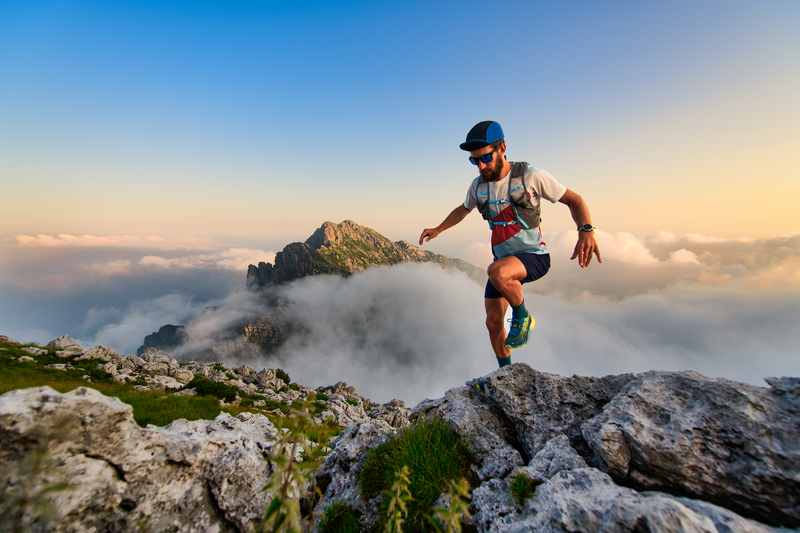
95% of researchers rate our articles as excellent or good
Learn more about the work of our research integrity team to safeguard the quality of each article we publish.
Find out more
ORIGINAL RESEARCH article
Front. Cell. Infect. Microbiol. , 21 September 2022
Sec. Biofilms
Volume 12 - 2022 | https://doi.org/10.3389/fcimb.2022.1006723
This article is part of the Research Topic Emerging Strategies in Combatting and Managing Bacterial Biofilms View all 10 articles
Some Brevundimonas spp. are globally emerging opportunistic pathogens that can be dangerous to individuals with underlying medical conditions and for those who are immunocompromised. Gram-negative Brevundimonas spp. can form resilient sessile biofilms and are found not only in different confined terrestrial settings (e.g., hospitals) but are also frequently detected in spacecraft which is inhabited by astronauts that can have altered immunity. Therefore, Brevundimonas spp. pose a serious health hazard in different environments, especially in its biofilm form. Conventional antimicrobials applied to disrupt, inactivate, or prevent biofilm formation have limited efficiency and applicability in different closed-loop systems. Therefore, new, effective, and safe biofilm control technologies are in high demand. The present work aimed to investigate antimicrobial photoinactivation (API) of Brevundimonas sp. ESA1 monocultural biofilms mediated by non-toxic, natural photosensitizers such as riboflavin (RF) and chlorophyllin (Chl) with an emphasis of this technology as an example to be safely used in closed-loop systems such as spacecraft. The present study showed that Chl-based API had a bactericidal effect on Brevundimonas sp. ESA1 biofilms at twice the lower irradiation doses than was needed when applying RF-based API. Long-term API based on RF and Chl using 450 nm low irradiance plate has also been studied in this work as a more practically applicable API method. The ability of Brevundimonas sp. ESA1 biofilms to reduce alamarBlue™ and regrowth analysis have revealed that after the applied photoinactivation, bacteria can enter a viable but non-culturable state with no ability to resuscitate in some cases.
Naturally most of the bacteria are found living in a multicellular coordinated functional communities known as biofilms. Bacterial biofilms consist of bacterial cells encased in a self-produced extracellular polymeric substance (EPS) made of polysaccharides, proteins, and extracellular DNA (eDNA) (Flemming et al., 2016; Penesyan et al., 2021). EPS is the underlying physical factor determining the ability of bacterial cells to be more resistant to adverse external impacts than free-living cells (Hall-Stoodley et al., 2004; Reichhardt et al., 2014). Bacterial biofilms are common in different industrial settings, food facilities, water systems, bathrooms, laboratories, hospitals, and even spacecraft (Buchovec et al., 2020). Once established, biofilms become less susceptible to common antimicrobials such as antibiotics, chemical disinfectants, physical stress, and the human immune system (Mora et al., 2019; Muhammad et al., 2020; Ciofu et al., 2022). Therefore, biofilms are of special concern not only in confined areas such as hospitals, industrial food-associated premises on Earth, but also closed-loop spacecraft systems. Human-manned spacecraft is a unique environment encountering dangerous microbial contamination even though the spacecraft is assembled in a cleanroom (Checinska et al., 2015; Mora et al., 2016; Bashir et al., 2016; Nakajima et al., 2017). Spacecraft conditions such as space radiation, microgravity, and elevated carbon dioxide levels adversely affect health and the immune system of the astronauts. Therefore, microbial contamination, especially in the form of biofilms, can be very dangerous for the immunocompromised spacecraft crew members and the overall material integrity (Decelle and Taylor, 1976; Sobisch et al., 2019). The most abundant airborne and surface bacteria in spacecraft belong to Staphylococcus spp., Bacillus spp., Enterococcus spp., Corynebacterium spp. and Propionibacterium spp. (Borisov et al., 2003; La Duc et al., 2004a; Novikova et al., 2006; Venkateswaran et al., 2014; Checinska et al., 2015; Koskinen et al., 2017; Regberg et al., 2020). Methylobacterium spp., Sphingomonas paucimobilis, Cupriavidus spp., Chryseobacterium spp., and Ralstonia spp. are most frequently found in potable water systems (La Duc et al., 2004b; Wong et al., 2010; Mijnendonckx et al., 2013; Nakajima et al., 2017; Thompson et al., 2020). Most spacecraft bacteria are human-associated and play the main role in the formation and the diversity of spacecraft microbiota (Mora et al., 2016; Be et al., 2017; Zea et al., 2018).
However, according to recent findings, one of the emerging bacterial genera that is not so abundant but is also frequently detected in different spacecraft samples is Brevundimonas spp. (Kawamura et al., 2001; La Duc et al., 2004b; Li et al., 2004; Ghosh et al., 2010; Stieglmeier et al., 2012; Vornhagen et al., 2013) Brevundimonas spp. are non-fermenting Gram-negative bacteria that can form sessile biofilms, with some of the species being a cause of serious infections in individuals with underlying medical conditions (Vornhagen et al., 2013). The genus was established by Segers et al. (1994) when authors presented the re-classification of Pseudomonas diminuta and Pseudomonas vesicularis to Brevundimonas diminuta and Brevundimonas vesicularis, respectively (Segers et al., 1994). B. diminuta and B. vesicularis are concerned to be emerging global opportunistic pathogens due to recent findings of multiple infections caused by these species indicating that the genus may be a more widespread pathogen than it was hitherto thought. And apparently, infections caused by Brevundimonas spp. can be invasive and dangerous for people having chronic diseases or those who are immunocompromised (Han and Andrade, 2005; Ryan and Pembroke, 2018). Therefore, it is a matter of concern that the species of the genus are being constantly detected not only in different terrestrial facilities but also in spacecraft. In a varying abundance, Brevundimonas spp. was recovered from ISS-associated potable water samples at various stages of their purification, storage, and transport. Detection of Brevundimonas spp. in the water system of spacecraft means that it can survive in spacecraft essential systems, forming more resistant biofilm forms (La Duc et al., 2004b; Vornhagen et al., 2013). A study of the abundance and diversity of microbial bioburden in European spacecraft-associated clean rooms by molecular analysis revealed that Brevundimonas was among the most common (Stieglmeier et al., 2012).
Recently, Brevundimonas sp., among some other species, was determined to form biofilms that developed higher concentration of antibiotic resistant bacteria (ARB) under the disinfection pressure of chlorination and chloramination. This study indicated that biofilm detachment might become a cause of the movement of biofilm clusters with higher ARB concentration into water, thereby increasing the antibiotic resistance of bacteria in tap water (Zhang et al., 2019a). In a study of Low et al. (2016), as a genus of Proteobacteria, Brevundimonas was found to be resistant to eight antibiotics and was reported to contain tetracycline resistance genes (Miranda et al., 2003; Adelowo and Fagade, 2009). Brevundimonas spp. can exhibit resistance to heavy metals as well (Zhang et al., 2019b).
The most interesting finding about Brevundimonas is that it is found to be one of a few bacteria exhibiting high survival rates under simulated Martian conditions. Dartnell et al. (2010) have studied the survival responses of some novel psychrotolerant bacterial strains (isolated from the Antarctic Dry Valleys) to ionizing radiation while frozen at -79 °C, the temperature that is typical to Martian near-subsurface environment. Interestingly, one of the novel isolates of the Antarctic Dry Valleys was identified as Brevundimonas sp. MV.7 and was determined to be the most resistant to radiation. Experimental irradiation combined with previous radiation modelling indicated that Brevundimonas sp. MV.7 in 30 cm deep Martian dust could survive the space radiation for up to 100,000 years before having a 106 population reduction (Dartnell et al., 2010).
Therefore, some of the Brevundimonas species, due to its multiple resilience should pose serious concern in some terrestrial and especially spacecraft environments. Since conventional antimicrobials applied to disrupt, inactivate, or prevent biofilm formation have limited efficiency and applicability in closed-loop systems like spacecraft, new, effective, environmentally friendly, and safe biofilm control technologies are in a high demand.
One of the potential alternatives that provide many significant advantages is antimicrobial photoinactivation (API) (also known as antimicrobial photodynamic therapy - aPDT) - a technology based on interaction between non-toxic photosensitizer (PS), molecular oxygen, and appropriate doses of visible light of a certain wavelength that excites the PS (St. Denis et al., 2011; Hamblin, 2016). Usually, after light excitation, the triplet-state of PS interacts with molecular oxygen, electron donors or acceptors and can produce reactive oxygen species (ROS), thereby triggering photo-oxidative reactions that initiate various cellular damages and destruction of microorganisms (Hu et al., 2018). Different investigators have confirmed that microorganisms, including bacteria, viruses, molds, and protozoa, whether in vitro or in vivo, can be killed by API treatment (Wainwright, 2004; Jori and Brown, 2004; Luksiene, 2005; Buchovec et al., 2016; Temba et al., 2016). One of the major advantages of API is that the resistance of bacteria to API is unlikely to occur and it can be safely used in closed-loop systems (Liu et al., 2015; Maisch, 2015; Kashef and Hamblin, 2017). For the inactivation of both planktonic and sessile biofilm forms of bacteria in spacecraft and other sensitive confined systems - a non-toxic, chemically pure and stable, non-bleaching, easy-to-produce, and water-soluble PSs should be used. Most of the natural PSs meet the above-listed criteria and are one of the safest options of PSs for spacecraft use that have been proposed to date. Currently, four main natural products have been applied for API: curcumin, riboflavin, perylenequinones (hypericin, hypocrellin), psoralens (Yin et al., 2014). Recently, chlorophyll derivatives, such as sodium chlorophyllin were shown to be effective as natural PSs as well (Luksiene et al., 2010; Buchovec et al., 2010; Buchovec et al., 2017; Krüger et al., 2019; Luksiene and Buchovec, 2019; Buchovec et al., 2022). This study focuses on natural promising PSs - riboflavin (RF) or vitamin B2 and chlorophyllin (Chl) that could be potentially used in closed-loop systems as non-toxic and harmless to humans and plants. Both PSs – RF and Chl - are known photoactive compounds used as food colorants having “Generally Recognized As Safe” (GRAS) status. Also, it is essential to note that both PSs, RF and Chl, are photoactive in the visible-light range and have absorption maximums at 440 and 402 nm, respectively (Buchovec et al., 2020). RF is a naturally occurring water-soluble compound and an essential human nutrient and is used as a food colorant E 101. It plays an important role in the metabolism of the cells and can be considered safe when administered to humans (Astanov et al., 2014; Sheraz et al., 2014). Chl is also water-soluble food colorant - sodium magnesium chlorophyllin (E 140 (ii)), which is extracted from different plants such as spinach, grass, dandelion, green cabbage, water hyacinth, and algae. Chl is a semi-synthetic porphyrin obtained from chlorophyll which generates ROS with antimicrobial activity after exposure to visible light (Buchovec et al., 2017). Nevertheless, studies and applications of the RF-based (RF-API) and Chl-based (Chl-API) API to control Gram-negative bacteria pathogens, especially their biofilms, remain scarce. Furthermore, no API, neither with natural nor with other PSs has been performed on Brevundimonas spp. that are a matter of concern in both terrestrial and spacecraft settings. The present study aims to investigate whether RF-based and Chl-based API can efficiently inactivate biofilms of Brevundimonas sp. ESA1.
Non-copperized chlorophyllin sodium salt (Chl) (MW = 684.9 g/mol) was obtained from Carl Roth (Germany). The aqueous stock solution of 0.15 mM Chl (pH = 6.8) was prepared by dissolving Chl in distilled water. Riboflavin (RF) (MW = 376.36 g/mol)) was obtained from Sigma-Aldrich (USA). Aqueous stock solution of 0.11 mM RF (pH 6.2) was prepared according to the method of Mazzotta et al. (2014): RF was dissolved in distilled water using a magnetic stirrer at 25°С in the dark. Both PSs were filter-sterilized before use for the photoinactivation experiments. All working solutions were freshly prepared by diluting them with 0.01 M PBS buffer (pH 7.4) (Carl Roth, Germany) on the day of use.
The LED-based light source (an irradiation box) (Figure 1A) for RF-API and Chl-API was developed at the Institute of Photonics and Nanotechnology of Vilnius University (VU). Two types of LEDs (402 nm and 440 nm) with emission peaks near the maximum absorption of RF and Chl (Buchovec et al., 2022) were used (Figure 1B).
Figure 1 3D render picture (A) of the developed illumination system (the irradiation box) and normalized emission spectra of the 402 nm (Kingbright KTDS-3534UV405B), 440 nm (Osram GD QSSPA1.14) LEDs installed into the illumination system (B).
The light irradiance at the surface of the samples reached 5, 20 and 25 mW/cm2. The irradiation dose was calculated as irradiance multiplied by irradiation time. The sample exposure time was adjusted according to the equation:
where E is the energy density (dose) in J/cm2, P is the irradiance (light flux density) in mW/cm2, and t is the time in seconds. The exemplary irradiation dose calculations are shown in Table 1.
The light source used for long-term irradiation of biofilms was developed and constructed as a Constant Irradiation Plate (CIP; developed in the Institute of Photonics and Nanotechnology, VU) with 1.4 mW/cm2 uniform irradiance by LEDs peaked at 450 nm (Figure 2). The CIP is a multicolor micro-controller controlled LED source for upward irradiation. The device has an internal temperature feed-back circuit to maintain irradiation stability under long-term experiments. CIP was calibrated before the experiments with a spectro-radiometer (Avantes AvaSpec-ULS2048LTEC with AvaSphere-50-LS-HAL-CAL).
Aqueous solutions of 0.011 mM RF (pH 7.4) and 0.015 mM Chl (pH 7.4) were illuminated with a LED-based light source (irradiation box) for analysis of the photostability of the PSs. The changes in the absorption spectra of RF and Chl were investigated after LED illumination at 440 nm and 402 nm, respectively. For this purpose, 200 µL of the PSs were transferred to sterile flat-bottom 96-well microtiter plates (MtP) and exposed to 5 mW/cm2 irradiance. After each irradiation dose applied separately, the samples (3 mL) were collected into cuvettes and used for spectrophotometric measurements.
The absorption spectra of both PSs solutions were recorded by means of a LAMBDA 950 UV-VIS-NIR spectrophotometer (PerkinElmer, USA) in spectral range of 300-600 nm. Polymethyl methacrylate cuvettes of 1 cm optical thickness were used for the measurements. All measurements were performed at 20 ± 2°C.
Bacterial strain that was used as a model organism for RF-API and Chl-API studies was obtained from the collection of microorganisms of the Department of Microbiology and Biotechnology, Institute of Biosciences, Life Sciences Center, VU. For the confirmation of the genus of the strain, DNA sequence of the bacteria coding for 16S rRNA was amplified as described previously (Gricajeva et al., 2016) using 27F (5′-GAG AGT TTG ATC CTG GCT CAG-3′) and 1495R (5′-CTA CGG CTA CCT TGT TAC GA-3′) universal primers (METABION, Germany). Purified amplicon was sequenced at VU Life Sciences Center, Institute of Biotechnology (Lithuania). NCBI Basic Local Alignment Search Tool (BLASTn) (Altschul et al., 1990) was used for database similarity searches.
Bacteria were grown in Luria Bertani (LB) (Carl Roth, Germany) broth at 37°C under constant 180 rpm shaking (IKA, Germany) overnight. The overnight culture was inoculated to a fresh LB media and grown under the same conditions until optical density (OD) of 0.22 at 600 nm (OD600) corresponding to the concentration of 109 CFU/mL was reached. Bacterial cells were harvested by centrifugation (5 min, 5000 × g), resuspended in 0.01 M PBS (pH 7.4) and immediately used for the RF-API and Chl-API experiments.
For the assay of antimicrobial inactivation of free-floating (planktonic) Brevundimonas sp. ESA1, bacterial cells of the strain were resuspended to a final concentration of 107 CFU/mL in 0.011 mM RF (pH 7.4) or 0.015 mM Chl (pH 7.4) in the dark. Solution consisting of bacterial suspension in 0.01 M PBS (pH 7.4) was used as a control. Aliquots of 200 μL of prepared mixtures of bacterial suspensions with appropriate PSs (RF or Chl, pH 7.4) or 0.01 M PBS (pH 7.4) were pipetted into sterile flat-bottom 96-well polystyrene MtP and exposed to different irradiation doses (Table 1). The light source (Figure 1A) used for the photoinactivation experiments consisted of a LED array (λ = 440 nm for RF and λ = 405 nm for Chl) with an intensity of 5 mW/cm2 at a distance of approximately 7 cm. Following irradiation, at each sampling step, withdrawn bacterial suspensions were appropriately diluted, spread on LB agar plates and incubated at 37°C for 16-36 h. Residual bacterial cell viability was determined by counting CFU. The numbers of surviving bacteria (CFU/mL) were transformed to log10 scale. CFU counts of the bacterial cells that were incubated with RF, Chl or PBS in the dark (dark controls) were also determined.
For the monocultural biofilm formation bacterial strain was grown as it was described in Section 2.5 until OD600 corresponding to the concentration of 108 CFU/mL. Then 100 µL of the suspension was pipetted into sterile flat-bottom 96-well polystyrene MtP wells. MtPs with the required number of wells filled with bacterial suspension were statically incubated 20 h at 37°C. Biofilms that formed in the wells of MtP were washed three times with 0.01 M PBS (pH 7.4) in order to remove residual planktonic cells. Biofilms were further used for the API (Section 2.8). Formation of Brevundimonas sp. ESA1 biofilms in the MtP wells was verified by staining with 0.1% solution of crystal violet (Merritt et al., 2005; Trotonda et al., 2008).
Brevundimonas sp. ESA1 biofilms were formed in MtPs as it was described in Section 2.7. For the API, the wells of the 96-well MtPs containing the biofilm were filled with 0.011 mM RF (pH 7.4), 0.015 mM Chl (pH 7.4) or 0.01 M PBS (pH 7.4) by adding 200 µL of the appropriate solution. Then biofilms were immediately placed into irradiation boxes and irradiated with 440 nm at 25 mW/cm2 or 402 nm at 20 mW/cm2 for the photoactivation of RF and Chl, respectively. The control samples were also illuminated with the same wavelength of light. Biofilms were exposed to different illumination doses (Table 1). At each step of sampling, bacterial biofilms were mechanically detached from the MtP well walls, vigorously vortexed and diluted for the further viability determination by counting CFU on LB agar plates which after the spreading of samples were incubated at 37°C for 16-36 h. The numbers of surviving bacteria (CFU/mL) were transformed to log10 scale. CFU counts of Brevundimonas sp. ESA1 biofilm-forming cells that were incubated with RF/Chl or without RF/Chl in the dark were also determined.
Following photoinactivation, additionally to residual CFU count determination after RF-API and Chl-API, viability, and metabolic function of Brevundimonas sp. ESA1 biofilms was quantitatively analyzed by evaluating their ability to reduce resazurin-based compound alamarBlue™ (Invitrogen, USA). Non-toxic reagent alamarBlue™ is used as an indicator of cellular reducing environment or cell viability and death. The reagent is modified in reducing conditions that are characteristic to viable cells and becomes detectable due to its subsequently occurring color change or/and high fluorescence. Dead or non-viable cells are not able to change the color of alamarBlue™ (Rampersad, 2012).
In the current experiment Brevundimonas sp. ESA1 biofilms after RF-API and Chl-API that caused ≥3 log10 reduction (and corresponding dark controls) were mechanically detached, vortexed and added to alamarBlue™. The volume ratio of alamarBlue™ and disrupted biofilms was 1:10. Samples were incubated 3 h at 37°C (according to the manufacturer’s recommendations) and then fluorescence changes were measured every hour (in total for 11 h) using a plate reader (Thermo Fisher Scientific Verioscan Flash, USA). Fluorescence changes were read using excitation at 560 nm and emission at 590 nm.
Percentage reduction of alamarBlue™ by fluorescence indicating cell viability or death of all RF-API and Chl-API tested groups was determined by using the equation: % reduction of alamarBlue™ =
where FI 590 is fluorescence intensity at 590 nm emission (excitation at 560 nm)
Long-term RF-based and Chl-based API experiments were performed to test the photoinactivation efficacy of Brevundimonas sp. ESA1 biofilm by using CIP that emits 450 nm blue light. For the long-term RF-API and Chl-API experiments, Brevundimonas sp. ESA1 biofilms were formed as described previously (Section 2.7). Wells with biofilms filled with 200 µL of 0.015 mM Chl, 0.011 mM RF and 0.01 M PBS solutions were illuminated with 450 nm light on the CIP (Figure 2A) at 1.4 mW/cm2 irradiance for 28 h (to achieve irradiation dose of 141.1 J/cm2) for RF-API and 20 h (to achieve irradiation dose of 100.8 J/cm2) for Chl-API. Irradiation doses using CIP were chosen to correspond to those that caused ≥3 log10 reduction of CFU of biofilms using irradiation boxes. The irradiation dose of long-term Chl-API was higher than using the irradiation box due to suboptimal Chl photoactivation wavelength emitted by CIP. Results of long-term irradiation were evaluated by determining CFU counts on LB plates and the ability of the photoinactivated biofilm cells to reduce alamarBlue™, as described previously (Sections 2.8 and 2.9).
For the evaluation of Brevundimonas sp. ESA1 biofilm-forming cell’s capability to resuscitate and re-grow after API, mechanically detached and vortexed biofilms were inoculated into liquid LB media and incubated for 72 h at 37°C under constant 180 rpm shaking. During the incubation, OD600 of the bacteria was measured once in 24 h. Re-growth capability of the dark controls (bacterial biofilms incubated with and without RF/Chl in the dark) of Brevundimonas sp. ESA1 were evaluated in the same way as for the irradiated bacteria.
The effect of RF-API and Chl-API on the morphology of Brevundimonas sp. ESA1 biofilm were investigated by scanning electron microscopy (SEM) (CamScan Apollo 300, Cambridge, UK). For the SEM analysis biofilms were grown in MtPs as it is described in Section 2.7. After the RF-API and Chl-API treatment (as described in Section 2.8) at 25 mW/cm2 for 95 min and 20 mW/cm2 for 60 min (irradiation conditions under which 3 log10 reduction was achieved), respectively, irradiated biofilms and dark controls were mechanically detached by scraping them of the MtP well walls by pipette tip, then 10 µl of each sample was transferred on a SEM specimen stub covered with copper foil tape, air-dried at room temperature and coated with 50 nm gold layer using Q150T ES sputter coater (Quorum Technologies, Lewes, England). The scanning was performed using an electron beam with an accelerating voltage of 20 kV.
All experiments were performed at least three times (independently) and the results were reported providing mean ± SD. Students t-test and one-way ANOVA statistical tests were applied. Statistically significant differences among groups were considered when p ≤ 0.05. Graph construction was performed with Origin Pro 8.1 (OriginLab Corporation, USA) and GraphPad Prism V. 6 (GraphPad Software, USA) software. Statistical analysis was performed with GraphPad Prism V. 6 software.
Before the API of Brevundimonas sp. ESA1, the photostability of the aqueous solutions of 0.011 mM RF (pH 6.4) and 0.015 mM Chl (pH 6.8) was tested. It is known that RF absorbance spectra have four maximums: 223, 267, 373, 444 nm (Astanov et al., 2014). Therefore, the LEDs of 440 nm (irradiation box, Section 2.2) were used in experiments for the optimal excitation of RF. Chl is widely known as a water-soluble photoactive compound with the main absorption maximum at about 405 nm (Buchovec et al., 2017). Therefore, 402 nm LEDs of the irradiation box were used in experiments for the optimal excitation of Chl.
Both PSs are known to exhibit optical absorbance reduction after the activation by light. These changes show the activation dependence on excitation dose and can be used to compare the irradiation efficiency by different spectral components. We primarily studied the absorption characteristics of RF and Chl after illumination with an optimal excitation wavelength of 440 nm and 402 nm, respectively (Figure 3).
Figure 3 Photostability as optical absorbance spectra of 0.011 mM RF (A) and 0.015 mM Chl (B) after treatment of different irradiation doses (5 mW/cm2 irradiance).
The aqueous solutions of RF are sensitive to light and degraded to various photoproducts: formylmethylflavin, lumichrome, lumiflavin, carboxymethylflavin, 2,3-butanedione, a β-keto acid and a diketo compound. The type of the photoproduct depends on the solvent, pH, buffer type, concentration, oxygen content, light intensity, and wavelengths used (Sheraz et al., 2014). Figure 3A illustrates the spectra of 0.011 mM RF (pH 6.4) after blue light irradiation at 5 mW/cm2 for several durations (0 - 30 min, corresponding to 0-9 J/cm2 irradiance doses). As shown in Figure 3A, the absorbance of 0.011 mM RF at 373 and 444 nm was dramatically decreased after 440 nm irradiation (9 J/cm2). The photodegradation experiments helped assess the level of RF stability and revealed its photodegradation products after certain illumination exposures. Studies have shown that 0.011 mM RF (pH 6.4) photodegraded and probable formation of lumichrome photoproduct is observed at 9 J/cm2 (440 nm) exposure, according to the previous studies (Buchovec et al., 2022).
Figure 3B shows the spectra recorded after illumination of 0.015 mM Chl solution (pH 6.8) in distilled water at 5 mW/cm2 for different time periods (0-30 min, which corresponds to 0-9 J/cm2 irradiance doses). The illumination at 402 nm significantly diminished the peak magnitude after 30 min (9 J/cm2).
Although PSs were photobleached after 30 min of irradiation, antimicrobial effect can still be effectively implemented. In the case of RF, photoproducts, which can serve as PSs, are produced. Additionally, 440 nm light itself can have antibacterial effect as well. In the case of Chl, after its photoexcitation ROS that can cause significant damage are produced, and further, when Chl photodegrades, the ROS produced by 402 nm itself can have an antibacterial effect (Gwynne and Gallagher, 2018; Buchovec et al., 2022; Hadi et al., 2020).
The main objective of the study was to determine the bactericidal effect of RF-based and Chl-based API on Brevundimonas sp. ESA1 monocultural biofilm, although planktonic cells were also studied. RF and Chl were used to inactivate planktonic cells and biofilm in combination with 440 nm and 402 nm lights, respectively. Moreover, Brevundimonas sp. ESA1 biofilms were subjected to RF-API and Chl-API using a lower irradiance plate emitting 450 nm light. Partial sequence of 16S rRNA gene of the microorganisms used as a model in this study was deposited in GeneBank under the accession number ON237360.
Bactericidal effect of API was defined as ≥3 log10 (99.9%) reduction in count of CFU according to the National Committee for Clinical Laboratory (NCCLS), M26-A standard (Thornsberry, 1983; CLSI, 1999). The minimal required dose of irradiation for the achievement of reduction of ≥3 log10 in CFU counts of planktonic cells after RF-API was determined to be 22 J/cm2. Inactivation causing ≥3 log10 reduction in CFU counts after Chl-API required almost twice lower irradiation dose - only 11.5 J/cm2 (Figures 4A, B).
Figure 4 The effect of RF-API (A) and Chl-API (B) on Brevundimonas sp. ESA1 free-floating (planktonic) cells. Horizontal and vertical dashed lines shown in the graphs indicate the minimal irradiation doses thet were required to achieve a 3 log10 reduction of CFU counts of the planktonic cells. +RF +440 nm: irradiated using RF, -RF +440 nm: irradiated not using RF, +Chl +402 nm: irradiated using Chl, -Chl +402 nm: irradiated not using Chl; ns – non-significant. Error bars of some points are too small to be visible.
During RF-API and Chl-API, respective samples of dark controls (samples that were incubated in the dark during/in parallel to API illuminations) were collected and analyzed by determining CFU counts as well. Respective dark controls with and without PSs (RF or Chl) during the time of its incubation parallel to the illumination experiments did not change significantly and fluctuated within appropriate limits (Table 2).
The minimal required doses of irradiation to achieve ≥3 log10 CFU reduction of Brevundimonas sp. biofilm ESA1 by RF-API and Chl-API were determined to be 138 J/cm2 and 67,5 J/cm2, respectively (Figures 5A, B). Overall, API studies revealed that Brevundimonas sp. ESA1 in its sessile and planktonic growth modes is more sensitive to 402 nm light in combination with Chl.
Figure 5 The effect of RF- (A) and Chl-API (B) on Brevundimonas sp. ESA1 biofilm. Horizontal and vertical dashed lines shown in the graphs indicate the minimal irradiation doses that were required to achieve 3 log10 reduction of CFU counts of the tested bacterial biofilm. +RF +440 nm: irradiated using RF, -RF +440 nm: irradiated not using RF, +Chl +402 nm: irradiated using Chl, -Chl +402 nm: irradiated not using Chl; ns – non-significant. Error bars of some points are too small to be visible.
During RF-API and Chl-API, respective samples of dark controls were collected and analyzed by determining CFU. Respective dark controls with and without PSs (RF or Chl) during the time of its incubation parallel to the illumination experiments did not change significantly and fluctuated within appropriate limits, as well (Table 3).
It was also determined that effective inactivation of Brevundimonas sp. ESA1 biofilms using 402 nm light irradiation without Chl can also be achieved. Although, to reach the minimal 3 log10 reduction, a higher dose of ~95 J/cm2 was required compared to Chl-based photoinactivation (Figure 5B).
For a better understanding of the intrinsic sensitivity of the Brevundimonas sp. ESA1 biofilms to the 402 nm light, high irradiation doses were also applied on the planktonic state cells. Results showed that planktonic cells of Brevundimonas sp. ESA1 are also sensitive to 402 nm light irradiation without using appropriate PS. Compared to biofilm, ~60 J/cm2 dose of 402 nm light was needed to achieve ≥3 log10 reduction of CFU counts. Either way, the photoinactivation of Brevundimonas sp. ESA1 biofilms solely by 402 nm light need to be studied in more detail to make unquestionable conclusions about the reasons and internal mechanisms of such sensitivity. Nevertheless, both planktonic and biofilm states Brevundimonas sp. ESA1 were generally determined to be more effectively killed by light (both 402 and 440 nm) in combination with the corresponding Chl and RF PSs used in this work. In addition, compared to the use of light only, using light in combination with PSs ensures that bacteria will not develop resistance to this antibacterial technology.
One of the possibilities to practically apply 402 or 440 nm illumination (in hospitals, food settings or spacecraft) to ensure API is to increase the blue part of the general illumination light sources. However, general illumination light sources (even if re-worked to have API function) cannot provide such high irradiance on a wide surface, e.g., 5 mW/cm2 and higher values that were used in this work to have a bactericidal effect on planktonic and sessile bacterial cells. Therefore, irradiation for more than 24 h to achieve the desired illumination doses should be considered. To test the long-term illumination, an experiment using constant lower irradiance (1.4 mW/cm2) plate emitting only 450 nm blue light was used (Figure 2A). Such a “Royal blue” color illumination spectra was chosen since it is widely used in LED-based illuminations systems from plant or aquarium illumination, color lighting to general lighting (part of white light). Furthermore, modern LEDs are achieving extraordinarily high efficiency compared to other light sources. In the current study, only Brevundimonas sp. ESA1 biofilm was subjected to a long-term API using a CIP.
Results of API of Brevundimonas sp. ESA1 biofilm using CIP at 1.4 mW/cm2 showed that long illumination using lower irradiance in combination with either RF or Chl or even without any PSs could be highly effective. For RF-API, irradiation time of 28 h using CIP was equal to a dose of 141.1 J/cm2, and for Chl-API, irradiation time for 20 h ensured a dose of 100.8 J/cm2 (Figure 6). Such long-term irradiation time were used for testing since it was determined that RF-API and Chl-API doses of 138 J/cm2 and 67.5 J/cm2, respectively, ensure ≥3 log10 reduction of CFU when using LED irradiation boxes (Figures 5A, B). Long-term Chl-API resulted in >4.3 log10 CFU count reduction of the biofilm (Figure 6), although 450 nm irradiance is not optimal for the photoexcitation of Chl. In the previous experiment using the irradiation box emitting 402 nm at 20 mW/cm2 in combination with Chl, a dose of 67,5 J/cm2 was needed to achieve a minimal 3 log10 reduction of CFU count of Brevundimonas sp. ESA1 biofilm (Figure 5B). Long-term Chl-API irradiation time for the achievement of minimal 3 log10 reduction can be probably reduced.
Long-term RF-API resulted in ≥3.3 log10 reductions of CFU counts of the biofilm (Figure 6). The latter result corresponds to the one obtained using the irradiation box (440 nm at 25 mW/cm2 dose 138 J/cm2) (Figure 5A). Long-term illumination of the biofilm without PSs resulted in a bactericidal effect (>3 log10 CFU reduction) as well. Therefore, long-term irradiation is efficient not only with RF but also Chl, even though 450 nm is not optimal for the photoexcitation of Chl. Interestingly, long-term blue light (450 nm) irradiation used without PSs is also highly effective in killing Brevundimonas sp. ESA1 biofilm caused ≥3.4 log10 CFU count reduction of the biofilm.
Figure 6 Effect of long-term Chl- and RF-API using CIP at 1.4 mW/cm2 (450 nm). +Chl+450 nm/-Chl+450 nm – irradiated 20 h with and without Chl, respectively; +RF+450 nm/-RF+450 nm – irradiated 28 h with and without RF, respectively; -PS+450 nm/-PS-450 nm – irradiated and not irradiated 20-28 h without PSs (Chl or RF), respectively.
Brevundimonas sp. ESA1 biofilm viability after the RF-API and Chl-API (using the irradiation box and irradiation plate) was also investigated by analyzing biofilm-forming cells’ ability to reduce resazurin-based dye alamarBlue™. The latter helps monitor the living cell’s reducing environment and quantitatively measure its viability changes. The dye is water-soluble, non-toxic and permeates cell membranes easily; it is stable (even in culture media) and, therefore, long monitoring is possible. The main active component of alamarBlue™ – resazurin is a redox indicator and has an oxidation-reduction potential (E0) of +380 mV at 25°C, pH 7. In a living cell, resazurin (oxidized form) that is blue and non-fluorescent can be reduced in multiple metabolic reactions (by NADPH (E0 = 320 mV), FADH (E0 = 220 mV), dihydrolipoamine dehydrogenases, NAD(P)H:quinoneoxidoreductases, etc.) to resofurin (reduced state) which has pink color and is highly fluorescent. The change from oxidized to reduced state allows quantitative colorimetric and fluorometric measurements, which in turn allow to determine cell health and viability (Rampersad, 2012; Bonnier et al., 2015). In this study alamarBlue™ changes from reduced to the oxidized state were measured by determining changes in fluorescence.
Viability determination via testing of photoinactivated Brevundimonas sp. ESA1 biofilm’s ability to reduce alamarBlueTM showed that 3 log10 CFU count reduction causing RF-API (irradiation dose ~138 J/cm2) and Chl-API (irradiation dose ~67.5 J/cm2) induced cellular changes that lead to the inability to reduce alamarBlue™. Such findings approve previously achieved results that Brevundimonas sp. ESA1 biofilm after the RF- and Chl-API suffers significant viability loss.
Calculated percentage reduction of alamarBlue™ during the recorded time of incubation of appropriately photoinactivated biofilm, and the dye did not reach more than 15% (Figure 7A) and 10% (Figure 7B) after RF-API (+RF+440 nm) and Chl-API (+Chl+402 nm), respectively. The biofilm irradiated with 440 nm light without RF (-RF+440 nm) could reduce alamarBlue™, and the ability grew during the incubation time until 8-9 h. At the later time point, percentage reduction reached ~68%; however, subsequent decline was further determined. Dark control biofilm that was incubated with RF (+RF-440 nm) showed an upward dye percentage reduction ability trend with a maximum of ~70% reached at 10-11 h. The biofilm that was incubated in the dark without RF (PBS buffer was used instead) showed increasing alamarBlue™ percent reduction ability during the time of incubation and reached maximum ~85% at 11 h of incubation. Test groups that were irradiated without RF (-RF+440 nm) and incubated (dark control) with RF (+RF-440 nm) had a rather strong ability to reduce the alamarBlue™, which means that the mentioned conditions of treatment of biofilms did not significantly affect the viability (Figure 7A). Test groups of biofilms that were irradiated without Chl (-Chl+402 nm) or incubated in the dark with (+Chl-402 nm) or without Chl (-Chl-402 nm), exhibited similar alamarBlue™ percentage reduction values (Figure 7B) as it was determined after RF-API (Figure 7A).
Figure 7 Brevundimonas sp. ESA1 biofilm ability to reduce alamarBlue™ after API: (A) - RF-API (440 nm at 25 mW/cm2 until dose of 138 J/cm2 was reached) and (B) – after Chl-API (402 nm at 20 mW/cm2 until dose 67.5 J/cm2 was reached) using the irradiation box; (C) - after RF-API (450 nm at 1.4 mW/cm2 until dose of 141.1 J/cm2 was reached) and (D) - Chl-API (450 nm at 1.4 mW/cm2 until the dose of 100.8 J/cm2 was reached) using irradiation plate. Described irradiation doses ensured the reduction of ≥3 log10 in CFU counts. Designations: A, B - +RF+440 nm/-RF+440 nm, +Chl+402 nm/-Chl+402 nm: biofilms irradiated by 440 nm or 402 nm with/without 0.011 mM RF or 0.015 mM Chl, respectively; +RF-440 nm/-RF-440 nm, +Chl-402 nm/-Chl-402 nm: dark controls where biofilms were and were not, respectively, incubated with 0.011 mM RF or 0.015 mM Chl; C, D - +RF-450 nm/-RF-450 nm, +Chl-450 nm/-Chl-450 nm: dark controls of long-term irradiation experiment where biofilms were and were not, respectively, incubated with 0.011 mM RF or 0.015 mM Chl. In cases where irradiation or incubation without PSs was performed, 0.01 M PBS (pH 7.4) was used.
In general, viability testing with alamarBlue™ after RF-API and Chl-API (using the irradiation box) confirmed the results obtained by determining CFU counts of surviving cells.
After the photoinactivation using 450 nm CIP at 1.4 mW/cm2 in combination with RF and Chl, the biofilm of Brevundimonas sp. ESA1 exhibited, respectively, only less than 10% or no alamarBlue™ reduction activity. Such results indicate that irradiation using a low irradiance intensity plate for a long period of time caused a significant or total viability loss when using RF or Chl, respectively, compared to AFI using the irradiation box. The control biofilm group that was irradiated with 450 nm without RF or Chl (PBS buffer was used instead of PSs) showed the ability to reduce no more than 20% of alamarBlue™ during all the incubation period (Figures 7C, D). Dark control groups, where biofilm was incubated with RF or Chl (groups +RF-450 nm and +Chl-450 nm, respectively) in the dark for an amount of time corresponding to the irradiation time that was used to cause ≥3 log10 CFU reduction, showed a low percentage ability of alamarBlue™ reduction. The percentage reduction of the latter tended to grow; however, in the last 4 h of incubation of biofilms with the alamarBlue™ it reached maximal values that were ~60% (Figures 7C, D). In comparison to +RF-440 nm, +Chl-402 nm and -RF-440 nm, -Chl-402 nm groups that were incubated for a shorter time using the irradiation box, +RF-450 nm, +Chl-450 nm and -RF/-Chl-450 nm after the long dark incubation showed ~20% lower ability to reduce alamarBlue™. The prolonged incubation that was carried out in the study with CIP could itself have a negative effect on reduction capability that reflects the viability of the biofilm. A negative effect on cell viability could have occurred due to the lack of nutrients during the long incubation time.
The ability of the Brevundimonas sp. ESA1 biofilm to resuscitate and grow in the planktonic state in nutrient-rich liquid LB media (in which planktonic cells for biofilm formation were routinely grown) was lost following irradiation with 450 nm at 1.4 mW/cm2 for 20 (Chl-API) and 28 h (RF-API). No changes in OD600 of the +RF+450 nm and +Chl+450 nm groups were detected for 72 h (Table 4).
Table 4 Ability of the biofilm-forming Brevundimonas sp. ESA1 cells to regrow in LB media after long-term RF-API and Chl-API (+RF+450 nm and +Chl+450 nm, respectively) using CIP, irradiation without PSs (-PSs(+PBS)+450 nm).
Regrowth analysis of the biofilm test group, which was illuminated with 450 nm without PSs (-PS+450 nm) showed that for the first 48 h the growth did not occur; however, on the third day (in 72 h) OD600 reached 1.96 ± 0.02. Irradiation with 450 nm without PSs delayed regrowth for 2 days. All the tested dark controls (+RF-450 nm, +Chl-450 nm, -PSs(+PBS)-450 nm) demonstrated the ability to regrow (Table 4).
Regrowth of Brevundimonas sp. ESA1 biofilm following 3 log10 CFU reduction causing RF- and Chl-API using the irradiation box did not occur for 48 h (OD600 = 0) but bacteria visibly resuscitated on the third day of cultivation in LB with OD600 values reaching 3.55 ± 0.07. Biofilms that were illuminated either with 402 or 440 nm regrew up to OD600 values equal to 3.5 ± 0.70 and 3.65 ± 0.91, respectively, on the second day of growth measurement. Illumination in combination with PSs and sole illumination delayed the regrowth of Brevundimonas sp. ESA1 for 2 and 1 day, respectively. Biofilms that formed dark control groups did not have such lag phases. OD600 changes of all tested biofilm groups after the photoinactivation using the irradiation box are shown in Table 5.
Table 5 Ability of the biofilm-forming Brevundimonas sp. ESA1 cells to regrow in LB media after short-term RF- and Chl-API (+RF+440 nm and +Chl+402 nm, respectively) using the irradiation box, irradiation without PSs (-RF+440 nm and -Chl+402 nm).
The studies of the ability of the biofilm cells to regrow in suspension and previous viability testing experiments via evaluation of alamarBlue™ reduction after the RF- and Chl-API have revealed that, apparently, some cells after the RF-, Chl-API or irradiation without PSs enter viable but non-culturable state (VNBC). Such a conclusion was made since some photoinactivated biofilm test groups were metabolically active (reduced alamarBlue™) but at the same time did not grow in the liquid nutritionally rich LB medium. The biofilm that was exposed to 450 nm light (test group +450 nm -RF/-Chl (+PBS)) was metabolically active and reduced alamarBlue™ for more than 11 h up to ~20% (Figure 7C). However, its OD600 values over 48 h were equal to 0 with subsequent growth detection at 72 h (Table 4). Such results indicate that only a part of the cells entered a VBNC state which was a cause of bacterial resuscitation, while most biofilm cells did not survive after exposure. VBNC state was also induced in Brevundimonas sp. ESA1 biofilm cells were exposed to RF-API (+RF+440 nm test group) and Chl-API (+Chl+402 nm test group) using the irradiation box. Biofilms of these test groups after appropriate PS-based photoinactivations could reduce alamarBlue™ up to ~60-70% at 12-14 h incubation points (Figures 7A, B), but at the same time cell growth in LB media was not detected for 48 h. Subsequently, bacteria resuscitated only on the third day of growth, reaching OD600 corresponding to those measured for the dark controls (Table 5), meaning that most of the bacterial cell population transitioned to VBNC state. Biofilm cells that were exposed to 402 or 440 nm irradiation without PS, recovered faster than those that were subjected to photoinactivation in combination with PSs (Table 5).
Scanning electron microscopy revealed that RF-API and Chl-API using the irradiation box at doses of 138 J/cm2 and ~70 J/cm2, respectively, have induced changes in biofilm cell morphology of investigated bacteria compared to untreated cells (Figure 8).
Figure 8 SEM images of Brevundimonas sp. ESA1 biofilm cells: untreated (-hV –PS: no PSs (RF nor Chl) in dark conditions); after RF-API: 440 nm +RF (treated with 0.011 mM RF and irradiated by 440 nm up to 140 J/cm2), 440 nm -RF (not treated with 0.011 mM RF prior to irradiation, cells were covered with 0.1 M phosphate buffer (pH 7.4) and irradiated by 440 nm up to 140 J/cm2), dark +RF (biofilms treated with 0.011 mM RF and incubated in the dark for an amount of time corresponding to time needed to achieve 140 J/cm2); after Chl-API: 402 nm +Chl (treated with 0,015 mM Chl and irradiated by 402 nm up to 70 J/cm2), 402 nm -Chl (not treated with 0,015 mM Chl prior to irradiation, cells were covered with 0.1 M phosphate buffer (pH 7.4) and irradiated by 402 nm up to 70 J/cm2), dark +Chl (biofilms treated with 0,015 mM M Chl and incubated in the dark for an amount of time corresponding to time needed to achieve 70 J/cm2).
After both RF-API and Chl-API, mechanically detached biofilm cells appeared to have disrupted and perforated cell walls, and some appeared lysed. Significant morphological changes were also seen after irradiation with 402 nm without using Chl. That is a logical outcome since CFU count analysis after irradiation has shown that 402 nm light without PS can have a reducing effect on viability as well (Figure 5B). Cells of the dark controls did not have any noticeable damage to the cell walls, but the surface of those incubated in the dark with RF did not look as smooth as after incubation with Chl.
This study was the first to demonstrate the efficiency of the RF- and Chl-mediated photoinactivation of Brevundimonas sp. ESA1 biofilms. In particular, it was shown that Chl-based API required half the irradiation dose compared to RF-API. Therefore, Chl-API can be considered a more effective method for the inactivation of studied biofilms and planktonic cells. Moreover, it was determined that effective inactivation of Brevundimonas sp. ESA1 biofilms using 402 nm light irradiation without Chl can also be achieved. Although to reach the minimal 3 log10 reductions, a higher dose was required compared to Chl-based photoinactivation (95 J/cm2 instead of 67.5 J/cm2). Such phenomenon has not been observed after RF-API and Chl-API of Brevundimonas sp. ESA1 planktonic cells in the used dosage range of the photoinactivation. The use of PS, in the case of Chl-API of Brevundimonas sp. ESA1 biofilm can be considered redundant/unnecessary to achieve inactivation (bactericidal or bacteriostatic effect). The fact that biofilms can be sensitive to light without PSs can have practical significance in facilitating the application of the technology in systems where spreading PSs can be complicated, e.g., in a spacecraft environment, where microgravity conditions prevail. However, the cause of Brevundimonas sp. ESA1 to be sensitive, particularly in its biofilm form to 402 nm only (without Chl) is currently unknown. In general, the sensitivity of biofilms to blue light can be explained by the fact that bacteria can have endogenous porphyrins that can be sensitized by 402 nm and can generate ROS, resulting in cellular photo-oxidative reactions that initiate various cellular damages and death; the light can also activate prophages that cause bacterial cell lysis (Ferrer-Espada et al., 2019). A few studies have shown that biofilms of different Gram-negative (Salmonella, Escherichia coli O157:H7, etc.) bacteria can be equally sensitive or even more sensitive to irradiation compared to planktonic cells (Niemira and Solomon, 2005; Niemira, 2007; Niemira, 2010). The sensitivity to API of the biofilm and the planktonic cells of bacteria can be different and depend on the metabolic states and the growth phase as well (Blee et al., 2020). It can also be assumed that the cause of the higher sensitivity of the Brevundimonas sp. ESA1 biofilm to the 402 nm light is somehow associated with its extracellular polymeric matrix (Flemming and Wingender, 2010). Although, EPS is usually the cause of the higher doses needed to inactivate bacteria in sessile form compared to planktonic cells. For example, this study’s experimental results have shown that about 6 times higher irradiation doses are needed to achieve the bactericidal effect on biofilms compared to planktonic cells. Therefore, the improvement of API technology for the more effective killing of bacterial biofilms should be certainly considered. The efficiency of API can be improved by testing higher concentrations of PSs, a step of preincubation with PSs before irradiation and synergy studies. Additionally, using antimicrobials can be done as well.
API is a two-stage technology consisting of the natural PS, which must be applied on a surface, and an illumination system providing a sufficient dose of light to inactivate bacteria. This makes it complicated, especially for the application in microgravity conditions, and requires a well-developed application plan as well as proper engineering solutions. Reworking of currently installed lighting fixtures (e.g. SSLAs on ISS), increasing the blue part of the general lightning, and long irradiation time could be one of the options. Based on this assumption, to test the long-term illumination, an experiment using constant lower irradiance (1.4 mW/cm2) plate emitting only 450 nm blue light was used (Figure 2A). Such color illumination spectrum was chosen since it is widely used in LED-based systems such as general lighting (part of white light).
Results of API of Brevundimonas sp. ESA1 biofilm using CIP at 1.4 mW/cm2 showed that long illumination using lower irradiance in combination with either RF or Chl or even without any PSs could be highly effective. However, some additional long-term irradiation experiments using CIP can be performed to evaluate the 450 nm effect in combination with RF, Chl or without the use of PSs, and if the internal porphyrins might have had a significance for the API of the Brevundimonas sp. ESA1. The latter phenomenon has already attracted increasing attention and several studies have shown that blue light, particularly in the wavelength range of 405–470 nm has intrinsic antimicrobial effect on different microbes including Gram-positive and Gram-negative bacteria without the addition of exogenous PSs (Dai et al., 2012; Hoenes et al., 2018; Gwynne and Gallagher, 2018: Hadi et al., 2020). Nevertheless, long-term irradiation indeed showed to be effective and promising for practical implementation of API customization. However, reworking installed lighting fixtures or sending additional API-dedicated illumination to spacecraft definitely results in extra expenses that must be evaluated and weighted in detail. On the other hand, when developing new modules for ISS or other space missions, the API could be considered an antimicrobial technique. Therefore, if performed on earth, the integration of API illumination systems would have a neglectable effect on the total costs and weight of the project.
Viability testing with alamarBlue™ after RF-API and Chl-API (using the irradiation box and CIP) confirmed the results obtained by determining CFU counts of surviving cells. However, alamarBlue™ reduction studies in addition to bacterial regrowth studies, had shown that Brevundimonas sp. ESA1 biofilm cells, after the application of RF-API and Chl-API, enter VBNC state.
Only a few published studies announce the transition of certain bacterial species to the VBNC state after exposure to blue light or violet-blue lights (Abana et al., 2017; Hoenes et al., 2018).
In general, VBNC state is characteristic to non-spore-forming, usually Gram-negative bacteria and appears under various stressful conditions. VBNC cells may be resuscitated back to cultivable cells under suitable stimuli. The conditions that trigger the induction of the VBNC state and resuscitation can be different for different bacteria species, e.g., pathogens usually resuscitate only in vivo (Li et al., 2014; Zhang et al., 2021). This study was the first to demonstrate that bacteria belonging to the genus Brevundimonas sp. ESA1 can enter the VBNC state after its photoinactivation and in some cases resuscitate in nutrient-rich conditions. Moreover, undesired growth after the long-term RF- and Chl-API is unlikely to occur even in nutrient-rich environments.
Despite the efficiency of Chl- and RF-based API against Brevundimonas sp. ESA1 and other bacteria (that was demonstrated elsewhere), use of the PSs have a huge potential for improvement. For example, the mixes of PSs and the combinations of PSs with polymers and other materials can be investigated. Moreover, an in-depth investigation of the long-term, low-irradiance API technologies could help find photoinactivation solutions under general or natural lighting conditions.
Design and development of the API PS solution already integrated into the newly developed space premises and/or modules should be considered in order to apply the technology conveniently in conditions of low gravity. If the integration of the PSs is not possible, investigation and design of API application techniques (e.g. sprinklers) in microgravity conditions must be considered as well.
API based on natural PSs RF or Chl and illumination by blue light (402-450 nm) has the potential to be applied as an antimicrobial technique against planktonic and, most importantly, more recalcitrant biofilm form of Brevundimonas sp. ESA1. Recently, some species of the latter bacterial genus have been revealed as a global opportunistic pathogen that can be dangerous for individuals with chronic underlying diseases and immunocompromised people. Brevundimonas spp. are abundant in terrestrial and even confined spacecraft environments.
The main advantage of the RF- and Chl-mediated API compared to other antimicrobial methods is that this technology is non-toxic and could be safely used in closed-loop, food, drinking water, and other systems as an antimicrobial technology posing no risk to humans or other living creatures. Moreover, there has been only a neglectable or no resistance development towards API observed since the beginning of its use. Implementing the API technology in the confined systems as spacecraft, its application could consist of three steps, in particular, preparation of the aqueous solutions of the PSs, application of the PSs on the target surface, and irradiation of that surface by the required light spectrum and sufficient light amount (dose).
In this work, by comparing the optical absorption spectra of the PSs and irradiance spectra of LEDs, it was determined that 402 nm and 440 nm are optimal to excite Chl and RF, respectively. Photostability experiments showed the photomodifications of both PSs under the conditions subsequently used to inactivate Brevundimonas sp. ESA1. Besides the demonstration of the RF-based and Chl-based API conditions needed to inactivate Brevundimonas biofilms using two different illumination systems, a study of the API treated bacteria to reduce alamarBlue™ and regrowth studies revealed that Brevundimonas sp. ESA1 enters VNBC state without resuscitation after long-term irradiation in conditions tested in this work.
Nevertheless, further investigation of the API applicability should be carried out, focusing on certain application areas such as HVAC, water supply, sanitary systems, plant growth facilities, etc.
The data presented in the study are deposited in the NCBI GenBank repository, accession number ON237360; https://www.ncbi.nlm.nih.gov/nuccore/ON237360.1/.
AG and IB, conceptualization, methodology, and data processing. AG, IB, and KB, experiment implementation. AG, formal analysis, writing – original draft, writing – review and editing, visualization. IB, LK, and PV, writing – original draft, writing – review and editing. All authors contributed to the article and approved the submitted version.
Part of the research was funded by the European Space Agency (LT5_1 ESA Contract No. 40000129495/19/NL/SSC).
Authors would like to acknowledge the European Space Agency (ESA).
The authors declare that the research was conducted in the absence of any commercial or financial relationships that could be construed as a potential conflict of interest.
All claims expressed in this article are solely those of the authors and do not necessarily represent those of their affiliated organizations, or those of the publisher, the editors and the reviewers. Any product that may be evaluated in this article, or claim that may be made by its manufacturer, is not guaranteed or endorsed by the publisher.
Abana, C. M., Brannon, J. R., Ebbott, R. A., Dunigan, T. L., Guckes, K. R., Fuseini, H., et al. (2017). Characterization of blue light irradiation effects on pathogenic and nonpathogenic Escherichia coli. Microbiologyopen. 6 (4), e00466. doi: 10.1002/mbo3.466
Adelowo, O. O., Fagade, O. E. (2009). The tetracycline resistance gene tet39 is present in both gram-negative and gram-positive bacteria from a polluted river, southwestern Nigeria. Lett. Appl. Microbiol. 48, 167–172. doi: 10.1111/j.1472-765x.2008.02523.x
Altschul, S. F., Gish, W., Miller, W., Myers, E. W., Lipman, D. J. (1990). Basic local alignment search tool. J. Mol. Biol. 215 (3), 403–410. doi: 10.1016/S0022-2836(05)80360-2
Astanov, S., Sharipov, M. Z., Fayzullaev, A. R., Kurtaliev, E. N., Nizomov, N. (2014). Spectroscopic study of photo and thermal destruction of riboflavin. J. Mol. Struct. 1071, 133–138. doi: 10.1016/j.molstruc.2014.04.077
Bashir, M., Ahmed, M., Weinmaier, T., Ciobanu, D., Ivanova, N., Pieber, T. R., et al. (2016). Functional metagenomics of spacecraft assembly cleanrooms: presence of virulence factors associated with human pathogens. Front. Microbiol. 9 (7). doi: 10.3389/fmicb.2016.01321
Be, N. A., Avila Herrera, A., Allen, J. E., Singh, N., Sielaff, A., Jaing, C., et al. (2017). Whole metagenome profiles of particulates collected from the international space station. Microbiome. 5 (1), 81. doi: 10.1186/s40168-017-0292-4
Blee, J. A., Roberts, I. S., Waigh, T. A. (2020). Membrane potentials, oxidative stress and the dispersal response of bacterial biofilms to 405 nm light. Phys. Biol. 17 (3), 036001. doi: 10.1088/1478-3975/ab759a
Bonnier, F., Keating, M. E., Wróbel, T. P., Majzner, K., Baranska, M., Garcia-Munoz, A., et al. (2015). Cell viability assessment using the alamar blue assay: A comparison of 2D and 3D cell culture models. Toxicol. Vitro. 29 (1), 124–131. doi: 10.1016/j.tiv.2014.09.014
Borisov, V., Deshevaya, E., Grachov, E., Grigoryan, O., Tchurilo, I., Tsetlin, V. (2003). The "SCORPION" experiment onboard the international space station. Preliminary results. Adv. Space Res. 32 (11), 2373–2378. doi: 10.1016/SO273-1177(03)00726-9
Buchovec, I., Gricajeva, A., Kalėdienė, L., Vitta, P. (2020). Antimicrobial photoinactivation approach based on natural agents for control of bacteria biofilms in spacecraft. IJMS. 21 (18), 6932. doi: 10.3390/ijms21186932
Buchovec, I., Klimkaitė, L., Sužiedėlienė, E., Bagdonas, S. (2022). Inactivation of opportunistic pathogens Acinetobacter baumannii and Stenotrophomonas maltophilia by antimicrobial photodynamic therapy. Microorganisms. 10 (3), 506. doi: 10.3390/microorganisms10030506
Buchovec, I., Lukseviciūtė, V., Kokstaite, R., Labeikyte, D., Kaziukonyte, L., Luksiene, Z. (2017). Inactivation of gram (–) bacteria Salmonella enterica by chlorophyllin-based photosensitization: Mechanism of action and new strategies to enhance the inactivation efficiency. J. Photochem. Photobiol. B Biol. 172, 1–10. doi: 10.1016/j.jphotobiol.2017.05.008
Buchovec, I., Lukseviciute, V., Marsalka, A., Reklaitis, I., Luksiene, Z. (2016). Effective photosensitization-based inactivation of gram (–) food pathogens and molds using the chlorophyllin–chitosan complex: Towards photoactive edible coatings to preserve strawberries. Photochem. Photobiol. Sci. 15, 506–516. doi: 10.1039/c5pp00376h
Buchovec, I., Paskeviciute, E., Luksiene, Z. (2010). Photodynamic inactivation of food pathogen listeria monocytogenes. Food Technol. Biotechnol. 48 (2), 207–213.
Checinska, A., Probst, A. J., Vaishampayan, P., White, J. R., Kumar, D., Stepanov, V. G., et al. (2015). Microbiomes of the dust particles collected from the international space station and spacecraft assembly facilities. Microbiome. 3, 50. doi: 10.1186/s40168-015-0116-3
Ciofu, O., Moser, C., Jensen, P.Ø., Høiby, N. (2022). Tolerance and resistance of microbial biofilms. Nat. Rev. Microbiol. 20, 621–635. doi: 10.1038/s41579-022-00682-4
Clinical and Laboratory Standards Institute (CLSI) (1999). Methods for determining bactericidal activity of antimicrobial agents; approved guideline. CLSI document M26-A 19 (18), 1–29.
Dai, T., Gupta, A., Murray, C. K., Vrahas, M. S., Tegos, G. P., Hamblin, M. R. (2012). Blue light for infectious diseases: Propionibacterium acnes, helicobacter pylori, and beyond? Drug Resist. Update 4 (15), 223–236. doi: 10.1016/j.drup.2012.07.001
Dartnell, L. R., Hunter, S. J., Lovell, K. V., Coates, A. J., Ward, J. M. (2010). Low-temperature ionizing radiation resistance of Deinococcus radiodurans and Antarctic dry valley bacteria. Astrobiology. 10 (7), 717–732. doi: 10.1089/ast.2009.0439
Decelle, J. G., Taylor, G. R. (1976). Auto flora in the upper respiratory tract of Apollo astronauts. Appl. Environ. Microbiol. 32, 659–665. doi: 10.1128/aem.32.5.659-665.1976
Ferrer-Espada, R., Liu, X., Goh, X. S., Dai, T. (2019). Antimicrobial blue light inactivation of polymicrobial biofilms. Front. Microbiol. 10. doi: 10.3389/fmicb.2019.00721
Flemming, H.-C., Wingender, J. (2010). The biofilm matrix. Nat. Rev. Microbiol. 8, 623–633. doi: 10.1038/nrmicro2415
Flemming, H.-C., Wingender, J., Szewzyk, U., Steinberg, P., Rice, S. A., Kjelleberg, S. (2016). Biofilms: an emergent form of bacterial life. Nat. Rev. Microbiol. 14, 563–575. doi: 10.1038/nrmicro.2016.94
Ghosh, S., Osman, S., Vaishampayan, P., Venkateswaran, K. (2010). Recurrent isolation of extremotolerant bacteria from the clean room where phoenix spacecraft components were assembled. Astrobiology. 10 (3), 325–335. doi: 10.1089/ast.2009.0396
Gricajeva, A., Bendikienė, V., Kalėdienė, L. (2016). Lipase of Bacillus stratosphericus L1: cloning, expression and characterization. Int. J. Biol. Macromol. 92, 96–104. doi: 10.1016/j.ijbiomac.2016.07.015
Gwynne, P. J., Gallagher, M. P. (2018). Light as broad-spectrum antimicrobial. Front. Microbiol. 9:119. doi: 10.3389/fmicb.2018.00119
Hadi, J., Wu, S., Brightwell, G. (2020). Antimicrobial blue light versus pathogenic bacteria: mechanism, application in the food industry, hurdle technologies and potential resistance. Foods. 9 (12), 1895. doi: 10.3390/foods9121895
Hall-Stoodley, L., Costerton, J. W., Stoodley, P. (2004). Bacterial biofilms: from the natural environment to infectious disease. Nat. Rev. Microbiol. 2, 95–108. doi: 10.1038/nrmicro821
Hamblin, M. R. (2016). Antimicrobial photodynamic inactivation: a bright new technique to kill resistant microbes. Curr. Opin. Microbiol. 33, 67–73. doi: 10.1016/j.mib.2016.06.008
Han, X. Y., Andrade, R. A. (2005). Brevundimonas diminuta infections and its resistance to fluoroquinolones. J. Antimicrob. Chemother. 55 (6), 853–859. doi: 10.1093/jac/dki139
Hoenes, K., Hess, M., Vatter, P., Spellerberg, B., Hessling, M. (2018). 405 nm and 450 nm photoinactivation of Saccharomyces cerevisiae. Eur. J. Microbiol. Immunol. 8 (4), 142–148. doi: 10.1556/1886.2018.00023
Hu, X., Huang, Y. Y., Wang, Y., Wang, X., Hamblin, M. R. (2018). Antimicrobial photodynamic therapy to control clinically relevant biofilm infections. Front. Microbiol. 9 (1299). doi: 10.3389/fmicb.2018.01299
Jori, G., Brown, S. B. (2004). Photosensitized inactivation of microorganisms. Photochem. Photobiol. Sci. 3 (5), 403–405. doi: 10.1039/b311904c
Kashef, N., Hamblin, M. R. (2017). Can microbial cells develop resistance to oxidative stress in antimicrobial photodynamic inactivation? Drug Resist. Updat. 31, 31–42. doi: 10.1016/j.drup.2017.07.003
Kawamura, Y., Li, Y., Liu, H., Huang, X., Li, Z., Ezaki, T. (2001). Bacterial population in Russian space station “Mir”. Microbiol. Immunol. 45, 819–828. doi: 10.1111/j.1348-0421.2001.tb01321.x
Koskinen, K., Rettberg, P., Pukall, R., Auerbach, A., Wink, L., Barczyk, S., et al. (2017). Microbial biodiversity assessment of the European space agency's ExoMars 2016 mission. Microbiome. 5 (1), 143. doi: 10.1186/s40168-017-0358-3
Krüger, M., Richter, P. R., Strauch, S. M., Nasir, A., Burkovski, A., Antunes, C. A., et al. (2019). What an Escherichia coli mutant can teach us about the antibacterial effect of chlorophyllin. Microorganisms. 7, 59. doi: 10.3390/microorganisms7020059
La Duc, M. T., Kern, R., Venkateswaran, K. (2004a). Microbial monitoring of spacecraft and associated environments. Microb. Ecol. 47 (2), 150–158. doi: 10.1007/s00248-003-1012-0
La Duc, M. T., Sumner, R., Pierson, D., Venkat, P., Venkateswaran, K. (2004b). Evidence of pathogenic microbes in the international space station drinking water: reason for concern? Habitation (Elmsford). 10 (1), 39–48. doi: 10.3727/154296604774808883
Li, Y., Kawamura, Y., Fujiwara, N., Naka, T., Liu, H., Huang, X., et al. (2004). Sphingomonas yabuuchiae sp. nov. and Brevundimonas nasdae sp. nov., isolated from the Russian space laboratory mir. Int. J. Syst. Evol. 54 (3), 819–825. doi: 10.1099/ijs.0.02829-0
Li, L., Mendis, N., Trigui, H., Oliver, J. D., Faucher, S. P. (2014). The importance of the viable but non-culturable state in human bacterial pathogens. Front. Microbiol. 5. doi: 10.3389/fmicb.2014.00258
Liu, Y., Qin, R., Zaat, S. A. J., Breukink, E., Heger, M. (2015). Antibacterial photodynamic therapy: Overview of a promising approach to fight antibiotic-resistant bacterial infections. J. Clin. Transl. Res. 1 (3), 140–167. doi: 10.18053/jctres.201503.002
Low, A., Ng, C., He, J. (2016). Identification of antibiotic resistant bacteria community and a GeoChip based study of resistome in urban watersheds. Water Res. 106, 330–338. doi: 10.1016/j.watres.2016.09.032
Luksiene, Z. (2005). New approach to inactivation of harmful and pathogenic microorganisms by photosensitization. Food Technol. Biotechnol. 43 (4), 411–418.
Luksiene, Z., Buchovec, I. (2019). Impact of chlorophyllin-chitosan coating and visible light on the microbial contamination, shelf life, nutritional and visual quality of strawberries. Innov. Food Sci. Emerg. Technol. 52, 463–472. doi: 10.1016/j.ifset.2019.02.003
Luksiene, Z., Buchovec, I., Paskeviciute, E. (2010). Inactivation of Bacillus cereus by Na-chlorophyllin-based photosensitization on the surface of packaging. J. Appl. Microbiol. 109, 1540–1548. doi: 10.1111/j.1365-2672.2010.04780.x
Maisch, T. (2015). Resistance in antimicrobial photodynamic inactivation of bacteria. Photochem. Photobiol. Sci. 14, 1518–1526. doi: 10.1038/s41598-018-35594-y
Mazzotta, C., Caragiuli, S., Caporossi, A. (2014). “"Riboflavin and the cornea and implications for cataracts”,” in Handbook of nutrition, diet and the eye. Ed. Preedy, V. R. (London, UK: Academic Press), 123–130. doi: 10.1016/B978-0-12-401717-7.00013-7
Merritt, J. H., Kadouri, D. E., O'Toole, G. A. (2005). Growing and analyzing static biofilms. Curr. Protoc. Microbiol. 1, 1B.1. doi: 10.1002/9780471729259.mc01b01s00
Mijnendonckx, K., Provoost, A., Ott, C. M., Venkateswaran, K., Mahillon, J., Leys, N., et al. (2013). Characterization of the survival ability of Cupriavidus metallidurans and Ralstonia pickettii from space-related environments. Microb. Ecol. 65 (2), 347–360. doi: 10.1007/s00248-012-0139-2
Miranda, C. D., Kehrenberg, C., Ulep, C., Schwarz, S., Roberts, M. C. (2003). Diversity of tetracycline resistance genes in bacteria from chilean salmon farms. Antimicrob. Agents Chemother. 47, 883–888. doi: 10.1128/AAC.47.3.883-888.2003
Mora, M., Mahnert, A., Koskinen, K., Pausan, M. R., Oberauner-Wappis, L., Krause, R., et al. (2016). Microorganisms in confined habitats: microbial monitoring and control of intensive care units, operating rooms, cleanrooms, and the international space station. Front. Microbiol. 7. doi: 10.3389/fmicb.2016.01573
Mora, M., Wink, L., Kogler, I., Mahnert, A., Rettberg, P., Schwendner, P., et al. (2019). Space station conditions are selective but do not alter microbial characteristics relevant to human health. Nat. Commun. 10 (1), 3990. doi: 10.1038/s41467-019-11682-z
Muhammad, M. H., Idris, A. L., Fan, X., Guo, Y., Yu, Y., Jin, X., et al. (2020). Beyond risk: bacterial biofilms and their regulating approaches. Front. Microbiol. 11. doi: 10.3389/fmicb.2020.00928
Nakajima, Y., Yoshizawa, S., Nakamura, K., Ogura, Y., Hayashi, T., Kogure, K. (2017). Draft genome sequences of Tersicoccus phoenicis DSM 30849T, isolated from a cleanroom for spacecraft sssembly, and tersicoccus sp. strain bi-70, isolated from a freshwater lake. Genome Announc. 5 (13), e00079–e00017. doi: 10.1128/genomeA.00079-17
Niemira, B. A. (2007). Irradiation sensitivity of planktonic and biofilm-associated Escherichia coli O157:H7 isolates is influenced by culture conditions. Appl. Environ. Microbiol. 73, 3239–3244. doi: 10.1128/aem.02764-06
Niemira, B. A. (2010). Irradiation sensitivity of planktonic and biofilm-associated Listeria monocytogenes and l. innocua as influenced by temperature of biofilm formation. Food Bioprocess Technol. 3, 257–264. doi: 10.1007/s11947-008-0079-5
Niemira, B. A., Solomon, E. B. (2005). Sensitivity of planktonic and biofilm-associated Salmonella spp. to ionizing radiation. Appl. Environ. Microbiol. 71 (5), 2732–2736. doi: 10.1128/AEM.71.5.2732-2736.2005
Novikova, N., De Boever, P., Poddubko, S., Deshevaya, F., Polikarpov, N., Rakova, N. (2006). Survey of environmental biocontamination on board the international space station. Res. Microbiol. 157 (1), 5–12. doi: 10.1016/j.resmic.2005.07.010
Penesyan, A., Paulsen, I. T., Kjelleberg, S., Gillings, M. R. (2021). Three faces of biofilms: a microbial lifestyle, a nascent multicellular organism, and an incubator for diversity. NPJ Biofilms Microbiomes. 7, 80. doi: 10.1038/s41522-021-00251-2
Rampersad, S. N. (2012). Multiple applications of alamar blue as an indicator of metabolic function and cellular health in cell viability bioassays. Sensors (Basel Switzerland). 12, 12347–12360. doi: 10.3390/s120912347
Regberg, A. B., Castro, C. L., Connolly, H. C., Jr., Davis, R. E., Dworkin, J. P., Lauretta, D. S., et al. (2020). Prokaryotic and fungal characterization of the facilities used to assemble, test, and launch the OSIRIS-REx spacecraft. Front. Microbiol. 11. doi: 10.3389/fmicb.2020.530661
Reichhardt, C., Lim, J. Y., Rice, D., Fong, J. N., Cegelski, L. (2014). Structure and function of bacterial biofilms by solid-state NMR. Biophys. J. 106 (2), 192A. doi: 10.1016/j.bpj.2013.11.1139
Ryan, M. P., Pembroke, J. T. (2018). Brevundimonas spp: Emerging global opportunistic pathogens. Virulence. 9 (1), 480–493. doi: 10.1080/21505594.2017.1419116
Segers, P., Vancanneyt, M., Pot, B., Torck, U., Hoste, B., Dewettinck, D., et al. (1994). Classification of Pseudomonas diminuta leifson and Hugh 1954 and Pseudomonas vesicularis büsing, döll, and freytag 1953 in Brevundimonas gen. nov. as Brevundimonas diminuta comb. nov. and Brevundimonas vesicularis comb. nov., respectively. Int. J. Syst. Bacteriol. 44, 499–510. doi: 10.1099/00207713-44-3-499
Sheraz, M. A., Kazi, S. H., Ahmed, S., Anwar, Z., Ahmad, I. (2014). Photo, thermal and chemical degradation of riboflavin. Beilstein J. Org. Chem. 10, 1999–2012. doi: 10.3762/bjoc.10.208
Sobisch, L.-Y., Rogowski, K.-M., Fuchs, J., Schmieder, W., Vaishampayan, A., Oles, P., et al. (2019). Biofilm forming antibiotic resistant gram-positive pathogens isolated from surfaces on the international space station. Front. Microbiol. 10. doi: 10.3389/fmicb.2019.00543
St Denis, T. G., Dai, T., Izikson, L., Astrakas, C., Anderson, R. R., Hamblin, M. R., et al. (2011). All you need is light: antimicrobial photoinactivation as an evolving and emerging discovery strategy against infectious disease. Virulence. 2 (6), 509–520. doi: 10.4161/viru.2.6.17889
Stieglmeier, M., Rettberg, P., Barczyk, S., Bohmeier, M., Pukall, R., Wirth, R., et al. (2012). Abundance and diversity of microbial inhabitants in European spacecraft-associated clean rooms. Astrobiology. 12 (6), 572–585. doi: 10.1089/ast.2011.0735
Temba, B. A., Fletcher, M. T., Fox, G. P., Harvey, J. J. W., Sultanbawa, Y. (2016). Inactivation of Aspergillus flavus spores by curcumin-mediated photosensitization. Food Control. 59, 708–713. doi: 10.1016/j.foodcont.2015.06.045
Thompson, A. F., English, E. L., Nock, A. M., Willsey, G. G., Eckstrom, K., Cairns, B., et al. (2020). Characterizing species interactions that contribute to biofilm formation in a multispecies model of a potable water bacterial community. Microbiol. (Reading Engl.). 166 (1), 34–43. doi: 10.1099/mic.0.000849
Thornsberry, C. (1983). NCCLS standards for antimicrobial susceptibility tests. Lab. Med. 14 (9), 549–553. doi: 10.1093/labmed/14.9.549
Trotonda, M. P., Tamber, S., Memmi, G., Cheung, A. L. (2008). MgrA represses biofilm formation in Staphylococcus aureus. infect. Immun. 76 (12), 5645–5654. doi: 10.1128/iai.00735-08
Venkateswaran, K., Vaishampayan, P., Cisneros, J., Pierson, D., Rogers, S. O., Perry, J. (2014). International space station environmental microbiome — microbial inventories of ISS filter debris. Appl. Microbiol. Biotechnol. 98 (14), 6453–6466. doi: 10.1007/s00253-014-5650-6
Vornhagen, J., Stevens, M., McCormick, D. W., Dowd, S. E., Eisenberg, J. N., Boles, B. R., et al. (2013). Coaggregation occurs amongst bacteria within and between biofilms in domestic showerheads. Biofouling. 29 (1), 53–68. doi: 10.1080/08927014.2012.744395
Wainwright, M. (2004). Photoinactivation of viruses. Photochem. Photobiol. Sci. 3, 406–411. doi: 10.1039/b311903n
Wong, W. C., Dudinsky, L. A., Garcia, V. M., Ott, C. M., Castro, V. A. (2010). Efficacy of various chemical disinfectants on biofilms formed in spacecraft potable water system components. Biofouling. 26 (5), 583–586. doi: 10.1080/08927014.2010.495772
Yin, R., Wang, M., Huang, Y.-Y., Landi, G., Vecchio, D., Chiang, L. Y., et al. (2014). Antimicrobial photodynamic inactivation with decacationic functionalized fullerenes: Oxygen-independent photokilling in presence of azide and new mechanistic insights. Free Radic. Biol. Med. 79, 14–27. doi: 10.1016/j.freeradbiomed.2014.10.514
Zea, L., Nisar, Z., Rubin, P., Cortesao, M., Luo, J., McBride, A., et al. (2018). Design of a spaceflight biofilm experiment. Acta Astronaut. 148, 294–300. doi: 10.1016/j.actaastro.2018.04.039
Zhang, X. H., Ahmad, W., Zhu, X. Y., Chen, J., Austin, B. (2021). Viable but nonculturable bacteria and their resuscitation: implications for cultivating uncultured marine microorganisms. Mar. Life Sci. Technol. 3, 189–203. doi: 10.1007/s42995-020-00041-3
Zhang, J., Li, W., Chen, J., Wang, F., Qi, W., Li, Y. (2019a). Impact of disinfectant on bacterial antibiotic resistance transfer between biofilm and tap water in a simulated distribution network. Environ. pollut. 246, 131–140. doi: 10.1016/j.envpol.2018.11.077
Keywords: bacterial biofilms, antimicrobial photoinactivation, riboflavin, chlorophyllin, Brevundimonas
Citation: Gricajeva A, Buchovec I, Kalėdienė L, Badokas K and Vitta P (2022) Riboflavin- and chlorophyllin-based antimicrobial photoinactivation of Brevundimonas sp. ESA1 biofilms. Front. Cell. Infect. Microbiol. 12:1006723. doi: 10.3389/fcimb.2022.1006723
Received: 01 August 2022; Accepted: 01 September 2022;
Published: 21 September 2022.
Edited by:
Rodnei Dennis Rossoni, Independent researcher, São José dos Campos, BrazilReviewed by:
Daniel Heredia, Universidad Nacional de Río Cuarto, ArgentinaCopyright © 2022 Gricajeva, Buchovec, Kalėdienė, Badokas and Vitta. This is an open-access article distributed under the terms of the Creative Commons Attribution License (CC BY). The use, distribution or reproduction in other forums is permitted, provided the original author(s) and the copyright owner(s) are credited and that the original publication in this journal is cited, in accordance with accepted academic practice. No use, distribution or reproduction is permitted which does not comply with these terms.
*Correspondence: Alisa Gricajeva, YWxpc2EuZ3JpY2FqZXZhQGdtYy52dS5sdA==
Disclaimer: All claims expressed in this article are solely those of the authors and do not necessarily represent those of their affiliated organizations, or those of the publisher, the editors and the reviewers. Any product that may be evaluated in this article or claim that may be made by its manufacturer is not guaranteed or endorsed by the publisher.
Research integrity at Frontiers
Learn more about the work of our research integrity team to safeguard the quality of each article we publish.