Strategies. World J. Clin. cases 6, 589. doi: 10.12998/wjcc.v6.i13.589
Jiang, C., Xie, C., Li, F., Zhang, L., Nichols, R. C., Krausz, K. W., et al. (2015). Intestinal Farnesoid X Receptor Signaling Promotes Nonalcoholic Fatty Liver Disease. J. Clin. Invest. 125, 386. doi: 10.1172/JCI76738
Jin, C. J., Sellmann, C., Engstler, A. J., Ziegenhardt, D., Bergheim, I. (2015). Supplementation of Sodium Butyrate Protects Mice From the Development of Non-Alcoholic Steatohepatitis (NASH). Br. J. Nutr. 114, 1745–1755. doi: 10.1017/S0007114515003621
Johansson, M., Ambort, D., Pelaseyed, T., Schütte, A., Gustafsson, J. K., Ermund, A., et al. (2011). Composition and Functional Role of the Mucus Layers in the Intestine. Cell. Mol. Life Sci. 68, p.3635–p.3641. doi: 10.1007/s00018-011-0822-3
Johansson, M., Jakobsson, H. E., Holmén-Larsson, J., Schütte, A., Hansson, G. C. (2015). Normalization of Host Intestinal Mucus Layers Requires Long-Term Microbial Colonization. Cell Host Microbe 18, 582–592. doi: 10.1016/j.chom.2015.10.007
Johnson, T. M., Overgard, E. B., Cohen, A. E., DiBaise, J. K. (2013). Nutrition Assessment and Management in Advanced Liver Disease. Nutr. Clin. Pract. 28, 15–29. doi: 10.1177/0884533612469027
Kaji, K., Takaya, H., Saikawa, S., Furukawa, M., Sato, S., Kawaratani, H., et al. (2017). Rifaximin Ameliorates Hepatic Encephalopathy and Endotoxemia Without Affecting the Gut Microbiome Diversity. World J. Gastroenterol. 23, 8355. doi: 10.3748/wjg.v23.i47.8355
Kanauchi, O., Fujiyama, Y., Mitsuyama, K., Araki, Y., Ishii, T., Nakamura, T., et al. (1999). Increased Growth of Bifidobacterium and Eubacterium by Germinated Barley Foodstuff, Accompanied by Enhanced Butyrate Production in Healthy Volunteers. Int. J. Mol. Med. 3, 175–184. doi: 10.3892/ijmm.3.2.175
Kuruvilla, S., Bustreo, F., Kuo, T., Mishra, C. K., Taylor, K., Fogstad, H. (2016). The Global Strategy for Women’s, Children’s and Adolescents’ Health (2016–2030): A Roadmap Based on Evidence and Country Experience. Bull World Health Organ. 94, 398. doi: 10.2471/BLT.16.170431
Kimer, N., Krag, A., Gluud, L. L. (2014). Safety, Efficacy, and Patient Acceptability of Rifaximin for Hepatic Encephalopathy. Patient Prefer. Adherence 8, 331. doi: 10.1002/hep.31708
Lazaridis, K. N., Larusso, N. F. (2016). Primary Sclerosing Cholangitis. N Engl. J. Med. 1, 1161–1170. doi: 10.1056/NEJMra1506330
Lebeaupin, C., Proics, E., De Bieville, C., Rousseau, D., Bonnafous, S., Patouraux, S., et al. (2015). ER Stress Induces NLRP3 Inflammasome Activation and Hepatocyte Death. Cell Death Dis. 6, e1879–e1879. doi: 10.1038/cddis.2015.248
Lebeer, S., Vanderleyden, J., Keersmaecker, S. D. (2010). Host Interactions of Probiotic Bacterial Surface Molecules: Comparison With Commensals and Pathogens. Nat. Rev. Microbiol. 8, 171–184. doi: 10.1038/nrmicro2297
Lechner, S., Yee, M., Limketkai, B. N., Pham, E. A. (2020). Fecal Microbiota Transplantation for Chronic Liver Diseases: Current Understanding and Future Direction. Dig. Dis. Sci. 65, 897–905. doi: 10.1007/s10620-020-06100-0
Leclercq, S., Matamoros, S., Cani, P. D., Neyrinck, A. M., Jamar, F., Stärkel, P., et al. (2014). Intestinal Permeability, Gut-Bacterial Dysbiosis, and Behavioral Markers of Alcohol-Dependence Severity. Proc. Natl. Acad. Sci. 111, E4485–E4493. doi: 10.1073/pnas.1415174111
Li, X., Sun, R., Liu, R. (2019). Natural Products in Licorice for the Therapy of Liver Diseases: Progress and Future Opportunities. Pharmacol. Res. 144, 210–226. doi: 10.1016/j.phrs.2019.04.025
Little, R., Wine, E., Kamath, B. M., Griffiths, A. M., Ricciuto, A. (2020). Gut Microbiome in Primary Sclerosing Cholangitis: A Review. World J. Gastroenterol. 26, 2768–2780. doi: 10.3748/wjg.v26.i21.2768
Liu, Y., Fan, L., Cheng, Z., Yu, L., Cong, S., Hu, Y., et al. (2021). Fecal Transplantation Alleviates Acute Liver Injury in Mice Through Regulating Treg/Th17 Cytokines Balance. Sci. Rep. 11, 1–10. doi: 10.1038/s41598-021-81263-y
Liu, J., Wu, D., Ahmed, A., Li, X., Ma, Y., Tang, L., et al. (2012). Comparison of the Gut Microbe Profiles and Numbers Between Patients With Liver Cirrhosis and Healthy Individuals. Curr. Microbiol. 65, 7–13. doi: 10.1007/s00284-012-0105-8
Llopis, M., Cassard, A., Wrzosek, L., Boschat, L., Bruneau, A., Ferrere, G., et al. (2016). Intestinal Microbiota Contributes to Individual Susceptibility to Alcoholic Liver Disease. Gut 65, 830–839. doi: 10.1136/gutjnl-2015-310585
Llovet, J. M., Kelley, R. K., Villanueva, A., Singal, A. G., Pikarsky, E., Roayaie, S., et al. (2021). Hepatocellular Carcinoma. Nat. Rev. Dis. Primers 7, 6. doi: 10.1038/s41572-020-00240-3
Loman, B. R., Hernández-Saavedra, D., An, R., Rector, R. S. (2018). Prebiotic and Probiotic Treatment of Nonalcoholic Fatty Liver Disease: A Systematic Review and Meta-Analysis. Nutr. Rev. 76, 822–839. doi: 10.1093/nutrit/nuy031
Loo, T. M., Kamachi, F., Watanabe, Y., Yoshimoto, S., Kanda, H., Arai, Y., et al. (2017). Gut Microbiota Promotes Obesity-Associated Liver Cancer Through PGE2-Mediated Suppression of Antitumor Immunity. Cancer Discov. 7, 522–538. doi: 10.1158/2159-8290.CD-16-0932
Ma, C., Han, M., Heinrich, B., Fu, Q., Zhang, Q., Sandhu, M., et al. (2018). Gut Microbiome–Mediated Bile Acid Metabolism Regulates Liver Cancer via NKT Cells. Science 360, eaan5931. doi: 10.1126/science.aan5931
Malaguarnera, M., Vacante, M., Antic, T., Giordano, M., Galvano, F. (2012). Bifidobacterium Longum With Fructo-Oligosaccharides in Patients With Non Alcoholic Steatohepatitis. Dig. Dis. Sci. 57, 545–553. doi: 10.1007/s10620-011-1887-4
Marialena, M., Wang, A. Y., Robert, B., Comelli, E. M., Arendt, B. M., Zhang, L., et al. (2016). Bile Acids and Dysbiosis in Non-Alcoholic Fatty Liver Disease. PloS One 11, e0151829. doi: 10.1007/s11154-019-09512-0
Matsumoto, K., Ichimura, M., Tsuneyama, K., Moritoki, Y., Kikuchi, K. (2017). Fructo-Oligosaccharides and Intestinal Barrier Function in a Methionine–Choline-Deficient Mouse Model of Nonalcoholic Steatohepatitis. PloS One 12, e0175406. doi: 10.1371/journal.pone.0175406
Mehedint, M. G., Zeisel, S. H. (2013). Choline's Role in Maintaining Liver Function: NewEvidence for Epigenetic Mechanisms. Curr. Opin. Clin. Nutr. Metab. Care. 16, 339–345. doi: 10.1097/MCO.0b013e3283600d46
Miele, L., Valenza, V., La Torre, G., Montalto, M., Cammarota, G., Ricci, R., et al. (2009). Increased Intestinal Permeability and Tight Junction Alterations in Nonalcoholic Fatty Liver Disease. Hepatology 49, 1877–1887. doi: 10.1002/hep.22848
Milosevic, I., Vujovic, A., Barac, A., Djelic, M., Korac, M., Radovanovic Spurnic, A., et al. (2019). Gut-Liver Axis, Gut Microbiota, and Its Modulation in the Management of Liver Diseases: A Review of the Literature. Int. J. Mol. Sci. 20, 395. doi: 10.3390/ijms20020395
Molinero, N., Ruiz, L., Sánchez, B., Margolles, A., Delgado, S. (2019). Intestinal Bacteria Interplay With Bile and Cholesterol Metabolism: Implications on Host Physiology. Front. Physiol. 10, 185. doi: 10.3389/fphys.2019.00185
Mukherjee, S., Hooper, L. (2015). Antimicrobial Defense of the Intestine. Immunity 42, 28–39. doi: 10.1016/j.immuni.2014.12.028
Mutlu, E. A., Gillevet, P. M., Rangwala, H., Sikaroodi, M., Naqvi, A., Engen, P. A., et al. (2012). Colonic Microbiome Is Altered in Alcoholism. Am. J. Physiology-Gastrointestinal Liver Physiol. 302, G966–G978. doi: 10.1152/ajpgi.00380.2011
Pda, A., Ctr, B., Aksm, C. (2019). Epidemiology of Alcohol Consumption and Societal Burden of Alcoholism and Alcoholic Liver Disease - ScienceDirect. Clin Liver Dis. 23, 39–50. doi: 10.1016/j.cld.2018.09.011
Peng, C., Torralba, M., Tan, J., Embree, M., Schnabl, B. (2014). Supplementation of Saturated Long-Chain Fatty Acids Maintains Intestinal Eubiosis and Reduces Ethanol-Induced Liver Injury in Mice. Gastroenterology 148, 203–214. doi: 10.1053/j.gastro.2014.09.014
Phongsamran, P. V., Kim, J. W., Abbott, J. C., Rosenblatt, A. (2010). Pharmacotherapy for Hepatic Encephalopathy. Drugs 70, 1131–1148. doi: 10.2165/10898630-000000000-00000
Ponziani, F. R., Scaldaferri, F., Petito, V., Sterbini, F. P., Pecere, S., Lopetuso, L. R., et al. (2016). The Role of Antibiotics in Gut Microbiota Modulation: The Eubiotic Effects of Rifaximin. Dig. Dis. 34, 269–278. doi: 10.1159/000443361
Qin, N., Yang, F., Li, A., Prifti, E., Chen, Y., Shao, L., et al. (2014). Alterations of the Human Gut Microbiome in Liver Cirrhosis. Nature 513, 59–64. doi: 10.1038/nature13568
Quraishi, M. N., Sergeant, M., Kay, G., Iqbal, T., Chan, J., Constantinidou, C., et al. (2017). The Gut-Adherent Microbiota of PSC–IBD Is Distinct to That of IBD. Gut 66, 386–388. doi: 10.1136/gutjnl-2016-311915
Rahman, K., Desai, C., Iyer, S. S., Thorn, N. E., Kumar, P., Liu, Y., et al. (2016). Loss of Junctional Adhesion Molecule a Promotes Severe Steatohepatitis in Mice on a Diet High in Saturated Fat, Fructose, and Cholesterol. Gastroenterology 151, 733–746.e712. doi: 10.1053/j.gastro.2016.06.022
Rai, R., Saraswat, V. A., Dhiman, R. K. (2015). Gut Microbiota: Its Role in Hepatic Encephalopathy. J. Clin. Exp. Hepatol. 5, S29–S36. doi: 10.1016/j.jceh.2014.12.003
Rakoff-Nahoum, S., Paglino, J., Eslami-Varzaneh, F., Edberg, S., Medzhitov, R. (2004). Recognition of Commensal Microflora by Toll-Like Receptors Is Required for Intestinal Homeostasis. Cell 118, 229–241. doi: 10.1016/j.cell.2004.07.002
Ridlon, J. M., Harris, S. C., Bhowmik, S., Kang, D. J., Hylemon, P. B. (2016). Consequences of Bile Salt Biotransformations by Intestinal Bacteria. Gut Microbes 7, 22–39. doi: 10.1080/19490976.2015.1127483
Ridlon, J. M., Kang, D. J., Hylemon, P. B., Bajaj, J. S. (2014). Bile Acids and the Gut Microbiome. Curr. Opin. Gastroenterol. 30, 332–338. doi: 10.1097/MOG.0000000000000057
Rinella, M. E. (2015). Nonalcoholic Fatty Liver Disease: A Systematic Review. Jama 313, 2263–2273. doi: 10.1001/jama.2015.5370
Rossen, N. G., Fuentes, S., Boonstra, K., D’Haens, G. R., Heilig, H. G., Zoetendal, E. G., et al. (2015). The Mucosa-Associated Microbiota of PSC Patients Is Characterized by Low Diversity and Low Abundance of Uncultured Clostridiales II. J. Crohn's Colitis 9, 342–348. doi: 10.1093/ecco-jcc/jju023
Rühlemann, M., Liwinski, T., Heinsen, F., Bang, C., Zenouzi, R., Kummen, M., et al. (2019). Consistent Alterations in Faecal Microbiomes of Patients With Primary Sclerosing Cholangitis Independent of Associated Colitis. Alimentary Pharmacol. Ther. 50, 580–589. doi: 10.1111/apt.15375
Sabino, J., Vieira-Silva, S., Machiels, K., Joossens, M., Falony, G., Ballet, V., et al. (2016). Primary Sclerosing Cholangitis Is Characterised by Intestinal Dysbiosis Independent From IBD. Gut 65, 1681–1689. doi: 10.1136/gutjnl-2015-311004
Sahuri-Arisoylu, M., Brody, L., Parkinson, J., Parkes, H., Navaratnam, N., Miller, A. D., et al. (2016). Reprogramming of Hepatic Fat Accumulation And'browning'of Adipose Tissue by the Short-Chain Fatty Acid Acetate. Int. J. Obes. 40, 955–963. doi: 10.1038/ijo.2016.23
Sarin, S. K., Kumar, M., Eslam, M., George, J., Al Mahtab, M., Akbar, S. M. F., et al. (2020). Liver Diseases in the Asia-Pacific Region: A Lancet Gastroenterology & Hepatology Commission. Lancet Gastroenterol. Hepatol. 5, 167–228. doi: 10.1016/S2468-1253(19)30342-5
Satish, O. S., Shaik, S. A. (2021). Thiamine Mediated Reversal of Left Ventricular Dysfunction in Patients With Alcoholic Cardiomyopathy. Indian Heart J. 73, 389–391. doi: 10.1016/j.ihj.2021.03.013
Schnabl, B., Brenner, D. A. (2014). Interactions Between the Intestinal Microbiome and Liver Diseases. Gastroenterology 146, 1513–1524. doi: 10.1053/j.gastro.2014.01.020
Schroeder, B. O. (2019). Fight Them or Feed Them: How the Intestinal Mucus Layer Manages the Gut Microbiota. Gastroenterol. Rep. 7, 3–12. doi: 10.1093/gastro/goy052
Seki, E., Schnabl, B. (2012). Role of Innate Immunity and the Microbiota in Liver Fibrosis: Crosstalk Between the Liver and Gut. J. Physiol. 590, 447–458. doi: 10.1113/jphysiol.2011.219691
Sherriff, J. L., O'Sullivan, T. A., Properzi, C., Oddo, J.-L., Adams, L. A. (2016). Choline, Its Potential Role in Nonalcoholic Fatty Liver Disease, and the Case for Human and Bacterial Genes. Adv. Nutr. 7, 5–13. doi: 10.3945/an.114.007955
Shi, X., Wei, X., Yin, X., Wang, Y., Zhang, M., Zhao, C., et al. (2015). Hepatic and Fecal Metabolomic Analysis of the Effects of Lactobacillus Rhamnosus GG on Alcoholic Fatty Liver Disease in Mice. J. Proteome Res. 14, 1174–1182. doi: 10.1021/pr501121c
Sinal, C. J., Tohkin, M., Miyata, M., Ward, J. M., Gonzalez, F. J. (2000). Targeted Disruption of the Nuclear Receptor FXR/BAR Impairs Bile Acid and Lipid Homeostasis. Cell 102, 731–744. doi: 10.1016/S0092-8674(00)00062-3
Spadoni, I., Fornasa, G., Rescigno, M. (2017). Organ-Specific Protection Mediated by Cooperation Between Vascular and Epithelial Barriers. Nat. Rev. Immunol. 17, 761–773. doi: 10.1038/nri.2017.100
Spencer, M. D., Hamp, T. J., Reid, R. W., Fischer, L. M., Zeisel, S. H., Fodor, A. A. (2011). Association Between Composition of the Human Gastrointestinal Microbiome and Development of Fatty Liver With Choline Deficiency. Gastroenterology 140, 976–986. doi: 10.1053/j.gastro.2010.11.049
Stadlbauer, V., Mookerjee, R. P., Hodges, S., Wright, G. A., Davies, N. A., Jalan, R. (2008). Effect of Probiotic Treatment on Deranged Neutrophil Function and Cytokine Responses in Patients With Compensated Alcoholic Cirrhosis. J. Hepatol. 48, 945–951. doi: 10.1016/j.jhep.2008.02.015
Stärkel, P., Schnabl, B. (2016). Bidirectional Communication Between Liver and Gut During Alcoholic Liver Disease. Semin. Liver Dis. 36, 331–339. doi: 10.1055/s-0036-1593882
Sun, C., Fan, J. G., Qiao, L. (2015). Potential Epigenetic Mechanism in Non-Alcoholic Fatty Liver Disease. Int. J. Mol. Sci. 16, 5161–5179. doi: 10.3390/ijms16035161
Tang, K. (2014). Protective Effect of Arachidonic Acid and Linoleic Acid on 1-Methyl-4-Phenylpyridinium-Induced Toxicity in PC12 Cells. Lipids Health Dis. 13, 197. doi: 10.1186/1476-511X-13-197
Thursz, M. R., Richardson, P., Allison, M., Austin, A., Forrest, E. H. (2015). Prednisolone or Pentoxifylline for Alcoholic Hepatitis. New Engl. J. Med. 372, 1619–1628. doi: 10.1056/NEJMoa1412278
Torres, J., Bao, X., Goel, A., Colombel, J. F., Pekow, J., Jabri, B., et al. (2016). The Features of Mucosa-Associated Microbiota in Primary Sclerosing Cholangitis. Alimentary Pharmacol. Ther. 43, 790–801. doi: 10.1111/apt.13552
Trebicka, J., Macnaughtan, J., Schnabl, B., Shawcross, D. L., Bajaj, J. S. (2021). The Microbiota in Cirrhosis and Its Role in Hepatic Decompensation. J. Hepatol. 75, S67–S81. doi: 10.1016/j.jhep.2020.11.013
Tripathi, A., Debelius, J., Brenner, D. A., Karin, M., Loomba, R., Schnabl, B., et al. (2018). The Gut–Liver Axis and the Intersection With the Microbiome. Nat. Rev. Gastroenterol. Hepatol. 15, 397–411. doi: 10.1038/s41575-018-0011-z
Tsochatzis, E. A., Bosch, J., Burroughs, A. K. (2014). Liver Cirrhosis. Lancet 383, 1749–1761. doi: 10.1016/S0140-6736(14)60121-5
Uesugi, T., Froh, M., Arteel, G. E., Bradford, B. U., Thurman, R. G. (2001). Toll-Like Receptor 4 Is Involved in the Mechanism of Early Alcohol-Induced Liver Injury in Mice. Hepatology 34, 101–108. doi: 10.1053/jhep.2001.25350
Valor, A., Romeu, E., Escobedo, G., Campos-Espinosa, A., Romero-Bello, I. I., Moreno-González, J., et al. (2019). Study of Methionine Choline Deficient Diet-Induced Steatosis in Mice Using Endogenous Fluorescence Spectroscopy. Molecules 24, 3150. doi: 10.3390/molecules24173150
Van den Bogert, B., Meijerink, M., Zoetendal, E. G., Wells, J. M., Kleerebezem, M. (2014). Immunomodulatory Properties of Streptococcus and Veillonella Isolates From the Human Small Intestine Microbiota. PloS One 9, e114277. doi: 10.1371/journal.pone.0114277
Wahlström, A., Sayin, S. I., Marschall, H.-U., Bäckhed, F. (2016). Intestinal Crosstalk Between Bile Acids and Microbiota and Its Impact on Host Metabolism. Cell Metab. 24, 41–50. doi: 10.1016/j.cmet.2016.05.005
Wang, L., Fouts, D., Strkel, P., Hartmann, P., Peng, C., Llorente, C., et al. (2016). Intestinal REG3 Lectins Protect Against Alcoholic Steatohepatitis by Reducing Mucosa-Associated Microbiota and Preventing Bacterial Translocation. Cell Host Microbe 19, 227–239. doi: 10.1016/j.chom.2016.01.003
Wheeler, M. D. (2003). Endotoxin and Kupffer Cell Activation in Alcoholic Liver Disease. Alcohol Res. Health 27, 300.
Williams, C. D., Stengel, J., Asike, M. I., Torres, D. M., Shaw, J., Contreras, M., et al. (2011). Prevalence of Nonalcoholic Fatty Liver Disease and Nonalcoholic Steatohepatitis Among a Largely Middle-Aged Population Utilizing Ultrasound and Liver Biopsy: A Prospective Study. Gastroenterology 140, 124–131. doi: 10.1053/j.gastro.2010.09.038
Xu, H. M., Huang, H. L., Zhou, Y. L., Zhao, H. L., Nie, Y. Q. (2021). Fecal Microbiota Transplantation: A New Therapeutic Attempt From the Gut to the Brain. Gastroenterol. Res. Pract. 2021, 6699268. doi: 10.1155/2021/6699268
Yang, X., Guo, Y., Chen, C., Shao, B., Zhao, L., Zhou, Q., et al. (2021). Interaction Between Intestinal Microbiota and Tumour Immunity in the Tumour Microenvironment. Immunology 164, 476–493. doi: 10.1111/imm.13397
Yoshimoto, S., Loo, T. M., Atarashi, K., Kanda, H., Sato, S., Oyadomari, S., et al. (2013). Obesity-Induced Gut Microbial Metabolite Promotes Liver Cancer Through Senescence Secretome. Nature 499, 97–101. doi: 10.1038/nature12347
Younossi, Z. M., Stepanova, M., Younossi, Y., Golabi, P., Mishra, A., Rafiq, N., et al. (2020). Epidemiology of Chronic Liver Diseases in the USA in the Past Three Decades. Gut 69, 564–568. doi: 10.1136/gutjnl-2019-318813
Yuan, J., Chen, C., Cui, J., Lu, J., Liu, D. (2019). Fatty Liver Disease Caused by High-Alcohol-Producing Klebsiella Pneumoniae. Cell Metab. 30, 675–688.e677. doi: 10.1016/j.cmet.2019.08.018
Yu, J., Marsh, S., Hu, J., Feng, W., Wu, C. (2016). The Pathogenesis of Nonalcoholic Fatty Liver Disease: Interplay Between Diet, Gut Microbiota, and Genetic Background. Gastroenterol. Res. Pract. 2016, 2862173. doi: 10.1155/2016/2862173
Yu, L. X., Schwabe, R. F. (2017). The Gut Microbiome and Liver Cancer: Mechanisms and Clinical Translation. Nat. Rev. Gastroenterol. Hepatology. 14, 527–539. doi: 10.1038/nrgastro.2017.72
Yu, L., Yan, H., Liu, Q., Yang, W., Wu, H., Wei, D., et al. (2010). Endotoxin Accumulation Prevents Carcinogen-Induced Apoptosis and Promotes Liver Tumorigenesis in Rodents. Hepatology 52, 1322–1333. doi: 10.1002/hep.23845
Zarrinpar, A., Loomba, R. (2012). Review Article: The Emerging Interplay Among the Gastrointestinal Tract, Bile Acids and Incretins in the Pathogenesis of Diabetes and Non-Alcoholic Fatty Liver Disease. Alimentary Pharmacol. Ther. 36, 909–921. doi: 10.1111/apt.12084
Zhang, Y. J., Li, S., Gan, R. Y., Zhou, T., Xu, D. P., Li, H. B. (2015). Impacts of Gut Bacteria on Human Health and Diseases. Int. J. Mol. Sci. 16, 7493. doi: 10.3390/ijms16047493
Zhang, X., Wang, H., Yin, P., Fan, H., Sun, L., Liu, Y. (2017). Flaxseed Oil Ameliorates Alcoholic Liver Disease via Anti-Inflammation and Modulating Gut Microbiota in Mice. Lipids Health Dis. 16, 44. doi: 10.1186/s12944-017-0431-8
Zhang, H. L., Yu, L. X., Yang, W., Tang, L., Lin, Y., Wu, H., et al. (2012). Profound Impact of Gut Homeostasis on Chemically-Induced Pro-Tumorigenic Inflammation and Hepatocarcinogenesis in Rats. J. Hepatol. 57, 803–812. doi: 10.1016/j.jhep.2012.06.011
Zhao, L., Huang, Y., Lin, L., Yang, W., Tao, H., Lin, Z., et al. (2018). Saturated Long-Chain Fatty Acid-Producing Bacteria Contribute to Enhanced Colonic Motility in Rats. Microbiome 6, 107. doi: 10.1186/s40168-018-0492-6
Zhao, C., Yang, C., Chen, M., Lv, X., Liu, B., Yi, L., et al. (2017). Regulatory Efficacy of Brown Seaweed Lessonia Nigrescens Extract on the Gene Expression Profile and Intestinal Microflora in Type 2 Diabetic Mice. Mol. Nutr. Food Res. 1700730. doi: 10.1002/mnfr.201700730
Zheng, R., Wang, G., Pang, Z., Ran, N., Gu, Y., Guan, X., et al. (2020). Liver Cirrhosis Contributes to the Disorder of Gut Microbiota in Patients With Hepatocellular Carcinoma. Cancer Med. 9, 4232–4250. doi: 10.1002/cam4.3045
Keywords: gut microbiota, chronic liver diseases, bile acids, choline, probiotics, prebiotics
Citation: Liu J, Yang D, Wang X, Asare PT, Zhang Q, Na L and Shao L (2022) Gut Microbiota Targeted Approach in the Management of Chronic Liver Diseases. Front. Cell. Infect. Microbiol. 12:774335. doi: 10.3389/fcimb.2022.774335
Received: 11 September 2021; Accepted: 21 February 2022;
Published: 04 April 2022.
Edited by:
Jing Yuan, Children’s Hospital of Capital Institute of Pediatrics, ChinaReviewed by:
Hiroshi Fukui, Nara Medical University, JapanMin Wang, Stanford University, United States
Mogana Rajagopal, UCSI University, Malaysia
Copyright © 2022 Liu, Yang, Wang, Asare, Zhang, Na and Shao. This is an open-access article distributed under the terms of the Creative Commons Attribution License (CC BY). The use, distribution or reproduction in other forums is permitted, provided the original author(s) and the copyright owner(s) are credited and that the original publication in this journal is cited, in accordance with accepted academic practice. No use, distribution or reproduction is permitted which does not comply with these terms.
*Correspondence: Lei Shao, ZmVpaHVzbEAxNjMuY29t
†These authors have contributed equally to this work