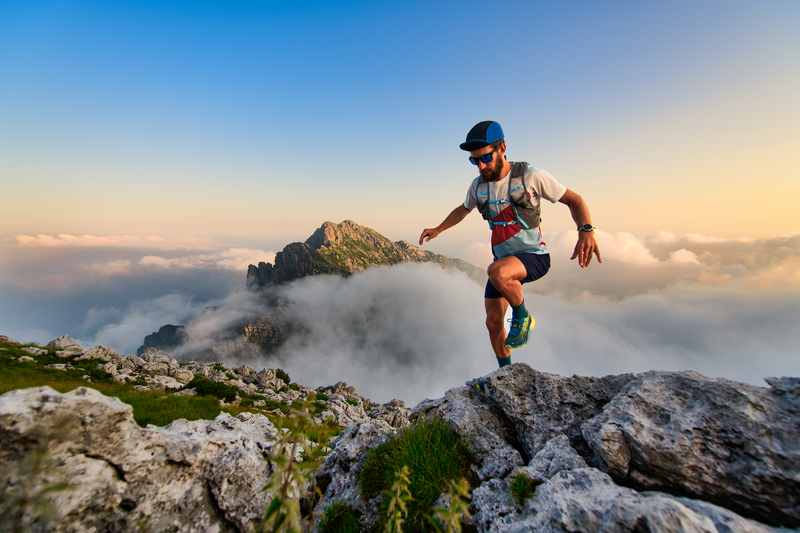
95% of researchers rate our articles as excellent or good
Learn more about the work of our research integrity team to safeguard the quality of each article we publish.
Find out more
MINI REVIEW article
Front. Cell. Infect. Microbiol. , 02 November 2022
Sec. Bacteria and Host
Volume 12 - 2022 | https://doi.org/10.3389/fcimb.2022.1049646
This article is part of the Research Topic Biological Drivers Of Vector-Pathogen Interactions - Vol II View all 7 articles
Ixodes scapularis is one of the predominant vectors of Borrelia burgdorferi, the agent of Lyme disease in the USA. The geographic distribution of I. scapularis, endemic to the northeastern and northcentral USA, is expanding as far south as Georgia and Texas, and northwards into Canada and poses an impending public health problem. The prevalence and spread of tick-borne diseases are influenced by the interplay of multiple factors including microbiological, ecological, and environmental. Molecular studies have focused on interactions between the tick-host and pathogen/s that determine the success of pathogen acquisition by the tick and transmission to the mammalian host. In this review we draw attention to additional critical environmental factors that impact tick biology and tick-pathogen interactions. With a focus on B. burgdorferi we highlight the interplay of abiotic factors such as temperature and humidity as well as biotic factors such as environmental microbiota that ticks are exposed to during their on- and off-host phases on tick, and infection prevalence. A molecular understanding of this ensemble of interactions will be essential to gain new insights into the biology of tick-pathogen interactions and to develop new approaches to control ticks and tick transmission of B. burgdorferi, the agent of Lyme disease.
Ixodes scapularis is one of the predominant tick vectors of B. burgdorferi, the agent of Lyme disease, and the most common tick-borne disease in North America emerging from the northeastern and north central United States foci, where 95% of Lyme Disease (LD) cases occur (Fleshman et al., 2021). Movement of B. burgdorferi within tick tissues, its maintenance across life stages as ticks molt, as well as its proliferation and transmission to vertebrate hosts during tick bloodmeal engorgement may all be subject to environmental influence, be it biotic factors such as the composition of the microbiome, abiotic factors such as temperature and humidity, and the responses of the tick to the spirochete, the vertebrate host and to the environmental components. There is an increasing understanding that environmental conditions affect tick physiology, population dynamics, and B. burgdorferi acquisition and transmission (Dumic and Severnini, 2018). This may be mediated through different components of tick physiology, immune processes, or host activity. Modeling studies have shown that environmental effects on I. scapularis can influence phenology and B. burgdorferi dynamics across geographic regions (Perret et al., 2000; Ogden et al., 2005; Ogden et al., 2008; Gaff et al., 2020). Further, the population dynamics of ticks is shaped by host species availability and behavior (Lord, 1992; Tosato et al., 2021). To understand the collective impacts of biotic and abiotic factors on tick biology and on its vectorial capacity is the holy grail of tick research.
Studies on the direct impacts of abiotic climatic factors on I. scapularis under natural conditions have demonstrated that both temperature and humidity impact tick survival (Lindsay et al., 1995; Bertrand and Wilson, 1996) and host-seeking success (Randolph and Storey, 1999; Randolph, 2004; Ginsberg et al., 2017) and, thus have a direct impact on spirochete infection prevalence in endemic areas. Understanding the direct impacts of these factors on ticks has been foundational to hypothesis about the geographic range, seasonal phenology, and survival of I. scapularis (Ogden et al., 2005) as well as the fitness of the human pathogens they transmit (Ogden et al., 2008; Ogden et al., 2014). Much of our current understanding of the role of the environment on I. scapularis and B. burgdorferi transmission dynamics comes from evaluation of effects on tick populations by estimating population measures such as the timing of peak abundance of each life stage (Ogden et al., 2018) or peak host seeking (Ginsberg et al., 2017). Such measures, while informative, are phenomenological such that the geographic variation observed in I. scapularis phenology has been associated with abiotic (Ogden et al., 2018) and biotic factors (Tsao et al., 2021). There is a burgeoning interest in expanding our mechanistic understanding of the drivers of I. scapularis phenotypic expression using experimental methods to estimate effect measures of light level (Perret et al., 2003), humidity, temperature, and population differences in ticks (Arsnoe et al., 2015) and how these influence the transmission dynamics of B. burgdorferi.
Temperature is a critical environmental factor, with evidence across diverse insect taxa establishing its importance in driving the development (Rebaudo and Rabhi, 2018), biophysical processes (Gillooly et al., 2001), and population growth (Savage et al., 2004). Fewer works have focused on the effect of temperature in longer lived arthropods, whose life stages span seasons, and years with notable exceptions (Sato and Sato, 2015). The phenological timing of peak abundance in the post-egg life stages of I. scapularis vary by region (Ogden et al., 2018) and are at least, in part, likely driven by climatic factors including temperature, relative humidity, and daylight. I. scapularis ticks spend most of their life cycle in the environment rather than on vertebrate hosts, and must contend with temperature fluctuations that occur daily, the changes in temperature over months, seasons, and years, and the cumulative effects of temperature over their lifetime. Moreover, their behavioral repertoire includes walking within and questing above the vegetative layer, and little is known regarding the impact of the type of vegetation on the fluctuations in temperature ticks experience as they move within and without of leaf litter. Importantly, arthropod-microbe symbioses, whether mutualistic or pathogenic, can alter the effects of temperature on arthropod hosts and may even mitigate the stress of heat shock experienced by arthropod hosts during a vertebrate blood meal (Neelakanta et al., 2010; Roma et al., 2019; Lemoine et al., 2020).
Temperature has a significant impact on B. burgdorferi (Tilly et al., 2008) serving as a critical cue to turn on or off B. burgdorferi genes important for colonization of the tick, transmission from the ticks to the vertebrate host and for survival in the host (Carroll et al., 2001; Revel et al., 2002; Ojaimi et al., 2002; Ojaimi et al., 2003). Shifting B. burgdorferi from 23 to 37°C, representing ambient temperatures of the tick or the mammalian host, was shown to alter B. burgdorferi transcriptome (Ojaimi et al., 2002; Tokarz et al., 2004) and these alterations are critical for B. burgdorferi survival in these vastly different milieus (Radolf et al., 2012). Clearly, temperature alone does not fully recapitulate the changes that occur in the vertebrate or invertebrate host (Mulay et al., 2009; Iyer and Schwartz, 2016) but it does highlight the vulnerability of B. burgdorferi transcriptome to alterations in temperature. Whether changes in ambient temperature that ticks must face in different regions of the USA would similarly impact B. burgdorferi gene expression is not known. For example, would increasing ambient temperatures increase expression of OspC, a critical outer surface protein of B. burgdorferi (Pal et al., 2004; Tilly et al., 2006) whose expression is regulated by changes in temperature (Schwan et al., 1995), and enhance B. burgdorferi transmission to the murine host? A simple binary interaction could theoretically produce such an outcome. Ginsberg et al. (2021) suggested that higher temperatures in the southern USA may cause desiccation stress, possibly resulting in ticks remaining under the leaf litter and thus impacting host seeking behavior of I. scapularis. In addition, the availability of hosts such as lizards that are easily accessible under the leaf litter and that readily clear B. burgdorferi infections further likely contributes to the absence of a correlation between tick abundance, infection prevalence, and Lyme disease incidences in the southern USA. These observations underscore the need to bear in mind that changes in ambient temperatures would simultaneously impact tick biology in complex ways and in-turn have a functional consequence on vectorial capacity (Schematically summarized in Figure 1).
Figure 1 Partitioning the microbiome. Panel (A) shows the components of the microbiome in Ixodes scapularis as partitioned by route of transmission including environmental acquisition (green), transovarial transmission (blue), and via a vertebrate blood-meal (pink) (B. burgdorferi s.l, Borrelia burgdorferi, sensu lato; A. phagocytophilum, Anaplasma phagocytophilum; Ba. microti, Babesia microti; B. miyamotoi, Borrelia miyamotoi; POWV, Powassan virus). Panel (B) illustrates the hypothetical (dashed arrows) and demonstrated (solid arrows) effects on gene expression, phenotypic expression, or fitness of the tick (green), B. burgdorferi (red), and their symbiotic interactions (blue) for each abiotic factor (temperature and relative humidity), and for vertebrate host factors such as immune response to parasitism.
The impact of humidity on tick survival and host-seeking behavior is profound (Ginsberg et al., 2017; Fleshman et al., 2021), and I. scapularis may be more sensitive to perturbations in humidity than temperature. Blacklegged ticks spend >95% of their lives off hosts, where larvae and nymphs are particularly subject to intense selection due to sensitivity to desiccation, and therefore are restricted to areas where the relative humidity approaches 100%, i.e., in the leaf litter and low-lying vegetation of the forests they inhabit (Perret et al., 2003; Berger et al., 2014). As nymphal I. scapularis frequently climb above the leaf litter to host-seek (Arsnoe et al., 2019), the ticks lose water through their integumentary space as they respire and return to below the leaf litter to rehydrate as needed. In I. scapularis, the consequences for the daily drops in humidity (below 85% RH) have cumulative survival consequences (Ginsberg et al., 2017). Importantly, tick nutritional reserve levels have been shown to relate negatively to tick movement and the drive to maintain adequate hydration (Crooks and Randolph, 2006; Ginsberg et al., 2017). This suggests that off-host survival is linked to the quality and quantity of the bloodmeals which, after hatching, are required to progress through the three motile life stages, larva, nymph, and adult.
Water absorption in ticks is dependent on their environment. In humid environments, passive absorption is the primary mechanism (Fielden and Lighton, 1996). Active absorption of water vapor can also occur once a certain threshold is reached (the “critical equilibrium humidity”) leading to additional water uptake. The critical equilibrium humidity for ticks generally lies between 75% to 96% relative humidity (Rodgers et al., 2007). Questing as a host-seeking behavior makes Ixodes scapularis “sit and wait” predators. This type of arthropod predators have comparatively low Standard Metabolic Rates (SMR), as demonstrated in spiders (Greenstone and Bennett, 1980). Though spiders are considered to have low SMRs, ticks have an SMR that is 12% of that (Lighton and Fielden, 1995). A low SMR rate allows ticks to maintain respiratory substrate (energy stores) for longer periods of time, and results in less frequent occurrences of the burst phase of their respiratory cycle. Active water absorption is energetically costly, as shown by increased metabolic rates in dehydrated Dermacentor andersoni when placed in highly humid environments (Fielden and Lighton, 1996), that was accompanied by net water gain.
It was shown in Culex pipiens mosquitos that exposure to dehydration resulted in depletion of fat reserves and this had a significant impact on egg production (Benoit et al., 2010). Little is known regarding the patterns of respiration and hydration of I. scapularis, or the impact of the percent relative humidity in the air on tick survival and fecundity in association with B. burgdorferi. Multiple studies have confirmed that I. ricinus has higher rates of survival in unfavorable thermo-hygrometric conditions when infected with B. burgdorferi (Benelli, 2020). Body composition analysis of Ixodes ricinus, the European Lyme disease vector, showed that B burgdorferi-infected I. ricinus have a higher fat content than uninfected (Herrmann et al., 2013). In theory, this allows for greater energy reserves, i.e., longer periods that ticks can survive in the environment before finding the next bloodmeal, and reserves for the caloric expenditures of questing above the leaf litter providing a potential mechanistic basis for tick-microbe-environment interactions (Figure 1).
Microbes can mediate arthropod host behavior to increase the probability of transmission, a phenomenon that has been demonstrated in insect-borne vertebrate pathogens such as Trypanosoma spp. and Dengue (Hurd, 2003; Lefevre and Thomas, 2008). Behaviors affected by parasites and viruses range from feeding behaviors, to reproduction and locomotion (Ribeiro, 2000; Rogers et al., 2002; Lacroix et al., 2005). Within the cycle of transmission by which it is maintained, B. burgdorferi is not pathogenic to ticks. Small vertebrate hosts involved in B. burgdorferi enzootic transmission cycle, from which the immature stages of I. scapularis (the blacklegged tick) take obligatory blood meals, tolerate B. burgdorferi infection. Evidence suggests that the ecological association between I. scapularis and B. burgdorferi may be characterized as a facultative symbiont. Thus, the language around B. burgdorferi as a pathogen, while appropriate in human studies, contradicts the nature of the ecological interactions between B. burgdorferi and arthropod hosts in the enzootic cycle. The non-pathogenic status of B. burgdorferi for I. scapularis and the primary enzootic reservoir host Peromyscus leucopus (the white footed mouse) is not in dispute (Schwanz et al., 2011). Yet even in studies focused on within-tick interactions, B. burgdorferi is often described as pathogenic. Benelli (2020) reviewed the hypothesis of parasite manipulation in tick-borne pathogens, a theory which suggests that tick traits are adaptively altered to improve pathogen fitness, asserting that, particularly for Lyme disease-causing spirochetes, this is a neglected area of research that warrants investigation.
There is increasing evidence that tick-B. burgdorferi association has a beneficial impact on the tick, and in-turn also influences B. burgdorferi transmission success (summarized in Figure 1). Ixodes ricinus ticks infected with B. burgdorferi sensu lato survive longer when exposed to unfavorable temperature and humidity conditions (Herrmann and Gern, 2010), have higher fat content (Herrmann et al., 2013; Couret et al., 2017) and increased walking behavior compared to uninfected ticks (Herrmann and Gern, 2015). Our observations on I. scapularis -B. burgdorferi also phenocopied these observations. We found greater and more rapid engorgement of larval I. scapularis upon successful B burgdorferi acquisition (Couret et al., 2017). B. burgdorferi-infection has also been shown to influence I. scapularis gene expression (Ramamoorthi et al., 2005; Kim et al., 2021; Tang et al., 2021). For example, the increase in expression of Salp15, a tick salivary immunomodulator, was shown to enhance B. burgdorferi transmission to the murine host (Ramamoorthi et al., 2005). Due to the reduced genome of B. burgdorferi, it has to scavenge nutrients such as carbohydrates, amino acids and lipids from it arthropod or mammalian host (Corona and Schwartz, 2015; Kerstholt et al., 2020; Gwynne et al., 2022). Whether the impact of B. burgdorferi on metabolic pathways of the tick (Corona and Schwartz, 2015) and consequent changes in nutritional reserves could have consequences on tick survival, development timing, respiration, locomotion, host-seeking and fecundity remains to be experimentally verified. A molecular and mechanistic understanding of how B. burgdorferi increases lipid and energy reserves in the tick or alters tick gene expressions to facilitate transmission and survival in the host will be critical to gain new insights into tick-B. burgdorferi interactions. This understanding may be exploited to develop strategies towards tick control measures and to prevent B. burgdorferi transmission. For example, key genes/pathways modulated by B. burgdorferi and that allow increased lipid reserves may be utilized to develop reservoir host-targeted vaccines to impair tick survival to reduce tick populations. Conceivably, specific gene functions may also be targeted using small molecule inhibitors-potentially developing specific and novel acaricides. Genes that facilitate B. burgdorferi transmission may be similarly developed as vaccines targeting reservoir host or for human use.
I. scapularis can be coinfected with other human pathogens including Anaplasma phagocytophilum, Borrelia miyamotoi, Babesia microti, and Powassan virus (Stafford et al., 1999; Scoles et al., 2001; Tokarz et al., 2010; Hermance and Thangamani, 2017). The interactions between the tick and these pathogens in the context of biotic and abiotic factors may additionally influence B. burgdorferi survival in the tick and its transmission to the vertebrate host (Ginsberg, 2008). Busby et al. (2012) and Villar et al. (2010) showed that A. phagocytophilum infection of ticks causes the upregulation of stress response proteins such as heat shock proteins (HSP) and Subolesin, an Ankyrin-like transcriptional regulator of multiple cellular functions (de la Fuente et al., 2008). A. phagocytophilum-infected ticks are therefore protected from heat stress and demonstrate increased questing speed (Busby et al., 2012), an adaptation that could increase tick survival by increasing the chances of finding a host to acquire a bloodmeal. B. burgdorferi infection also appears to enhance the ability of ticks to survive desiccation (Herrmann and Gern, 2010; Herrmann et al., 2013), facilitating host seeking over longer distances (Herrmann and Gern, 2015). Whether warming climatic conditions would result in increased survival of ticks coinfected with B. burgdorferi and A. phagocytophilum would require a deeper understanding of interactions between the tick, the vertebrate host, and these pathogens. Neelakanta et al., 2010 showed that A. phagocytophilum-infection of I. scapularis results in increased expression of an anti-freeze glycoprotein and this increase allows ticks to survive cold temperatures significantly better than uninfected or B. burgdorferi-infected ticks. Whether co-infected ticks similarly survive cold stress has not been examined. It is worth noting that while B. burgdorferi colonization of the tick gut is enhanced in the presence of an intact peritrophic matrix (Narasimhan et al., 2014), A. phagocytopilum infection of the tick is enhanced when the peritrophic matrix integrity is compromised (Abraham et al., 2017). These potentially contrasting interactions between tick-B. burgdorferi and tick-A. phagocytophilum during pathogen acquisition by the tick may have functional consequences on the prevalence of coinfected ticks in nature. Consistent with this, Levine and Fish (Levin and Fish, 2001) showed that when larval ticks fed on white-footed mice coinfected with A. phagocytophilum and B. burgdorferi the ability of the larval ticks to efficiently acquire these pathogens is impaired. In contrast, Levine and Fish (Levin and Fish, 2000) showed that coinfection of ticks with A. phagocytophilum and B. burgdorferi did not impact efficiency of transmission of either pathogen to the murine host. Further, prior infection of nymphal ticks by either A. phagocytophilum or B. burgdorferi did not impact acquisition of either pathogen from the murine host suggesting little interaction between these pathogens in the tick vector once they are established in the tick. Interestingly, using a laboratory Mus musculus model of coinfection, Thomas et al. (2001) demonstrated that larval ticks fed on coinfected mice acquired both B. burgdorferi and A. phagocytophilum more effectively when compared to larvae that fed on single-infected mice. These contrasting observations could be due to differences in the immune responses of white-footed mice, the reservoir host, and laboratory mice to infections and co-infections. Ticks can feed on diverse mammalian hosts (McCoy et al., 2013), and it is critical to bear in mind that differential host immune responses may additionally impact infection prevalence in nature.
Highest prevalence of coinfections in ticks in nature are with B. burgdorferi and Babesia microti and is suggested to be in the range of 0-13% in nymphal ticks (Diuk-Wasser et al., 2016; Sanchez-Vicente et al., 2019). Hersh et al. (2014) suggest that this increased coinfection of B. burgdorferi and B. microti is aided in-part due to the reservoir competence of small mammalian hosts such as Peromyscus leucopus to be infected simultaneously with these two pathogens. Further, Dunn et al. (2014) showed that infection of P. leucopus with B. burgdorferi promotes infection, and transmission of B. microti from the mammalian host to the tick vector. Using a Mus musculus model and a different strain of B. burgdorferi Djockik et al. (Djokic et al., 2019) showed that B. burgdorferi restricts B. microti in coinfected mice and exacerbates Lyme disease. Despite the contrasting observations, these studies underscore potential interactions between the two pathogens in the mammalian host. Very little is understood of B. microti-I. scapularis interactions (Antunes et al., 2017). Given the increased prevalence of B. burgdorferi/Babesia microti coinfected ticks and the concurrent risk of human coinfections (Diuk-Wasser et al., 2016), it is important to determine how coinfections with B. burgdorferi and B. microti might influence the transmission of these pathogens to and from the tick. Although co-infections of B. burgdorferi with Powassan virus and Borrelia miyamotoi are less frequent (Tokarz et al., 2010; Diuk-Wasser et al., 2016), the gap in our understanding of the interactions of these pathogens in the tick vector and in the mammalian host needs to be addressed.
In addition to the interplay of different tick-borne pathogens, we must be aware that several genospecies of B. burgdorferi (B. burgdorferi sensu lato species) are maintained in nature enzootically (Swanson and Norris, 2008), including B. burgdorferi in the North America (Derdakova et al., 2004), and B. burgdorferi, B. garinii and B. afzeli in Eurasia (Misonne et al., 1998). There is also significant diversity within these genospecies (Rogovskyy and Bankhead, 2014) and this diversity is stably maintained in reservoir host populations ( (Swanson and Norris, 2008; Walter et al., 2016). Using two distinct Borrelia genospecies, B. burgdorferi and B. afzelii, Bhatia et al. (2018) showed that when infected nymphs feed on seropositive Mus musculus, the infectivity of the homologous spirochete strain is significantly attenuated within the tick gut facilitating the transmission of only the heterologous Borrelia strain to the animals. These observations highlight the seamless interaction between the host-vector and pathogen in the maintenance of B. burgdorferi diversity by ensuring the selection of rare variants of polymorphic surface antigens of the spirochete critical for spirochete fitness in natural hosts.
The life cycle of I. scapularis offers ample opportunity for interaction with environmental microbiota that potentially include virus, protozoa, fungi and bacteria represented in soil and leaf litter (during their off-host phase) or on the skin of the mammalian host (during their on-host phase) (Narasimhan et al., 2021). de la Fuente et al. (2017) and Cabezas-Cruz et al. (2017) highlight the need to investigate the influence of non-pathogenic microbiota in ticks on the tick and B burgdorferi. Since the environment of the tick (be it lab colonies or field samples) is diverse, there is significant disparity among studies on the tick microbiome composition (Narasimhan et al., 2021). The emerging understanding is that there is no core tick microbiome, and that it is transient and variable (Ross et al., 2018). In addition to microbiota acquired from the environment, the tick also harbors endosymbionts that are transovarially transmitted and stably maintained in the tick (Kurtti et al., 2015). Since I. scapularis is an obligate hematophagous arthropod, its diet is lacking in essential B-vitamins including thiamine, pyridoxine, and folate (Duron et al., 2018) and the tick is likely dependent on its microbiota to provide these vitamins. It has been suggested that Rickettsia buchneri, the predominant endosymbiont of I. scapularis, provides the essential vitamins and is critical for tick fitness (Hunter et al., 2015). However, a recent study by Oliver et al. (2021) generated R. buchneri-free ticks by antibiotic treatment and showed that the absence of R.buchneri does not impact development or fecundity. It is likely that antibiotic treatment may not completely cure the ticks of R. buchneri in one cycle of antibiotic feeding. It is likely that a “stress” test might be more revealing of the role R. buchneri in tick biology. It is not known whether R. buchneri impacts B. burgdorferi acquisition ot transmission. Since R. buchneri is an intracellular bacterium and B. burgdorferi is an extracellular bacterium, a direct interaction is unlikely. An earlier study by Zhang et al. (2016) demonstrated that B. burgdorferi does not require thiamine or Vitamin B1, a key cofactor for most living organisms, for its growth and replication and that it may have evolved to live in a Vitamin B-constrained environment of the tick. Sonenshine and Stewart (2021) also raise the possibility that environmental bacteria that form the bulk of the ectosymbionts found in the midgut milieu are also capable of providing essential aminoacids, metabolites and vitamins to the tick and hence influence tick fitness and in-turn the vectorial capacity of the tick. Narasimhan et al. (2014) have shown that changes in the environmental microbiota composition influence larval engorgement and B. burgdorferi colonization of the tick. If so, changes in the composition of environmental microbiota in different regions of the USA would also influence infection prevalence in natural settings. Further, there is mounting evidence that the tick salivary transcriptome and proteome may be differentially expressed on different host species (Tirloni et al., 2017; Narasimhan et al., 2019). Whether this differential expression is signaled by host-specifc immune components in the bloodmeal or by the host skin microbiome and its metabolites at the skin-host interface remains to be investigated. This also underscores the impact of the host species on tick fitness and ultimately success of pathogen transmission. To fully understand how specific environmental microbiota manipulate the tick, approaches to generate aposymbiotic, and gnotobiotic ticks must be developed. Additionally, while tick microbiome studies have been largely bacteriocentric, it is important to examine other potentially key players including fungi, and viruses.
With the understanding that tick saliva contains several immunomodulators that facilitate tick feeding-a process essential for tick-borne pathogens to enter or exit the tick (Francischetti et al., 2009), the concept of saliva assisted transmission or SAT (Nuttall, 2019) has been at the centre stage of research on tick transmission of human pathogens. The availability of the genome sequence of I. scapularis (Gulia-Nuss et al., 2016) has allowed comprehensive cataloging of the salivary and midgut transcriptomes and proteomes and revealed new insights into tick biology and tick-host-pathogen interactions (Chmelar et al., 2016; de la Fuente et al., 2017; Wikel, 2018; Nuss et al., 2021). The last decade has brought into focus the role of the commensal and symbiotic microbiota associated with the tick in the context of tick-pathogen interactions (Bonnet et al., 2017; Narasimhan et al., 2021; Sonenshine and Stewart, 2021). Importantly, the geographic expansion of tick populations, potentially enhanced by climate change (Ogden et al., 2021), has signaled the need to understand how changes in abiotic factors influence the tick, and its pathogenic and commensal microbes. Thus, these multiples factors are entwined and together orchestrate pathogen movement to and from the mammalian host.
As we acknowledge that tick-pathogen interactions are orchestrated by biotic and abiotic factors, it also draws attention to the limitations of laboratory studies. Almost all data published on the influence of tick and B. burgdorferi gene expression have been performed with tick colonies raised under laboratory conditions. Ticks are routinely maintained at constant light cycling, humidity, and temperature conditions. The vast amount of knowledge gathered from controlled laboratory conditions indeed provide critical and foundational insights into tick-host-pathogen interactions. It is not feasible for laboratory studies to attempt to mimic field conditions that include diversity in host species and seasonal variations in abiotic factors and in biotic factors. Nevertheless, we must bear in mind that data collected from tick experiments performed in the laboratory may not fully recapitulate what ensues in ticks collected from the field. Future efforts may benefit from conducting “translational’ studies to calibrate and fine tune laboratory observations to address this limitation.
JC and SN reviewed the literature, wrote sections of the manuscript. SS helped in collecting literature, and in writing. The complete manuscript was edited and revised by all co-authors. All authors contributed to the article and approved the submitted version.
Funding was provided in part by the joint NIH-NSF-NIFA Ecology and Evolution of Infectious Disease award 1R01GM148992-01 and by the USDA National Institute of Food and Agriculture.
The authors declare that the research was conducted in the absence of any commercial or financial relationships that could be construed as a potential conflict of interest.
All claims expressed in this article are solely those of the authors and do not necessarily represent those of their affiliated organizations, or those of the publisher, the editors and the reviewers. Any product that may be evaluated in this article, or claim that may be made by its manufacturer, is not guaranteed or endorsed by the publisher.
Abraham, N. M., Liu, L., Jutras, B. L., Yadav, A. K., Narasimhan, S., Gopalakrishnan, V., et al. (2017). Pathogen-mediated manipulation of arthropod microbiota to promote infection. Proc. Natl. Acad. Sci. United States America. 114 (5), E781–EE90. doi: 10.1073/pnas.1613422114
Antunes, S., Rosa, C., Couto, J., Ferrolho, J., Domingos, A. (2017). Deciphering babesia-vector interactions. Front. Cell. infection Microbiol. 7, 429. doi: 10.3389/fcimb.2017.00429
Arsnoe, I. M., Hickling, G. J., Ginsberg, H. S., McElreath, R., Tsao, J. I. (2015). Different populations of blacklegged tick nymphs exhibit differences in questing behavior that have implications for human lyme disease risk. PloS One 10 (5), e0127450. doi: 10.1371/journal.pone.0127450
Arsnoe, I., Tsao, J. I., Hickling, G. J. (2019). Nymphal Ixodes scapularis questing behavior explains geographic variation in Lyme borreliosis risk in the eastern united states. Ticks tick-borne diseases. 10 (3), 553–563. doi: 10.1016/j.ttbdis.2019.01.001
Benelli, G. (2020). Pathogens manipulating tick behavior-through a glass, darkly. Pathogens 9 (8), 664. doi: 10.3390/pathogens9080664
Benoit, J. B., Patrick, K. R., Desai, K., Hardesty, J. J., Krause, T. B., Denlinger, D. L. (2010). Repeated bouts of dehydration deplete nutrient reserves and reduce egg production in the mosquito culex pipiens. J. Exp. Biol. 213 (Pt 16), 2763–2769. doi: 10.1242/jeb.044883
Berger, K. A., Ginsberg, H. S., Dugas, K. D., Hamel, L. H., Mather, T. N. (2014). Adverse moisture events predict seasonal abundance of Lyme disease vector ticks (Ixodes scapularis). Parasites vectors. 7, 181. doi: 10.1186/1756-3305-7-181
Bertrand, M. R., Wilson, M. L. (1996). Microclimate-dependent survival of unfed adult Ixodes scapularis (Acari:Ixodidae) in nature: life cycle and study design implications. J. Med. entomology. 33 (4), 619–627. doi: 10.1093/jmedent/33.4.619
Bhatia, B., Hillman, C., Carracoi, V., Cheff, B. N., Tilly, K., Rosa, P. A. (2018). Infection history of the blood-meal host dictates pathogenic potential of the Lyme disease spirochete within the feeding tick vector. PloS pathogens. 14 (4), e1006959. doi: 10.1371/journal.ppat.1006959
Bonnet, S. I., Binetruy, F., Hernandez-Jarguin, A. M., Duron, O. (2017). The tick microbiome: Why non-pathogenic microorganisms matter in tick biology and pathogen transmission. Front. Cell. infection Microbiol. 7, 236. doi: 10.3389/fcimb.2017.00236
Busby, A. T., Ayllon, N., Kocan, K. M., Blouin, E. F., de la Fuente, G., Galindo, R. C., et al. (2012). Expression of heat shock proteins and subolesin affects stress responses, anaplasma phagocytophilum infection and questing behaviour in the tick, ixodes scapularis. Med. veterinary entomology 26 (1), 92–102. doi: 10.1111/j.1365-2915.2011.00973.x
Cabezas-Cruz, A., Estrada-Pena, A., Rego, R. O., de la Fuente, J. (2017). Tick-pathogen ensembles: Do molecular interactions lead ecological innovation? Front. Cell. infection Microbiol. 7, 74. doi: 10.3389/fcimb.2017.00074
Carroll, J. A., El-Hage, N., Miller, J. C., Babb, K., Stevenson, B. (2001). Borrelia burgdorferi RevA antigen is a surface-exposed outer membrane protein whose expression is regulated in response to environmental temperature and pH. Infection immunity. 69 (9), 5286–5293. doi: 10.1128/IAI.69.9.5286-5293.2001
Chmelar, J., Kotal, J., Karim, S., Kopacek, P., Francischetti, I. M. B., Pedra, J. H. F., et al. (2016). Sialomes and mialomes: A systems-biology view of tick tissues and tick-host interactions. Trends parasitology. 32 (3), 242–254. doi: 10.1016/j.pt.2015.10.002
Corona, A., Schwartz, I. (2015). Borrelia burgdorferi: Carbon metabolism and the tick-mammal enzootic cycle. Microbiol. Spectr. 3 (3), 1128. doi: 10.1128/9781555818883.ch8
Couret, J., Dyer, M. C., Mather, T. N., Han, S., Tsao, J. I., Lebrun, R. A., et al. (2017). Acquisition of Borrelia burgdorferi infection by larval Ixodes scapularis (Acari: Ixodidae) associated with engorgement measures. J. Med. entomology. 54 (4), 1055–1060. doi: 10.1093/jme/tjx053
Crooks, E., Randolph, S. E. (2006). Walking by ixodes ricinus ticks: intrinsic and extrinsic factors determine the attraction of moisture or host odour. J. Exp. Biol. 209 (Pt 11), 2138–2142. doi: 10.1242/jeb.02238
de la Fuente, J., Antunes, S., Bonnet, S., Cabezas-Cruz, A., Domingos, A. G., Estrada-Pena, A., et al. (2017). Tick-pathogen interactions and vector competence: Identification of molecular drivers for tick-borne diseases. Front. Cell. infection Microbiol. 7, 114. doi: 10.3389/fcimb.2017.00114
de la Fuente, J., Maritz-Olivier, C., Naranjo, V., Ayoubi, P., Nijhof, A. M., Almazan, C., et al. (2008). Evidence of the role of tick subolesin in gene expression. BMC Genomics 9, 372. doi: 10.1186/1471-2164-9-372
Derdakova, M., Dudioak, V., Brei, B., Brownstein, J. S., Schwartz, I., Fish, D. (2004). Interaction and transmission of two Borrelia burgdorferi sensu stricto strains in a tick-rodent maintenance system. Appl. Environ. Microbiol. 70 (11), 6783–6788. doi: 10.1128/AEM.70.11.6783-6788.2004
Diuk-Wasser, M. A., Vannier, E., Krause, P. J. (2016). Coinfection by ixodes tick-borne pathogens: Ecological, epidemiological, and clinical consequences. Trends parasitology. 32 (1), 30–42. doi: 10.1016/j.pt.2015.09.008
Djokic, V., Akoolo, L., Primus, S., Schlachter, S., Kelly, K., Bhanot, P., et al. (2019). Protozoan parasite babesia microti subverts adaptive immunity and enhances Lyme disease severity. Front. Microbiol. 10, 1596. doi: 10.3389/fmicb.2019.01596
Dumic, I., Severnini, E. (2018). "Ticking bomb": The impact of climate change on the incidence of Lyme disease. Can. J. Infect. Dis. Med. Microbiol. 2018, 5719081. doi: 10.1155/2018/5719081
Dunn, J. M., Krause, P. J., Davis, S., Vannier, E. G., Fitzpatrick, M. C., Rollend, L., et al. (2014). Borrelia burgdorferi promotes the establishment of babesia microti in the northeastern united states. PloS One 9 (12), e115494. doi: 10.1371/journal.pone.0115494
Duron, O., Morel, O., Noel, V., Buysse, M., Binetruy, F., Lancelot, R., et al. (2018). Tick-bacteria mutualism depends on b vitamin synthesis pathways. Curr. Biol. CB. 28 (12), 1896–902 e5. doi: 10.1016/j.cub.2018.04.038
Fielden, L. J., Lighton, J. R. B. (1996). Effects of water stress and relative humidity on ventilation in the tick Dermacentor andersoni (Acari: Ixodidae). Physiol. Zoology. 69 (3), 599–617. doi: 10.1086/physzool.69.3.30164218
Fleshman, A. C., Graham, C. B., Maes, S. E., Foster, E., Eisen, R. J. (2021). Reported county-level distribution of Lyme disease spirochetes, Borrelia burgdorferi sensu stricto and borrelia mayonii (Spirochaetales: Spirochaetaceae), in host-seeking Ixodes scapularis and Ixodes pacificus ticks (Acari: Ixodidae) in the contiguous united states. J. Med. entomology. 58 (3), 1219–1233. doi: 10.1093/jme/tjaa283
Francischetti, I. M., Sa-Nunes, A., Mans, B. J., Santos, I. M., Ribeiro, J. M. (2009). The role of saliva in tick feeding. Front. bioscience J. virtual library. 14, 2051–2088. doi: 10.2741/3363
Gaff, H., Eisen, R. J., Eisen, L., Nadolny, R., Bjork, J., Monaghan, A. J. (2020). LYMESIM 2.0: An updated simulation of blacklegged tick (Acari: Ixodidae) population dynamics and enzootic transmission of Borrelia burgdorferi (Spirochaetales: Spirochaetaceae). J. Med. entomology 57 (3), 715–727. doi: 10.1093/jme/tjz252
Gillooly, J. F., Brown, J. H., West, G. B., Savage, V. M., Charnov, E. L. (2001). Effects of size and temperature on metabolic rate. Science. 293 (5538), 2248–2251. doi: 10.1126/science.1061967
Ginsberg, H. S. (2008). Potential effects of mixed infections in ticks on transmission dynamics of pathogens: comparative analysis of published records. Exp. Appl. acarology 46 (1-4), 29–41. doi: 10.1007/s10493-008-9175-5
Ginsberg, H. S., Albert, M., Acevedo, L., Dyer, M. C., Arsnoe, I. M., Tsao, J. I., et al. (2017). Environmental factors affecting survival of immature Ixodes scapularis and implications for geographical distribution of Lyme disease: The Climate/Behavior hypothesis. PloS One 12 (1), e0168723. doi: 10.1371/journal.pone.0168723
Ginsberg, H. S., Hickling, G. J., Burke, R. L., Ogden, N. H., Beati, L., LeBrun, R. A., et al. (2021). Why Lyme disease is common in the northern US, but rare in the south: The roles of host choice, host-seeking behavior, and tick density. PloS Biol. 19 (1), e3001066. doi: 10.1371/journal.pbio.3001066
Ginsberg, H. S., Lee, C., Volson, B., Dyer, M. C., Lebrun, R. A. (2017). Relationships between maternal engorgement weight and the number, size, and fat content of larval Ixodes scapularis (Acari: Ixodidae). J. Med. entomology. 54 (2), 275–280. doi: 10.1371/journal.pbio.3001066
Greenstone, M. H., Bennett, A. F. (1980). Foraging strategy and metabolic-rate in spiders. Ecol. 61 (5), 1255–1259. doi: 10.2307/1936843
Gulia-Nuss, M., Nuss, A. B., Meyer, J. M., Sonenshine, D. E., Roe, R. M., Waterhouse, R. M., et al. (2016). Genomic insights into the Ixodes scapularis tick vector of Lyme disease. Nat. Commun. 7, 10507. doi: 10.1038/ncomms10507
Gwynne, P. J., Clendenen, L. H., Turk, S. P., Marques, A. R., Hu, L. T. (2022). Antiphospholipid autoantibodies in Lyme disease arise after scavenging of host phospholipids by Borrelia burgdorferi. J. Clin. Invest. 132 (6), e152506. doi: 10.1172/JCI152506
Hermance, M. E., Thangamani, S. (2017). Powassan virus: An emerging arbovirus of public health concern in north America. Vector borne zoonotic diseases. 17 (7), 453–462. doi: 10.1089/vbz.2017.2110
Herrmann, C., Gern, L. (2010). Survival of Ixodes ricinus (Acari: Ixodidae) under challenging conditions of temperature and humidity is influenced by Borrelia burgdorferi sensu lato infection. J. Med. entomology. 47 (6), 1196–1204. doi: 10.1603/ME10111
Herrmann, C., Gern, L. (2015). Search for blood or water is influenced by Borrelia burgdorferi in ixodes ricinus. Parasites Vectors 8, 6. doi: 10.1186/s13071-014-0526-2
Herrmann, C., Voordouw, M. J., Gern, L. (2013). Ixodes ricinus ticks infected with the causative agent of Lyme disease, Borrelia burgdorferi sensu lato, have higher energy reserves. Int. J. parasitology. 43 (6), 477–483. doi: 10.1016/j.ijpara.2012.12.010
Hersh, M. H., Ostfeld, R. S., McHenry, D. J., Tibbetts, M., Brunner, J. L., Killilea, M. E., et al. (2014). Co-Infection of blacklegged ticks with babesia microti and borrelia burgdorferi is higher than expected and acquired from small mammal hosts. PloS One 9 (6), e99348. doi: 10.1371/journal.pone.0099348
Hunter, D. J., Torkelson, J. L., Bodnar, J., Mortazavi, B., Laurent, T., Deason, J., et al. (2015). The rickettsia endosymbiont of ixodes pacificus contains all the genes of De novo folate biosynthesis. PloS One 10 (12), e0144552. doi: 10.1371/journal.pone.0144552
Hurd, H. (2003). Manipulation of medically important insect vectors by their parasites. Annu. Rev. entomology. 48, 141–161. doi: 10.1146/annurev.ento.48.091801.112722
Iyer, R., Schwartz, I. (2016). Microarray-based comparative genomic and transcriptome analysis of borrelia burgdorferi. Microarrays (Basel). 5 (2), 9. doi: 10.3390/microarrays5020009
Kerstholt, M., Netea, M. G., Joosten, L. A. B. (2020). Borrelia burgdorferi hijacks cellular metabolism of immune cells: Consequences for host defense. Ticks tick-borne diseases. 11 (3), 101386. doi: 10.1016/j.ttbdis.2020.101386
Kim, T. K., Tirloni, L., Bencosme-Cuevas, E., Kim, T. H., Diedrich, J. K., Yates, J. R., 3rd, et al. (2021). Borrelia burgdorferi infection modifies protein content in saliva of Ixodes scapularis nymphs. BMC Genomics 22 (1), 152. doi: 10.1186/s12864-021-07429-0
Kurtti, T. J., Felsheim, R. F., Burkhardt, N. Y., Oliver, J. D., Heu, C. C., Munderloh, U. G. (2015). Rickettsia buchneri sp. nov., a rickettsial endosymbiont of the blacklegged tick Ixodes scapularis. Int. J. systematic evolutionary Microbiol. 65 (Pt 3), 965–970. doi: 10.1099/ijs.0.000047
Lacroix, R., Mukabana, W. R., Gouagna, L. C., Koella, J. C. (2005). Malaria infection increases attractiveness of humans to mosquitoes. PloS Biol. 3 (9), e298. doi: 10.1371/journal.pbio.0030298
Lefevre, T., Thomas, F. (2008). Behind the scene, something else is pulling the strings: emphasizing parasitic manipulation in vector-borne diseases. Infection Genet. Evol. J. Mol. Epidemiol. evolutionary Genet. Infect. diseases. 8 (4), 504–519. doi: 10.1016/j.meegid.2007.05.008
Lemoine, M. M., Engl, T., Kaltenpoth, M. (2020). Microbial symbionts expanding or constraining abiotic niche space in insects. Curr. Opin. Insect science. 39, 14–20. doi: 10.1016/j.cois.2020.01.003
Levin, M. L., Fish, D. (2000). Acquisition of coinfection and simultaneous transmission of Borrelia burgdorferi and ehrlichia phagocytophila by Ixodes scapularis ticks. Infection immunity. 68 (4), 2183–2186. doi: 10.1128/IAI.68.4.2183-2186.2000
Levin, M. L., Fish, D. (2001). Interference between the agents of Lyme disease and human granulocytic ehrlichiosis in a natural reservoir host. Vector-Borne Zoonot 1 (2), 139–13+. doi: 10.1089/153036601316977741
Lighton, J. R. B., Fielden, L. J. (1995). Mass scaling of standard metabolism in ticks - a valid case of low metabolic rates in sit-and-Wait strategists. Physiol. Zoology. 68 (1), 43–62. doi: 10.1086/physzool.68.1.30163917
Lindsay, L. R., Barker, I. K., Surgeoner, G. A., McEwen, S. A., Gillespie, T. J., Robinson, J. T. (1995). Survival and development of Ixodes scapularis (Acari: Ixodidae) under various climatic conditions in Ontario, Canada. J. Med. entomology. 32 (2), 143–152. doi: 10.1093/jmedent/32.2.143
Lord, C. C. (1992). Nymphal Ixodes dammini: models of the temporal abundance patterns. Int. J. parasitology. 22 (6), 759–765. doi: 10.1016/0020-7519(92)90125-5
McCoy, K. D., Leger, E., Dietrich, M. (2013). Host specialization in ticks and transmission of tick-borne diseases: a review. Front. Cell. infection Microbiol. 3, 57. doi: 10.3389/fcimb.2013.00057
Misonne, M. C., Van Impe, G., Hoet, P. P. (1998). Genetic heterogeneity of Borrelia burgdorferi sensu lato in Ixodes ricinus ticks collected in Belgium. J. Clin. Microbiol. 36 (11), 3352–3354. doi: 10.1128/JCM.36.11.3352-3354.1998
Mulay, V. B., Caimano, M. J., Iyer, R., Dunham-Ems, S., Liveris, D., Petzke, M. M., et al. (2009). Borrelia burgdorferi bba74 is expressed exclusively during tick feeding and is regulated by both arthropod- and mammalian host-specific signals. J. bacteriology. 191 (8), 2783–2794. doi: 10.1128/JB.01802-08
Narasimhan, S., Booth, C. J., DePonte, K., Wu, M. J., Liang, X., Mohanty, S., et al. (2019). Host-specific expression of Ixodes scapularis salivary genes. Ticks tick-borne diseases. 10 (2), 386–397. doi: 10.1016/j.ttbdis.2018.12.001
Narasimhan, S., Rajeevan, N., Liu, L., Zhao, Y. O., Heisig, J., Pan, J., et al. (2014). Gut microbiota of the tick vector Ixodes scapularis modulate colonization of the lyme disease spirochete. Cell Host Microbe 15 (1), 58–71. doi: 10.1016/j.chom.2013.12.001
Narasimhan, S., Swei, A., Abouneameh, S., Pal, U., Pedra, J. H. F., Fikrig, E. (2021). Grappling with the tick microbiome. Trends parasitology. 37 (8), 722–733. doi: 10.1016/j.pt.2021.04.004
Neelakanta, G., Sultana, H., Fish, D., Anderson, J. F., Fikrig, E. (2010) Anaplasma phagocytophilum induces ixodes scapularis ticks to express an antifreeze glycoprotein gene that enhances their survival in the cold. J. Clin. Invest. 120 (9), 3179–3190. doi: 10.1172/JCI42868
Nuss, A., Sharma, A., Gulia-Nuss, M. (2021). Genetic manipulation of ticks: A paradigm shift in tick and tick-borne diseases research. Front. Cell. infection Microbiol. 11, 678037. doi: 10.3389/fcimb.2021.678037
Nuttall, P. A. (2019). Wonders of tick saliva. Ticks tick-borne diseases. 10 (2), 470–481. doi: 10.1016/j.ttbdis.2018.11.005
Ogden, N. H., Ben Beard, C., Ginsberg, H. S., Tsao, J. I. (2021). Possible effects of climate change on ixodid ticks and the pathogens they transmit: Predictions and observations. J. Med. entomology. 58 (4), 1536–1545. doi: 10.1093/jme/tjaa220
Ogden, N. H., Bigras-Poulin, M., Hanincova, K., Maarouf, A., O'Callaghan, C. J., Kurtenbach, K. (2008). Projected effects of climate change on tick phenology and fitness of pathogens transmitted by the north American tick ixodes scapularis. J. Theor. Biol. 254 (3), 621–632. doi: 10.1016/j.jtbi.2008.06.020
Ogden, N. H., Bigras-Poulin, M., O'Callaghan, C. J., Barker, I. K., Lindsay, L. R., Maarouf, A., et al. (2005). A dynamic population model to investigate effects of climate on geographic range and seasonality of the tick ixodes scapularis. Int. J. Parasitol. 35 (4), 375–389. doi: 10.1016/j.ijpara.2004.12.013
Ogden, N. H., Pang, G., Ginsberg, H. S., Hickling, G. J., Burke, R. L., Beati, L., et al. (2018). Evidence for geographic variation in life-cycle processes affecting phenology of the Lyme disease vector Ixodes scapularis (Acari: Ixodidae) in the united states. J. Med. entomology. 55 (6), 1386–1401. doi: 10.1093/jme/tjy104
Ogden, N. H., Radojevic, M., Wu, X., Duvvuri, V. R., Leighton, P. A., Wu, J. (2014). Estimated effects of projected climate change on the basic reproductive number of the Lyme disease vector ixodes scapularis. Environ. Health Perspect. 122 (6), 631–638. doi: 10.1289/ehp.1307799
Ojaimi, C., Brooks, C., Akins, D., Casjens, S., Rosa, P., Elias, A., et al. (2002). Borrelia burgdorferi gene expression profiling with membrane-based arrays. Methods enzymology. 358, 165–177. doi: 10.1016/S0076-6879(02)58088-5
Ojaimi, C., Brooks, C., Casjens, S., Rosa, P., Elias, A., Barbour, A., et al. (2003). Profiling of temperature-induced changes in Borrelia burgdorferi gene expression by using whole genome arrays. Infection immunity. 71 (4), 1689–1705. doi: 10.1128/IAI.71.4.1689-1705.2003
Oliver, J. D., Price, L. D., Burkhardt, N. Y., Heu, C. C., Khoo, B. S., Thorpe, C. J., et al. (2021). Growth dynamics and antibiotic elimination of symbiotic rickettsia buchneri in the tick Ixodes scapularis (Acari: Ixodidae). Appl. Environ. Microbiol. 87 (3), e01672-20. doi: 10.1128/AEM.01672-20
Pal, U., Yang, X., Chen, M., Bockenstedt, L. K., Anderson, J. F., Flavell, R. A., et al. (2004). OspC facilitates Borrelia burgdorferi invasion of Ixodes scapularis salivary glands. J. Clin. Invest. 113 (2), 220–230. doi: 10.1172/JCI200419894
Perret, J. L., Guerin, P. M., Diehl, P. A., Vlimant, M., Gern, L. (2003). Darkness induces mobility, and saturation deficit limits questing duration, in the tick Ixodes ricinus. J. Exp. Biol. 206 (Pt 11), 1809–1815. doi: 10.1242/jeb.00345
Perret, J. L., Guigoz, E., Rais, O., Gern, L. (2000). Influence of saturation deficit and temperature on Ixodes ricinus tick questing activity in a Lyme borreliosis-endemic area (Switzerland). Parasitol. Res. 86 (7), 554–557. doi: 10.1007/s004360000209
Radolf, J. D., Caimano, M. J., Stevenson, B., Hu, L. T. (2012). Of ticks, mice and men: understanding the dual-host lifestyle of Lyme disease spirochaetes. Nat. Rev. Microbiol. 10 (2), 87–99. doi: 10.1038/nrmicro2714
Ramamoorthi, N., Narasimhan, S., Pal, U., Bao, F., Yang, X. F., Fish, D., et al. (2005). The Lyme disease agent exploits a tick protein to infect the mammalian host. Nature. 436 (7050), 573–577. doi: 10.1038/nature03812
Randolph, S. E. (2004). Tick ecology: processes and patterns behind the epidemiological risk posed by ixodid ticks as vectors. Parasitology. 129 Suppl, S37–S65. doi: 10.1017/S0031182004004925
Randolph, S. E., Storey, K. (1999). Impact of microclimate on immature tick-rodent host interactions (Acari: Ixodidae): implications for parasite transmission. J. Med. entomology. 36 (6), 741–748. doi: 10.1093/jmedent/36.6.741
Rebaudo, F., Rabhi, V. B. (2018). Modeling temperature-dependent development rate and phenology in insects: review of major developments, challenges, and future directions. Entomol Exp. Appl. 166 (8), 607–617. doi: 10.1111/eea.12693
Revel, A. T., Talaat, A. M., Norgard, M. V. (2002). DNA Microarray analysis of differential gene expression in Borrelia burgdorferi, the Lyme disease spirochete. Proc. Natl. Acad. Sci. United States America. 99 (3), 1562–1567. doi: 10.1073/pnas.032667699
Ribeiro, J. M. (2000). Blood-feeding in mosquitoes: probing time and salivary gland anti-haemostatic activities in representatives of three genera (Aedes, anopheles, culex). Med. veterinary entomology. 14 (2), 142–148. doi: 10.1046/j.1365-2915.2000.00227.x
Rodgers, S. E., Miller, N. J., Mather, T. N. (2007). Seasonal variation in nymphal blacklegged tick abundance in southern new England forests. J. Med. entomology. 44 (5), 898–900. doi: 10.1093/jmedent/44.5.898
Rogers, M. E., Chance, M. L., Bates, P. A. (2002). The role of promastigote secretory gel in the origin and transmission of the infective stage of leishmania mexicana by the sandfly Lutzomyia longipalpis. Parasitology. 124 (Pt 5), 495–507. doi: 10.1017/S0031182002001439
Rogovskyy, A. S., Bankhead, T. (2014). Bacterial heterogeneity is a requirement for host superinfection by the Lyme disease spirochete. Infection immunity. 82 (11), 4542–4552. doi: 10.1128/IAI.01817-14
Roma, J. S., D'Souza, S., Somers, P. J., Cabo, L. F., Farsin, R., Aksoy, S., et al. (2019). Thermal stress responses of Sodalis glossinidius, an indigenous bacterial symbiont of hematophagous tsetse flies. PloS. Negl. Trop. diseases. 13 (11), e0007464. doi: 10.1371/journal.pntd.0007464
Ross, B. D., Hayes, B., Radey, M. C., Lee, X., Josek, T., Bjork, J., et al. (2018). Ixodes scapularis does not harbor a stable midgut microbiome. ISME. J. 12 (11), 2596–2607. doi: 10.1038/s41396-018-0161-6
Sanchez-Vicente, S., Tagliafierro, T., Coleman, J. L., Benach, J. L., Tokarz, R. (2019). Polymicrobial nature of tick-borne diseases. mBio. 10 (5), e02055-19. doi: 10.1128/mBio.02055-19
Sato, Y., Sato, S. (2015). Spring temperature predicts the long-term molting phenology of two cicadas, cryptotympana facialis and graptopsaltria nigrofuscata (Hemiptera: Cicadidae). Ann. Entomological Soc. America. 108 (4), 494–500. doi: 10.1093/aesa/sav036
Savage, V. M., Gillooly, J. F., Woodruff, W. H., West, G. B., Allen, A. P., Enquist, B. J., et al. (2004). The predominance of quarter-power scaling in biology. Funct. Ecol. 18 (2), 257–282. doi: 10.1111/j.0269-8463.2004.00856.x
Schwan, T. G., Piesman, J., Golde, W. T., Dolan, M. C., Rosa, P. A. (1995). Induction of an outer surface protein on Borrelia burgdorferi during tick feeding. Proc. Natl. Acad. Sci. United States America. 92 (7), 2909–2913. doi: 10.1073/pnas.92.7.2909
Schwanz, L. E., Voordouw, M. J., Brisson, D., Ostfeld, R. S. (2011). Borrelia burgdorferi has minimal impact on the Lyme disease reservoir host peromyscus leucopus. Vector borne zoonotic diseases. 11 (2), 117–124. doi: 10.1089/vbz.2009.0215
Scoles, G. A., Papero, M., Beati, L., Fish, D. (2001). A relapsing fever group spirochete transmitted by Ixodes scapularis ticks. Vector borne zoonotic diseases. 1 (1), 21–34. doi: 10.1089/153036601750137624
Sonenshine, D. E., Stewart, P. E. (2021). Microbiomes of blood-feeding arthropods: Genes coding for essential nutrients and relation to vector fitness and pathogenic infections. A Review. Microorganisms. 9 (12), 2433. doi: 10.3390/microorganisms9122433
Stafford, K. C., Massung, R. F., Magnarelli, L. A., Ijdo, J. W., Anderson, J. F. (1999). Infection with agents of human granulocytic ehrlichiosis, Lyme disease, and babesiosis in wild white-footed mice (Peromyscus leucopus) in Connecticut. J. Clin. Microbiol. 37 (9), 2887–2892. doi: 10.1128/JCM.37.9.2887-2892.1999
Swanson, K. I., Norris, D. E. (2008). Presence of multiple variants of Borrelia burgdorferi in the natural reservoir peromyscus leucopus throughout a transmission season. Vector borne zoonotic diseases. 8 (3), 397–405. doi: 10.1089/vbz.2007.0222
Tang, X., Cao, Y., Arora, G., Hwang, J., Sajid, A., Brown, C. L., et al. (2021). The Lyme disease agent co-opts adiponectin receptor-mediated signaling in its arthropod vector. eLife 10, e72568. doi: 10.7554/eLife.72568.sa2
Thomas, V., Anguita, J., Barthold, S. W., Fikrig, E. (2001). Coinfection with Borrelia burgdorferi and the agent of human granulocytic ehrlichiosis alters murine immune responses, pathogen burden, and severity of Lyme arthritis. Infection immunity. 69 (5), 3359–3371. doi: 10.1128/IAI.69.5.3359-3371.2001
Tilly, K., Krum, J. G., Bestor, A., Jewett, M. W., Grimm, D., Bueschel, D., et al. (2006). Borrelia burgdorferi OspC protein required exclusively in a crucial early stage of mammalian infection. Infection immunity. 74 (6), 3554–3564. doi: 10.1128/IAI.01950-05
Tilly, K., Rosa, P. A., Stewart, P. E. (2008). Biology of infection with borrelia burgdorferi. Infect. Dis. Clinics North America 22 (2), 217–234. doi: 10.1016/j.idc.2007.12.013
Tirloni, L., Kim, T. K., Pinto, A. F. M., Yates, J. R., 3rd, da Silva Vaz, I., Jr., Mulenga, A. (2017). Tick-host range adaptation: Changes in protein profiles in unfed adult Ixodes scapularis and amblyomma americanum saliva stimulated to feed on different hosts. Front. Cell. infection Microbiol. 7, 517. doi: 10.3389/fcimb.2017.00517
Tokarz, R., Anderton, J. M., Katona, L. I., Benach, J. L. (2004). Combined effects of blood and temperature shift on Borrelia burgdorferi gene expression as determined by whole genome DNA array. Infection immunity. 72 (9), 5419–5432. doi: 10.1128/IAI.72.9.5419-5432.2004
Tokarz, R., Jain, K., Bennett, A., Briese, T., Lipkin, W. I. (2010). Assessment of polymicrobial infections in ticks in new York state. Vector borne zoonotic diseases. 10 (3), 217–221. doi: 10.1089/vbz.2009.0036
Tosato, M., Nah, K., Wu, J. (2021). Are host control strategies effective to eradicate tick-borne diseases (TBD)? J. Theor. Biol. 508, 110483. doi: 10.1016/j.jtbi.2020.110483
Tsao, J. I., Hamer, S. A., Han, S., Sidge, J. L., Hickling, G. J. (2021). The contribution of wildlife hosts to the rise of ticks and tick-borne diseases in north America. J. Med. entomology. 58 (4), 1565–1587. doi: 10.1093/jme/tjab047
Villar, M., Ayllon, N., Busby, A. T., Galindo, R. C., Blouin, E. F., Kocan, K. M., et al. (2010). Expression of heat shock and other stress response proteins in ticks and cultured tick cells in response to anaplasma spp. Infection Heat Shock. Int. J. Proteomics. 2010, 657261. doi: 10.1155/2010/657261
Walter, K. S., Carpi, G., Evans, B. R., Caccone, A., Diuk-Wasser, M. A. (2016). Vectors as epidemiological sentinels: Patterns of within-tick Borrelia burgdorferi diversity. PloS pathogens. 12 (7), e1005759. doi: 10.1371/journal.ppat.1005759
Wikel, S. K. (2018). Tick-host-pathogen systems immunobiology: an interactive trio. Front. Biosci. (Landmark Ed). 23 (2), 265–283. doi: 10.2741/4590
Keywords: Ixodes scapularis, tick, Borrelia burgdorferi, environmental factors, microbiome
Citation: Couret J, Schofield S and Narasimhan S (2022) The environment, the tick, and the pathogen – It is an ensemble. Front. Cell. Infect. Microbiol. 12:1049646. doi: 10.3389/fcimb.2022.1049646
Received: 20 September 2022; Accepted: 17 October 2022;
Published: 02 November 2022.
Edited by:
Alejandro Cabezas-Cruz, l’alimentation et l’environnement (INRAE), FranceReviewed by:
Peter Kraiczy, Goethe University Frankfurt, GermanyCopyright © 2022 Couret, Schofield and Narasimhan. This is an open-access article distributed under the terms of the Creative Commons Attribution License (CC BY). The use, distribution or reproduction in other forums is permitted, provided the original author(s) and the copyright owner(s) are credited and that the original publication in this journal is cited, in accordance with accepted academic practice. No use, distribution or reproduction is permitted which does not comply with these terms.
*Correspondence: Jannelle Couret, bmNvdXJldEB1cmkuZWR1; Sukanya Narasimhan, c3VrYW55YS5uYXJhc2ltaGFuQHlhbGUuZWR1
Disclaimer: All claims expressed in this article are solely those of the authors and do not necessarily represent those of their affiliated organizations, or those of the publisher, the editors and the reviewers. Any product that may be evaluated in this article or claim that may be made by its manufacturer is not guaranteed or endorsed by the publisher.
Research integrity at Frontiers
Learn more about the work of our research integrity team to safeguard the quality of each article we publish.