Corrigendum: Antagonistic Coevolution Limits the Range of Host Defense in C. elegans Populations
- 1Department of Biology, Emory University, Atlanta, GA, United States
- 2Population Biology, Ecology, and Evolution Graduate Program, Emory University, Atlanta, GA, United States
- 3Department of Environmental Sciences, Emory University, Atlanta, GA, United States
Host populations often evolve defenses against parasites due to the significant fitness costs imposed by infection. However, adaptation to a specific parasite may alter the effectiveness of the host’s defenses in general. Consequently, the specificity of host defense may be influenced by a host population’s evolutionary history with parasites. Further, the degree of reciprocal change within an interaction may profoundly alter the range of host defense, given that antagonistic coevolutionary interactions are predicted to favor defense against specific parasite genotypes. Here, we examined the effect of host evolutionary history on host defense range by assessing the mortality rates of Caenorhabditis elegans host populations exposed to an array of Serratia marcescens bacterial parasite strains. Importantly, each of the host populations were derived from the same genetic background but have different experimental evolution histories with parasites. Each of these histories (exposure to either heat-killed, fixed genotype, or coevolving parasites) carries a different level of evolutionary reciprocity. Overall, we observed an effect of host evolutionary history in that previously coevolved host populations were generally the most susceptible to novel parasite strains. This data demonstrates that host evolutionary history can have a significant impact on host defense, and that host-parasite coevolution can increase host susceptibility to novel parasites.
Introduction
Parasites are ubiquitous in nature and are thought to be a key factor in the evolution and maintenance of genetic diversity within host populations (Hamilton, 1980; Anderson and May, 1982; Rainey et al., 2000; Thomson, 2009). Parasites can impose strong selective pressure on host populations, due to the fitness advantage experienced by uninfected or tolerant individuals, and thus select for the evolution of elevated host defense over time. Generally, natural observations have aligned with expectations, as hosts have evolved a multitude of strategies for defending against infection (Roy and Kirchner, 2000; Ellis, 2001; Weiss et al., 2014; Lemaitre and Hoffmann, 2007; Diamond et al., 2009; Parker et al., 2011; War et al., 2012; De Roode et al., 2013). These observations have been further supported by experimental studies, which have demonstrated the ability of hosts to evolve defense against novel parasites in experiments across various systems. Some of these systems include beetles (Bérénos et al., 2009), birds (Bonneaud et al., 2011), Daphnia (Duncan and Little, 2007), Drosophila (Kraaijeveld and Godfray, 1997), isopods (Hasu et al., 2009), moths (Fuxa and Richter, 1989; Boots and Begon, 1993), nematodes (Schulte et al., 2010; Penley et al., 2017), paramecium (Lohse et al., 2006) and snails (Webster and Woolhouse, 1999; Koskella and Lively, 2007). Despite the benefit of evolved host defenses and the ubiquity of parasites, natural populations experience considerable variance in levels of host defense over space and time (Allen et al., 2004; Laine, 2004).
Several mechanisms have been proposed to explain the widespread observations of variance in host defense (Koskella, 2018), including the costs associated with maintaining defenses (Sheldon and Verhulst, 1996; Strauss et al., 2002; Lenski, 2007; Graham et al., 2010; Melnyk et al., 2015; Cipollini et al., 2017) and parasite reciprocal adaptation (Ebert and Hamilton, 1996; Carius et al., 2001; Schulte et al., 2010) as mechanisms with strong support. Another potential factor that may contribute to the temporal and spatial variance in host defense is the evolutionary history of host populations. Host defenses exist on a spectrum ranging from more general, and effective against a broad range of parasites, to more specific and tailored to a particular parasite genotype. A host population’s evolutionary history with parasites may determine the degree to which broad or specific defenses are evolved or maintained. In particular, the evolution of highly specific host defenses may inhibit, limit, or alter the evolution and maintenance of more general defenses. Coevolutionary interactions can drive the evolution of highly specific host defenses and parasite infection strategies via reciprocal adaptation. Such specificity between host and parasite populations is known as local adaptation (Gandon and Zandt, 1998) and has been observed in natural and experimental parasite populations across various systems (Edmunds and Alstad, 1978; Ebert and Hamilton, 1996; Lively and Dybdahl, 2000; Greischar and Koskella, 2007; Hoeksema and Forde, 2008; Leimu and Fischer, 2008; Vos et al., 2009; Morran et al., 2014; Bellis et al., 2021). While local adaptation is more often observed in parasite populations, host populations are capable of exhibiting local adaptation (Gandon et al., 1996; Kawecki and Ebert, 2004). Importantly, host populations that reciprocally evolve in response to locally adapted parasites may also exhibit a degree of specificity in their defense (Aiba et al., 2010; Kniskern et al., 2011; Lemoine et al., 2012). This specificity in host defense may come at a cost and ultimately increase a host population’s susceptibility to novel parasites. Therefore, a host population’s evolutionary history with parasites may be a factor that contributes to the maintenance of variation in host defense within and between populations.
Here, we aimed to determine the effects of evolved host defense on host interactions with novel parasites. Evolved host defenses are generally the result of coevolved or one-sided evolutionary interactions, which are predicted to produce different outcomes in terms of host defense range (Antonovics et al., 2013). Given that both one-sided and coevolutionary interactions may determine the nature and specificity of host defense, it is critical to distinguish between the predicted effects of these different evolutionary histories on host defense. Coevolution can drive numerous reciprocal changes in hosts and parasites. The genotypes that evolve to confer host defense under coevolution are likely to be highly specific, diverging substantially between different host populations and providing resistance against the local parasite population (Perlman and Jaenike, 2003; Antonovics et al., 2013). One-sided evolution, which can be accomplished via frequent infection of hosts that are incapable of transmitting the parasite (Holt and Gomulkiewicz, 1997), can favor the evolution of host resistance without permitting parasite reciprocal adaptation. The absence of a coevolutionary arms race can limit the degree of evolutionary change and divergence in the host population because evolved host defenses maintain their effectiveness over time and subsequent change is not favored. Thus, one-sided evolution is predicted to generate less host-parasite specificity than coevolutionary interactions, but still result in the evolution of elevated host defense overall.
Therefore, testing the effects of host population evolutionary history on host defense requires a host-parasite system capable of one-sided and coevolution, a known host evolutionary history, and a diverse set of parasite genotypes to assay host defense range. The free-living nematode Caenorhabditis elegans, and its bacterial parasite Serratia marcescens (Mallo et al., 2002), provide a system suitable for this test. While C. elegans lack an adaptive immune system, their innate immune system exhibits specific responses to different bacterial species (Wong et al., 2007), providing the opportunity to measure host defense range. In a previous study, obligately outcrossing populations of host C. elegans populations were experimentally evolved under conditions that facilitated coevolution or one-sided evolution with Serratia marcescens strain SM2170, or with heat killed SM2170 as a control (Morran et al., 2011). The resulting coevolved and one-sided host populations adapted to their respective parasite populations, and the coevolved parasite populations showed clear signatures of local adaptation (Morran et al., 2014). Control populations, as expected, did not adapt to SM2170. Thus, these experimentally evolved host populations experienced vastly different evolutionary histories with S. marcescens parasites.
In this study, we evaluated the impact of evolutionary history on the range of evolved host defense. We exposed populations of C. elegans, which had been previously evolved against S. marcescens SM2170 in three treatments (coevolved, one-sided & a no parasite control) (Morran et al., 2011), to various genotypes of Serratia which either were, or were not, derived from SM2170. We predicted that coevolved host populations would exhibit greater specificity in their defense when compared to one-sided and control populations, and as a result the coevolved populations would be most susceptible to novel (non-SM2170 derived) parasite strains. Further, we predicted that the effectiveness of evolved host defense would generally decrease against parasite strains that were not derived from SM2170.
Materials and Methods
Host Populations
All C. elegans host populations used in this study were derived from the obligately outcrossing and highly inbred PX386 strain, which is a derivative of the CB4856 strain (Morran et al., 2009). To generate PX386, the fog-2 (q71) mutant allele, which prevents hermaphrodites from self-fertilizing (Schedl and Kimble, 1988), was backcrossed into an inbred CB4856 background for five generations and was subsequently inbred for ten additional generations (Morran et al., 2009). Then five populations of PX386 were independently mutagenized with ethyl-methanesulfonate to generate genetically variable populations prior to selection (Morran et al., 2011). Following backcrossing, populations were kept under standard laboratory conditions for 4 generations in order to purge the most deleterious mutations. These populations were maintained on 10cm Petri dishes filled with NGM Lite (Nematode Growth Medium-Lite, US Biological, Swampscott, MA, USA) seeded with 30μL of OP50 stored at 20°C.
The methods above were used to generate five independent and genetically unique populations. Previous experimental evolution of these C. elegans host populations is fully described in Morran et al. (2011). Briefly, each of the five genetically unique populations of obligately outcrossing C. elegans were divided into 3 treatments (one-sided, coevolved and control) and evolved with S. marcescens SM2170 on Serratia Selection Plates (SSPs) for 30 generations respectively (Figure 1). SSPs consist of a 10 cm Petri dish with a lawn of Serratia opposite a lawn of E. coli. Worms are placed directly on the Serratia lawn which ensures hosts encounter the parasite before reaching their relatively benign lab food source, OP50 E. coli (Morran et al., 2009). Within the context of the experiment, C. elegans individuals must survive and reproduce for their offspring to be passaged to the next round of selection. Coevolved host populations are unique in that they were passaged along with parasite populations. In these treatments, parasites were required to infect and kill a host to be passaged to the next round of selection, thus allowing for reciprocal evolution in host and parasite populations. One- sided populations were passaged using similar methods, except parasites were not passaged and a static ancestral SM2170 plated each passage. Control populations were passaged with heat killed SM2170. Following thirty generations of experimental evolution, multiple samples from each host and parasite population were frozen (Morran et al., 2011). Prior to being used in this experiment, host populations were thawed and maintained under standard laboratory conditions for approximately 4 generations to permit recovery.
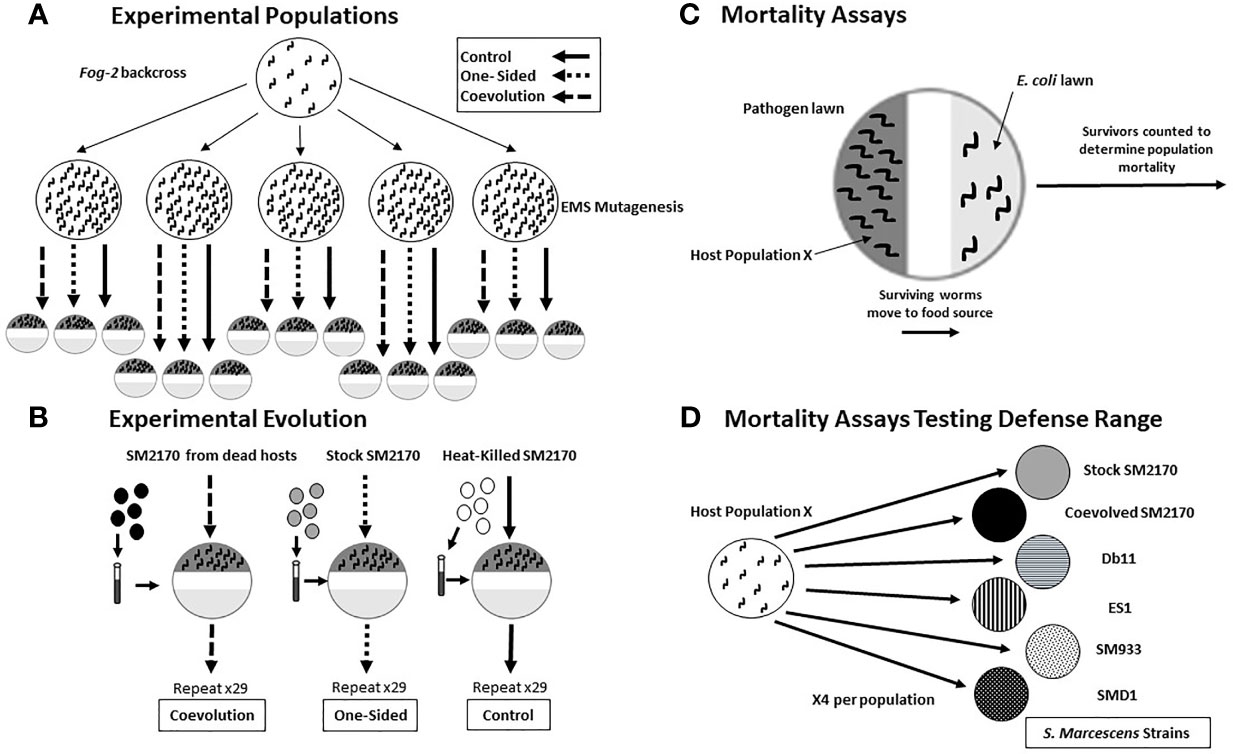
Figure 1 Experimental Overview. (A) The fog-2 allele was backcrossed into inbred CB4856 strain C. elegans, which were subsequently mutagenized to induce variation. After several rounds of reproduction to purge deleterious mutations, worms were separated into 5 groups. Each group was then divided into 3 (control, one- sided, coevolution) and subjected to 30 rounds of exposure to their treatment parasite via Serratia Selection Plates. At the end of each round of selection, the surviving C. elegans are moved to the next plate to begin the process again. (B) In the control group, selection plates were seeded using heat killed Serratia SM2170. One-sided treatment plates received their bacterial lawns from a static stock of Serratia SM2170. Coevolving populations were seeded with SM2170 bacterial colonies removed from the guts of killed worms. (C) To determine population resistance, 200 worms were exposed to the same Serratia Selection Plate protocols as in (A). (D) To assess host range each of our experiential treatments were assayed against 6 Serratia strains. Each of the 5 replicate populations within each treatment were assayed 4 times. However, here worms were not moved to another plate, and instead were counted.
Parasite Populations
S. marcescens is an established bacterial parasite of C. elegans (Mallo et al., 2002), with notable variance in mortality rate depending on strain (Schulenburg and Ewbank, 2004). In this experiment, populations were transferred from frozen stock to Luria Broth (LB) and grown overnight at 28°C. Colonies in LB were then used to seed 10cm Petri dishes filled with NGM-Lite and grown up at 28°C. Parasite mortality assays were completed using S. marcescens strains Db11, ES1, SMD1, SM2170, coevolved SM2170, and SM933.
The SM2170 genotype is highly virulent to C. elegans (Schulenburg and Ewbank, 2004). The ES1 strain was derived from SM2170, via passaging with C. elegans strain CB4856 under selection for increased virulence for 30 generations (Lynch et al., 2018). The coevolved SM2170 assays were conducted using parasite populations that were coevolved with hosts in the previous experiment and isolated after 20 passages (Morran et al., 2011). Each of the five coevolved host populations thus has its own respective sympatric coevolved parasite population. Db11 is a streptomycin resistant derivative of Db10 (Flyg et al., 1980) and has been shown to be moderately virulent in comparison to SM2170. The other Serratia strains used for our treatments, SMD1and SM933, are strains available via Carolina Biological Supply (Burlington, NC). Importantly, Db11, SMD1, SM933 were not directly derived from SM2170 and thus represent novel parasite strains.
Measuring Adaptation and Defense Specificity
Here we use host mortality as a measure of host defense, and a representative measure of host fitness (Penley et al., 2017). Mortality assays were conducted using SSPs identical to those used during experimental evolution (Figure 1C), with the exception of the parasite strain used. A mortality rate was calculated for every treatment against S. marcescens Db11, SMD1, SM933, SM2170, and the coevolved SM2170 populations. Approximately 200 L4 C. elegans were suspended in M9 buffer and transferred to a lawn of SM2170 on a 10 cm Petri dish (NGM-Lite agar). The average number of individuals transferred was calculated by determining densities of C. elegans in the buffer and taking the mean of plated controls. After 48 hours of exposure, we counted the number of surviving worms on the plate. Mortality rates are calculated by dividing the number of dead nematodes by the total number transferred (Morran et al., 2011). It is important to note that while the majority of resistant worms move from the Serratia lawn to the opposite E. coli lawn, some individuals remain in the parasite lawn and those individuals are also counted. Every population (5) in each treatment (3) was replicated 4 times per bacterial strain (technical replicates). Mean mortality rates were analyzed using a generalized linear model (GLM) fitted with a normal distribution and identity link function, testing for effects of Bacteria, Treatment (coevolved, one-sided, control), and the interaction between Bacteria and Treatment. Contrast tests were used to compare mean mortality between treatments post hoc. Additionally, we tested for overdispersion using a Pearson test, and did not detect a significant level of overdispersion. Finally, we performed a binomial GLM after converting our mortality data into a binomial distribution (alive vs dead). The results of our binomial analysis were qualitatively similar to the normal GLM, thus we report the stats of the GLM fitted with the normal distribution. We used JMP Pro (v13) for the GLM analyses.
Results
We first tested for the evolution of elevated host defense (decreased mortality) in our coevolved and one-sided host populations against the coevolved parasite populations derived from SM2170. We found that the coevolved hosts exhibited lower rates of mortality than the control hosts when exposed to the coevolved populations of SM2170 (Figure 2; = 5.174, P =.023), indicating the coevolved hosts adapted to their respective antagonists during experimental coevolution. Interestingly, the one-sided hosts also performed significantly better than the control groups against coevolved parasites (Figure 2). We then found that the one-sided evolution hosts adapted to the SM2170 strain as they exhibited reduced mortality in comparison to both the control and coevolved populations when exposed to SM2170 (Figure 2; = 9.798, P =.002). Thus, the coevolved and one-sided evolution hosts evolved greater levels of host defense and exhibited unique evolutionary trajectories relative to one another and the controls.
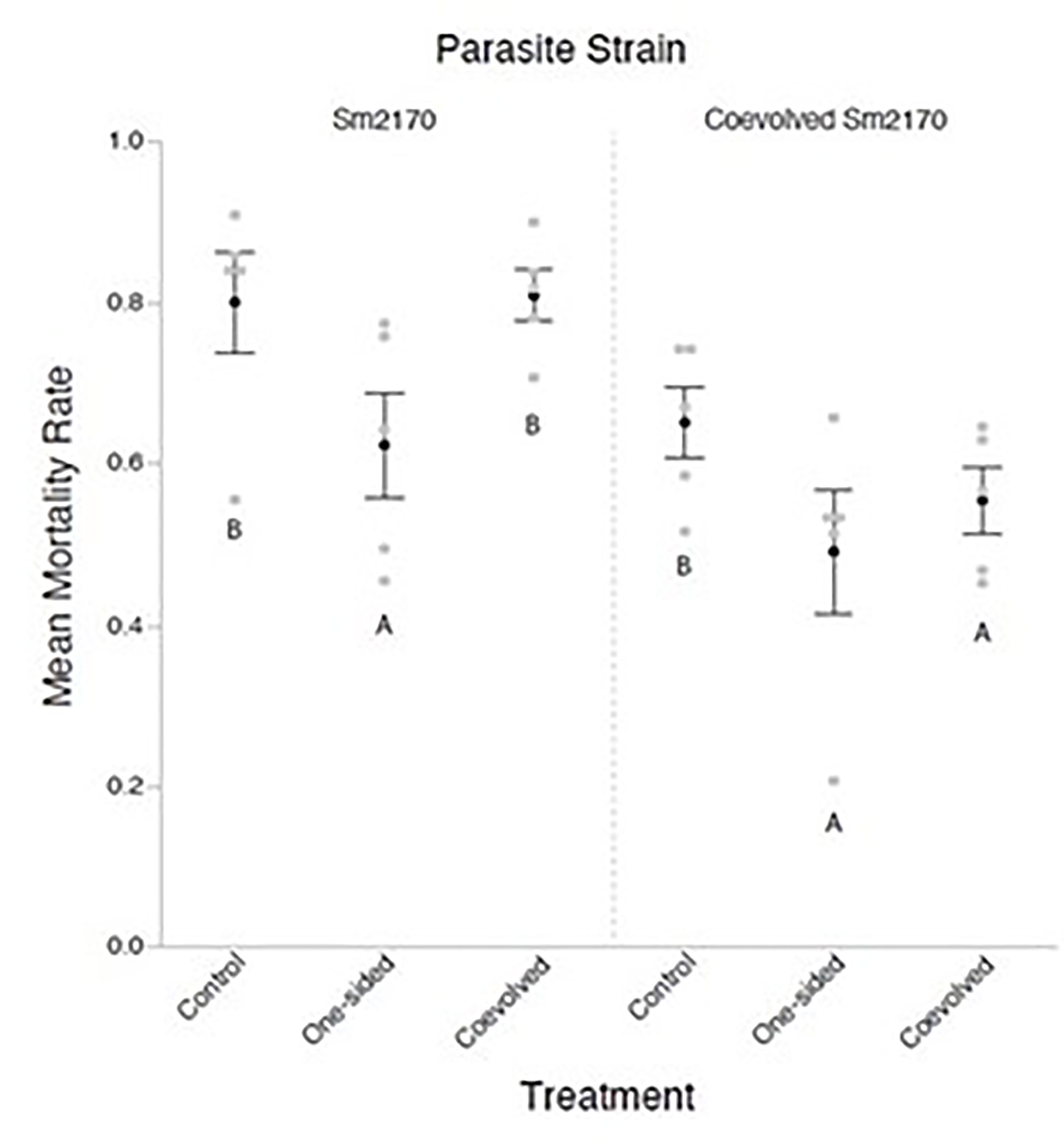
Figure 2 Host Mortality across related parasites. For each mortality assay 200 Worms were exposed to S. marcescens for a period of 48 hours using Serratia Selection Plates. Surviving worms were counted and the mortality is expressed as (worms plated – worms counted)/worms plated). Black circles represent the average mortality rate across all host populations for each bacterial treatment group. White circles represent the average mortality rate across all replicates for one host population. Points which share letters are statistically indistinguishable from each other, and only apply within their respective column. Error bars represent standard error. Letters are differentiated by α= 0.05.
To determine the impact of host evolutionary history on the specificity of evolved defense, we compared mortality rates of three groups of hosts with different evolutionary histories (control, coevolved & one-sided) against four strains of S. marcescens (Db11, ES1, SMD1, and SM933). Overall, we found a significant difference in the mortality exhibited by hosts with different evolutionary histories. Specifically, coevolved hosts exhibited higher overall mortality rates than one-sided ( = 8.44, P =.004) and control ( = 4.85, P =.028) host populations (Supplementary 1, 2). However, the dynamics of host mortality responses varied significantly between parasite strains (Figures 2, 3; = 26.221, P =.004).
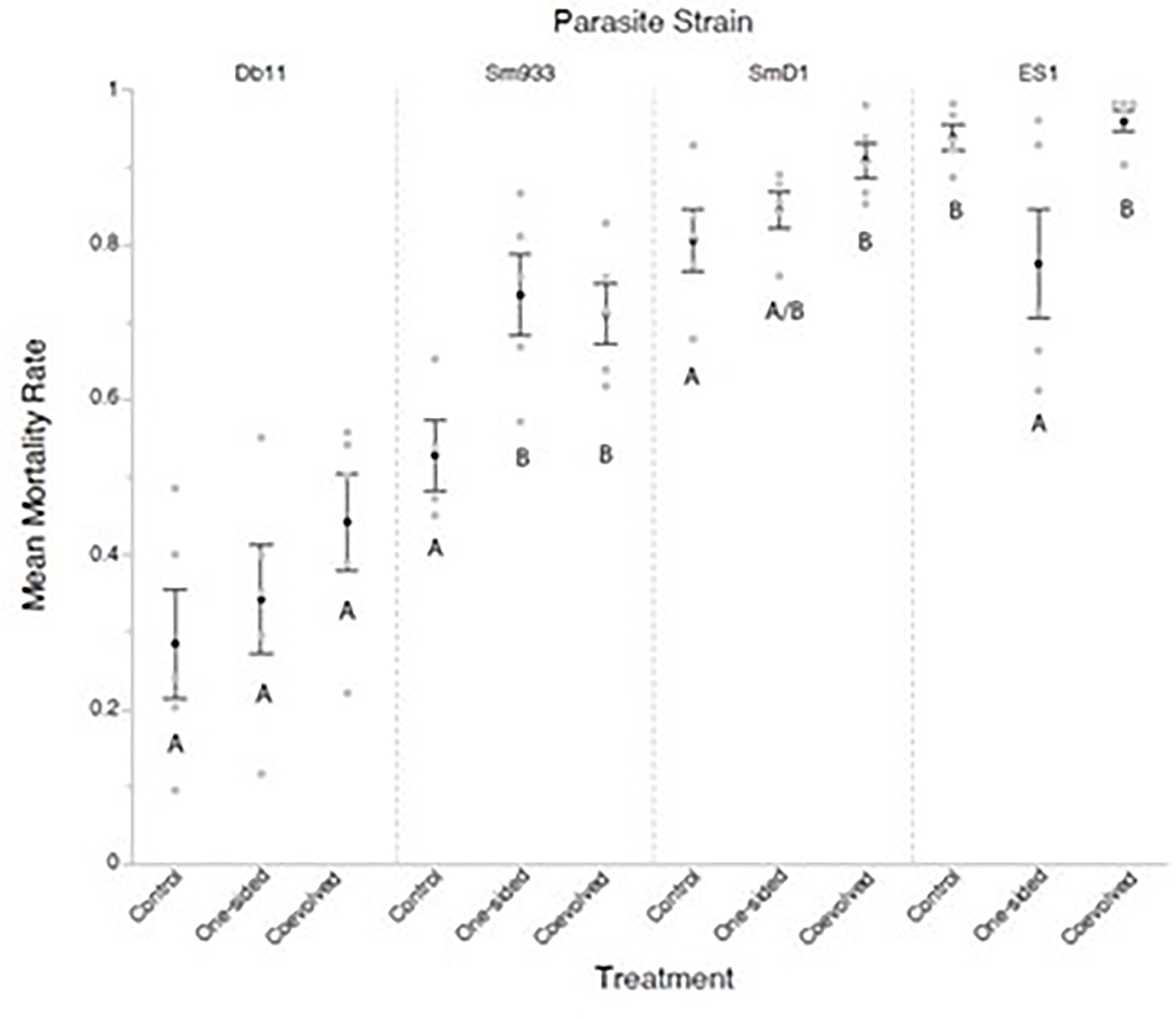
Figure 3 Host Mortality across unrelated parasites. For each mortality assay 200 Worms were exposed to S. marcescens for a period of 48 hours using Serratia Selection Plates. Surviving worms were counted and the mortality is expressed as (worms plated – worms counted)/worms plated). Black circles represent the average mortality rate across all host populations for each bacterial treatment group. White circles represent the average mortality rate across all replicates for one host population. Points which share letters are statistically indistinguishable from each other, and only apply within their respective column. Error bars represent standard error. Letters are differentiated by α= 0.05.
In the presence of ES1, which is derived from SM2170, one-sided host populations experienced lower mortality than control and coevolved populations (Figure 3; = 4.86, P =.028). Conversely, control hosts exhibited reduced mortality against the SM933 relative to the coevolved and one-sided evolution hosts (Figure 3; = 9.68, P =.002). Then, coevolved hosts performed significantly worse on DB11 relative to both control and one-sided evolution hosts (Figure 3; = 5.64, P= .018). Finally, SMD1 did not inflict significantly different mortality rates regardless of host evolutionary history (Figure 3; = 2.16, P =0.142). Therefore, host evolutionary history significantly altered host defense against novel parasite strains.
Discussion
In this study, we investigated the effects of a host population’s evolutionary history with parasites on the subsequent defense range of those hosts. Our data support the hypothesis that a host population’s defense range can be altered by its past evolutionary interactions with parasites. Further, they suggest that coevolution and reciprocal adaptation can have a significant effect on host defense, beyond adaptation, to a coevolving antagonist. Among our host populations, coevolved hosts displayed elevated defense against only their co-evolved parasitic partner relative to the control hosts (Figures 2, 3). Otherwise, the coevolved host populations were overall more susceptible to novel parasites. This aligns with theory suggesting coevolution can lead to highly specific host defenses, due in part to the “arms-race” dynamics surrounding the evolution of those selected traits (Antonovics et al., 2013). This idea is further supported by the coevolved hosts performance against SM2170, where they experienced mortality rates statistically similar to the S. marcescens naive control populations (Figure 2). Importantly, the SM2170 strain was the ancestral strain for each of the coevolved parasite populations, and yet the hosts maintained a very limited ability to defend against the strain. Thus, an evolutionary history of reciprocal adaptation with the parasite resulted in a very high degree of specificity over a relatively short period of time.
As expected, one-sided host populations displayed elevated defense when assayed with SM2170, the same genotype they had been exposed to for 30 generations. However, the one-sided host populations also showed elevated defense against all SM2170 derived parasite genotypes, exhibiting the lowest mortality rates against ES1 and rates similar to coevolved hosts against the coevolved parasites (Figure 2). This provides further evidence that one-sided populations adapted to their parasites, and points towards those defenses as having some general applicability against similar parasite genotypes. This also aligns with theory, as one-sided evolution is predicted to favor any genetic combination in the host which provides adequate defense, rather than a genotype-specific response (Antonovics et al., 2013). Interestingly, the control populations consistently experienced lower mortality rates when assayed against parasites that were not derived from SM2170 (Figure 3). Taken into context with the comparatively high mortality rates control populations experienced when exposed to SM2170 derived genotypes, this validates the control populations by showing a lack of defense evolution during experimental evolution and points toward a certain degree of evolutionary naiveté being beneficial for general defense. In other words, a lack of evolutionary history with parasites seems to confer an overall greater ability to defend against novel parasite strains. Therefore, host evolutionary history with a parasite, or lack thereof, can be an important factor shaping host defense range.
One limitation of this experiment is that coevolution was done in the presence of a single parasite population. This distinction is important, as research suggests infections in the wild commonly consist of multiple strains or species (Petney and Andrews, 1998; Cox, 2001; Telfer et al., 2008; Balmer and Tanner, 2011). Further, multi-genotype infections can alter the fitness of both hosts and parasites, thus having implications on their respective evolutionary trajectories (Alizon et al., 2013; Lange et al., 2014; King et al., 2016). Thus, passaging host populations on single genotype bacterial lawns may have biased evolution in ways which are not applicable to some natural settings. However, while multi-strain infections are commonplace, particular strains may still disproportionately drive the host’s adaptive response, particularly those which invoke the most drastic fitness costs. Indeed, in some natural systems host defense evolution is predominantly driven by interactions with one highly virulent parasite, despite the presence of other parasites (Lively et al., 1990; Paczesniak et al., 2019). An additional limitation of this experiment is that experimental evolution itself may have biased our results by relaxing the strength of non-parasite selective pressures on host populations (Kawecki, 2012). As such, genes conferring defense may have risen in frequency which would not have in nature due to adverse pleiotropic effects. However, as such effects would be constant among all treatment groups, this still allows for the identification of relevant differences between treatments groups. Further, parasites can dictate host evolutionary trajectories through strong selection pressure. This may allow sufficiently beneficial defense alleles to increase in frequency despite other pleiotropic effects (Otto, 2004; Olson-Manning et al., 2012), or closely linked deleterious alleles (Hartfield and Otto, 2011). An additional limitation of this experiment is that all host populations were derived from the same genetic background. As such, while treatments can respond differently to selection pressures during experimental evolution, the responses of the host populations are not fully representative of all possible genotypes. This work demonstrates that the evolutionary history of host populations can shape host defense range. However, such effects of evolutionary history may differ between host genetic backgrounds, which could account for some of the variation in host defense within and between host populations in nature.
In this experiment, we showed that evolution with a parasite can have a profound impact on the characteristics of host defenses. Specifically, the amount of evolutionary reciprocity within the interaction can influence the effective range of host defenses, with increased reciprocity resulting in more narrow ranges (Figures 2, 3). This aligns with research showing that parasites evolved with homogenous host populations exhibited more narrow host ranges (White et al., 2020; Gibson et al., 2020a; Gibson et al., 2020b). Therefore, host and parasite populations with an immediate evolutionary history of coevolution may often be constrained in genotypic space, pigeonholed by combinations of alleles that were previously advantageous but are contemporarily unfavorable. Further, host-parasite interactions may alter the evolutionary trajectories of host populations in many ways, including reducing levels of genetic variation in host populations (White et al., 2021) and favoring the evolution of certain traits beyond host defense (Lively, 1987; Morran et al., 2011). Generally, evolutionary history influences the evolutionary trajectory of a population because the genetic background of the population is determined to some extent by past interactions. This is made more complex due to pleiotropy and epistasis (Tyler et al., 2009; Hansen, 2013). These phenomena can impact how a population adapts to a given environment (Østman et al., 2012; Hansen, 2013) and determine the underlying genetic architecture of host defense (Wilfert and Schmid-Hempel, 2008, Lambrechts, 2010). Thus, host-parasite interactions can have implications that extend far beyond the direct outcome of the interaction itself.
Future adaptation may be limited by past adaptation, perhaps constraining the evolution of novel defense, or increasing rates of extinction in tightly co-evolved hosts that encounter significantly different parasites. Coevolutionary interactions can dominate a population’s evolutionary trajectory as reciprocal adaptation occurs, but a population’s evolutionary path can also be influenced by coevolution after the interaction has ended. Within the context of the wider phenomena of host defense varying within and between populations, and over space and time, this suggests evolutionary history does matter. It is said that host-parasite interactions reflect a “mosaic” of coevolution, with various coevolutionary processes occurring between populations across a landscape (Thompson, 2009). It is clear that coevolution in the past can influence the composition of the present and, perhaps, future coevolutionary mosaic.
Data Availability Statement
The raw data supporting the conclusions of this article will be made available by the authors, without undue reservation.
Author Contributions
JL and LM contributed to the conception of the study, writing of the manuscript and the statistical analysis. JL, LM, and MP assisted in designing the experiment. JL, HS, and SA conducted the experimental protocols. All authors contributed to the article and approved the submitted version.
Funding
This work was funded by the National Science Foundation (Graduate Research Fellowship Program 2017246734 to JL and DEB-1750553 to LM).
Author Disclaimer
Any opinion, findings, and conclusions or recommendations expressed in this material are those of the author(s) and do not necessarily reflect the views of the National Science Foundation.
Conflict of Interest
The authors declare that the research was conducted in the absence of any commercial or financial relationships that could be construed as a potential conflict of interest.
Publisher’s Note
All claims expressed in this article are solely those of the authors and do not necessarily represent those of their affiliated organizations, or those of the publisher, the editors and the reviewers. Any product that may be evaluated in this article, or claim that may be made by its manufacturer, is not guaranteed or endorsed by the publisher.
Acknowledgments
We would like to thank Arthur Menezes and Raythe Owens for logistical assistance.
Supplementary Material
The Supplementary Material for this article can be found online at: https://www.frontiersin.org/articles/10.3389/fcimb.2022.758745/full#supplementary-material
References
Aiba, S., Huet, M., Kaltz, O. (2010). Experimental Evolution of Local Parasite Maladaptation. J. Evol Biol. 23, 1195–1205. doi: 10.1111/j.1420-9101.2010.01985.x
Alizon, S., Roode, J. C. D., Michalakis, Y. (2013). Multiple Infections and the Evolution of Virulence. Ecol. Lett. 16, 556–567. doi: 10.1111/ele.12076
Allen, R. L., Bittner-Eddy, P. D., Grenville-Briggs, L. J., Meitz, J. C., Rehmany, A. P., Rose, L. E., et al. (2004). Host-Parasite Coevolutionary Conflict Between Arabidopsis and Downy Mildew. Science 306, 1957–1960. doi: 10.1126/science.1104022
Anderson, R. M., May, R. M. (1982). Coevolution of Hosts and Parasites. Parasitology 85, 411–426. doi: 10.1017/S0031182000055360
Antonovics, J., Boots, M., Ebert, D., Koskella, B., Poss, M., Sadd, B. M. (2013). The Origin of Specificity by Means of Natural Selection: Evolved and Nonhost Resistance in Host-Pathogen Interactions. Evolution 67, 1–9. doi: 10.1111/j.1558-5646.2012.01793.x
Balmer, O., Tanner, M. (2011). Prevalence and Implications of Multiple-Strain Infections. Lancet Infect. Dis. 11, 868–878. doi: 10.1016/S1473-3099(11)70241-9
Bellis, E. S., Mclaughlin, C. M., Depamphilis, C. W., Lasky, J. R. (2021). The Geography of Parasite Local Adaptation to Host Communities. Ecography 44, 1205–1217. doi: 10.1111/ecog.05730
Bérénos, C., Schmid-Hempel, P., Wegner, K. M. (2009). Evolution of Host Resistance and Trade-Offs Between Virulence and Transmission Potential in an Obligately Killing Parasite. J. Evol Biol. 22, 2049–2056. doi: 10.1111/j.1420-9101.2009.01821.x
Bonneaud, C., Balenger, S. L., Russell, A. F., Zhang, J., Hill, G. E., Edwards, S. V. (2011). Rapid Evolution of Disease Resistance is Accompanied by Functional Changes in Gene Expression in a Wild Bird. Proc. Natl. Acad. Sci. 108, 7866–7871. doi: 10.1073/pnas.1018580108
Boots, M., Begon, M. (1993). Trade-Offs With Resistance to a Granulosis Virus in the Indian Meal Moth, Examined by a Laboratory Evolution Experiment. Funct. Ecol. 7, 528–528. doi: 10.2307/2390128
Carius, H. J., Little, T. J., Ebert, D. (2001). Genetic Variation in a Host-Parasite Association: Potential for Coevolution and Frequency-Dependent Selection. Evolution 55, 1136–1145. doi: 10.1111/j.0014-3820.2001.tb00633.x
Cipollini, D., Walters, D., Voelckel, C. (2017). Costs of Resistance in Plants: From Theory to Evidence. Annu. Plant Rev. Online 47, 263–307. doi: 10.1002/9781119312994.apr0512
Cox, F. E. G. (2001). Concomitant Infections, Parasites and Immune Responses. Parasitology 122, S23–S38. doi: 10.1017/S003118200001698X
De Roode, J. C., Lefèvre, T., Hunter, M. D. (2013). Self-Medication in Animals. Science 340, 150–150. doi: 10.1126/science.1235824
Diamond, G., Beckloff, N., Weinberg, A., Kisich, K. (2009). The Roles of Antimicrobial Peptides in Innate Host Defense. Curr. Pharm. Design 15, 2377–2392. doi: 10.2174/138161209788682325
Duncan, A. B., Little, T. J. (2007). Parasite-Driven Genetic Change in a Natural Population of Daphnia. Evolution 61, 796–803. doi: 10.1111/j.1558-5646.2007.00072.x
Ebert, D. (1994). Virulence and Local Adaptation of a Horizontally Transmitted Parasite. Science 265, 1084–1086. doi: 10.1126/science.265.5175.1084
Ebert, D., Hamilton, W. D. (1996). Sex Against Virulence: The Coevolution of Parasitic Diseases. Trends Ecol. Evol. 11, 79–82. doi: 10.1016/0169-5347(96)81047-0.PMID: 21237766.
Edmunds, G. F., Alstad, D. N. (1978). Coevolution in Insect Herbivores and Conifers. Science 199, 941–945. doi: 10.1126/science.199.4332.941
Ellis, A. E. (2001). Innate Host Defense Mechanisms of Fish Against Viruses and Bacteria. Dev. Comp. Immunol. 25, 827–839. doi: 10.1016/S0145-305X(01)00038-6
Flyg, C., Kenne, K., Boman, H. G. (1980). Insect Pathogenic Properties of Serratia Marcescens: Phage-Resistant Mutants With a Decreased Resistance to Cecropia Immunity and a Decreased Virulence to Drosophila. Microbiology 120, 173–181. doi: 10.1099/00221287-120-1-173
Fuxa, J. R., Richter, A. R. (1989). Reversion of Resistance by Spodoptera Frugiperda to Nuclear Polyhedrosis Virus. J. Invertebr Pathol. 53, 52–56. doi: 10.1016/0022-2011(89)90073-6
Gandon, S., Capowiez, Y., Dubois, Y., Michalakis, Y., Olivieri, I. (1996). Local Adaptation and Gene-for-Gene Coevolution in a Metapopulation Model. Proceedings of the Royal Society of London. Ser. B: Biol. Sci. 263, 1003–1009. doi: 10.1098/rspb.1996.0148
Gandon, S., Zandt, P. A. V. (1998). Local Adaptation and Host–Parasite Interactions. Trends Ecol. Evol. 13, 214–216. doi: 10.1016/S0169-5347(98)01358-5
Gibson, A. K., Baffoe-Bonnie, H., Penley, M. J., Lin, J., Owens, R., Khalid, A., et al. (2020a). The Evolution of Parasite Host Range in Heterogeneous Host Populations. J. Evol Biol. 33, 773–782. doi: 10.1111/jeb.13608
Gibson, A. K., White, P. S., Penley, M. J., Roode, J. C. D., Morran, L. T. (2020b). An Experimental Test of Parasite Adaptation to Common Versus Rare Host Genotypes. Biol. Lett. 16, 20200210–20200210. doi: 10.1098/rsbl.2020.0210
Graham, A. L., Hayward, A. D., Watt, K. A., Pilkington, J. G., Pemberton, J. M., Nussey, D. H. (2010). Fitness Correlates of Heritable Variation in Antibody Responsiveness in a Wild Mammal. Science 330, 662–665. doi: 10.1126/science.1194878
Greischar, M. A., Koskella, B. (2007). A Synthesis of Experimental Work on Parasite Local Adaptation. Ecol. Lett. 10, 418–434. doi: 10.1111/j.1461-0248.2007.01028.x
Hansen, T. F. (2013). Why Epistasis is Important for Selection and Adaptation. Evolution 67, 3501–3511. doi: 10.1111/evo.12214
Hartfield, M., Otto, S. P. (2011). Recombination and Hitchhiking of Deleterious Alleles. Evolution 65, 2421–2434. doi: 10.1111/j.1558-5646.2011.01311.x
Hasu, T., Benesh, D. P., Valtonen, E. T. (2009). Differences in Parasite Susceptibility and Costs of Resistance Between Nexposed and Unexposed Host Populations. J. Evol. Biol. 22, 699–707. doi: 10.1111/j.1420-9101.2009.01704.x
Hoeksema, J. D., Forde, S. E. (2008). A Meta-Analysis of Factors Affecting Local Adaptation between Interacting Species. Am. Nat. 171, 3, 275–3, 290.
Holt, R. D., Gomulkiewicz, R. (1997). How Does Immigration Influence Local Adaptation? A Reexamination of a Familiar Paradigm. Am. Nat. 149, 3, 563–3, 572.
Kawecki, T. J., Ebert, D. (2004). Conceptual Issues in Local Adaptation. Ecol. Lett. 7 (12), 1225–1241. doi: 10.1111/j.1461-0248.2004.00684.x
Kawecki, T. J., Lenski, R. E., Ebert, D., Hollis, B., Olivieri, O., Whitlock, M. C. (2012). Experimental Evolution. Trends. Ecol. Evol. 27 (10), 547–560. doi: 10.1016/j.tree.2012.06.001
King, K. C., Brockhurst, M. A., Vasieva, O., Paterson, S., Betts, A., Ford, S. A., et al. (2016). Rapid Evolution of Microbe-Mediated Protection Against Pathogens in a Worm Host. ISME J. 10, 8 10, 1915–1924. doi: 10.1038/ismej.2015.259
Kniskern, J. M., Barrett, L. G., Bergelson, J. (2011). Maladaptation in Wild Populations of the Generalist Plant Pathogen Pseudomonas Syringae. Evolution 65, 818–830. doi: 10.1111/j.1558-5646.2010.01157.x
Koskella, B. (2018). Resistance Gained, Resistance Lost: An Explanation for Host-Parasite Coexistence. PloS Biol. 16, e3000013–e3000013. doi: 10.1371/journal.pbio.3000013
Koskella, B., Lively, C. M. (2007). Advice of the Rose: Experimental Coevolution of a Trematode Parasite and its Snail Host. Evolution 61, 152–159. doi: 10.1111/j.1558-5646.2007.00012.x
Kraaijeveld, A. R., Godfray, H. C. J. (1997). Trade-Off Between Parasitoid Resistance and Larval Competitive Ability in Drosophila Melanogaster. Nature 389, 6648 389, 278–280. doi: 10.1038/38483
Laine, A.-L. (2004). Resistance Variation Within and Among Host Populations in a Plant–Pathogen Metapopulation: Implications for Regional Pathogen Dynamics. J. Ecol. 92, 990–1000. doi: 10.1111/j.0022-0477.2004.00925.x
Lambrechts, L. (2010). Dissecting the Genetic Architecture of Host–Pathogen Specificity. PloS Pathog. 6, e1001019–e1001019. doi: 10.1371/journal.ppat.1001019
Lange, B., Reuter, M., Ebert, D., Muylaert, K., Decaestecker, E. (2014). Diet Quality Determines Interspecific Parasite Interactions in Host Populations. Ecol. Evol. 4, 3093–3102. doi: 10.1002/ece3.1167
Leimu, R., Fischer, M. (2008). A Meta-Analysis of Local Adaptation in Plants. PloS One 3, e4010–e4010. doi: 10.1371/journal.pone.0004010
Lemaitre, B., Hoffmann, J. (2007). The Host Defense of Drosophila Melanogaster. Annu. Rev. Immunol. 25, 697–743. doi: 10.1146/annurev.immunol.25.022106.141615
Lemoine, M., Doligez, B., Richner, H. (2012). On the Equivalence of Host Local Adaptation and Parasite Maladaptation: An Experimental Test. Am. Nat. 179, 270–281. doi: 10.1086/663699
Lenski, R. E. (1997). The Cost of Antibiotic Resistance—from the Perspective of a Bacterium. In Ciba Foundation Symposium 207 - Antibiotic Resistance: Origins, Evolution, Selection and Spread. (eds Chadwick, D. J., Goode, J.). doi: 10.1002/9780470515358.ch9
Lively, C. M. (1987). Evidence From a New Zealand Snail for the Maintenance of Sex by Parasitism. Nature 328, 6130 328, 519–521. doi: 10.1038/328519a0
Lively, C. M., Craddock, C., Vrijenhoek, R. C. (1990). Red Queen Hypothesis Supported by Parasitism in Sexual and Clonal Fish. Nature 344, 6269 344, 864–866. doi: 10.1038/344864a0
Lively, C. M., Dybdahl, M. F. (2000). Parasite Adaptation to Locally Common Host Genotypes. Nature 405, 679–681. doi: 10.1038/35015069
Lohse, K., Gutierrez, A., Kaltz, O. (2006). Experimental Evolution of Resistance in Paramecium Caudatum Against the Bacterial Parasite Holospora Undulata. Evolution 60, 1177–1186. doi: 10.1111/j.0014-3820.2006.tb01196.x
Lynch, Z. R., Penley, M. J., Morran, L. T. (2018). Turnover in Local Parasite Populations Temporarily Favors Host Outcrossing Over Self-Fertilization During Experimental Evolution. Ecol. Evol. 8, 6652–6662. doi: 10.1002/ece3.4150
Mallo, G. V., Kurz, C. L., Couillault, C., Pujol, N., Granjeaud, S., Kohara, Y., et al. (2002). Inducible Antibacterial Defense System in C. Elegans. Curr. Biol. 12, 1209–1214. doi: 10.1016/S0960-9822(02)00928-4
Melnyk, A. H., Wong, A., Kassen, R. (2015). The Fitness Costs of Antibiotic Resistance Mutations. Evol Appl. 8, 273–283. doi: 10.1111/eva.12196
Morran, L. T., Parmenter, M. D., Phillips, P. C. (2009). Mutation Load and Rapid Adaptation Favour Outcrossing Over Self-Fertilization. Nature 462, 7271 462, 350–352. doi: 10.1038/nature08496
Morran, L. T., Parrish, R. C., Gelarden, I. A., Allen, M. B., Lively, C. M. (2014). Experimental Coevolution: Rapid Local Adaptation by Parasites Depends on Host Mating System. Am. Nat. 184, S91–S100. doi: 10.1086/676930
Morran, L. T., Schmidt, O. G., Gelarden, I. A., Parrish, R. C., Lively, C. M. (2011). Running With the Red Queen: Host-Parasite Coevolution Selects for Biparental Sex. Science 333, 216–218. doi: 10.1126/science.1206360
Olson-Manning, C. F., Wagner, M. R., Mitchell-Olds, T. (2012). Adaptive Evolution: Evaluating Empirical Support for Theoretical Predictions. Nat. Rev. Genet. 13, 12 13, 867–877. doi: 10.1038/nrg3322
Østman, B., Hintze, A., Adami, C. (2012). Impact of Epistasis and Pleiotropy on Evolutionary Adaptation. Proc. R. Soc. B: Biol. Sci. 279, 247–256. doi: 10.1098/rspb.2011.0870
Otto, S. (2004). Two Steps Forward, One Step Back: The Pleiotropic Effects of Favoured Alleles. Proc. R. Soc. B: Biol. Sci. 271, 705–714.
Paczesniak, D., Klappert, K., Kopp, K., Neiman, M., Seppälä, K., Lively, C. M., et al. (2019). Parasite Resistance Predicts Fitness Better Than Fecundity in a Natural Population of the Freshwater Snail Potamopyrgus Antipodarum. Evolution 73, 1634–1646. doi: 10.1111/evo.13768
Parker, B. J., Barribeau, S. M., Laughton, A. M., De Roode, J. C., Gerardo, N. M. (2011). Non-Immunological Defense in an Evolutionary Framework. Trends Ecol. Evol. 26, 242–248. doi: 10.1016/j.tree.2011.02.005
Penley, M. J., Ha, G. T., Morran, L. T. (2017). Evolution of Caenorhabditis Elegans Host Defense Under Selection by the Bacterial Parasite Serratia Marcescens. PloS One 12, e0181913–e0181913. doi: 10.1371/journal.pone.0181913
Perlman, S. J., Jaenike, J. (2003). Infection Success in Novel Hosts: An Experimental and Phylogenetic Study of Drosophila- Parasitic Nematodes. Evolution 57, 544–557. doi: 10.1111/j.0014-3820.2003.tb01546.x
Petney, T. N., Andrews, R. H. (1998). Multiparasite Communities in Animals and Humans: Frequency, Structure and Pathogenic Significance. Int. J. Parasitol 28, 377–393. doi: 10.1016/S0020-7519(97)00189-6
Rainey, P. B., Buckling, A., Kassen, R., Travisano, M. (2000). The Emergence and Maintenance of Diversity: Insights From Experimental Bacterial Populations. Trends Ecol. Evol. 15, 243–247. doi: 10.1016/S0169-5347(00)01871-1
Roy, B. A., Kirchner, J. W. (2000). Evolutionary Dynamics of Pathogen Resistance and Tolerance. Evolution 54, 51–63. doi: 10.1111/j.0014-3820.2000.tb00007.x
Schedl, T., Kimble, J. (1988). Fog-2, a Germ-Line-Specific Sex Determination Gene Required for Hermaphrodite Spermatogenesis in Caenorhabditis Elegans. Genetics 119, 43–61. doi: 10.1093/genetics/119.1.43
Schulenburg, H., Ewbank, J. J. (2004). Diversity and Specificity in the Interaction Between Caenorhabditis Elegans and the Pathogen Serratia Marcescens. BMC Evol Biol. 4, 1 4, 1–1 4, 8. doi: 10.1186/1471-2148-4-49
Schulte, R. D., Makus, C., Hasert, B., Michiels, N. K., Schulenburg, H. (2010). Multiple Reciprocal Adaptations and Rapid Genetic Change Upon Experimental Coevolution of an Animal Host and its Microbial Parasite. Proc. Natl. Acad. Sci. 107, 7359–7364. doi: 10.1073/pnas.1003113107
Sheldon, B. C., Verhulst, S. (1996). Ecological Immunology: Costly Parasite Defences and Trade-Offs in Evolutionary Ecology. Trends Ecol. Evol. 11, 317–321. doi: 10.1016/0169-5347(96)10039-2
Strauss, S. Y., Rudgers, J. A., Lau, J. A., Irwin, R. E. (2002). Direct and Ecological Costs of Resistance to Herbivory. Trends Ecol. Evol. 17, 278–285. doi: 10.1016/S0169-5347(02)02483-7
Telfer, S., Birtles, R., Bennett, M., Lambin, X., Paterson, S., Begon, M. (2008). Parasite Interactions in Natural Populations: Insights From Longitudinal Data. Parasitology 135, 767–781. doi: 10.1017/S0031182008000395
Tyler, A. L., Asselbergs, F. W., Williams, S. M., Moore, J. H. (2009). Shadows of Complexity: What Biological Networks Reveal About Epistasis and Pleiotropy. BioEssays 31, 220–227. doi: 10.1002/bies.200800022
Vos, M., Birkett, P. J., Birch, E., Griffiths, R. I., Buckling, A. (2009). Local Adaptation of Bacteriophages to Their Bacterial Hosts in Soil. Science 325, 833–833. doi: 10.1126/science.1174173
War, A. R., Paulraj, M. G., Ahmad, T., Buhroo, A. A., Hussain, B., Ignacimuthu, S., et al. (2012). Mechanisms of Plant Defense Against Insect Herbivores. Plant Signaling Behav. 7, 1306–1320. doi: 10.4161/psb.21663
Webster, J. P., Woolhouse, M. E. J. (1999). Cost of Resistance: Relationship Between Reduced Fertility and Increased Resistance in a Snail Schistosome Host Parasite System. Proceedings of the Royal Society of London. Ser. B: Biol. Sci. 266, 391–396. doi: 10.1098/rspb.1999.0650
Weiss, J., Bayer, A. S., Yeaman, M. (2014). Cellular and Extracellular Defenses Against Staphylococcal Infections. In Gram-Positive Pathogens (eds Fischetti, V. A., Novick, R. P., Ferretti, J. J., Ferretti, D. A., Portnoy, D. A., Rood, J. I.). doi: 10.1128/9781555816513.ch45
White, P. S., Arslan, D., Kim, D., Penley, M., Morran, L. (2021). Host Genetic Drift and Adaptation in the Evolution and Maintenance of Parasite Resistance. J. Evol Biol. 34, 845–851. doi: 10.1111/jeb.13785
White, P. S., Choi, A., Pandey, R., Menezes, A., Penley, M., Gibson, A. K., et al. (2020). Host Heterogeneity Mitigates Virulence Evolution. Biol. Lett. 16. 20190744. doi: 10.1098/rsbl.2019.0744
Wilfert, L., Schmid-Hempel, P. (2008). The Genetic Architecture of Susceptibility to Parasites. BMC Evol Biol. 8, 187–187. doi: 10.1186/1471-2148-8-187
Wong, D., Bazopoulou, D., Pujol, N., Tavernarakis, N., Ewbank, J. J. (2007). Genome-Wide Investigation Reveals Pathogen-Specific and Shared Signatures in the Response of Caenorhabditis Elegans to Infection. Genome Biol. 8, 9 8, 1–9 8,18. doi: 10.1186/gb-2007-8-9-r194
Keywords: coevolution, experimental evolution, host defense, Ceanorhabditis elegans, Serratia macrescens, antagonistic coevolution
Citation: Lewis JA, Penley MJ, Sylla H, Ahumada SD and Morran LT (2022) Antagonistic Coevolution Limits the Range of Host Defense in C. elegans Populations. Front. Cell. Infect. Microbiol. 12:758745. doi: 10.3389/fcimb.2022.758745
Received: 14 August 2021; Accepted: 19 January 2022;
Published: 17 February 2022.
Edited by:
Javier E. Irazoqui, University of Massachusetts Medical School, United StatesReviewed by:
Christoph Vorburger, Swiss Federal Institute of Aquatic Science and Technology, SwitzerlandMaurine Neiman, The University of Iowa, United States
Copyright © 2022 Lewis, Penley, Sylla, Ahumada and Morran. This is an open-access article distributed under the terms of the Creative Commons Attribution License (CC BY). The use, distribution or reproduction in other forums is permitted, provided the original author(s) and the copyright owner(s) are credited and that the original publication in this journal is cited, in accordance with accepted academic practice. No use, distribution or reproduction is permitted which does not comply with these terms.
*Correspondence: Jordan A. Lewis, Sm9yZGFuLmFsZXhhbmRlci5sZXdpc0BlbW9yeS5lZHU=