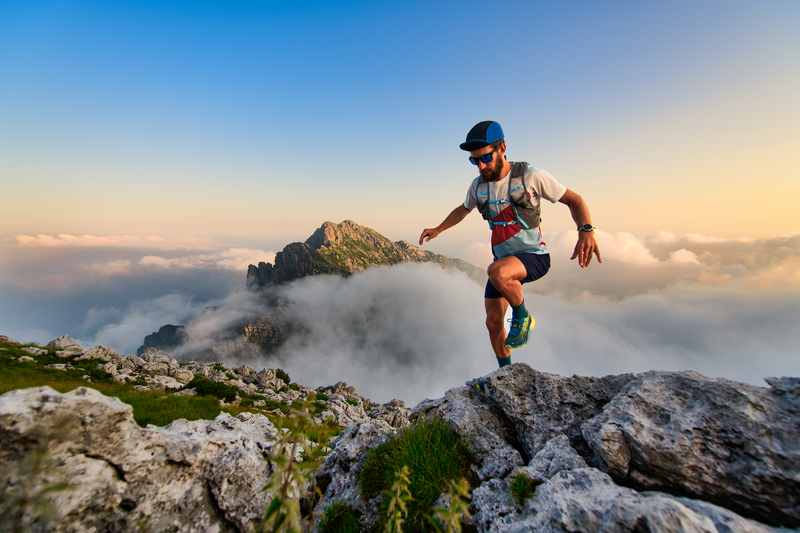
95% of researchers rate our articles as excellent or good
Learn more about the work of our research integrity team to safeguard the quality of each article we publish.
Find out more
ORIGINAL RESEARCH article
Front. Cell. Infect. Microbiol. , 04 January 2023
Sec. Microbiome in Health and Disease
Volume 12 - 2022 | https://doi.org/10.3389/fcimb.2022.1025714
The human microbiota influences physiology, disease, and metabolic reproduction. The origin of uterine bacteria is controversial. The main assumption is that the germs enter the uterine cavity from the vagina through the cervical canal, bloodstream, fallopian tubes, and gynecological surgical channels. Understanding the microbiota at various anatomical sites is critical to the female reproductive system and pregnancy. Today’s study focuses on the role of uterine bacteria in pregnancy and embryo implantation. According to our findings, the uterine microbiome influences embryo implantation and pregnancy outcome. Pregnancy is a natural, evolutionarily selected approach to human reproduction. During pregnancy, the microbiota of the reproductive tract changes, facilitating the maintenance of pregnancy, and the human immune system undergoes a series of changes that recognize and adapt to the non-self. From the beginning of pregnancy, a non-self fetus must establish a placenta of embryonic origin to protect itself and promote growth; the VMB tends to be more stable and lactobacillus-dominated in late gestation than in early gestation. Any material that disrupts this connection, such as microbial changes, is associated with a higher risk of poor health and poor pregnancy outcomes in women (eclampsia). The presence of any material that disrupts this connection, such as microbial changes, is associated with a higher risk of poor health and poor pregnancy outcomes (preeclampsia, preterm birth, gestational diabetes, etc.). In this work, we review the last decade of relevant research to improve our understanding of the mechanisms by which the microbiota of the female reproductive tract influences female reproductive health. This work discusses the mechanisms associated with the reproductive tract microbiota and pregnancy immunity, as well as the impact of an abnormal microbiota on adverse pregnancy outcomes. Emphasis is placed on the characteristics and sources of the female vaginal, uterine, and placental microbiota and the importance of a well-stabilized local human microbiota and immune system for embryo implantation, placental development, fetal growth, and pregnancy outcome.
The human microbiome consists of trillions of microbes living in various parts of the body, including bacteria, archaea, protozoa, fungi, and viruses (the collective genome is called the “microbiome”) (Lloyd-Price et al., 2016; Schirmer et al., 2018). They participate in regulating food metabolism and preserving the integrity of the intestinal epithelium. They are present in the human body as a result of their intricate interaction with numerous elements of host physiology and metabolism. In supporting the host in nutritional absorption and immunological development, they serve a crucial function in protecting the host against infections (Sepich-Poore et al., 2021). The age of multi-omics in humans has increased scientific development in all fields of biology, notably the study of the microbial population and normal flora, during the last decade. In the eighteen years following the release of the first human genome, the Human Microbiome Project (HMP) has shifted its focus from oral and intestinal cultures to the molecular profiling of microbial biochemistry in all human ecological settings (Gill et al., 2006; Costello et al., 2009). Diverse microorganisms have distinct properties in various bodily areas. For instance, higher variety in the gastrointestinal system is generally anticipated, but it may be linked with dysbiosis and an increased risk of adverse outcomes in the female reproductive tract. The Human Microbiome Project (HMP) program consists of two parts (HMP1 and HMP2). HMP2 extends the analysis of host biological characteristics and the microbiome, and a longitudinal cohort study was conducted on three typical microbiome-associated diseases: pregnancy and PTB (vaginal microbiome of pregnant women), Inflammatory Bowel Disease (microbiome), and Prediabetes (intestinal and nasal microbiome). The majority of pregnancy and PTB research has focused on the vaginal microbiome, whereas the uterine microbiome has received very less attention. Therefore, we will further explore the relevance of uterine microbiome to PTB and its research progress.
All individuals involved in this study signed informed consent. The study was approved by Ethics Committee of Shanghai Tongji Hospital and in line with the ethical standards of the Declaration of Helsinki.
Intrauterine secretions were collected from 131 cases of pregnant women during pregnancy with an average age of 52.7 ± 6.25 from the Shanghai Tongji Hospital. In accordance with the diagnostic criteria of the International Federation of Gynecology and Obstetrics (FIGO) systems (2014), patients were examined by two senior or more gynecologists by combined pathogenic microbiology and other examinations. They were diagnosed as having an altered microbial abundance in the uterus in combination with pregnancy, while not having HPV infection or cervical lesions. The samples were stored at -80°C.
In order to perform uterine microbiome analysis of PTB, the V3-4 region of 16S-rRNA gene was amplified and sequenced by Guangzhou Kidio Biotechnology Co.
We retrospectively analyzed the uterine secretions of 131 patients in our fertility center by 16S RNAseq and found that dysbiosis of the uterine cavity microbiota has an impact on implantation and pregnancy outcome (Figure 1).
The majority of women have strains of the genus Lactobacillus that produce lactic acid, and these strains predominate in the vaginal microbiota. Other compounds generated by Lactobacillus in the vaginal milieu, such as hydrogen peroxide and bacteriocin, limit the development of potential pathogens in addition to lactic acid. These molecules are created in the vaginal microenvironment. The changes in vaginal balance in human nature lead to dysbacteriosis, abnormal inflammatory responses, and immune responses that result in the occurrence of many types of diseases of the female reproductive system. In 2011, a landmark study of high-throughput 16S rRNA taxonomic analysis classified vaginal colonization in women of non-pregnant reproductive age into five types, namely CSTI (L. crispatus), CST II (L. gasseri), CST III (L. iners), and CST V (L. jensenii), by Ravel et al. and a fifth - more complex - CST IV (composed mainly of several anaerobic species), CST IV was further subdivided into IVA (dominated by BVAB1), IVB (dominated by Gardnerella vaginalis), IVC0 (dominated by Prevotella), IVC1 (dominated by Streptococcus), IVC2 (dominated by Enterococcus), IVC3 (dominated by Bifidobacterium), and IVC4 (dominated by Bifidobacterium) (dominated by Staphylococcus) (Ravel et al., 2011). In this study, the structure of vaginal microflora was different in different ethnic groups. Studies have shown that vaginal pH often fluctuates. In some women, menstruation or sexual activity may trigger a switch between CSTs at different times (Gajer et al., 2012). Follicular phase estrogen causes vaginal epithelial cells to thicken and secrete more glycogen; this breakdown produces lactic acid and hydrogen peroxide, which lower vaginal PH and facilitate and tend to stabilize the proliferation of lactobacilli, while reducing the number and diversity of other anaerobes (Onderdonk et al., 2016). Menstrual blood neutralizes the acidic vaginal milieu, and a rise in intravaginal PH results in a substantial increase in the number of anaerobic microorganisms as symbionts, with less Lactobacillus (Kaur et al., 2020). Vaginal flora The imbalance can lead to susceptibility to infection or complications in reproduction. When vaginal microecological homeostasis is disturbed, there is an imbalance of vaginal flora, abnormal inflammatory responses and immune responses, etc. This may lead to bacterial vaginosis, intraepithelial neoplasia of the cervix, gynecologic cancers, and other gynecologic diseases. There is now mounting evidence that the vaginal microbiota influences the fetal and neonatal health of pregnant women (Ravel et al., 2011). The most common gynecologic condition in women of reproductive age is vaginal microflora, which is also associated with an increased risk of genital infections, pelvic inflammatory disease, and adverse pregnancy outcomes (including preterm delivery、preeclampsia) (Younes et al., 2018; Fettweis et al., 2019). Several studies in recent years have demonstrated the important role of the female reproductive tract microbiota in the prediction and prevention of preterm birth (Table 1) (Witkin et al., 2019).
Changes in vaginal flora can lead to different pregnancy outcomes. During pregnancy, estrogen levels continue to increase, and lactobacillus plays a greater role. Some studies have shown that changes in the microbiota are associated with PTB, premature rupture of membranes, chorioamnionitis, pregnancy termination, and other adverse pregnancy outcomes (Fettweis et al., 2019). A higher in vitro fertilization result was linked to a lower microbiota index, as measured by examination of vaginal microflora on the day of embryo transfer. Higher levels of Liszt vaginalis were associated with a higher probability of pregnancy at ICSI (Koedooder et al., 2019). In none of these studies was the flora of the upper genital tract sampled and analyzed, so the final results are not very conclusive. Therefore, it is of great importance to improve the study of bacterial flora in the upper genital tract for the emerging study of pregnancy outcomes.
Is there a real fixed microbiota in the uninfected upper genital tract? This concept has long been controversial. As early as 1900, it was agreed that a healthy uterine cavity was sterile. Later, the “germ-free uterus” hypothesis was challenged by several reports in the mid-to late 1980s, even in healthy, asymptomatic women in whom the uterus was found to be enriched with endemic bacteria using a bacterial culture. The cervical mucus suppository can inhibit, but not block, the passage of microorganisms from the vagina through the cervical canal into the uterine cavity (Hansen et al., 2014). Recently, several studies have targeted the putative endometrial microbiota by 16S rRNA sequencing, and each study has documented the presence of the uterine microbiota (Fang et al., 2016; Franasiak et al., 2016; Moreno et al., 2016; Verstraelen et al., 2016; Chen et al., 2017; Miles et al., 2017; Winters et al., 2019; Lozano et al., 2021; Oberle et al., 2021; Wang et al., 2021; Wessels et al., 2021). Table 2 provides an overview of these recent studies over the last 5 years that rely on 16S rRNA sequencing to assess the microbial composition of the human uterus. Franasiak et al. (2016) examined the endometrial microbiota of 33 women by collecting material from catheter tips used for ET during assisted reproductive technology. Lactobacillus and Flavobacterium were identified as the most abundant genera in the nonpregnant and pregnant groups. In a study focused on the relationship between endometrial polyps and local microbiota, In 2016, another pilot study showed that pregnancy rates in women with a non-lactobacillus dominant (NLD) uterine environment dropped by nearly 40 percent after uterine microbial composition was identified as a new focus (Moreno et al., 2016). Many scientists have attempted to describe the natural microbiota of the uterus to determine what impact the uterine microbiota has on pregnancy outcomes (Chen et al., 2017).
DNA kits have their own unique microbiome called the “dragon group” (de Goffau et al., 2018). Researchers have indicated that low levels of kit contamination are not detected in high biomass sites, such as the intestine, while when examining extremely low biomass sites, such as the placenta or uterus, results can be confounded by the kit microbiome (Kim et al., 2017). There are studies both for and against microbial colonization of the upper genital tract, and more recently, there are studies suggesting that the uterus lacks its own microbiome (Perez-Muñoz et al., 2017). In 2019, Theis KR (Theis et al., 2019)performed multiple microbiological examinations in a study of the microbiota of cesarean placenta specimens and found no normal microbiota; the results of another study also demonstrated no microbiota in prenatal fetal feces (Kennedy et al., 2021). However, it is known that pathogenic microorganisms can cause infections of the placenta (Payne and Bayatibojakhi, 2014), uterus, fallopian tubes (Lenz and Dillard, 2018), and ovaries (Brunham et al., 2015), which affect female reproductive health and pregnancy outcomes. Therefore, further studies are needed to confirm whether the native microbiome is indeed present in the upper female reproductive tract and how it is distributed.
How can one account for the origins of the microbiome found in the uterus? Several hypotheses have been put forward to explain this. One popular idea proposes that bacterial vaginal infections may spread to the uterus if they go up the cervical canal (Figure 2). While there is a cervical mucus plug that blocks the transmission of bacteria to protect the uterine environment, we know that during sex, semen can enter the uterus through the cervical mucus in a small channel. Studies have shown that uterine pumps can deliver radioactive isotopes from the vagina to the uterus within 15 minutes of intercourse. Consistent with this hypothesis, a study in pregnant mice observed that bioluminescent E. coli ascended from the vagina into the uterine cavity and resulted in preterm delivery (Suff et al., 2018). However, there are significant anatomical and physiological differences between the female reproductive tract of mice and that of humans, making it difficult to generalize this observation to the human population.
Figure 2 Womb microbiota transmission pathways. (A) scension from vagina; (B) Hematogenous spread of bacteria; (C) Digestive tract transmission; (D) Other e.g. retrograde spread through fallopian tubes,ART-related gynecologic procedure etc.
Second, the possible routes of transmission are presumed blood-based transmission of intestinal and oral microbiota or other bacteria produced by the bloodstream and transmission by retrograde peristalsis of the fallopian tube into the uterine cavity. Periodontitis has been identified as a risk factor for preterm birth, and similar microbial colonization in the mouth has been found in preterm infants (Dörtbudak et al., 2005). Another study showed that a genetically labeled Enterococcus faecalis introduced into the oral cavity of mice could also be detected in the placenta (Jiménez et al., 2008). Other routes of transmission include gynecologic assisted reproductive technology procedures, such as oocyte retrieval, which can introduce vaginal bacteria into the uterus (Pereira et al., 2016); insertion or removal of intrauterine devices can introduce bacteria into the uterus (Sparks et al., 1981). Given these possible origins of the uterine microbiota, it is important to understand the microbiota at different anatomical sites for the female reproductive system and pregnancy outcomes.
Numerous investigations have pointed to bacterial infections as the root cause of PPROM and PTB as their respective causes. Prior to the year 2014, it was generally believed that the placenta did not have its own unique microbiome. On the placental side, an inflammatory condition known as chorioamnionitis may occur. The bacteria Bacteroides, E. coli, Gardnerella vaginalis, Mycoplasma hominis, Streptococcus, and Ureaplasma urealyticum are the most prevalent types of infectious agents. This lends credence to the hypothesis that pathogenic bacteria are capable of penetrating the amniotic and choronic membranes of the placenta (Shane et al., 2017). The idea that the placenta contains its own healthy microbiome first emerged after the groundbreaking publication by Aagaard et al. in 2014 (Aagaard et al., 2014). The results show that the placenta contains a microbiome with low abundance but high metabolic content. The placental microbiota is mainly composed of non-pathogenic commensal microbiota of Firmicutes, Ascomycetes, Proteobacteria, Bacteroidetes, and Fusobacteria. About 100 recent studies that have begun to examine the placental microbiome and its macrogenetic data have all found that it has a unique microbiota. Another recent study found that the uterine microbiota may help regulate the local immune system in preparation for embryonic implantation and placental formation (Benner et al., 2018), which could directly impact the development of PE. So how do bacteria enter the placenta? The currently proposed hypothesis is that colonization of the placenta occurs by three possible routes: first, by upstream migration from the vagina (Goldenberg et al., 2008), second, by hematogenous spread from the intestine (Perez et al., 2007), and finally, by hematogenous spread from the oral cavity (Fardini et al., 2010). However, several recent studies have challenged the idea of a neonatal and placental microbiota. Perez-Munoz et al. (Perez et al., 2007) argued that next-generation sequencing methods are limited by biomass, infection, and kit infection, and the accuracy of the measured data is questionable, so they do not support the presence of a microbiota in a healthy placental environment. Lauder et al. (2016) used PCR and Illumina sequencing to compare a series of contaminated controls to healthy placental samples and found that placental samples contained low and indistinguishable 16S rRNA copy numbers compared to extracted blanks using two different DNA extraction methods. There was also a follow-up study by Leiby et al. (2018) that examined placentas from spontaneous preterm births and found no difference between negative background controls and placenta samples. A recent groundbreaking study demonstrated that the resident microbiota in the placenta cannot be identified. This was found after researchers carefully screened for possible contaminants through a series of tests, including culture, qPCR, 16S rRNA gene sequencing, and shotgun metagenomics. The study was published in the journal Science. Even though this study was done with the most advanced technology available at the time, it is almost impossible that there is no link between the placental microbiota and the uterine microbiota if the uterine microbiota is present, as a large number of studies have shown. It is conceivable that the placental microbiota cannot be detected at present because of the very low biomass or the inadequacy of the technology available today. However, it is too early to say that there is no way to do so.
Pregnancy is a normal physiological process in which the female body undergoes tremendous metabolic, immunological, and endocrine changes as it enters the reproductive phase (Soma-Pillay et al., 2016). At the same time, the structure, composition, and abundance of microbial communities that colonize different parts of the body change. In early pregnancy, a completely new microbiota (Atopobium, Aerococcus, Gemella, Sneathia, Parvimonas, Gardnerella, and Megasphaera) is observed in the vaginal microenvironment, with a marked increase in the diversity of the vaginal flora and a relative decrease in Lactobacillus abundance (Kaur et al., 2020). As pregnancy progresses, estrogen levels gradually increase during mid-pregnancy and stabilize in late pregnancy. Estrogen promotes vaginal epithelial thickening and intracellular glycogen production, which can be hydrolyzed into glucose and maltose by host α-amylase, and Lactobacillus ferments glucose and maltose into lactic acid, supporting the value-added production of members of the genus Lactobacillus, and since then, Lactobacillus has become an important colony in the reproductive tract in mid- and late pregnancy. Since then, Lactobacillus has become an important colony in the reproductive tract in mid and late pregnancy and provides microenvironmental stability of the reproductive tract throughout pregnancy (Hickey et al., 2012). It has been found that the composition of the reproductive tract microbiota and its diversity are important predictors of pregnancy outcome. The diversity of vaginal microbiota is significantly higher in women with preterm delivery than in women with full-term pregnancy, and variations in “species level” (especially in Lactobacillus) are also closely associated with preterm delivery, such as Stimia, TM7-H1, and BVA-B1 (bacteria associated with bacterial vaginosis) (Fettweis et al., 2019). The dynamic composition of the microbiome and functional differences are currently hot topics in preterm birth research. Finally, maternal estrogen levels decrease rapidly at the time of delivery of the fetal placenta. The stability of the VMB is also disrupted by the mixing of fetus, amniotic fluid, placenta, and blood in the vagina, and studies have found that it can take several months for the vaginal VMB to return to a steady state, even in asymptomatic women. There is research to suggest that pregnancy intervals less than 1 year apart are associated with an increased risk of pregnancy complications (preterm births, etc.) (Shachar et al., 2016; Kangatharan et al., 2017)。
Pregnancy is a battle against the immune system for human reproduction, because a fetus (which is not derived from a different gene from either parent) must be maintained throughout the gestation period. Like many other living things, mammals use the placenta, which develops from the embryo, to protect and nourish the developing fetus. Fertilization in the fallopian tube sets in motion a complex series of events that must be completed before the embryo can successfully implant in the uterine lining. There are three crucial phases in the implantation of a fertilized egg: the in situ phase, attachment (adhesion), and penetration (invasion). The immune system plays a critical role at each stage of embryonic development by generating a healthy, viable fetal egg as a mother cell that carries the maternal antigen, which is recognized by the immune system as “self.” When a sperm reaches an egg, it penetrates the fallopian tube ampulla and sets off a cascade of events leading to the development of maternal and paternal antigens. In the early stages of development, the embryo is considered a maternal cell before it begins to present its own foreign antigen. The embryo is protected from the mother’s immune cells by the zona pellucida until it is ready to transfer its antigen. In addition, certain maternal cumulus cells may provide protection during the early stages of life. After the first few days, the embryo must make contact with the maternal system. When a donor embryo is successfully implanted, the embryo must communicate to prevent attack by the maternal immune system. This communication occurs 4-5 days after embryo transfer. During this period, a series of events take place between the offspring and the mother, such as implantation into the endometrium, immune tolerance, and finally implantation (Bardos et al., 2019). We speculated that the mechanism of the uterine microbiome in human pregnancy immunity might be as follows (Bardos et al., 2019): (1) the local microbiome may affect pregnancy with clinical complications. It could be that the microbiome causes alterations in regional signaling pathways. An altered microbiome may trigger aberrant inflammatory responses via uterine Toll-like receptors, which may alter the cytokine milieu, altering local responses to pro-inflammatory and anti-tolerant immune cell responses. (2) It may alter the integrity of the epithelial barrier of the endometrium. Some pathogens cause a local decrease in matrix-degrading proteins, which may affect the placenta. (3) The local human microbiome has a competitive advantage. If it adapts and becomes the best nutrient scavenger in the region, it can eliminate potential invasive species through competitive exclusion. (4) Local microorganisms excrete certain metabolites, such as short-chain fatty acids, which inhibit the growth of certain species. Multiple modalities exist that would be used to selectively circumvent or engage different aspects of the immune response. The microbiota of the uterus may potentially regulate the immune cell subsets required for implantation and have implications for histomorphology (Benner et al., 2018).
Immune cells in the female genital tract play a contradictory role, both maintaining endogenous immunity to infection and creating immunological tolerance to sperm and embryo/fetus in the upper vaginal tract. Congenital lymphocytes (ILCs) are the largest population of maternal leukocytes that accumulate at the maternal-fetal interface and contribute to decidualization and implantation; NK cells are known to arise from a subset of the various ILC developmental pathways. Decidual natural killer (DNK) cells, unlike circulating NNK cells, release a variety of growth factors, angiogenic factors, and cytokines. In early pregnancy, decidual basal cells (site of implantation and trophoblast invasion/transformation) and decidual natural killer cells (DNK) make up the majority of immune cells (70%), followed by decidual macrophages (20–25%) and T cells (3–10%) (Liu et al., 2017). It is generally accepted that decidual natural killer cells (DNK) and regulatory T cells (Treg) play a critical role in promoting fetal angiogenesis, trophoblast migration, and immunological tolerance. The imbalance of immune cells in the endometrium/decidua is closely related to infertility, miscarriage, and other obstetric complications (Lee et al., 2015). The endometrium is composed of a number of immune cells, such as mast cells, macrophages, neutrophils, dendritic cells, T cells, and B cells. The composition of immune cells and cytokines at the maternal-fetal interface is in a dynamic state. Increased production of inflammatory cytokines and accumulation of decidual immune cells define the “pro-inflammatory” milieu of early pregnancy, which is essential for embryo implantation and placental development. The “anti-inflammatory phenotype” of the maternal-fetal interface develops between weeks 14 and 26 of gestation. Tolerance and maintenance of normal fetal growth depend on the development of M2-type macrophages, natural killer (NK) cells, and regulatory T cells (Tregs) in the deciduum (Yang et al., 2019).
NK cells are the most abundant cells in maternal-fetal immune environment, accounting for 70% of decidua leukocytes in early pregnancy. CD56 bright CD16 phenotype was found in most of the NK cells in decidua. There are three main theories about the origin of decidual NK (DNK) cells: 1 Carlino et al. suggest that DNK cells mainly come from the recruitment of PNK cells under the action of Chemokine receptor in the uterus; And this process depends on progesterone (Carlino et al., 2008). 2 vacca et al. suggested that the interaction of various molecules in the maternal-fetal environment may induce CD34+ precursor cells in decidua to differentiate into DNK cells (Vacca et al., 2011). 3manaster et al. suggest that DNK cells are immature NK cells in the endometrium, which are activated by Il-15. The origin of DNK cells may be the result of the combined action of multiple mechanisms, which participate in the maintenance of pregnancy (Manaster et al., 2008). Numerous growth factors, angiogenic factors, and cytokines can be produced by DNK cells. Substances such as interferon-gamma, vascular endothelial growth factor, and tumor necrosis factor are secreted by mature DNK cells to support decidual remodeling and implantation of blastocysts. DNK also supported EVT invasion by releasing IL -8 and CXCL10. Binding of HLA-G (expressed on EVT) to the inhibitory receptor KIR2DL4 regulates DNK cell cytotoxicity. Maternal intolerance can be avoided thanks to DM phagocytizing apoptotic trophoblast cells and generating Ido, which inhibits T cell activation. To some extent, Treg cells control how active APC and effector T cells are. Exosomes secreted by syncytiotrophoblasts produce TRAIL and FASL, and the lack of MHC expression by this cell type also helps promote maternal tolerance. These cytokines promote maternal blood supply to the implantation site, stimulate trophoblast invasion, and help remodel the decidua and spiral arteries (Hanna et al., 2006). In a mouse model, interferon-γ (IFN-γ) secreted by DNK induces dilation of spiral arteries (Ashkar and Croy, 1999). The deletion of DNK cells during mouse pregnancy is related to the decrease of fetal viability and the abnormal formation of decidual structure and spiral artery at the site of implantation (Hofmann et al., 2014). Similarly, endometrial biopsies found significantly fewer NK cells in patients with unexplained infertility than in fertile women (Klentzeris et al., 1994). Interestingly, DNK cells exhibiting enhanced IFN-γ and VEGF α production by subcohorte were recently identified to be highly enriched in women with multiple births. These pregnancy-associated DNK (PTdNK) cells are transcriptionally distinct from other DNK cells and have higher expression of genes associated with NK cell activation, growth factors, and immunomodulatory proteins (Gamliel et al., 2018) (Figure 3).
The various different types of T cells are part of the adaptive immune response. T helper cells (Th) are activated when they bind to T cell receptors (TCRs) to recognize peptide antigens of the major histocompatibility complex (MHC) class II presented on antigen-presenting cells (APCs). When stimulated, Th cells proliferate and/or form effector cell clones that are individually highly specialized for a particular MHC-II antigen class. These CD4+ Th effector cells can be divided into four distinct subsets. The different types have different functional properties based on the cytokines they secrete. Based on their phenotype, these cells are classified as either type 1 T helper cells (Th1), type 2 T helper cells (Th2), type 17 T helper cells (Th17), or regulatory T helper cells (Treg) (Zhou et al., 2009). Interferon and tumor necrosis factor are two cytokines produced by Th1 cells. Because of their ability to eliminate cancer cells and protect against intracellular infection by viruses, bacteria, and microorganisms that develop in macrophages, these cells have found wide application. Interleukin (IL)-4, IL-5, IL-10, and IL-13 are secreted by Th2 cells; these cytokines stimulate antibody formation and help fight parasites. IL-4, IL-5-induced eosinophilic granulocytes, and IL-3 and IL-4-stimulated mast cell proliferation and degranulation make parasites vulnerable, while Th2 cells activate B cells that can resist immunoglobulin (IG) production. A third group, called Th17 cells, plays a critical role in tissue inflammation and neutrophil activation to fight extracellular pathogens by secreting IL-17, IL-17F, IL-6, IL-22, and TNF-a.The triggering of immune-mediated inflammatory tolerance and negative regulation is largely dependent on their presence. By and large, Th1 cells facilitate cellular immune responses, whereas TH2 cells enhance humoral responses. Cytokines released by Th1, Th2, and Th17 populations compete with each other, and at any given time, the response of one subtype to a particular pathogen is more pronounced than that of the others (Kaiko et al., 2008). The local microbiota can influence TGF-β and increase Th17 or Treg cell populations (Brown et al., 2019). Treg cell dysfunction is associated with autoimmunity, inflammation, allergy, and acute and chronic infections (Figure 4).
How can an embryo convince the mother to accept itself? Treg cells have been found to acquire “non-self” antigens presented to them by downregulating Th1 and Th17 responses and promoting active tolerance to these antigens (Shevach, 2002). Treg cells inhibit the additional proliferation of T cells and the production of proinflammatory cytokines by producing TGF-β and IL10. They also suppress dendritic cell and macrophage development and activation, as well as B cell proliferation, antibody formation, and NK cell cytotoxicity (Ghiringhelli et al., 2005).As early as possible after conception, a critical period for the survival of developing embryos, Treg cells are attracted to the uterus. This is the critical period for the embryo to interact directly with the maternal immune system, such as during orthotopia, implantation, and early placental morphogenesis. Poor immune tolerance can lead to inadequate implantation, which can be the cause of intrauterine growth retardation and pregnancy termination (Maltepe et al., 2010). In addition, PEC, a common condition in late pregnancy, is associated with difficulties in placental development. A shift in transmission toward Th1 cells and away from Treg cells may be induced by the local microbiome of the intima. For example, bacterioides are thought to increase the number of Th1 cells in the body. The upregulation of Th1 cells leads to an enhancement of the local immune response and causes more local inflammation. As mentioned earlier, implantation and pregnancy outcome could be affected by increased inflammation of the endometrium. However, a healthy microbiome locally in the uterus can boost Treg cells, dampen the immune response, and induce pregnancy-or species-specific tolerance (Baker et al., 2018), thus maintaining a healthy pregnancy state and outcome.
After 20 weeks of gestation, preeclampsia (PEC) may develop, a multisystemic complication of pregnancy defined by new-onset hypertension with proteinuria or systemic disease primarily affecting the liver and kidneys. PEC currently affects 2 - 8% of global pregnancies (Jeyabalan, 2013). PEC has a complex etiology involving both maternal and fetal influences. However, the entire mechanism has not yet been deciphered. Insufficient tolerance of immune adaptations between maternal, paternal, and fetal tissues; implantation of the placenta with abnormal trophoblast infiltration; oxidative stress leading to endothelial cell dysfunction; and genetic factors, including susceptibility genes and epigenetic effects, are currently the four most likely explanations for the development of PEC. Impaired vascular function and activation of systemic inflammation in numerous maternal organs define this condition, which can be exacerbated by a number of circumstances, including infection. One of the main causes of placental dysfunction is a problem with the blood vessels in the uterus (Chaiworapongsa et al., 2014). Normal female pregnancy is associated with many hormonal, metabolic, and immunologic changes, and alterations in any of these factors may be associated with other conditions outside pregnancy, such as preeclampsia. The pathogenesis of PEC has been studied by many scientists in a variety of ways (Maynard et al., 2003; Levine et al., 2004; James et al., 2010; Verlohren et al., 2012; Souders et al., 2015; Widmer et al., 2015; DiGiulio et al., 2015; Agrawal et al., 2019; Umapathy et al., 2020). The influence of microorganisms on the multifactorial pathway of PEC has also been the focus of recent scientific studies. Microorganisms and their derivatives can trigger infections and inflammatory responses through the production of antigens and other inflammatory factors. Bacterial communities in the oral cavity, intestine, vagina, cervix, and uterus of the mother, as well as microbial communities in the placenta and amniotic fluid, may be involved in the development of PEC. A meta-analysis of epidemiological studies has shown that any viral or bacterial infection is associated with a higher risk of PEC (Rustveld et al., 2008). Another meta-analysis found that both periodontal disease and urinary tract infections during pregnancy increased the risk of PEC (Conde-Agudelo et al., 2008). Polymerase chain reaction (PCR) and next-generation sequencing showed that 12.7 percent of placental samples from women with PEC were PCR-positive for the 16S rRNA gene. Listeria monocytogenes, Salmonella, and Escherichia coli were just some of the commensal and pathogenic bacteria revealed by the samples’ microbiome (Amarasekara et al., 2015). The original theory was that an overgrowth of Bacillus or thick-walled bacteria might contribute to the development of maternal obesity. A common consequence of maternal obesity is ecological dysbiosis of the gut and “leaky gut syndrome” (Fändriks, 2017; Taddei et al., 2018). Leaky gut syndrome is defined as a weakening or failure of the epithelial barrier of the gut and is caused by stress, chronic inflammation, and dysbiosis of the gut flora. This can lead to an exacerbation of the inflammatory state during pregnancy, resulting in abnormal placental development and PEC. Maternal obesity has been shown to be an important predictor of childhood obesity and metabolic complications in the offspring. Although these mechanisms have not been fully elucidated, researchers suggest that the microbiome alters metabolism during pregnancy and modulates weight gain in both the mother and offspring (Gohir et al., 2015). On the other hand, studies have shown that long-term intake of milk-based probiotics appears to be beneficial in reducing the incidence of PEC, as they may alter the inflammatory basis of severe PEC (Nordqvist et al., 2018). Overall, these findings support the idea that microbial interactions play a role in the development of PEC and demonstrate that bacteria may contribute to inflammatory responses. Therefore, the study of the influence of bacteria on the multifactorial pathway of PEC has been a hot topic of scientific research in recent years. It has been suggested that the “gut-placenta” axis may play an important role in the etiology of PEC and that dysbiosis of gut ecology may cause the development of preeclampsia and affect the own blood pressure of women with PEC (Chen et al., 2020). We believe that a possible mechanism of pathogenesis may be that translocation of bacteria or their components to the intrauterine environment may promote inflammatory and abnormal immune responses in the placenta and maternal circulation (Chen and Gur, 2019). Although the underlying mechanisms leading to alteration of the microbiota are not known, changes in the mucosal surface of the immune system can alter the microbiota (Dunn et al., 2019). However, the specific microorganisms that can trigger PEC are still controversial. The different composition and aggregate abundance of the above maternal microbiota may contribute to impaired placental trophoblast function, leading to endothelial dysfunction and oxidative damage to nutrients and the placenta, ultimately resulting in increased maternal blood pressure, proteinuria, and PEC development (Amarasekara et al., 2015)。
Preterm birth (PTB) is a birth of less than 37 weeks of gestation due to multiple causes, including medically induced and spontaneous preterm births.PTB is the most common cause of neonatal morbidity and mortality worldwide (17.7%) (Perin et al., 2022). It is estimated that up to 25-40% of spontaneous preterm births are caused by microorganisms, and intrauterine infections caused by microorganisms can activate the innate immune system, thus increasing the risk of spontaneous preterm birth score (Goldenberg et al., 2008; Callahan et al., 2017). On the maternal mucosal surface, microorganisms secrete A2 phospholipase, which is converted into prostaglandins (PG, PGE2, PGF2A) and secretes endotoxins that stimulate a cascade of pro-inflammatory cytokines at the maternal-fetal interface leading to degradation of the collagen matrix of the cervix, fetal membranes, placenta and uterus triggering preterm labor (Yang et al., 2015). We know that with increasing weeks of gestation, the vaginal microbiome is generally dominated by Lactobacillus as the dominant flora in mid- to late pregnancy (Serrano et al., 2019). Several studies have detected Ureaplasma, Gardnerella, Clostridium sensu, Aerococcus, BVAB1 and BVAB2 flora in intrauterine infections and can induce spontaneous PTB (Table 1) (Kosti et al., 2020). Although we are not sure whether vaginal microorganisms cause intrauterine infections through upstream transmission, increased relative abundance of several microorganisms, for example, Ureaplasma and certain BV-associated taxa, were authentically detected in the VBM of women with preterm labor. Thus, it was hypothesized that microbial pathogens may enter the uterine cavity through the four aforementioned pathways, thus leading to uterine infections inducing preterm labor. We found that many PTB-associated flora are components of CST IV, such as Gardnerella, Atopobium, Dialister, Prevotella, and Sneathia. It is believed that CST IV is positively correlated with PTB. Despite the overwhelming evidence supporting the role of vaginal flora in PTB, the mechanism remains poorly understood. Several groups of studies have reported an association between the absence of Lactobacillus or poor vaginal flora and local inflammation at the cervicovaginal interface in the context of PTB, such as the analysis of cervicovaginal cytokines, chemokines and β-defensins (Fettweis et al., 2019; Elovitz et al., 2019; Florova et al., 2021). The role of the complement system in microbially driven PTB was recently proposed by Chan D in February 2022, who found that a decrease in vaginal Lactobacillus species and elevated bacterial diversity resulted in increased mannose-binding lectin (MBL), specific bacterial DNA, IgM, IgG, C3b, C5, IL-8, IL-6 and IL-1β and increased the risk of PTB (Payne et al., 2021; Chan et al., 2022). Various parameters such as cervical length (Gudicha et al., 2021), VBM composition (Table 1), and local expression of inflammatory cytokines (Wei et al., 2010; Fettweis et al., 2019)have been reported nowadays to predict PTB.In conclusion, microorganisms play an important role in the study of prevention of preterm birth in pregnancy.
The metabolic requirements of the developing fetus are met by the usual physiological adaptations of pregnancy. Gestational diabetes mellitus (GDM) is characterized by hyperglycemia and carbohydrate intolerance throughout pregnancy. Up to fourteen percent of pregnant women develop gestational diabetes, a distinct group of metabolic diseases (Szmuilowicz et al., 2019). There is already evidence of a link between microbiome and metabolism. The researchers speculate that microbiome may affect pregnancy metabolism and the development of GDM (Kuang et al., 2017). The microbes that colonize the gut affect nutrient, hunger, satiety, and lipid and glucose metabolism. Researchers examined the microbiome associated with gestational diabetes (Crusell et al., 2018). In different studies, it was found that patients with GDM had a higher frequency of microecological dysbiosis and a lower overall microbial diversity. More pathogens, including more viral and fungal species, were found in patients with GDM (Wang et al., 2018). We hope that the relationship between microecological disorder and gestational diabetes mellitus needs further study in the future.
It is a new concept that the microbial community can influence the function of the host immune system and that the host immune system and the microbial community maintain a symbiotic relationship. Bacteria in other mucous membranes, such as the intestinal mucosa, are often confined within the lumen of the epithelial lining, although they can sometimes break through this physical barrier and cause infection. After entering the body, these commensal bacteria are taken up by resident macrophages and then transported by DCS to local lymphoid aggregates that drive the adaptive immune response. The human immune system has different abilities to defend against external pathogens in different parts of the female genital tract. There are differences between the lower genital tract (the vagina and vaginal part of the cervix) and the upper genital tract (the uterus and endocervix). Like the intestine, the vagina consists of a symbiotic flora in which the immune system must allow the growth of beneficial microorganisms while preventing the growth of pathogens. The human immune system has different capacities to defend against external pathogens in different parts of the female genital tract, with differences between the lower genital tract (vagina, vaginal part of the cervix) and the upper genital tract (uterus, endocervix). Like the intestine, the vagina consists of a symbiotic flora in which the immune system must allow the growth of beneficial microorganisms while preventing the growth of pathogens.
In summary, a good and stable human microbiome can play an important role in the local interaction between the embryo and the endometrium, and can influence implantation, placentation, and embryo growth, ultimately affecting the course and outcome of pregnancy.
The original contributions presented in the study are included in the article/supplementary material. Further inquiries can be directed to the corresponding authors.
All individuals involved in this study signed informed consent. The study was approved by Ethics Committee of Shanghai Tongji Hospital and in line with the ethical standards of the Declaration of Helsinki.
The authors LS, LH, and AS conducted bibliographic research and authored the manuscript. WW, WH, CJ, and YY gathered patient data and conducted statistical analysis. All authors participated to and approved the final draft of the research.
This work was supported by the Changning Maternity and Infant Health Hospital, East China Normal University, Shanghai Medical Key Specialty Project (ZK2019B02)&Project (2022Y-2); the Postdoctoral Science Foundation of China (2020M681399); the Zhejiang Medicine and Health Science and Technology Program Project (2021KY277), the Medical Research Project of Jiangsu Provincial Health and Health Commission (2019179).
The authors declare that the research was conducted in the absence of any commercial or financial relationships that could be construed as a potential conflict of interest.
All claims expressed in this article are solely those of the authors and do not necessarily represent those of their affiliated organizations, or those of the publisher, the editors and the reviewers. Any product that may be evaluated in this article, or claim that may be made by its manufacturer, is not guaranteed or endorsed by the publisher.
Aagaard, K., Ma, J., Antony, K. M., Ganu, R., Petrosino, J., Versalovic, J. (2014). The placenta harbors a unique microbiome. Sci. Transl. Med. 6 (237), 237ra65. doi: 10.1126/scitranslmed.3008599
Agrawal, S., Shinar, S., Cerdeira, A. S., Redman, C., Vatish, M. (2019). Predictive performance of PlGF (Placental growth factor) for screening preeclampsia in asymptomatic women: A systematic review and meta-analysis. Hypertension 74 (5), 1124–1135. doi: 10.1161/HYPERTENSIONAHA.119.13360
Amarasekara, R., Jayasekara, R. W., Senanayake, H., Dissanayake, V. H. (2015). Microbiome of the placenta in pre-eclampsia supports the role of bacteria in the multifactorial cause of pre-eclampsia. J. Obstet Gynaecol Res. 41 (5), 662–669. doi: 10.1111/jog.12619
Ashkar, A. A., Croy, B. A. (1999). Interferon-gamma contributes to the normalcy of murine pregnancy. Biol. Reprod. 61 (2), 493–502. doi: 10.1095/biolreprod61.2.493
Baker, J. M., Chase, D. M., Herbst-Kralovetz, M. M. (2018). Uterine microbiota: Residents, tourists, or invaders? Front. Immunol. 9, 208. doi: 10.3389/fimmu.2018.00208
Bardos, J., Fiorentino, D., Longman, R. E., Paidas, M. (2019). Immunological role of the maternal uterine microbiome in pregnancy: Pregnancies pathologies and alterated microbiota. Front. Immunol. 10, 2823. doi: 10.3389/fimmu.2019.02823
Benner, M., Ferwerda, G., Joosten, I., van der Molen, R. G. (2018). How uterine microbiota might be responsible for a receptive, fertile endometrium. Hum. Reprod. Update 24 (4), 393–415. doi: 10.1093/humupd/dmy012
Brown, E. M., Kenny, D. J., Xavier, R. J. (2019). Gut microbiota regulation of T cells during inflammation and autoimmunity. Annu. Rev. Immunol. 37, 599–624. doi: 10.1146/annurev-immunol-042718-041841
Brunham, R. C., Gottlieb, S. L., Paavonen, J. (2015). Pelvic inflammatory disease. N Engl. J. Med. 372 (21), 2039–2048. doi: 10.1056/NEJMra1411426
Callahan, B. J., DiGiulio, D. B., Goltsman, D., Sun, C. L., Costello, E. K., Jeganathan, P., et al. (2017). Replication and refinement of a vaginal microbial signature of preterm birth in two racially distinct cohorts of US women. Proc. Natl. Acad. Sci. U.S.A. 114 (37), 9966–9971. doi: 10.1073/pnas.1705899114
Carlino, C., Stabile, H., Morrone, S., Bulla, R., Soriani, A., Agostinis, C., et al. (2008). Recruitment of circulating NK cells through decidual tissues: a possible mechanism controlling NK cell accumulation in the uterus during early pregnancy. Blood 111 (6), 3108–3115. doi: 10.1182/blood-2007-08-105965
Chaiworapongsa, T., Chaemsaithong, P., Yeo, L., Romero, R. (2014). Pre-eclampsia part 1: current understanding of its pathophysiology. Nat. Rev. Nephrol. 10 (8), 466–480. doi: 10.1038/nrneph.2014.102
Chan, D., Bennett, P. R., Lee, Y. S., Kundu, S., Teoh, T. G., Adan, M., et al. (2022). Microbial-driven preterm labour involves crosstalk between the innate and adaptive immune response. Nat. Commun. 13 (1), 975. doi: 10.1038/s41467-022-28620-1
Chen, H. J., Gur, T. L. (2019). Intrauterine microbiota: Missing, or the missing link? Trends Neurosci. 42 (6), 402–413. doi: 10.1016/j.tins.2019.03.008
Chen, X., Li, P., Liu, M., Zheng, H., He, Y., Chen, M. X., et al. (2020). Gut dysbiosis induces the development of pre-eclampsia through bacterial translocation. Gut 69 (3), 513–522. doi: 10.1136/gutjnl-2019-319101
Chen, C., Song, X., Wei, W., Zhong, H., Dai, J., Lan, Z., et al. (2017). The microbiota continuum along the female reproductive tract and its relation to uterine-related diseases. Nat. Commun. 8 (1), 875. doi: 10.1038/s41467-017-00901-0
Conde-Agudelo, A., Villar, J., Lindheimer, M. (2008). Maternal infection and risk of preeclampsia: systematic review and metaanalysis. Am. J. Obstet Gynecol 198 (1), 7–22. doi: 10.1016/j.ajog.2007.07.040
Costello, E. K., Lauber, C. L., Hamady, M., Fierer, N., Gordon, J. I., Knight, R. (2009). Bacterial community variation in human body habitats across space and time. Science 326 (5960), 1694–1697. doi: 10.1126/science.1177486
Crusell, M., Hansen, T. H., Nielsen, T., et al. (2018). Gestational diabetes is associated with change in the gut microbiota composition in third trimester of pregnancy and postpartum. Microbiome 6 (1), 89. doi: 10.1186/s40168-018-0472-x
de Goffau, M. C., Lager, S., Salter, S. J., et al. (2018). Recognizing the reagent microbiome. Nat. Microbiol. 3 (8), 851–853. doi: 10.1038/s41564-018-0202-y
DiGiulio, D. B., Callahan, B. J., McMurdie, P. J., Costello, E. K., Lyell, D. J., Robaczewska, A., et al. (2015). Temporal and spatial variation of the human microbiota during pregnancy. Proc. Natl. Acad. Sci. U.S.A. 112 (35), 11060–11065. doi: 10.1073/pnas.1502875112
Dörtbudak, O., Eberhardt, R., Ulm, M., Persson, G. R. (2005). Periodontitis, a marker of risk in pregnancy for preterm birth. J. Clin. Periodontol 32 (1), 45–52. doi: 10.1111/j.1600-051X.2004.00630.x
Dunn, A. B., Hanson, L., VandeVusse, L., Leslie, S. (2019). Through the microbial looking glass: Premature labor, preeclampsia, and gestational diabetes: A scoping review. J. Perinat Neonatal Nurs. 33 (1), 35–51. doi: 10.1097/JPN.0000000000000375
Elovitz, M. A., Gajer, P., Riis, V., Brown, A. G., Humphrys, M. S., Holm, J. B., et al. (2019). Cervicovaginal microbiota and local immune response modulate the risk of spontaneous preterm delivery. Nat. Commun. 10 (1), 1305. doi: 10.1038/s41467-019-09285-9
Fändriks, L. (2017). Roles of the gut in the metabolic syndrome: an overview. J. Intern. Med. 281 (4), 319–336. doi: 10.1111/joim.12584
Fang, R. L., Chen, L. X., Shu, W. S., Yao, S. Z., Wang, S. W., Chen, Y. Q. (2016). Barcoded sequencing reveals diverse intrauterine microbiomes in patients suffering with endometrial polyps. Am. J. Transl. Res. 8 (3), 1581–1592.
Fardini, Y., Chung, P., Dumm, R., Joshi, N., Han, Y. W. (2010). Transmission of diverse oral bacteria to murine placenta: evidence for the oral microbiome as a potential source of intrauterine infection. Infect. Immun. 78 (4), 1789–1796. doi: 10.1128/IAI.01395-09
Fettweis, J. M., Serrano, M. G., Brooks, J. P., Edwards, D. J., Girerd, P. H., Parikh, H. I., et al. (2019). The vaginal microbiome and preterm birth. Nat. Med. 25 (6), 1012–1021. doi: 10.1038/s41591-019-0450-2
Florova, V., Romero, R., Tarca, A. L., Galaz, J., Motomura, K., Ahmad, M. M., et al. (2021). Vaginal host immune-microbiome interactions in a cohort of primarily African-American women who ultimately underwent spontaneous preterm birth or delivered at term. Cytokine 137, 155316. doi: 10.1016/j.cyto.2020.155316
Franasiak, J. M., Werner, M. D., Juneau, C. R., Tao, X., Landis, J., Zhan, Y., et al. (2016). Endometrial microbiome at the time of embryo transfer: next-generation sequencing of the 16S ribosomal subunit. J. Assist. Reprod. Genet. 33 (1), 129–136. doi: 10.1007/s10815-015-0614-z
Gajer, P., Brotman, R. M., Bai, G., Sakamoto, J., Schütte, U. M., Zhong, X., et al. (2012). Temporal dynamics of the human vaginal microbiota. Sci. Transl. Med. 4 (132), 132ra52. doi: 10.1126/scitranslmed.3003605
Gamliel, M., Goldman-Wohl, D., Isaacson, B., Gur, C., Stein, N., Yamin, R., et al. (2018). Trained memory of human uterine NK cells enhances their function in subsequent pregnancies. Immunity 48 (5), 951–962.e5. doi: 10.1016/j.immuni.2018.03.030
Ghiringhelli, F., Ménard, C., Terme, M., Flament, C., Taieb, J., Chaput, N., et al. (2005). CD4+CD25+ regulatory T cells inhibit natural killer cell functions in a transforming growth factor-beta-dependent manner. J. Exp. Med. 202 (8), 1075–1085. doi: 10.1084/jem.20051511
Gill, S. R., Pop, M., Deboy, R. T., Eckburg, P. B., Turnbaugh, P. J., Samuel, B. S., et al. (2006). Metagenomic analysis of the human distal gut microbiome. Science 312 (5778), 1355–1359. doi: 10.1126/science.1124234
Gohir, W., Ratcliffe, E. M., Sloboda, D. M. (2015). Of the bugs that shape us: maternal obesity, the gut microbiome, and long-term disease risk. Pediatr. Res. 77 (1-2), 196–204. doi: 10.1038/pr.2014.169
Goldenberg, R. L., Culhane, J. F., Iams, J. D., Romero, R.. (2008). Epidemiology and causes of preterm birth. Lancet 371 (9606), 75–84. doi: 10.1016/S0140-6736(08)60074-4
Gudicha, D. W., Romero, R., Kabiri, D., Hernandez-Andrade, E., Pacora, P., Erez, O., et al. (2021). Personalized assessment of cervical length improves prediction of spontaneous preterm birth: a standard and a percentile calculator. Am. J. Obstet Gynecol 224 (3), 288.e1–288.e17. doi: 10.1016/j.ajog.2020.09.002
Hanna, J., Goldman-Wohl, D., Hamani, Y., Avraham, I., Greenfield, C., Natanson-Yaron, S., et al. (2006). Decidual NK cells regulate key developmental processes at the human fetal-maternal interface. Nat. Med. 12 (9), 1065–1074. doi: 10.1038/nm1452
Hansen, L. K., Becher, N., Bastholm, S., Glavind, J., Ramsing, M., Kim, C. J., et al. (2014). The cervical mucus plug inhibits, but does not block, the passage of ascending bacteria from the vagina during pregnancy. Acta Obstet Gynecol Scand. 93 (1), 102–108. doi: 10.1111/aogs.12296
Hickey, R. J., Zhou, X., Pierson, J. D., Ravel, J., Forney, L. J. (2012). Understanding vaginal microbiome complexity from an ecological perspective. Transl. Res. 160 (4), 267–282. doi: 10.1016/j.trsl.2012.02.008
Hofmann, A. P., Gerber, S. A., Croy, B. A. (2014). Uterine natural killer cells pace early development of mouse decidua basalis. Mol. Hum. Reprod. 20 (1), 66–76. doi: 10.1093/molehr/gat060
James, J. L., Whitley, G. S., Cartwright, J. E. (2010). Pre-eclampsia: fitting together the placental, immune and cardiovascular pieces. J. Pathol. 221 (4), 363–378. doi: 10.1002/path.2719
Jeyabalan, A. (2013). Epidemiology of preeclampsia: impact of obesity. Nutr. Rev. 71 Suppl 1 (0 1), S18–S25. doi: 10.1111/nure.12055
Jiménez, E., Marín, M. L., Martín, R., Odriozola, J. M., Olivares, M., Xaus, J., et al. (2008). Is meconium from healthy newborns actually sterile? Res. Microbiol. 159 (3), 187–193. doi: 10.1016/j.resmic.2007.12.007
Kaiko, G. E., Horvat, J. C., Beagley, K. W., Hansbro, P. M. (2008). Immunological decision-making: how does the immune system decide to mount a helper T-cell response? Immunology 123 (3), 326–338. doi: 10.1111/j.1365-2567.2007.02719.x
Kangatharan, C., Labram, S., Bhattacharya, S. (2017). Interpregnancy interval following miscarriage and adverse pregnancy outcomes: systematic review and meta-analysis. Hum. Reprod. Update 23 (2), 221–231. doi: 10.1093/humupd/dmw043
Kaur, H., Merchant, M., Haque, M. M., Mande, S. S. (2020). Crosstalk between female gonadal hormones and vaginal microbiota across various phases of women's gynecological lifecycle. Front. Microbiol. 11, 551. doi: 10.3389/fmicb.2020.00551
Kennedy, K. M., Gerlach, M. J., Adam, T., Heimesaat, M. M., Rossi, L., Surette, M. G., et al. (2021). Fetal meconium does not have a detectable microbiota before birth. Nat. Microbiol. 6 (7), 865–873. doi: 10.1038/s41564-021-00904-0
Kim, D., Hofstaedter, C. E., Zhao, C., Mattei, L., Tanes, C., Clarke, E., et al. (2017). Optimizing methods and dodging pitfalls in microbiome research. Microbiome 5 (1), 52. doi: 10.1186/s40168-017-0267-5
Klentzeris, L. D., Bulmer, J. N., Warren, M. A., Morrison, L., Li, T. C., Cooke, I. D. (1994). Lymphoid tissue in the endometrium of women with unexplained infertility: morphometric and immunohistochemical aspects. Hum. Reprod. 9 (4), 646–652. doi: 10.1093/oxfordjournals.humrep.a138564
Koedooder, R., Singer, M., Schoenmakers, S., Savelkoul, P., Morré, S. A., de Jonge, J. D., et al. (2019). The vaginal microbiome as a predictor for outcome of in vitro fertilization with or without intracytoplasmic sperm injection: a prospective study. Hum. Reprod. 34 (6), 1042–1054. doi: 10.1093/humrep/dez065
Kosti, I., Lyalina, S., Pollard, K. S., Sirota, M. (2020). Meta-analysis of vaginal microbiome data provides new insights into preterm birth. Front. Microbiol. 11, 476. doi: 10.3389/fmicb.2020.00476
Kuang, Y. S., Lu, J. H., Li, S. H., Li, J. H., Yuan, M. Y., He, J. R., et al. (2017). Connections between the human gut microbiome and gestational diabetes mellitus. Gigascience 6 (8), 1–12. doi: 10.1093/gigascience/gix058
Lauder, A. P., Roche, A. M., Sherrill-Mix, S., Bailey, A., Laughlin, A. L., Bittinger, K., et al. (2016). Comparison of placenta samples with contamination controls does not provide evidence for a distinct placenta microbiota. Microbiome 4 (1), 29. doi: 10.1186/s40168-016-0172-3
Lee, S. K., Kim, C. J., Kim, D. J., Kang, J. H. (2015). Immune cells in the female reproductive tract. Immune Netw. 15 (1), 16–26. doi: 10.4110/in.2015.15.1.16
Leiby, J. S., McCormick, K., Sherrill-Mix, S., Clarke, E. L., Kessler, L. R., Taylor, L. J., et al. (2018). Lack of detection of a human placenta microbiome in samples from preterm and term deliveries. Microbiome 6 (1), 196. doi: 10.1186/s40168-018-0575-4
Lenz, J. D., Dillard, J. P. (2018). Pathogenesis of neisseria gonorrhoeae and the host defense in ascending infections of human fallopian tube. Front. Immunol. 9, 2710. doi: 10.3389/fimmu.2018.02710
Levine, R. J., Maynard, S. E., Qian, C., Lim, K. H., England, L. J., Yu, K. F., et al. (2004). Circulating angiogenic factors and the risk of preeclampsia. N Engl. J. Med. 350 (7), 672–683. doi: 10.1056/NEJMoa031884
Liu, S., Diao, L., Huang, C., Li, Y., Zeng, Y., Kwak-Kim, J. (2017). The role of decidual immune cells on human pregnancy. J. Reprod. Immunol. 124, 44–53. doi: 10.1016/j.jri.2017.10.045
Lloyd-Price, J., Abu-Ali, G., Huttenhower, C. (2016). The healthy human microbiome. Genome Med. 8 (1), 51. doi: 10.1186/s13073-016-0307-y
Lozano, F. M., Bernabeu, A., Lledo, B., Morales, R., Diaz, M., Aranda, F. I., et al. (2021). Characterization of the vaginal and endometrial microbiome in patients with chronic endometritis. Eur. J. Obstet Gynecol Reprod. Biol. 263, 25–32. doi: 10.1016/j.ejogrb.2021.05.045
Maltepe, E., Bakardjiev, A. I., Fisher, S. J. (2010). The placenta: transcriptional, epigenetic, and physiological integration during development. J. Clin. Invest. 120 (4), 1016–1025. doi: 10.1172/JCI41211
Manaster, I., Mizrahi, S., Goldman-Wohl, D., Sela, H. Y., Stern-Ginossar, N., Lankry, D., et al. (2008). Endometrial NK cells are special immature cells that await pregnancy. J. Immunol. 181 (3), 1869–1876. doi: 10.4049/jimmunol.181.3.1869
Maynard, S. E., Min, J. Y., Merchan, J., Lim, K. H., Li, J., Mondal, S., et al. (2003). Excess placental soluble fms-like tyrosine kinase 1 (sFlt1) may contribute to endothelial dysfunction, hypertension, and proteinuria in preeclampsia. J. Clin. Invest. 111 (5), 649–658. doi: 10.1172/JCI17189
Miles, S. M., Hardy, B. L., Merrell, D. S. (2017). Investigation of the microbiota of the reproductive tract in women undergoing a total hysterectomy and bilateral salpingo-oopherectomy. Fertil Steril 107 (3), 813–820.e1. doi: 10.1016/j.fertnstert.2016.11.028
Moreno, I., Codoñer, F. M., Vilella, F., Valbuena, D., Martinez-Blanch, J. F., Jimenez-Almazán, J., et al. (2016). Evidence that the endometrial microbiota has an effect on implantation success or failure. Am. J. Obstet Gynecol 215 (6), 684–703. doi: 10.1016/j.ajog.2016.09.075
Nordqvist, M., Jacobsson, B., Brantsæter, A. L., Myhre, R., Nilsson, S., Sengpiel, V. (2018). Timing of probiotic milk consumption during pregnancy and effects on the incidence of preeclampsia and preterm delivery: a prospective observational cohort study in Norway. BMJ Open 8 (1), e018021. doi: 10.1136/bmjopen-2017-018021
Oberle, A., Urban, L., Falch-Leis, S., Ennemoser, C., Nagai, Y., Ashikawa, K., et al. (2021). 16S rRNA long-read nanopore sequencing is feasible and reliable for endometrial microbiome analysis. Reprod. BioMed. Online 42 (6), 1097–1107. doi: 10.1016/j.rbmo.2021.03.016
Onderdonk, A. B., Delaney, M. L., Fichorova, R. N. (2016). The human microbiome during bacterial vaginosis. Clin. Microbiol. Rev. 29 (2), 223–238. doi: 10.1128/CMR.00075-15
Payne, M. S., Bayatibojakhi, S. (2014). Exploring preterm birth as a polymicrobial disease: an overview of the uterine microbiome. Front. Immunol. 5, 595. doi: 10.3389/fimmu.2014.00595
Payne, M. S., Newnham, J. P., Doherty, D. A., Furfaro, L. L., Pendal, N. L., Loh, D. E., et al. (2021). A specific bacterial DNA signature in the vagina of Australian women in midpregnancy predicts high risk of spontaneous preterm birth (the Predict1000 study). Am. J. Obstet Gynecol 224 (2), 206.e1–206.e23. doi: 10.1016/j.ajog.2020.08.034
Pereira, N., Hutchinson, A. P., Lekovich, J. P., Hobeika, E., Elias, R. T. (2016). Antibiotic prophylaxis for gynecologic procedures prior to and during the utilization of assisted reproductive technologies: A systematic review. J. Pathog. 2016, 4698314. doi: 10.1155/2016/4698314
Perez, P. F., Doré, J., Leclerc, M., Levenez, F., Benyacoub, J., Serrant, P., et al. (2007). Bacterial imprinting of the neonatal immune system: lessons from maternal cells? Pediatrics 119 (3), e724–e732. doi: 10.1542/peds.2006-1649
Perez-Muñoz, M. E., Arrieta, M. C., Ramer-Tait, A. E., Walter, J. (2017). A critical assessment of the "sterile womb" and "in utero colonization" hypotheses: implications for research on the pioneer infant microbiome. Microbiome 5 (1), 48. doi: 10.1186/s40168-017-0268-4
Perin, J., Mulick, A., Yeung, D., Villavicencio, F., Lopez, G., Strong, K. L., et al. (2022). Global, regional, and national causes of under-5 mortality in 2000-19: an updated systematic analysis with implications for the sustainable development goals. Lancet Child Adolesc. Health 6 (2), 106–115. doi: 10.1016/S2352-4642(21)00311-4
Ravel, J., Gajer, P., Abdo, Z., Schneider, G. M., Koenig, S. S., McCulle, S. L., et al. (2011). Vaginal microbiome of reproductive-age women. Proc. Natl. Acad. Sci. U.S.A. 108 Suppl 1 (Suppl 1), 4680–4687. doi: 10.1073/pnas.1002611107
Rustveld, L. O., Kelsey, S. F., Sharma, R. (2008). Association between maternal infections and preeclampsia: a systematic review of epidemiologic studies. Matern Child Health J. 12 (2), 223–242. doi: 10.1007/s10995-007-0224-1
Schirmer, M., Franzosa, E. A., Lloyd-Price, J., McIver, L. J., Schwager, R., Poon, T. W., et al. (2018). Dynamics of metatranscription in the inflammatory bowel disease gut microbiome. Nat. Microbiol. 3 (3), 337–346. doi: 10.1038/s41564-017-0089-z
Sepich-Poore, G. D., Zitvogel, L., Straussman, R., Hasty, J., Wargo, J. A., Knight, R. (2021). The microbiome and human cancer. Science 371 (6536). doi: 10.1126/science.abc4552
Serrano, M. G., Parikh, H. I., Brooks, J. P., Edwards, D. J., Arodz, T. J., Edupuganti, L., et al. (2019). Racioethnic diversity in the dynamics of the vaginal microbiome during pregnancy. Nat. Med. 25 (6), 1001–1011. doi: 10.1038/s41591-019-0465-8
Shachar, B. Z., Mayo, J. A., Lyell, D. J., Baer, R. J., Jeliffe-Pawlowski, L. L., Stevenson, D. K., et al. (2016). Interpregnancy interval after live birth or pregnancy termination and estimated risk of preterm birth: a retrospective cohort study. BJOG 123 (12), 2009–2017. doi: 10.1111/1471-0528.14165
Shane, A. L., Sánchez, P. J., Stoll, B. J. (2017). Neonatal sepsis. Lancet 390 (10104), 1770–1780. doi: 10.1016/S0140-6736(17)31002-4
Shevach, E. M. (2002). CD4+ CD25+ suppressor T cells: more questions than answers. Nat. Rev. Immunol. 2 (6), 389–400. doi: 10.1038/nri821
Soma-Pillay, P., Nelson-Piercy, C., Tolppanen, H., Mebazaa, A. (2016). Physiological changes in pregnancy. Cardiovasc. J. Afr 27 (2), 89–94. doi: 10.5830/CVJA-2016-021
Souders, C. A., Maynard, S. E., Yan, J., Wang, Y., Boatright, N. K., Sedan, J., et al. (2015). Circulating levels of sFlt1 splice variants as predictive markers for the development of preeclampsia. Int. J. Mol. Sci. 16 (6), 12436–12453. doi: 10.3390/ijms160612436
Sparks, R. A., Purrier, B. G., Watt, P. J., Elstein, M. (1981). Bacteriological colonisation of uterine cavity: role of tailed intrauterine contraceptive device. Br. Med. J. (Clin Res. Ed) 282 (6271), 1189–1191. doi: 10.1136/bmj.282.6271.1189
Suff, N., Karda, R., Diaz, J. A., Ng, J., Baruteau, J., Perocheau, D., et al. (2018). Ascending vaginal infection using bioluminescent bacteria evokes intrauterine inflammation, preterm birth, and neonatal brain injury in pregnant mice. Am. J. Pathol. 188 (10), 2164–2176. doi: 10.1016/j.ajpath.2018.06.016
Szmuilowicz, E. D., Josefson, J. L., Metzger, B. E. (2019). Gestational diabetes mellitus. Endocrinol. Metab. Clin. North Am. 48 (3), 479–493. doi: 10.1016/j.ecl.2019.05.001
Taddei, C. R., Cortez, R. V., Mattar, R., Torloni, M. R., Daher, S. (2018). Microbiome in normal and pathological pregnancies: A literature overview. Am. J. Reprod. Immunol. 80 (2), e12993. doi: 10.1111/aji.12993
Theis, K. R., Romero, R., Winters, A. D., Greenberg, J. M., Gomez-Lopez, N., Alhousseini, A., et al. (2019). Does the human placenta delivered at term have a microbiota? results of cultivation, quantitative real-time PCR, 16S rRNA gene sequencing, and metagenomics. Am. J. Obstet Gynecol 220 (3), 267.e1–267.e39. doi: 10.1016/j.ajog.2018.10.018
Umapathy, A., Chamley, L. W., James, J. L. (2020). Reconciling the distinct roles of angiogenic/anti-angiogenic factors in the placenta and maternal circulation of normal and pathological pregnancies. Angiogenesis 23 (2), 105–117. doi: 10.1007/s10456-019-09694-w
Vacca, P., Vitale, C., Montaldo, E., Conte, R., Cantoni, C., Fulcheri, E., et al. (2011). CD34+ hematopoietic precursors are present in human decidua and differentiate into natural killer cells upon interaction with stromal cells. Proc. Natl. Acad. Sci. U.S.A. 108 (6), 2402–2407. doi: 10.1073/pnas.1016257108
Verlohren, S., Herraiz, I., Lapaire, O., Schlembach, D., Moertl, M., Zeisler, H., et al. (2012). The sFlt-1/PlGF ratio in different types of hypertensive pregnancy disorders and its prognostic potential in preeclamptic patients. Am. J. Obstet Gynecol 206 (1), 58.e1–58.e8. doi: 10.1016/j.ajog.2011.07.037
Veronika, M., František, G., Búda. (2018). A Possible Role of Human Herpes Viruses Belonging to the Subfamily Alphaherpesvirinae in the Development of Some Cancers. Možná úloha ĺudských herpetických vírusov podčeĺade Alphaherpesvirinae pri vzniku niektorých nádorových ochorení. Klin Onkol. 31 (3), 178–183.
Verstraelen, H., Vilchez-Vargas, R., Desimpel, F., Jauregui, R., Vankeirsbilck, N., Weyers, S., et al. (2016). Characterisation of the human uterine microbiome in non-pregnant women through deep sequencing of the V1-2 region of the 16S rRNA gene. PeerJ 4, e1602. doi: 10.7717/peerj.1602
Wang, J., Li, Z., Ma, X., Du, L., Jia, Z., Cui, X., et al. (2021). Translocation of vaginal microbiota is involved in impairment and protection of uterine health. Nat. Commun. 12 (1), 4191. doi: 10.1038/s41467-021-24516-8
Wang, J., Zheng, J., Shi, W., Du, N., Xu, X., Zhang, Y., et al. (2018). Dysbiosis of maternal and neonatal microbiota associated with gestational diabetes mellitus. Gut 67 (9), 1614–1625. doi: 10.1136/gutjnl-2018-315988
Wei, S. Q., Fraser, W., Luo, Z. C. (2010). Inflammatory cytokines and spontaneous preterm birth in asymptomatic women: a systematic review. Obstet Gynecol 116 (2 Pt 1), 393–401. doi: 10.1097/AOG.0b013e3181e6dbc0
Wessels, J. M., Domínguez, M. A., Leyland, N. A., Agarwal, S. K., Foster, W. G. (2021). Endometrial microbiota is more diverse in people with endometriosis than symptomatic controls. Sci. Rep. 11 (1), 18877. doi: 10.1038/s41598-021-98380-3
Widmer, M., Cuesta, C., Khan, K. S., Conde-Agudelo, A., Carroli, G., Fusey, S., et al. (2015). Accuracy of angiogenic biomarkers at ≦̸20weeks' gestation in predicting the risk of pre-eclampsia: A WHO multicentre study. Pregnancy Hypertens. 5 (4), 330–338. doi: 10.1016/j.preghy.2015.09.004
Winters, A. D., Romero, R., Gervasi, M. T., Gomez-Lopez, N., Tran, M. R., Garcia-Flores, V., et al. (2019). Does the endometrial cavity have a molecular microbial signature? Sci. Rep. 9 (1), 9905. doi: 10.1038/s41598-019-46173-0
Witkin, S. S., Moron, A. F., Ridenhour, B. J., Minis, E., Hatanaka, A., Sarmento, S., et al. (2019). Vaginal biomarkers that predict cervical length and dominant bacteria in the vaginal microbiomes of pregnant women. mBio 10 (5). doi: 10.1128/mBio.02242-19
Yang, S., Reid, G., Challis, J. R., Kim, S. O., Gloor, G. B., Bocking, A. D. (2015). Is there a role for probiotics in the prevention of preterm birth? Front. Immunol. 6, 62. doi: 10.3389/fimmu.2015.00062
Yang, F., Zheng, Q., Jin, L. (2019). Dynamic function and composition changes of immune cells during normal and pathological pregnancy at the maternal-fetal interface. Front. Immunol. 10, 2317. doi: 10.3389/fimmu.2019.02317
Younes, J. A., Lievens, E., Hummelen, R., van der Westen, R., Reid, G., Petrova, M. I. (2018). Women and their microbes: The unexpected friendship. Trends Microbiol. 26 (1), 16–32. doi: 10.1016/j.tim.2017.07.008
Keywords: pregnancy immunity, vaginal microbiota, vaginal dysbiosis, uterine microflora, uterine dysbiosis
Citation: Shen L, Wang W, Hou W, Jiang C, Yuan Y, Hu L and Shang A (2023) The function and mechanism of action of uterine microecology in pregnancy immunity and its complications. Front. Cell. Infect. Microbiol. 12:1025714. doi: 10.3389/fcimb.2022.1025714
Received: 23 August 2022; Accepted: 22 November 2022;
Published: 04 January 2023.
Edited by:
Rosa Sessa, Sapienza University of Rome, Rome, ItalyReviewed by:
Tingtao Chen, Nanchang University, ChinaCopyright © 2023 Shen, Wang, Hou, Jiang, Yuan, Hu and Shang. This is an open-access article distributed under the terms of the Creative Commons Attribution License (CC BY). The use, distribution or reproduction in other forums is permitted, provided the original author(s) and the copyright owner(s) are credited and that the original publication in this journal is cited, in accordance with accepted academic practice. No use, distribution or reproduction is permitted which does not comply with these terms.
*Correspondence: Anquan Shang, c2hhbmdhbnF1YW5AdG9uZ2ppLmVkdS5jbg==; Liqing Hu, MTM2MDU4ODc4NThAMTYzLmNvbQ==
†These authors have contributed equally to this work
Disclaimer: All claims expressed in this article are solely those of the authors and do not necessarily represent those of their affiliated organizations, or those of the publisher, the editors and the reviewers. Any product that may be evaluated in this article or claim that may be made by its manufacturer is not guaranteed or endorsed by the publisher.
Research integrity at Frontiers
Learn more about the work of our research integrity team to safeguard the quality of each article we publish.