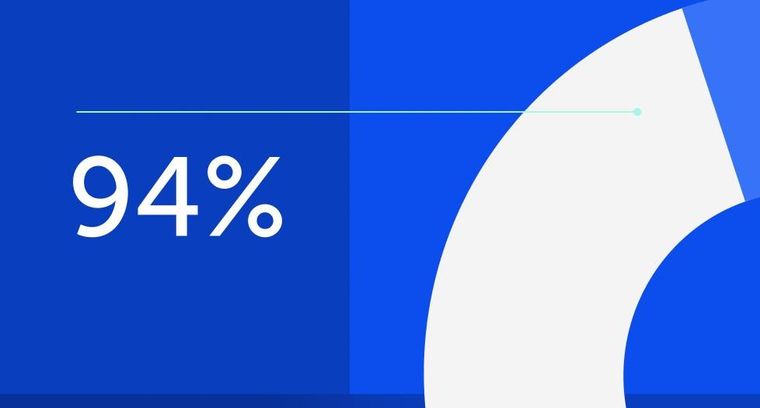
94% of researchers rate our articles as excellent or good
Learn more about the work of our research integrity team to safeguard the quality of each article we publish.
Find out more
ORIGINAL RESEARCH article
Front. Cell. Infect. Microbiol., 12 January 2023
Sec. Clinical Microbiology
Volume 12 - 2022 | https://doi.org/10.3389/fcimb.2022.1000445
This article is part of the Research TopicEnterobacteriaceae Antimicrobial Agents and Resistance: Relationship with the Therapeutic Approach, Volume IIView all 11 articles
Rapid evaluation of antimicrobial susceptibility is important in the treatment of nosocomial infections by Gram-negative bacteria, which increasingly carry carbapenemases and metallo-β-lactamases. We developed loop-mediated isothermal amplification (LAMP)-based assays for four β-lactamase genes (blaKPC, blaNDM-1, blaIMP-1 group, and blaVIM). The assays were evaluated using eight reference bacterial strains (Klebsiella pneumoniae, Escherichia coli, Pseudomonas aeruginosa, and Acinetobacter bereziniae) harboring six β-lactamase genes. A total of 55 Gram-negative bacterial strains, including 47 clinical P. aeruginosa isolates, fully characterized by next-generation sequencing (NGS), were used to evaluate the LAMP assays. The results were compared to those of conventional PCR. The LAMP assays were able to detect as few as 10 to 100 copies of a gene, compared to 10 to 104 copies for conventional PCR. The LAMP assay detected four β-lactamase genes with a sensitivity similar to that using purified DNA as the template in DNA-spiked urine, sputum, and blood specimens. By contrast, the sensitivity of PCR was 1- to 100-fold lower with DNA-spiked clinical specimens. Therefore, the LAMP assays were proved to be an appropriate tool for the detection of four β-lactamases.
Antimicrobial resistance (AMR) is a serious public health problem globally (Laxminarayan et al., 2016). Increased use of antimicrobial drugs promotes the emergence of antimicrobial-resistant bacterial strains and related infections. Some of those bacteria have become resistant to several antibiotics, including carbapenems and third-generation cephalosporins (Arumugham et al., 2022; Palacios-Baena et al., 2021)—the best-available antibiotics for treating infections by multidrug-resistant bacteria. In 2017, the World Health Organization (WHO) published a global priority list of antibiotic-resistant bacteria to guide research, discovery, and the development of new antibiotics (WHO, 2017). These critical multidrug-resistant bacteria include carbapenem-resistant Acinetobacter baumannii, carbapenem-resistant Pseudomonas aeruginosa, and carbapenem-resistant/third-generation cephalosporin-resistant Enterobacteriaceae (including Klebsiella pneumoniae, Escherichia coli, and Enterobacter spp.). These have marked effects on mortality and healthcare.
Carbapenemases are categorized into Ambler class A, B, and D β-lactamases. Ambler classes A and D are serine proteases while class B enzymes are metallo-β-lactamases (MBL). Klebsiella pneumoniae carbapenemase (blaKPC) is plasmid-encoded and is the most prevalent class A carbapenemase worldwide, including in the United States (Chen et al., 2014). New Delhi metallo-β-lactamase (blaNDM), imipenem-resistant Pseudomonas type carbapenemase (blaIMP), and Verona integron-encoded metallo-β-lactamase (blaVIM) are class B carbapenemases that can be transmitted among different strains of the same bacterial species and among different bacterial species or genera (WHO, 2020b). The transmission of resistance can occur by transformation (bacteria take up DNA from the environment), transduction (DNA transfer between bacterial cells by bacteriophages), conjugation (plasmids harboring mobile elements), and the transfer of plasmids between bacterial cell via outer membrane vesicles. Thus, in a hospital, several bacterial species may have the same resistance determinants which were transferred by mobile genetic elements.
AMR, especially in Gram-negative bacteria, is complex, has a variety of underlying mechanisms and links to diseases in humans and other animals (WHO, 2017). The resistance of common bacteria, including P. aeruginosa and Enterobacteriaceae, is rendering treatments for common infections ineffective, worsening outcomes and increasing healthcare costs (WHO, 2020a). Global laboratory-based surveillance for AMR is now critical to understand the spread of multidrug resistant organisms as well as evaluate the impact of interventions designed to reduce the clinical and community burden of these dangerous pathogens.
Rapid, simple, and reliable identification of AMR is essential to ensure that antibiotic use is appropriate, and for surveillance in low- or middle-income countries. Carbapenem resistance is examined by calculating the minimum inhibitory concentration (MIC) using the disc diffusion test, gradient method, or synergy test (double-disc test), but these do not clarify the mechanism of drug resistances. Molecular assays are the standard tests for the identification of carbapenemase-encoding genes (Solanki et al., 2014).
Genotype-based methods (i.e., PCR, real-time PCR, and microarrays) can subgroup carbapenemases and monitor the transmission of resistance. Conventional PCR-based assays can detect β-lactamase genes but require well-equipped laboratories. However, the equipment required for conventional PCR or real-time PCR assays is expensive, and these techniques are complex, hampering their adoption by laboratories with limited experience in molecular testing, particularly in low- or middle-income countries (WHO, 2019).
The loop-mediated isothermal amplification (LAMP) assay overcomes the limitations of phenotyping and PCR methods (Mori and Notomi, 2019). This method uses a unique priming mechanism that yields specific DNA products more rapidly than PCR. It does not require expensive equipment or a sophisticated laboratory. LAMP is convenient in terms of point-of-care testing (POCT). For surveillance, it is appropriate for laboratories lacking experience with molecular testing. We developed LAMP assays for four β-lactamase genes (blaKPC, blaNDM-1, blaIMP-1 group, and blaVIM) and evaluated each using clinical strains isolated at diverse geographical locations (Kos et al., 2015). There are several previous reports mentioning the LAMP assay for the four β-lactamase genes identification (Moreno-Morales et al., 2020; Feng et al., 2021). While these reports used isolates from a limited number of regions, this is the first report to use clinical strains isolated at diverse geographical locations. In addition, phylogenetical analysis verified the extent of its efficacy. This is in stark contrast to previous reports that simply referred to effectiveness.
A total of 63 bacterial strains, 8 reference and 55 clinical were used to develop the LAMP assays. The reference strains harboring six β-lactamase genes (blaKPC, blaNDM-1, blaIMP, blaVIM, blaOXA-48, and blaGES) were three K. pneumoniae, one E. coli, three P. aeruginosa, and one Acinetobacter bereziniae strains; all were provided by AstraZeneca (Waltham, MA) (Table 1).
Genomic DNA was extracted using a Maxwell 16-Cell DNA Purification kit (Promega, Madison, WI). The concentration of purified genomic DNA was determined using a NanoDrop 1000 Spectrophotometer (Thermo Fisher Scientific Inc., Waltham, MA). The genomic DNA copy number was calculated based on genome size (5.4 Mbp for K. pneumoniae [Kp52.154, GenBank accession number: FO834906.1], 5.2 Mbp for E. coli [CFT073, AE014075.1], 6.5 Mbp for P. aeruginosa [PB369, CP025049.1], 4.5 Mbp for A. bereziniae [XH901, NZ_CP018259.1], and 4.0 Mbp for A. baumannii [ATCC19606, CP059040.1]).
The eight standard reference strains including seven β-lactamase genes (Table 1) were used to evaluate the specificity of the LAMP assay for each targeted β-lactamase gene. The specificity of each LAMP reactions was examined using 105 copies of reference genomic DNA per reaction. A serial 10- times dilution of reference genomic DNA was used to test the sensitivity of the LAMP assays in comparison to that of PCR.
Fifty-five clinical Gram-negative isolates harboring β-lactamase genes were used to evaluate the LAMP assays (Table 2). Among them, 47 clinical P. aeruginosa strains were randomly selected from 388 strains of known genotypes and phenotypes (Kos et al., 2015) isolated at diverse geographical locations (Colombia, India, Spain, France, Greece, Germany, Argentina, Croatia, China, Brazil, Mexico, Romania, and the Philippines) between 2003 and 2012. Originally, those isolates were obtained from the International Health Management Association (IHMA). Whole-genome sequences were analyzed on the HiSeq 2000 or MiSeq platform (Illumina, San Diego, CA, United States) (Kos et al., 2015). Susceptibility to meropenem was explored using frozen Trek-Sensititre custom plates (Thermo Fisher Scientific Inc.) following the 2012 guidelines of the Clinical and Laboratory Standards Institute (Clinical and Laboratory Standards Institute, 2012). The MICs for meropenem are listed in Table 2. Other eight clinical Gram-negative isolates including three A. baumannii, two E. coli, two K. pneumoniae, and one E. cloacae were obtained from AstraZeneca culture collection (Table 2).
To design primers for blaKPC, blaNDM, blaIMP, and blaVIM, we compared the sequences of variants using Clustal X v. 2.0 (Larkin et al., 2007) to identify consensus regions and to select the target region. The GenBank accession numbers and names of the blaKPC, blaNDM, blaIMP, and blaVIM sequences are listed in Supplementary Table 1. The alignments of the four target genes are shown in Supplementary Figure 1.
LAMP primers were designed using Primer Explorer v. 5 software according to the sequences of blaKPC (GenBank accession number NG_049253.1), blaNDM-1 (FN396876.1), blaIMP-1 group (GU831546.1), and blaVIM (GQ853417.1) (Supplementary Figure 2). Nucleotide 22 of the backward inner primer (BIP) for blaVIM was changed from G to C to increase the sensitivity and prevent primer dimer formation (Table 3).
Based on the primer sequences, we aligned primers’ binding sites of variants of each β-lactamase gene, also phylogenetic trees were conducted by the neighbor-joining method using MEGA v. 11 (Tamura et al., 2021) (Supplementary Figure 3). According to an in silico analysis (FastPCR (PrimerDigital, 2022)), variants in the blue square in Supplementary Figure 3 were expected to be detected by the LAMP assays.
The LAMP reaction mixture (25 μL) contained 1.6 μM FIP and BIP, 0.2 μM F3 and B3, 0.8 μM LF and LB, 8 U of Bst Polymerase (large fragment) (New England Biolabs, Ipswich, MA), 1.4 mM four deoxynucleoside triphosphates, 0.8 M betaine (Sigma, St. Louis, MO), 20 mM Tris-HCl (pH 8.8), 10 mM KCl, 10 mM (NH4)2SO4, 8 mM MgSO4, 0.1% (v/v) Tween 20, and template DNA (2 μL) (Takano et al., 2019). Reactions were incubated at 63-67°C for 60 min (Table 3) and heated at 80°C for 2 min to terminate the reaction.
The LAMP reaction was monitored by measuring turbidity in real time using Loopamp® Real-Time Turbidimeters (LA-500 and LA-200; Eiken Chemical Co., Tokyo, Japan) at a wavelength of 650 nm (OD650) at 6 s intervals. The reaction time was recorded when the turbidity level exceeded 0.1 in accordance with the manufacturer’s protocol. Amplified products were also evaluated visually. Amplified products were sequenced at the Akita Prefectural University Biotechnology Center using a BigDye® Terminator v. 3.1 Cycle Sequencing Kit and 3130xL Genetic Analyzer (Applied Biosystems, Foster City, CA). The F2 and B2 primers used to sequence the target regions are shown in Supplementary Table 2.
The PCR primers and conditions for the β-lactamases (Supplementary Table 3) were described previously (Senda et al., 1996; Monteiro et al., 2012). PCR assays were performed in a 25 μL reaction mixture containing 0.2 mM each deoxynucleoside triphosphate, 10 mM Tris-HCl (pH 8.0), 10 mM KCl, 2.5 mM MgCl2, 0.4 μM each primer, 2.5 U of Ex Taq DNA Polymerase (Takara, Shiga, Japan), and template DNA (2 μL). Reactions were performed on a SimpliAmp™ Thermal Cycler (Applied Biosystems). The PCR program was 5 min at 94°C; followed by 35 cycles of denaturation at 94°C for 90 s, annealing at 55°C for 30 s, and extension at 72°C; and a final extension at 72°C for 15 min, followed by storage at 4°C. The PCR products were subjected to agarose gel electrophoresis and visualized with Midori Green Advance (NIPPON Genetics, Tokyo, Japan).
To analyze the effects of biological substances on the established LAMP assays, the detection limits of the LAMP assays were determined using DNA-spiked clinical samples. Urine and blood specimens were collected from five healthy volunteers at Nihon University School of Medicine. The blood specimens were heparinized and stored at -80°C. Sputum specimens were obtained from seven patients at Ageo Central General Hospital and frozen at -80°C to inactivate bacteria. After approval by the Biosafety Committee of Nihon University, the specimens were handled using the risk group 2 protocol of the Laboratory Biosafety Manual of the WHO, Geneva, 2004. Urine specimens were boiled at 95°C for 5 min and centrifuged at 1500 rpm. DNA was extracted from blood and sputum samples using a Loopamp™ PURE DNA Extraction Kit (Eiken Chemical Co.) according to the manufacturer’s instructions. Purified β-lactamase DNA (blaKPC, ARC2945; blaNDM-1, ARC3600; blaIMP-1 group, ARC2780; and blaVIM, ARC3471) was added to the specimens and used to determine the detection limits of the PCR and LAMP assays.
The LAMP assays amplified the target sequences, as confirmed by turbidity in the reaction tube and by real-time turbidimetry (Table 1 and Figure 1). Of the eight strains including 7 β-lactamase genes, each assay detected only the target gene. In contrast, no other genes were not amplified in this assay (Table 1). The LAMP reactions reached the detection threshold (turbidity level of 0.1) within 35 min, whereas no turbidity rise was detected for 60 min in negative reaction. The sequences of the LAMP products were identical to those expected (Supplementary Figures 2, 4).
Figure 1 blaKPC-LAMP assay detecting Visual detection of a white precipitate in positive samples. The figure shows a positive (blaKPC-2) and a negative (non-blaKPC, blaNDM-1) sample, together with a negative control (neg.).
Serial 10-fold-diluted DNA samples (adjusted at 104, 103, 102, 10, and 1, and 0 genome copies per reaction in 25 μL) were amplified by LAMP and the results were compared to those of PCR. The detection limits of the LAMP assays were 102 copies per reaction for blaKPC, 102 copies for blaNDM-1, 102 copies for blaIMP-1, and 10 copies for blaVIM. The detection limits for PCR were 104 copies for blaKPC, 103 copies for blaNDM-1, 102 copies for blaIMP, and ten copies for blaVIM (Table 4). Therefore, the LAMP assays were up to 100-fold more analytically sensitive than PCR. Although the detection limits of the LAMP assays for blaIMP-1 and blaVIM were identical to those of PCR, the LAMP reaction was more rapid, and the results could be confirmed visually.
Table 4 Detection limits of the PCR and LAMP assays used to detect blaKPC, blaNDM-1, blaIMP-1 and blaVIM in DNA-spiked specimens.
The detection limit of the LAMP assays using clinical specimens was determined using DNA-spiked urine, sputum, and blood samples. The detection limit of the LAMP assays was 102 copies per reaction for blaKPC, 102 copies for blaNDM-1, 102 copies for blaIMP-1, and 10 copies for blaVIM in DNA-spiked urine, sputum, and blood specimens. These values were identical to those obtained using purified DNA as the template (Table 4).
The detection limit of PCR for blaKPC in DNA-spiked urine, sputum, and blood specimens was 104 copies per reaction, identical to that using purified DNA as the template. The detection limit of PCR for blaNDM-1 was 104 copies per reaction in DNA-spiked urine, sputum, and blood specimens, which was 10-fold lower than when purified DNA was used as the template. The detection limit of PCR for blaIMP in DNA-spiked urine and sputum specimens was 102 copies, identical to that using purified DNA as a template. However, the sensitivity decreased 100-fold (104 copies per reaction) for the DNA-spiked blood specimens compared to using purified DNA as the template. Finally, the detection limit of PCR for blaVIM in DNA-spiked urine and sputum specimens was 10-fold less sensitive (102 copies per reaction) and that in DNA-spiked blood specimens was 100-fold less sensitive (103 copies per reaction) compared to the use of purified DNA as the template (10 copies per reaction). The sensitivity of LAMP for DNA-spiked blood specimens was up to 100-fold higher than that of PCR.
The LAMP assays for four β-lactamases were validated using 55 clinical Gram-negative bacterial strains isolated at diverse geographical locations (Table 2). The LAMP assay for blaKPC specifically amplified the target blaKPC segments (blaKPC-2) and no other genotype. The LAMP assay for blaNDM-1 specifically amplified three isolates with the target gene blaNDM-1, and no other genotype. The LAMP assay for blaIMP-1 amplified two isolates with blaIMP-1 and blaIMP-4, but not three isolates with blaIMP-13, blaIMP-15, and blaIMP-18 (Table 2) or any other genotype. The LAMP assay for blaVIM amplified 11 isolates with blaVIM-1, blaVIM-2, blaVIM-4, blaVIM-5, and blaVIM-11, but not an isolate with blaVIM-7 (Table 2) or any other genotype. The results of the LAMP assays for blaKPC, blaNDM-1, blaIMP-1, and blaVIM were thus identical to those simulated in silico (blue squares in Supplementary Figure 3).
We developed LAMP assays for four β-lactamase genes (blaKPC, blaNDM-1, blaIMP-1 group, and blaVIM). Standard reference strains and 55 β-lactamase-carrying clinical isolates were correctly identified, although most Metallo β-lactamase genes have sequence variations and 47 clinical P. aeruginosa isolates were from diverse geographical locations. Theoretically, the capacity of detection will not change if the sequence of the genes is the same; however, the genome size of P. aeruginosa, co-existing drug-resistant genes, and the phenotype of the isolates are different depending on geographic distribution (Kos et al., 2015). Thus, the investigation using diverse geographic locations is important to assess the quality of the assay.
The results were in agreement with those of our in silico simulations. The sensitivity of the LAMP assays was comparable between purified DNA and DNA-spiked clinical specimens. The LAMP reactions were not inhibited by contaminants in DNA-spiked samples. By contrast, PCR assays for three β-lactamase genes (blaNDM-1, blaIMP-1 group, and blaVIM) are inhibited by the contaminants. The LAMP assays were up to 100-fold more sensitive than PCR. Because of their robustness to biological substances, the LAMP assays detected DNA added to blood specimens with up to 100-fold higher sensitivity than PCR.
PCR assays are affected by, for example, heparin (Satsangi et al., 1994), heme, leukocyte DNA, and IgG (Al-Soud et al., 2000; Al-Soud and Radstrom, 2001). Therefore, it is not appropriate to use a simple DNA extraction method such as the Loopamp™ PURE DNA Extraction Kit (Eiken Chemical Co.), which is a contamination-resistant kit that does not require a centrifuge or refrigerator. Because of their robustness to biological substances, the LAMP assays can be performed at the bedside or resource-limited settings. The LAMP assays will promote the control of β-lactam resistance because rapid and accurate POCT for drug resistance is essential and will facilitate research on AMR and the effectiveness of the One Health approach to limit the spread of resistance (WHO, 2017).
In this study, we struggled to detect sequence variations for each of the MBL genotypes. Most MBL genes have sequence variations. Indeed, 93 variants of blaKPC, 40 of blaNDM, 87 of blaIMP, and 77 variants of blaVIM have been reported (Supplementary Table 1). To select the target region, we compared the sequences of the four genotypes, and identified a consensus region of each genotype (Supplementary Figure 1). The 93 blaKPC variants do not differ markedly and all were expected to be detected by the LAMP assay (Supplementary Figures 1, 3). The 40 blaNDM variants also do not differ markedly and all were expected to be detected by the LAMP assay (Supplementary Figures 1, 3). blaIMP has 87 highly different variants, which prevented identification of a consensus region. Therefore, we focused on the blaIMP-1 group, which is main group among the variants of blaIMP (Supplementary Figures 1, 3). Among the 77 blaVIM variants, we identified a consensus region for 73; however, 4 variants (blaVIM-7, blaVIM-18, blaVIM-61, and blaVIM-69) were considerably different (Supplementary Figures 1, 3). Therefore, we focused on the 73 blaVIM variants as targets for the LAMP assays.
We designed LAMP primers for four target genes (nucleotides 141 to 359 of blaKPC, 76 to 287 of blaNDM-1, 463 to 676 of blaIMP-1, and 223 to 446 of blaVIM) (Supplementary Figure 2). The results agreed with those of in silico simulations (blue squares in Supplementary Figure 3). Using the LAMP primers for the blaIMP-1 group, blaIMP-1 and blaIMP-4 (blue square in Supplementary Figure 3) were detected but blaIMP-13, blaIMP-15, blaIMP-18 (without the blue square in Supplementary Figure 3) were not. The LAMP primers for blaVIM detected blaVIM-1, 2, 4, 5, and 11 (blue square in Supplementary Figure 3), but not blaVIM-7 (without the blue square in Supplementary Figure 3). The LAMP primers for blaKPC detected blaKPC-2 and blaKPC-3 (blue square in Supplementary Figure 3), and that for blaNDM-1 detected blaNDM-1 (blue square in Supplementary Figure 3).
Visual inspection of LAMP assay is sufficient to confirm positive results. For more complex diagnostic assays, such as arrays and whole-genome sequencing, complex analyses are needed to interpret the raw data. Indeed, LAMP-based diagnostic tests for extended-spectrum β-lactamases and carbapenemases are suitable for widespread use, including in low- or middle-income countries.
This study had a several limitations. The specificity of LAMP assays for blaIMP and blaVIM does not cover all of the genotypes. If MBL is suspected clinically but negative results are obtained, additional qualification should be considered, e.g., sequencing. Additional work using clinical specimens from patients is required.
In conclusion, we developed novel LAMP assays for four β-lactamase genes (blaKPC, blaNDM-1, blaIMP-1 group, and blaVIM). Further evaluation of these assays is required using additional clinical specimens. Low-complexity and low-cost tests may be suitable for most types of laboratories, whereas high-complexity and high-cost tests may be suitable only for established national reference laboratories with a sufficient budget. Laboratories with no prior experience in molecular testing may consider low-complexity, low-cost tests such as LAMP-based assays. Further development of additional LAMP primer sets for blaVIM and blaIMP is now ongoing.
The datasets presented in this study can be found in online repositories. The names of the repository/repositories and accession number(s) can be found in the article/Supplementary Material.
The studies involving human participants were reviewed and approved by the Ethics Committees of Ageo Central General Hospital (approval # 434) and the IRB of Nihon University School of Medicine (approval # 28-9-0). Using the IRB-approved protocol, seven patient sputum specimens (Ageo Central General Hospital) were collected in accordance with the recommendations of the Japan Society of Clinical Examination Medicine. This guidance enables access to specimens when it is difficult to obtain consent, the sample is anonymized, and if the IRB has approved the study protocol. The requirement for written consent was waived because specimens were anonymized samples discarded by the Hospital’s clinical laboratory. We also used urine and blood specimens from five healthy volunteers at Nihon University School of Medicine. The study protocol was reviewed and approved by the Institutional Review Board (IRB) of the Nihon University School of Medicine (# 28-9-0). Written informed consent was obtained from the five healthy volunteers. The patients/participants provided their written informed consent to participate in this study.
HG, RM, PK, SH, TH, DK, and MS contributed the conception of this study. EK, DK and MS designed the experiments. EK, JL, YY, DL, YB, TI, and MS performed the experiments. HG, RM, CT, SH and PK acquired the samples. EK, JL, JS, CT and MS analyzed the data. EK, JS, TI, DK, AN, TO, TH, and MS performed the phylogenetic and in silico analyses. EK, JS, MS, DK, and SH interpreted the data, drafted the manuscript. All authors contributed to the article and approved the submitted version.
DWK was supported by the grant NRF-2021R1A2C1010857 from National Research Foundation (NRF) of Korea and a grant of the Korea Health Technology R&D Project through the Korea Health Industry Development Institute (KHIDI), funded by the Ministry of Health & Welfare, Republic of Korea (grant number: HI19C0748) and Institute of Information & Communications Technology Planning & Evaluation (IITP) grant No.2020-0-01343 funded by Ministry of Science and ICT (MSIT) of Korea. EJK was supported by the grant NRF- 2020R1C1C1009992 from National Research Foundation (NRF) of Korea. MS was supported by Miyata Research Grant (A). MS and DWK were supported by Japan-Korea Basic Scientific Cooperation Program between JSPS and NRF, (grant number: JPJSBP120228812 and 2022K2A9A2A08000136). JS was supported by JSPS KAKENHI (grant number: JP22K16376). SH was supported by Nihon University Research Grant for 2022.
We are grateful to Dr. Kumasaka, Ageo Central General Hospital for his supports to this project.
HG was employed by Harbour Biomed. It did not influence to the study design, collection, analysis, interpretation of data, the writing of this article, or the decision to submit it for publication.
The remaining authors declare that the research was conducted in the absence of any commercial or financial relationships that could be construed as a potential conflict of interest.
All claims expressed in this article are solely those of the authors and do not necessarily represent those of their affiliated organizations, or those of the publisher, the editors and the reviewers. Any product that may be evaluated in this article, or claim that may be made by its manufacturer, is not guaranteed or endorsed by the publisher.
The Supplementary Material for this article can be found online at: https://www.frontiersin.org/articles/10.3389/fcimb.2022.1000445/full#supplementary-material
Al-Soud, W. A., Jonsson, L. J., Radstrom, P. (2000). Identification and characterization of immunoglobulin G in blood as a major inhibitor of diagnostic PCR. J. Clin. Microbiol. 38 (1), 345–350. doi: 10.1128/JCM.38.1.345-350.2000
Al-Soud, W. A., Radstrom, P. (2001). Purification and characterization of PCR-inhibitory components in blood cells. J. Clin. Microbiol. 39 (2), 485–493. doi: 10.1128/JCM.39.2.485-493.2001
Arumugham, V. B., Gujarathi, R., Cascella, M. (2022). Third generation cephalosporins, in StatPearls (Treasure Island (FL):StatPearls Publishing).
Chen, L., Mathema, B., Chavda, K. D., DeLeo, F. R., Bonomo, R. A., Kreiswirth, B. N. (2014). Carbapenemase-producing Klebsiella pneumoniae: molecular and genetic decoding. Trends Microbiol. 22 (12), 686–696. doi: 10.1016/j.tim.2014.09.003
Clinical and Laboratory Standards Institute (2012). Methods for dilution antimicrobial susceptibility tests for bacteria that grow aerobically, approved standard M07-A9 (Wayne, PA: Clinical and Laboratory Standards Institute).
Feng, W., Niu, S., Chang, Y., Jia, X., Huang, S., Yang, P. (2021). Design of rapid detection system for five major carbapenemase families (blaKPC, blaNDM, blaVIM, blaIMP and blaOXA-48-Like) by colorimetric loop-mediated isothermal amplification. Infect. Drug Resist. 14, 1865–1874. doi: 10.2147/IDR.S301757
Kos, V. N., Deraspe, M., McLaughlin, R. E., Whiteaker, J. D., Roy, P. H., Alm, R. A., et al. (2015). The resistome of pseudomonas aeruginosa in relationship to phenotypic susceptibility. Antimicrob. Agents Chemother. 59 (1), 427–436. doi: 10.1128/AAC.03954-14
Larkin, M. A., Blackshields, G., Brown, N. P., Chenna, R., McGettigan, P. A., McWilliam, H., et al. (2007). Clustal W and clustal X version 2.0. Bioinformatics 23 (21), 2947–2948. doi: 10.1093/bioinformatics/btm404
Laxminarayan, R., Matsoso, P., Pant, S., Brower, C., Rottingen, J. A., Klugman, K., et al. (2016). Access to effective antimicrobials: a worldwide challenge. Lancet 387 (10014), 168–175. doi: 10.1016/S0140-6736(15)00474-2
Monteiro, J., Widen, R. H., Pignatari, A. C., Kubasek, C., Silbert, S. (2012). Rapid detection of carbapenemase genes by multiplex real-time PCR. J. Antimicrob. Chemother. 67 (4), 906–909. doi: 10.1093/jac/dkr563
Moreno-Morales, J., Vergara, A., Kostyanev, T., Rodriguez-Bano, J., Goossens, H., Vila, J. (2020). Evaluation of a loop-mediated isothermal amplification assay to detect carbapenemases directly from bronchoalveolar lavage fluid spiked with acinetobacter spp. Front. Microbiol. 11, 597684. doi: 10.3389/fmicb.2020.597684
Mori, Y., Notomi, T. (2019). Loop-mediated isothermal amplification (LAMP): Expansion of its practical application as a tool to achieve universal health coverage. J. Infect. Chemother. 26 (1), 13–17. doi: 10.1016/j.jiac.2019.07.020
Palacios-Baena, Z. R., Giannella, M., Manissero, D., Rodriguez-Bano, J., Viale, P., Lopes, S., et al. (2021). Risk factors for carbapenem-resistant gram-negative bacterial infections: A systematic review. Clin. Microbiol. Infect. 27(2), 228–235. doi: 10.1016/j.cmi.2020.10.016
PrimerDigital. (2022) FastPCR is an integrated tool for PCR primers or probe design, in silico PCR, oligonucleotide assembly and analyses, alignment and repeat searching. Available at: https://primerdigital.com/fastpcr.html.
Satsangi, J., Jewell, D. P., Welsh, K., Bunce, M., Bell, J. I. (1994). Effect of heparin on polymerase chain reaction. Lancet 343 (8911), 1509–1510. doi: 10.1016/s0140-6736(94)92622-0
Senda, K., Arakawa, Y., Ichiyama, S., Nakashima, K., Ito, H., Ohsuka, S., et al. (1996). PCR detection of metallo-beta-lactamase gene (blaIMP) in gram-negative rods resistant to broad-spectrum beta-lactams. J. Clin. Microbiol. 34 (12), 2909–2913. doi: 10.1128/JCM.34.12.2909-2913.1996
Solanki, R., Vanjari, L., Subramanian, S., Aparna, B., Nagapriyanka, E., Lakshmi, V. (2014). Comparative evaluation of multiplex PCR and routine laboratory phenotypic methods for detection of carbapenemases among gram negative bacilli. J. Clin. Diagn. Res. 8 (12), DC23–DC26. doi: 10.7860/JCDR/2014/10794.5322
Takano, C., Seki, M., Kim, D. W., Gardner, H., McLaughlin, R. E., Kilgore, P. E., et al. (2019). Development of a novel loop-mediated isothermal amplification method to detect Guiana extended-spectrum (GES) beta-lactamase genes in pseudomonas aeruginosa. Front. Microbiol. 10. doi: 10.3389/fmicb.2019.00025
Tamura, K., Stecher, G., Kumar, S. (2021). MEGA11: Molecular evolutionary genetics analysis version 11. Mol. Biol. Evol. 38 (7), 3022–3027. doi: 10.1093/molbev/msab120
WHO. (2017). Global priority list of antibiotic-resistant bacteria to guid research, discovery, and development of new antibiotics. (Geneva: WHO).
WHO. (2019). Molecular methods for antimicrobial resistance (AMR) diagnostics to enhance the global antimicrobial resistance surveillance system. (Geneva: WHO).
WHO. (2020a). A financial model for an impact investment fund for the development of antibacterial treatments and diagnostics: a user guide. (Geneva: WHO).
Keywords: loop-mediated isothermal amplification, ß-lactamase gene, Gram-negative bacteria, blaKPC, blaNDM-1, blaIMP-1 group, blaVIM
Citation: Kim EJ, Lee J, Yoon Y, Lee D, Baek Y, Takano C, Sakai J, Iijima T, Kanamori D, Gardner H, McLaughlin RE, Kilgore PE, Nakamura A, Ogihara T, Hayakawa S, Hoshino T, Kim DW and Seki M (2023) Development of a novel loop-mediated isothermal amplification assay for ß-lactamase gene identification using clinical isolates of Gram-negative bacteria. Front. Cell. Infect. Microbiol. 12:1000445. doi: 10.3389/fcimb.2022.1000445
Received: 22 July 2022; Accepted: 12 December 2022;
Published: 12 January 2023.
Edited by:
Nahed Ismail, University of Illinois at Chicago, United StatesReviewed by:
Prasanth Manohar, Zhejiang University-University of Edinburgh Institute, ChinaCopyright © 2023 Kim, Lee, Yoon, Lee, Baek, Takano, Sakai, Iijima, Kanamori, Gardner, McLaughlin, Kilgore, Nakamura, Ogihara, Hayakawa, Hoshino, Kim and Seki. This is an open-access article distributed under the terms of the Creative Commons Attribution License (CC BY). The use, distribution or reproduction in other forums is permitted, provided the original author(s) and the copyright owner(s) are credited and that the original publication in this journal is cited, in accordance with accepted academic practice. No use, distribution or reproduction is permitted which does not comply with these terms.
*Correspondence: Mitsuko Seki, bWl0c3Vrby5zZWtpQGRlbnQubWVpa2FpLmFjLmpw; Dong Wook Kim, ZG9uZ3dvb2tAaGFueWFuZy5hYy5rcg==
Disclaimer: All claims expressed in this article are solely those of the authors and do not necessarily represent those of their affiliated organizations, or those of the publisher, the editors and the reviewers. Any product that may be evaluated in this article or claim that may be made by its manufacturer is not guaranteed or endorsed by the publisher.
Research integrity at Frontiers
Learn more about the work of our research integrity team to safeguard the quality of each article we publish.