- 1Department of Functional Food and Biotechnology, Jeonju University, Jeonju, South Korea
- 2Department of Agricultural Biotechnology and Research Institute of Agriculture and Life Science, Seoul National University, Seoul, South Korea
In this study, we investigated the relation of probiotic activity of Lacticaseibacillus rhamnosus strain GG (LGG) and expression of microRNA to immune response and longevity in Caenorhabditis elegans host model. First, we evaluated the survival rate of C. elegans due to LGG exposure and bacterial colonization in the intestine. Next, the expression of mRNA and miRNA was analyzed in C. elegans exposure to LGG for 24 h using microarray. After exposure to LGG to C. elegans, colonized LGG was observed in the intestines of C. elegans and induced to extend lifespan. Moreover, persistent LGG in the intestine significantly enhanced the resistance of C. elegans exposed to both pathogenic bacteria and prolonged the lifespan of C. elegans. Transcriptome analysis indicated that LGG affected the expression levels of genes related to the innate immune response and upregulated the abundance of genes in multiple pathways of C. elegans, including Wnt signaling, TGF-beta signaling and mitogen-activated protein kinase (MAPK) pathways. In addition, qRT-PCR analysis confirmed that the expression of antibacterial genes was increased by LGG. Moreover, as the expression of microRNA miR-34 and immune-related pathways increased by exposure to LGG, the lifespan of C. elegans increased. However, in the miR-34 mutant C. elegans, the lifespan by LGG did not increase, so it was determined that miR-34 indirectly affects immune-related pathways. There was no significant difference in the expression of PMK-1 for LGG exposure in miR-34 mutants, suggesting that miR-34 may regulate PMK-1. In conclusion, we suggest that exposure of LGG to C. elegans enhances lifespan and resistance to food-borne pathogen infection by stimulating miR-34 and indirectly promoting PMK-1 activity.
Introduction
Lacticaseibacillus rhamnosus strain GG (LGG) is one of the most widely studied probiotic strains for dairy foods including yogurt and cheese, and is generally recognized as safe (GRAS) by the Food and Drug Administration (Groele et al., 2017). LGG, originally isolated from human feces by Gorbach and Goldin (Segers and Lebeer, 2014), is an ideal probiotic that is resistant to stomach acid and bile, so it can survive the gastrointestinal tract, has the ability to consistently implant human intestinal epithelial cells and colonize the gut, produces an antimicrobial substance, has a rapid growth rate, and has beneficial effects on health (Doron et al., 2005). In addition, LGG has been applied to various disease states in clinical trials and has shown many benefits to the host, including improvements in diarrhea (Arvola et al., 1999; Basu et al., 2009), atopic diseases (Kalliomäki et al., 2003), anti-obesity (Luoto et al., 2010) and respiratory pathology (Davidson et al., 2011). Recently, LGG has been reported to have a beneficial effect on the host by restoring the intestinal flora imbalance caused by disease and maintaining intestinal homeostasis (Durack et al., 2017; Zhang et al., 2018; Han et al., 2019). Yan et al. reported that the LGG-derived protein p40 prevents and treats colon epithelial cell damage and inflammation in a colitis model (Yan et al., 2011). In addition, Lin et al. reported that LGG can protect against deoxynivalenol-induced bowel damage by regulating the intestinal microflora to promote the production of butyrate and thereby inhibit the IRE1a/XBP1 signaling pathway (Lin et al., 2020). In particular, LGG can reduce the risk of colon cancer by regulating the intestinal microflora and downregulating pro-inflammatory molecules (Gamallat et al., 2019).
Importantly, probiotic strains have been reported to be effective in gut health by regulating microRNA expression (Chen et al., 2017; Heydari et al., 2019). MicroRNAs (miRNAs) are small noncoding RNA molecules of approximately 20-22 nucleotides (Desvignes et al., 2019). miRNA controls gene expression via base complementarity between the seed region of miRNA and the 3’-untranslated region (UTP) of the target mRNA (Allegra et al., 2020). In many cells, miRNAs have been reported to regulate pro- and anti-inflammatory responses to maintain homeostasis of the host (Behrouzi et al., 2020) and are also involved in innate and adaptive immunity (Lindsay, 2008; Garo and Murugaiyan, 2016). In addition, commensal bacteria in the gut are involved in host health and disease, and miRNAs have been shown to modulate human-microbial interactions in the host gut (Behrouzi et al., 2020). Unfortunately, when the literature was reviewed, there had been no previous studies in which LGG regulates miRNA expression in the host. Caenorhabditis elegans is known as a suitable experimental model for host microbiota studies (Cabreiro and Gems, 2013). In particular, the innate immune system, the first line of defense against microbial infections, is the first and phylogenetically oldest line of defense in all multicellular organisms (Isailovic et al., 2015). Research on C. elegans, a genetically tractable model, has provided valuable insight into the functional aspects of antiaging and innate immunity and has greatly contributed to our understanding of the multiple genetic pathways that determine longevity (Ikeda et al., 2007; Irazoqui et al., 2010; Park et al., 2018). The mechanisms of microbial etiology, host defense and the role of probiotics in C. elegans have previously been reviewed (Mylonakis and Aballay, 2005; Kim and Mylonakis, 2012; Pukkila-Worley and Ausubel, 2012; Balla and Troemel, 2013; Komura et al., 2013; Park et al., 2018). Recent studies have reported that miRNAs play an important role in this mechanism (Dai et al., 2015; Isik et al., 2016; Xiong et al., 2016). In particular, studies using C. elegans as a mechanism related to miRNAs have been reported (Zhou et al., 2019; Li et al., 2020). The purpose of this study was to demonstrate the longevity and resistance of C. elegans exposed to LGG to pathogenic bacterial infections. We also aimed to demonstrate that LGG can have beneficial effects on a host by regulating immunity-related genes or miRNAs in the host.
Materials and Methods
Bacterial Strains and C. elegans Growth Conditions
L. rhamnosus strain GG (ATCC 53103), Salmonella Typhimurium SL1344, Escherichia coli OP50, Staphylococcus aureus RN6390 and Enterococcus faecalis MMH594 were grown at 37°C using De Man, Rogosa, and Sharpe (MRS; Difco, Detroit, MI) broth for LGG, Luria-Bertani (LB; Difco) medium for S. Typhimurium SL1344 and E. coli OP50, tryptic soy broth (TSB; Difco) for S. aureus RN6390, and brain heart infusion (BHI; Difco) for E. faecalis MMH594. For long-term storage, cultures were maintained at −80°C with 15% glycerol. All strains were sub-cultured twice prior to experimental analysis. To prepare live bacterial lawns for C. elegans feeding, after the bacteria were grown in each growth medium and washed five times with M9 medium, 500 μl of each type of cell (2.0 × 109 CFU/ml) was spread on Nematode Growth Medium (NGM) plates and dried at room temperature for 3 h. Each live bacterial lawn was stored at 4°C and used within a week.
C. elegans N2 Bristol wild-type, CF512 fer-15(b26)II;fem-1(hc17)IV strains were used in this study. C. elegans were routinely maintained in NGM agar inoculated with OP50, an internationally established feed using standard techniques (Park et al., 2018). Eggs were extracted from sodium hypochlorite-sodium hydroxide solution (1.0%–1.3% w/v sodium hypochlorite, 500 mM sodium hydroxide) from egg-bearing C. elegans to obtain L1 C. elegans and then transferred to NGM plates seeded with OP50. Synchronized L1 C. elegans were grown at 25°C to obtain L4/young adult C. elegans.
C. elegans Longevity Assay and Killing Assay
C. elegans longevity was assessed as previously described method (Kim and Mylonakis, 2012; Park et al., 2018). Briefly, 100 μl aliquots of concentrated bacteria (2.0 × 109 CFU/ml) were plated on 35-mm NGM agar plates, and L4/young adult C. elegans of N2 or mutant C. elegans were individually transferred with a platinum wire onto OP50 or LGG plates and maintained at 25°C. The numbers of live C. elegans were measured daily for viability using a dissection microscope (Olympus SZ40, Olympus, Japan). Live C. elegans were transferred to fresh plates daily during the progeny production period and, thereafter, were transferred every 3 days. C. elegans was determined to be dead if it was not moved by gentle touching with a platinum wire pick. In addition, for C. elegans killing analysis, L4/young adult C. elegans were placed on plates exposed to LGG or OP50 for 24 h at 25°C. C. elegans pre-exposed to LGG or OP50 were then transferred to NGM plates plated with pathogenic bacteria (S. Typhimurium SL1344, S. aureus RN6390 and E. faecalis MMH594) and examined at 24 h intervals until all the C. elegans died at 25°C.
Bacterial Attachment Assay in C. elegans Intestinal Tract
The bacterial attachment assay was performed according to an established method (Kim and Mylonakis, 2012; Park et al., 2018) with some modifications. LGG attachment assay in the C. elegans intestinal tract collected C. elegans after 24 h of exposure to LGG lawn prepared on NGM agar plates. Analysis of E. faecalis attachment in C. elegans intestinal tracts was exposed to LGG lawns for 24 h and then transferred to E. faecalis lawns. C. elegans were then collected on 0, 1, 3 and 5 days. Collected C. elegans were washed five times with M9 buffer and transferred to BHI plates containing kanamycin (100 μg/ml) and streptomycin (100 μg/ml). C. elegans were washed with 5 μL drops of gentamicin solution (25 μg/ml) for 5 min to remove attached bacteria from the body of the C. elegans. After removing surface bacteria, C. elegans were washed five times with M9 buffer, and C. elegans were placed in new sterile tubes containing M9 buffer with 1% Triton X-100 and mechanically disrupted by using a mortar and pestle (Kontes, Vineland, NJ, USA). C. elegans lysates were serially diluted in M9 buffer, which was plated on BHI agar containing 80 μg/ml kanamycin for E. faecalis or modified MRS (pH 5.0) agar for LGG. Plates were incubated at 37°C for 24 h. Colonies were quantified and used to calculate the number of bacteria per C. elegans.
Transmission Electron Microscopy (TEM) in the C. elegans Intestinal Tract
For microscopic observation, C. elegans were quickly placed on 2% agarose pads with drops of 10 mM NaN3 in M9 butter. C. elegans were observed under a microscope (Nikon Eclipse Ts2, Nikon, Japan). Simultaneously, bacterial attachment was visualized by TEM (Hitachi H-7650, Hitachi High Technologies, Japan) to determine their persistence on the C. elegans intestinal epithelium. C. elegans were plated on 60-mm NGM plates seeded with LGG or control plates seeded with OP50. Observations for each sample were evaluated for at least 10 cross sections, and representative images were selected.
Transcriptome Analysis for mRNA and miRNA Expression
Microarray experiments were performed to compare the expression of mRNA and miRNA with OP50 when C. elegans was exposed to LGG for 24 h. C. elegans were collected through M9 buffer after 24 h exposure to LGG or OP50, washed three times, and pelleted by centrifugation for RNA isolation. After centrifugation, total RNA was isolated from C. elegans using QlAzol (QIAGEN, Germany) and RNeasy mini kit (Qiagen, Valencia, CA) according to the manufacturer’s instructions. RNA was quantified using a 2100 Bioanalyzer (Agilent Technologies, Santa Clara, CA, USA) with an RNA 6000 nano kit. Targeted microarray analysis for mRNA and miRNA was performed by a commercial company (eBiogen, Seoul, Korea) using a C. elegans GE Microarray 4x44K (Agilent) and Affymetrix GeneChip_miRNA arrays (Affymetrix), respectively. A gene was considered differentially expressed when the p value for comparing chips was lower than 0.05 (to assure that the change in gene expression was statistically significant and that false positives arose less than 5%). The differential gene expression data have been deposited in the NCBI Gene Expression Omnibus (http://www.ncbi.nlm.nih.gov/geo/) and are accessible through Accession No. GSE190701.
Cluster analysis was based on protein functional enrichment by DAVID Bioinformatics Resources 6.8. Cluster analysis based on functional enrichment was used to study potential connections and differences in specific functions (KEGG pathways and protein domains). Functional classification information and corresponding P-values were collected, and functional classes were screened in one or more protein clusters. The Z-transformed dataset was analyzed by hierarchical clustering (Euclidean distance, complete connected clustering). The clustering association was visualized using heatmap.2 in the R package (v3.6.3).
qRT-PCR Validation
Total RNA from C. elegans was quickly isolated following the protocol of TRIzol reagent (Invitrogen, USA) and purified using the RNeasy minikit (Qiagen, Germany). After RNA isolation, 50 ng of total RNA was used for qRT-PCR using the SuperScript III Platinum SYBR green one-step qRT-PCR kit (Invitrogen, USA). qRT-PCR was performed using Applied Biosystems StepOnePlus Real-Time PCR Systems (Applied Biosystems, USA). Primer validation and qRT-PCR were performed as described previously (Kim and Mylonakis, 2012). Relative expression levels were calculated using the 2−ΔΔCT threshold cycle method (Livak and Schmittgen, 2001), and the control gene snb-1 was used to normalize the gene expression data (Irazoqui et al., 2008).
Immunoblot Analysis
Immunoblot analysis was performed according to an established method (Jeong et al., 2018) with some modifications. Briefly, C. elegans was exposed to LGG and OP50 for 24 h. After treatment, the C. elegans were washed with M9 buffer and homogenized in cold PBS supplemented with protease and phosphatase inhibitor cocktail (Thermo Scientific, USA). The residues in the lysates were removed by centrifugation at 800 × g for 10 min at 4°C, and the protein content was measured. Approximately 30 µg of protein was loaded onto 12% reducing SDS-acrylamide gels and electrophoresed and transferred to a polyvinylidene fluoride (PVDF) membrane with a 0.2 μm pore size (Bio-Rad Lab, USA). After transfer, the membranes were blocked with 5% skimmed milk in Tris-buffered saline containing 0.1% Tween 20 (TBST) for 2 h. After blocking in 5% skimmed milk in TBST, the membranes were incubated for 1 h with primary antibodies [phospho-PMK-1 (Cell Signaling) or β-actin (Santa Cruz)] diluted in TBST with 5% skimmed milk at RT, followed by incubation with the secondary antibodies anti-rabbit IgG (Thermo Fisher) or anti-mouse IgG (Santa Cruz) at RT for 1 h. HRP signals were visualized using chemiluminescence (ECL kit, Amersham) and an image analyzer (C-DiGit® Blot Scanner, Li-COR).
Statistical Analysis
C. elegans survival analysis was performed using the Kaplan–Meier method, and the significance of differences between survival curves was determined using the log-rank test (STATA6; STATA, College Station, TX, USA). Student’s t-test was performed to determine significant differences for CFU counting for determining bacterial abundance. The data shown are presented as the means ± SEM of three or more independent experiments, and the differences were considered statistically significant at p<0.05 using Student’s t-test.
Results and Discussion
LGG Is Not Harmful to the C. elegans Host and Colonizes the Intestine of the Host
We performed toxicity studies to evaluate whether LGG, a well-known probiotic (Capurso, 2019), is harmful to C. elegans hosts. To this end, lifespan analyses were performed by monitoring the LGG-fed C. elegans, starting at the L4 larval stage, compared to the control C. elegans exposed to the nonpathogenic prey E. coli OP50 or probiotic strain LGG (Figure 1A). We found no significant difference in the lifespan of C. elegans exposed to OP50 and LGG in C. elegans (Figure 1B). Hence, we concluded that LGG was not toxic to C. elegans.
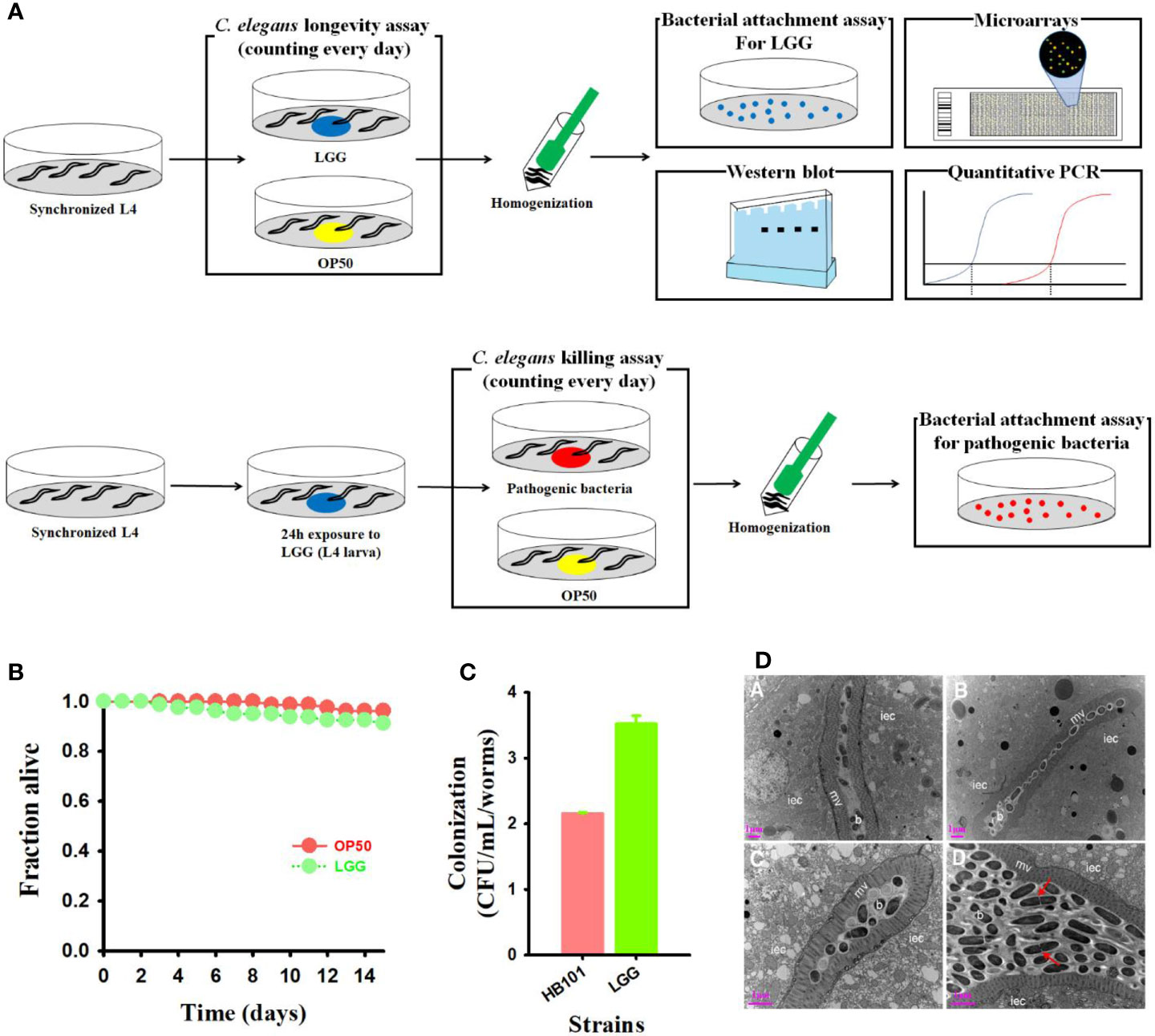
Figure 1 Treatment of probiotics LGG to C. elegans and high adhesion in the intestine. (A) Schematic diagram of experimental design (Details are in materials and methods). (B) Monitoring the viability of C. elegans fer-15;fem-1 C. elegans (n=40 per well) exposed to LGG or OP50 (normal feed). (C) Bacterial counts (colony forming unit) in C. elegans intestine and (D) TEM images of transverse mid-body sections of C. elegans fed with OP50 (a, b) or LGG (c, d). Higher magnification showing microvilli, intestinal epithelial cells, bacterial colonization in the intestinal lumen iec, intestinal epithelial cell; mv, microvilli; b, bacterial cells (OP50 or LGG). Scale bar = 1 μm.
LGG contains two pilus gene clusters, SpaCBA and SpaFED, which play a beneficial role in the host due to its intestinal attachment ability (Guerin et al., 2016). Intestinal cells of C. elegans are similar in structure to human intestinal cells and are often used to determine the defense mechanisms of the intestines of the host (Park et al., 2018). Therefore, we assessed adhesion ability by exposing LGG to C. elegans to confirm the attachment of LGG. LGG showed high colonization ability and an approximately 1.6-fold higher CFU count than OP50 (Figure 1C). Similar to the plate number, the intestine of the C. elegans was completely colonized with LGG after feeding LGG from the C. elegans, and LGG was not digested as a cell mass surrounded by an extracellular matrix. (Figure 1D). In contrast, the negative control OP50-fed C. elegans showed no expanded intestinal lumen or intestinal epithelial cells filled with bacterial cell masses (data not shown). These results suggested that LGG could be attached to the C. elegans intestine, as determined by plate counting results and TEM images.
LGG Enhanced Resistance to Pathogenic Infections in C. elegans
In recent studies, C. elegans has been used as a laboratory animal model for screening for potential probiotic strains (Park et al., 2014; Zhou et al., 2014; Oh et al., 2018). In particular, C. elegans has a short life cycle and an inducible immune response resembling a significant part of the mammalian innate immune system, which is advantageous for studies on aging and immunity (Zhou et al., 2014; Park et al., 2018). We previously reported that probiotic strains, including Lactobacillus and Bacillus, improve lifespan by regulating the immune response of C. elegans (Kim and Mylonakis, 2012; Park et al., 2015; Park et al., 2018). We examined the immune response of C. elegans when exposed to LGG. Agar-based solid killing assays were performed with modifications according to a previously described method (Kim and Mylonakis, 2012; Park et al., 2018) to explore whether LGG augments the C. elegans defense response against pathogenic bacteria. C. elegans were transferred to LGG-plated plates for 24 h and then exposed to S. aureus RN6390 or S. Typhimurium SL1344 pathogenic bacteria, using a solid killing assay with the fer-15;fem-1 C. elegans. C. elegans exposed to LGG for 24 h clearly exhibited less susceptibility to pathogenic bacterial infection than the control strain OP50 (P = 0.0102 for S. aureus, P < 0.0001 for S. Typhimurium, respectively, compared to OP50) (Figures 2A, B).
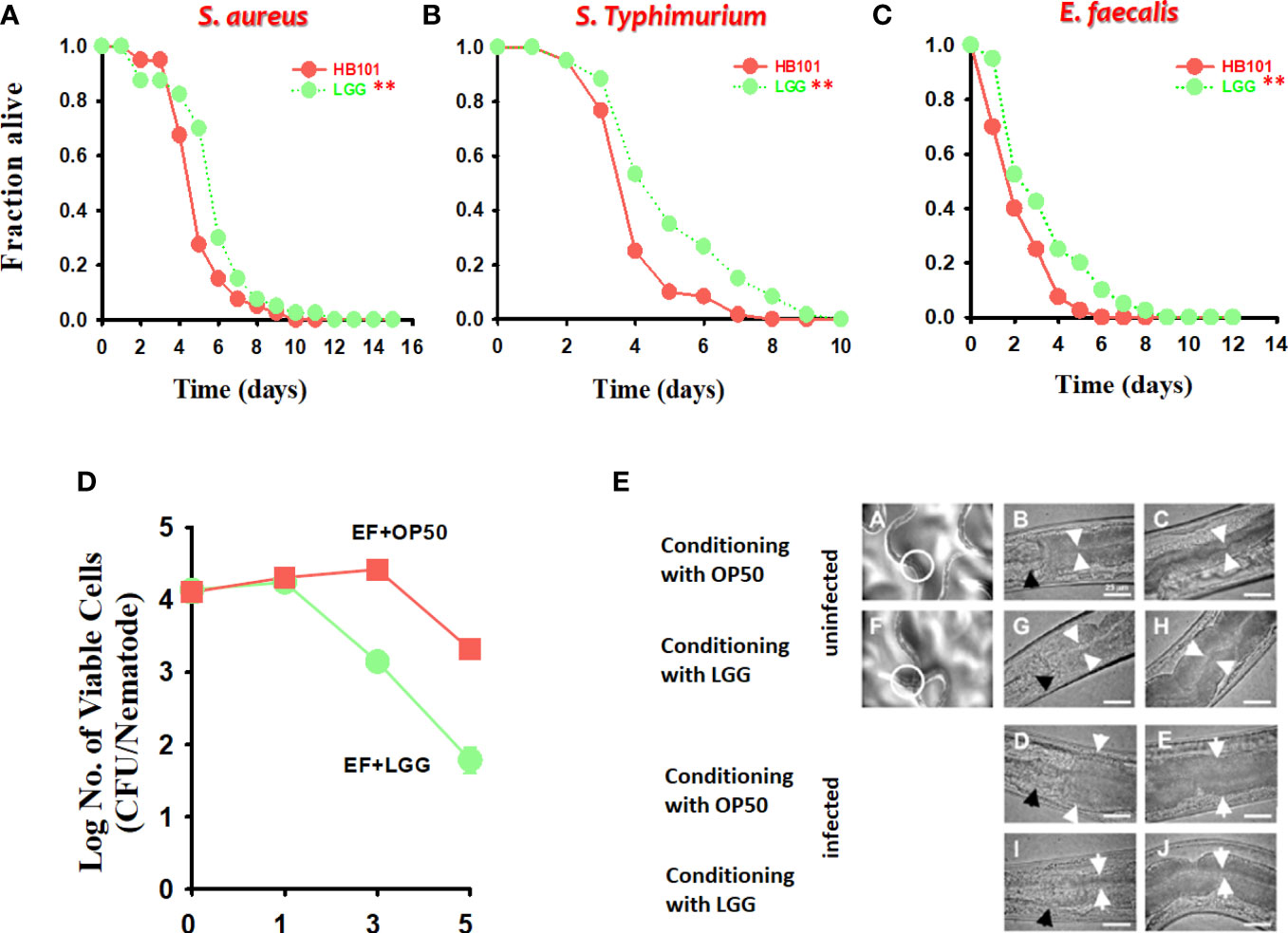
Figure 2 The effect of preconditioned LGG on the survival of C. elegans infected with both gram-positive and gram-negative pathogens. C. elegans killing assays of strains fer-15;fem-1 infected with (A) S. aureus RN6390 or (B) S. Typhimurium SL1344 and fer-15;ferm-1 and glp-4 infected with (C) E. faecalis MMH594 after exposure to LGG for 24 h. The significance of differences between survival curves (Kaplan–Meier method) was determined using the log-rank test. *p < 0.05; **p < 0.01. (D, E) Bacterial burden (CFU/nematode) of E. faecalis in fer-15;fem-1 nematodes after pre-exposure to OP50 or LGG. The white circle in A (exposed to OP50) and F (exposed to LGG) is the digestive tract and this area is for viewing at a higher magnification. White arrows indicate the borders of the intestinal lumen, and black arrows indicate the grinder organ. Scale bar, 25 μm.
Next, we evaluated whether LGG would colonize the C. elegans intestine, decreasing the number of pathogenic bacteria and prolonging C. elegans survival. To investigate this possibility, we evaluated the presence of cells by infecting C. elegans with E. faecalis, a pathogenic bacterium that colonizes the intestinal tract of the C. elegans to form a persistent lethal infection (Kim and Mylonakis, 2012) after 24 h of C. elegans exposure to LGG. First, we employed C. elegans fer-15;fem-1 (Murphy et al., 2003) mutants and found that C. elegans exposed to LGG prolonged survival during E. faecalis infection (P = 0.0200 with fer-15;fem-1 for LGG compared to C. elegans pre-exposed to OP50) (Figure 2C). Next, to measure the burden of E. faecalis in C. elegans, the C. elegans were exposed to E. faecalis infection after exposure to LGG and OP50 for 24 h. To remove surface bacteria, extensive washing was performed on BHI plates containing gentamicin as described previously (Kim and Mylonakis, 2012). In C. elegans pre-exposed to LGG or OP50, the number of E. faecalis cells in the C. elegans intestine was approximately 1.0 × 104 CFU/C. elegans on days 0 and 1 after 24 h exposure to E. faecalis, and there was no difference between the two groups. However, in the case of C. elegans pre-exposed to LGG, the number of E. faecalis cells in the C. elegans intestine began to decrease from 3 days, and after approximately 5 days, the number of cells was confirmed to be approximately 8.0 × 101 CFU/C. elegans. In the C. elegans pre-exposed to OP50, the number of E. faecalis cells in the C. elegans intestine was found to decrease from 5 days and colonize approximately 3.0 × 103 CFU/C. elegans on day 5 (Figure 2D). Similar to the CFU results, in microscopic studies, C. elegans exposed to LGG showed a thin intestinal lumen after infection with E. faecalis, whereas the intestinal lumen of C. elegans pre-exposed to OP50 was distended by E. faecalis cells (Figure 2E). Consistent with our findings, LGG had a beneficial effect on the lifespan of C. elegans, colonizing the intestines of C. elegans (Zanni et al., 2015). Taken together, our results showed that LGG colonized the C. elegans intestine, which significantly inhibited the pathogenic bacteria in the small intestine of the C. elegans when the pathogen was infected, thereby increasing survival.
LGG Regulates Specific Gene Transcriptions
We determined the mechanism by which LGG is associated with the immunomodulation of C. elegans. Based on our findings that pre-exposure to LGG increases survival in C. elegans for pathogenic bacterial infections, we postulated that when pre-exposed to LGG, specific immune factors would be regulated in pathogenic bacterial infections of C. elegans. Based on previous studies (Kim and Mylonakis, 2012), we selected 14 genes from C. elegans, including asp-1, C15C8.3 (encodes aspartyl proteases), cpr-1, cpr-2, cpr-4 and cpr-5 (encodes a cysteine protease), clec-52, clec-60, clec-71 (encodes C-type lectins), thn-2 (thaumatin/PR-5), lys-5 (encodes a lysozyme), fmo-2 (encodes a flavin-containing monooxygenase), ilys-3 (encodes an invertebrate lysozyme), and F53A9.8 (encodes a short His-rich protein), which are more than 4-fold increased by pathogenic bacteria. Additionally, we selected six genes related to stress in C. elegans (Hsu et al., 2003; Wang et al., 2010). Quantitative real-time PCR (qRT-PCR) was used to find that almost all selected genes in the C. elegans exposed to LGG were upregulated by 3.0-fold or more by LGG. Specifically, qRT-PCR results indicated that asp-1 (5.7 ± 1.4-fold), C15C8.3 (20.3 ± 4.8-fold), cpr-1 (7.0 ± 2.1-fold), cpr-4 (5.4 ± 0.94-fold), cpr-5 (20.1 ± 3.7-fold), lys-5 (6.1 ± 1.4-fold), clec-60 (10.8 ± 4.2-fold), and hsp-12.6 (5.0 ± 1.0-fold) transcription was induced dramatically by exposure to LGG (Figure 3). In contrast, four pathogenic bacteria-specific genes (thn-2, clec-52, ilys-3, F53A9.8) and five common stress-related genes (sod-3, sod-5, mtl-1, old-1 and gst-10) were not affected by LGG exposure. These results are consistent with our results of the killing assay in which LGG exposure stimulated the resistance of C. elegans to infection by pathogenic bacteria (Figure 2). Our results are similar to studies showing that C. elegans survival was increased by the upregulation of immune-related genes when C. elegans exposed to Lactobacillus strains were infected with pathogenic bacteria (Kim and Mylonakis, 2012; Park et al., 2018). In particular, our group reported that antimicrobial genes, including C15C8.3, cpr-1, cpr-5, lys-5, and clec-60, were associated with the TIR-1, PMK-1, and BAR-1 signaling pathways (Kim and Mylonakis, 2012). Similar to previous studies (Kim and Mylonakis, 2012), C15C8.3, cpr-1, cpr-5, lys-5, and clec-60 were also upregulated 6 times or more in this study. These results indicated that conditioning with LGG specifically stimulated the transcription of host defense genes associated with response to pathogen bacteria, thereby increasing lifespans.
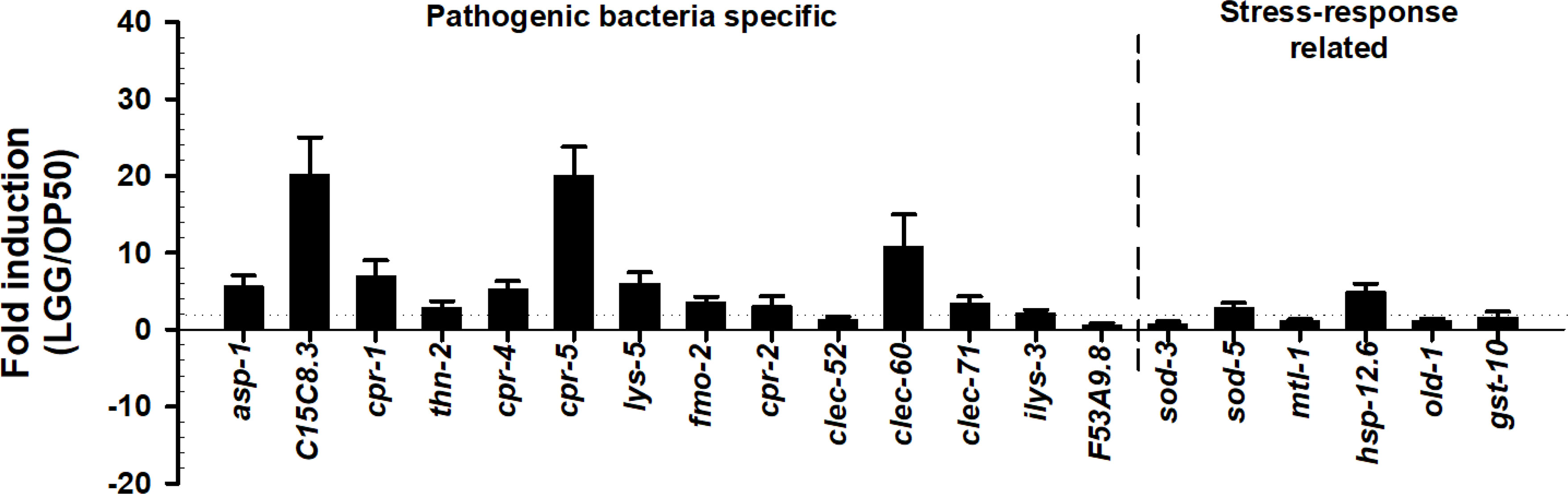
Figure 3 The alteration of the transcription of immune and stress related genes. Expression levels (LGG/OP50, normalized fold changes assessed by qRT-PCR analysis) of fourteen pathogenic bacteria specific immune response (asp-1, C15C8.3, cpr-1, thn-2, cpr-4, lys-5, fmo-2, cpr-2, clec-52, clec-60, clec-71, ilys-3, F53A9.8) and six stress response related genes (sod-3, sod-5, mtl-1, hsp-12.6, old-1, gst-10) in C. elegans. The dotted line shows 2-fold changes in LGG-induced gene expression compared to that of OP50-induced genes.
Expression Profiling Analysis for mRNA and miRNA of C. elegans Exposed to LGG
We also used a microarray to identify changes in mRNA and miRNA expression in LGG-exposed C. elegans. Exposure to LGG for 24 h caused significant changes in transcript profiles in C. elegans. As a result, 261 downregulated and 1059 upregulated differentially expressed genes were screened out, while only 25 upregulated and 4 downregulated differentially expressed miRNAs had >2.0-fold changes compared with the control groups. For an in-depth understanding of the genome-wide distribution of C. elegans exposed to LGG, a comprehensive gene ontology (GO) enrichment analysis was performed. There were 21 upregulated and 8 downregulated GO terms belonging to biological processes (Figures 4A, B); 3 upregulated and 4 downregulated GO terms belonging to cellular components (Figures 4C, D); and 17 upregulated and 12 downregulated GO terms belonging to molecular functions (Figures 4E, F). Similar to the results of qRT-PCR, from the biological process-related GO enrichment analysis (Figure 3), the significantly upregulated GO terms against C. elegans immunity in LGG-exposed C. elegans included defense response, innate immune response, and defense response to gram-negative bacteria. Previously, other studies have reported that lactobacilli, including LGG, have positive effects on life and pathogenic infections by stimulating innate immune responses (Pinto et al., 2009; Kim and Mylonakis, 2012; Poupet et al., 2020). Additionally, the protein domain enrichment analyses in mRNA increased by 22 protein domains, including “F-box”, “cytochrome P450”, “C-type lectin” and “UDP-glucoronosyl/UDP-glucosyl transferase”, associated with immunity (Figure 5A) (Simonsen et al., 2012; Shimizu et al., 2021). The F-box protein targets virulent and pathogenic bacterial or viral proteins, contributing to its breakdown, and has been speculated to function as part of the C. elegans innate immune system (Thomas, 2006). C-type lectins have been reported to be involved in serum glycoprotein homeostasis, pathogen detection, and initiation of an immune response (Mayer et al., 2017). UDP-glucuronyl transferase (UGT) and cytochrome P450 are involved in chemical detoxification and innate immune responses (Simonsen et al., 2012; Tan et al., 2020). Tan et al. reported that C-type lectin, UDP-glucuronyl transferase, and F-box A protein were downregulated when infected with pathogenic E. coli, and they were upregulated in probiotic treatment (Tan et al., 2020). Therefore, exposure to LGG can improve the innate immune response of the C. elegans while prolonging its lifespan.
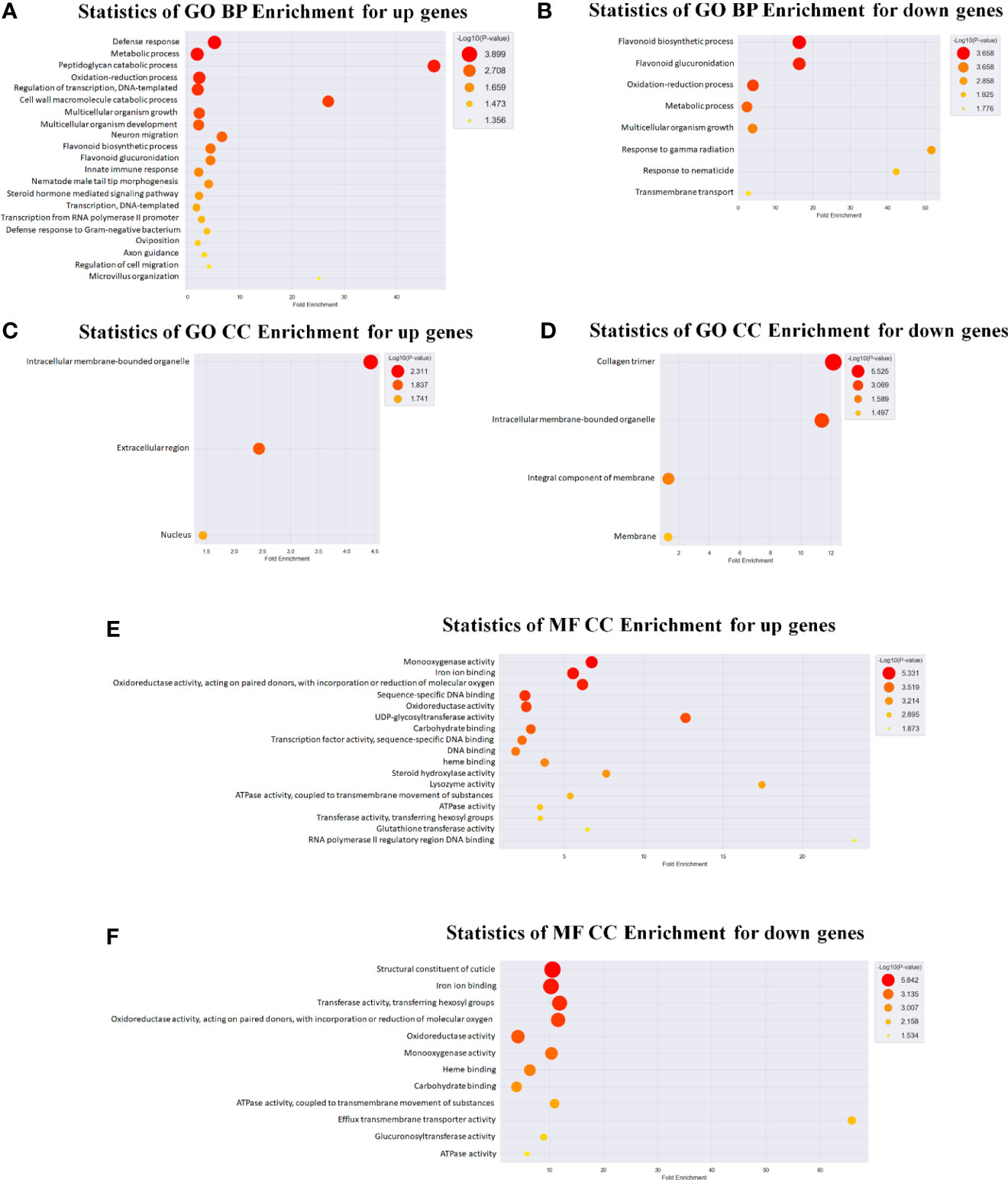
Figure 4 Gene ontology (GO) analysis in C. elegans exposed to LGG. GO analysis of differentially expressed genes was also performed. GO analysis was performed using the genes whose expression levels were changed by ≥3 folds compared with the control levels. Genes were classified based on DAVID (https://david.ncifcrf.gov/) and Medline databases (http://www.ncbi.nlm.nih.gov/). Data mining and graphic visualization were performed using ExDEGA (E-Biogen, Inc., Seoul, Korea). Dot plot shows the upregulated and downregulated genes GO terms (FDR <0.05) sorted by the most significant. The size and color of the dot shows the pathway enrichment significance. (A, B) Genes were classified into different hierarchical categories based on biological process, (C, D) cellular component and (E, F) molecular function.
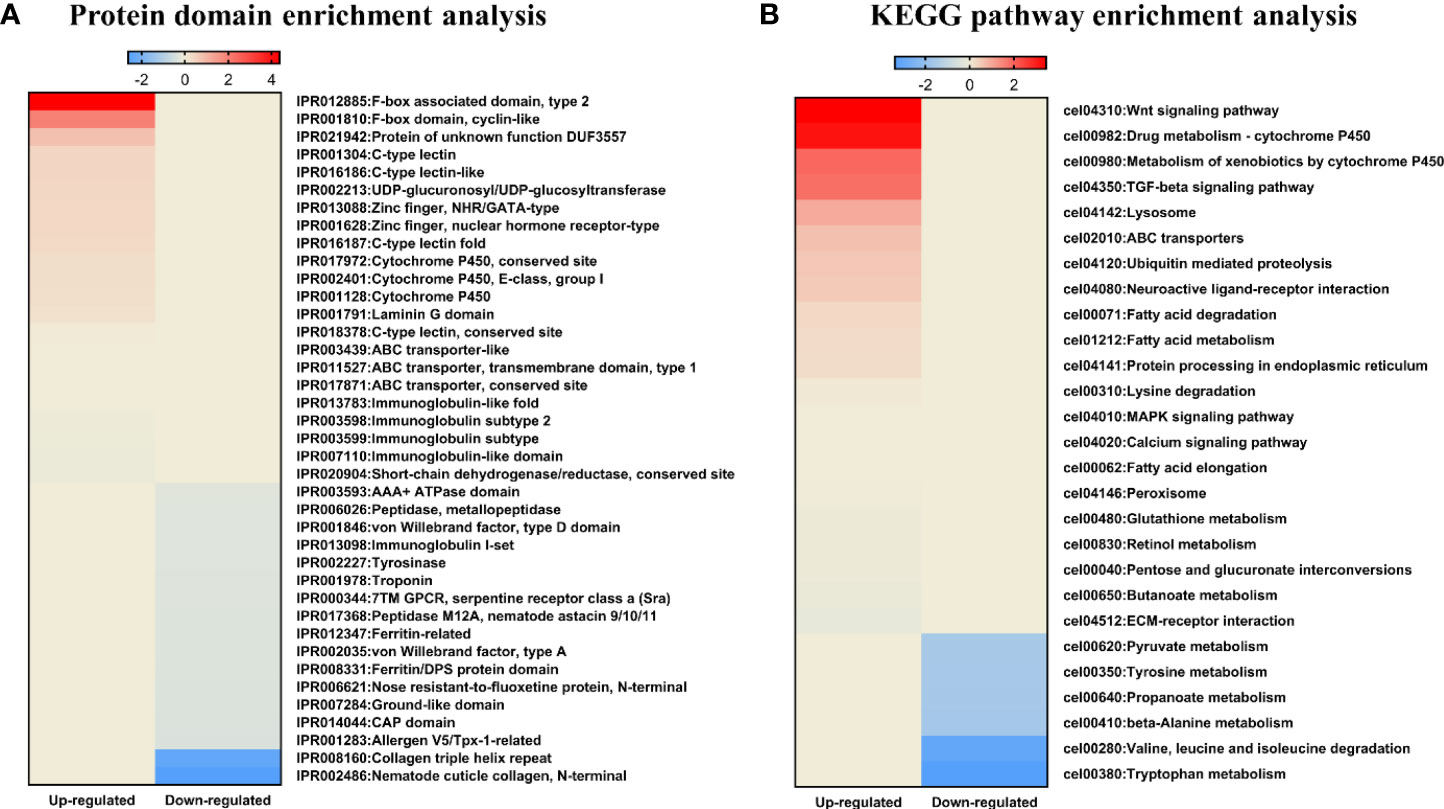
Figure 5 KEGG pathway and protein domain enrichment analyses in C. elegans exposed to LGG. Heatmap depicting abundance of selected protein domain and KEGG pathways. Different colors represent different expression level as determined by Fisher’s exact test p values (− log10). Red color represents up-regulated expression and blue color represents down-regulated expression. Each row denotes the categories of the protein domain and metabolic pathway. (A) Protein domain enrichment results of upregulated and downregulated proteins. (B) KEGG pathway enrichment results for upregulated and downregulated pathways.
In addition, we used DAVID (version 6.8) (Harvald et al., 2017) for mRNAs that changed after exposure to LGG to identify the enriched KEGG (Kyoto Encyclopedia of Genes and Genomes) pathways within each cluster (Figure 5B). Among the enriched KEGG pathways, we identified several immune response pathways, including the MAPK signaling pathway, Wnt signaling pathway and TGF-beta signaling pathway. The MAPK pathway is the innate immune system, the first line of defense against environmental microbial infections, and is evolutionarily conserved between vertebrates and invertebrates (Sun et al., 2016). Other studies found that the innate immune response of C. elegans exposed to Lactobacillus spp. were mediated by the MAPK signaling pathway (Kim and Mylonakis, 2012; Park et al., 2018). In another study, lactoferrin uptake was reported to modulate several genes that stimulate the immune function of C. elegans through induction of the TGF-β and Wnt signaling pathways and encode molecular effectors involved in the innate immune response (Nicholas and Hodgkin, 2004; Martorell et al., 2017). TGF-β pathway function is associated with protection against pathogenic bacteria, axonal pathfinding, body size/male tail development, and dauer formation (Patterson and Padgett, 2000). The Wnt signaling pathway is formed by highly conserved secreted signaling molecules that regulate cell-cell interactions, cell proliferation and differentiation during embryogenesis and affect the development of the central nervous system in vertebrates (Kim et al., 2013; Rosso and Inestrosa, 2013). Therefore, exposure to LGG was found to be related to various immune responses of C. elegans, thereby affecting the protective effect against pathogenic bacteria and the increase in lifespan.
Next, we investigated on the miRNAs target prediction using TargetScan (http://www.targetscan.org/). Since miRNA regulates gene expression through degradation or translation inhibition of target mRNA (Bushati and Cohen, 2007), we finally investigated mRNA whose expression correlates negatively with miRNA expression. Therefore, we identified 20 predicted target genes that were downregulated in 25 upregulated miRNAs and 1 target gene that was upregulated in 4 downregulated miRNAs (Table 1). In particular, we identified 3 miRNAs (cel-miR-796, cel-miR-34-3p, cel-miR-239a-5p) that regulate more than 4 target genes. Additionally, Liu et al. reported that loss of miR-34 accelerates aging and brain damage in Drosophila (Liu et al., 2012). These results suggest that miR-34 may play a crucial role in regulating the immune response of C. elegans by indirectly regulating immune-related genes instead of directly.
LGG Prolongs the Lifespans by Increasing miR-34 Expression in C. elegans
Based on our microarray results showing that LGG regulates miR-34 in C. elegans and prolongs its life, we revalidated the results using qRT-PCR and loss-of-function miR-34 C. elegans mutant. We analyzed the TargetScan database (Krek et al., 2005) for predicting target sequences of miR-34. The putative miR-34 targets include collagen genes (col-101), chondroitin proteoglycan (cpg-9), and gelsolin-like protein 1 (gsnl-1). The expression of miR-34 was increased in C. elegans exposed to LGG, and the putative target genes of miR-34, col-101, cpg-9 and gsnl-1 were significantly downregulated by LGG (Figure 6A). In addition, in N2 wild-type C. elegans exposed to LGG, survival in pathogenic bacteria (S. aureus) was prolonged, but exposure to LGG in C. elegans with deleted miR-34 did not affect survival (Figure 6B). In the aging assay, survival was extended in N2 wild C. elegans exposed to LGG, but exposure to LGG did not affect survival in the mir-34 mutant strain (Figure 6C). In particular, aging assays after exposure to LGG were performed using mutations that deleted the pmk-1 gene, which has been reported to play a role in regulating longevity and innate immunity genes. As a result, the pmk-1 mutant showed a shortened lifespan similar to the miR-34 mutant (Figure 6D). Next, we determined the level of phosphorylated PMK-1 using a specific antibody that recognizes the phosphorylated form of PMK-1 to determine whether LGG affects the activity of PMK-1. While the levels of phosphorylation of PMK-1 were critically upregulated by LGG in N2 wild C. elegans, this indicates that phosphorylation levels were not regulated by exposure to LGG in miR-34 mutant C. elegans (Figure 6E). We found that LGG exposure affects miRNAs in C. elegans, resulting in enhanced immunity and longevity. miR-34 was associated with lifespan and the onset of age-related diseases in model organisms, but the direction and mechanism underlying its effects are still unclear (Pinto et al., 2018). Previous studies have shown that miR-34 significantly prolongs life in removed mutations (Pinto et al., 2018), but other studies have reported findings that either did not affect survival (De Lencastre et al., 2010) or even the reverse, prolonged life (Liu et al., 2012). We found that when C. elegans was exposed to LGG, it upregulated miR-34 and affected lifespan (Figures 6A–C). However, mutations with miR-34 removed did not affect lifespan even when exposed to LGG (Figures 6B–D). Importantly, LGG has been shown to affect the PMK-1 pathway involved in the innate immunity of C. elegans (Figure 6E) (Park et al., 2018). Our group has previously identified that Lactobacillus strains enhance susceptibility to infection of pathogenic bacteria of C. elegans via the PMK-1 pathway (Kim and Mylonakis, 2012; Park et al., 2018). Zhou et al. also reported that Lacticaseibacillu zeae regulates C. elegans signaling through the p38 MAPK pathway to control the production of antimicrobial peptides and defense molecules to combat ETEC infection (Zhou et al., 2018). Similarly, our results show that LGG effectively stimulates the PMK-1 signaling pathway in C. elegans thereby combating pathogenic bacteria. Xiong et al. reported that miR-34 is related to innate immunity (Xiong et al., 2016). Similarly, our study supports that miR-34 affects PMK-1 as there is no significant change in PMK-1 expression in miR-34 mutant C. elegans by LGG exposure. Thus, we found that miR-34 stimulated by LGG exposure of C. elegans activates the PMK-1 pathway by downregulating a candidate gene (Figure 6F).
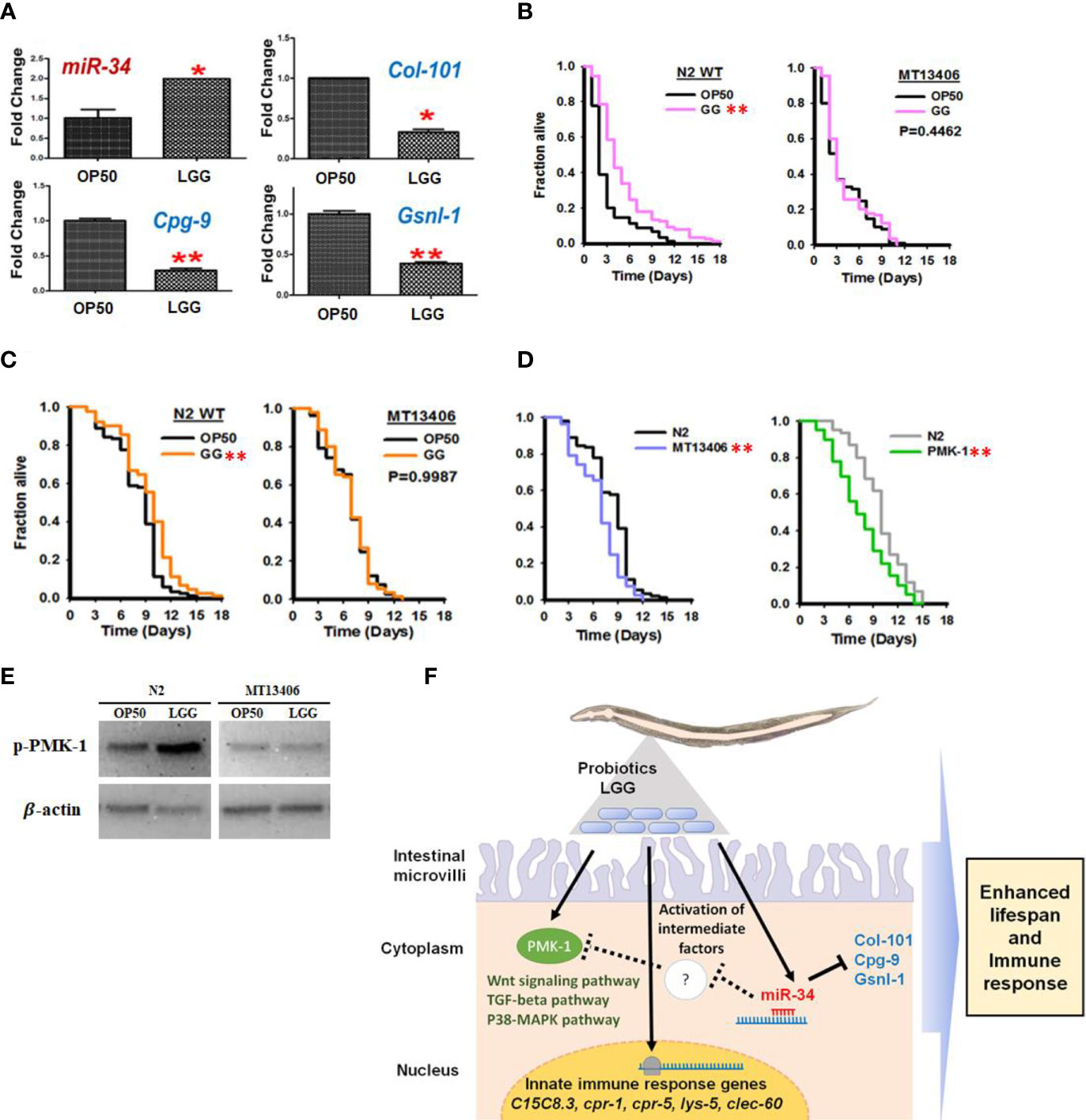
Figure 6 Stimulation of miR-34 and PMK-1 pathways in C. elegans by ingestion of LGG. (A) C. elegans exposed to LGG upregulated miR-34 and inhibited its putative target genes col-101, cpg-9 and gsnl-1 which was selected among down regulated genes from Affymetrix GeneChip_miRNA array data. (B) The killing assay of N2 wild type and miR-34 deletion mutant (MT13406) exposed to OP50 or LGG against to pathogenic bacteria (S. aureus). (C) The longevity assay of N2 wild type and miR-34 mutant (MT13406) exposed to OP50 or LGG (D) The longevity assay of pmk-1 deletion mutant (km25)IV exposed to OP50 or LGG. The values are *P<0.05; **P<0.01 compared with OP50. (E) Protein expression of phosphorylated PMK-1 in LGG or OP50 exposed to miR-34 mutant / N2 WT assessed by Western blotting. β-actin was used as loading control. (F) Potential mechanism of LGG exposure and their functions in regulating innate immunity in C. elegans through stimulating miR-34 and PMK-1. Intermediate factors including may exist between miR-34 activation and PMK-1 pathway.
Conclusions
In this study, we have demonstrated that LGG has a beneficial effect on C. elegans lifespan and pathogenic bacterial infections. We confirmed that LGG protects against pathogen infection and prolongs lifespan by enhancing MAPK signaling pathway, Wnt signaling pathway and TGF-β signaling pathway of C. elegans. Our study also confirmed that antimicrobial genes were significantly increased by LGG. In addition, it was confirmed that the expression of miR-34 was increased due to LGG, and they could potentially activate the PMK-1. Taken together, our results confirm another potential of LGG probiotics for prevention of aging and metabolic diseases in functional foods. In addition, our study enhances the immune-modulated miRNA repertoire in host and provides new insights into the interaction between innate immunity. We concluded that LGG stimulated miRNAs in C. elegans to enhance resistance to pathogen infection and prolong lifespan.
Data Availability Statement
The original contributions presented in the study are included in the article/Supplementary Material. Further inquiries can be directed to the corresponding authors.
Author Contributions
BY, YK, and SO conceived and designed the experiments. BY, SR, MK, JL, JY, YK, and SO performed the experiments. BY, SR, MK, JL, JY, YK, and SO analyzed the data. BY, YK, and SO wrote the paper. All authors contributed to the article and approved the submitted version.
Funding
This research was supported by a National Research Foundation of Korea Grant, funded by the Korean government (MEST) (NRF-2018R1D1A3B07050304 to SO and 2021R1A2C3011051 to YK).
Conflict of Interest
The authors declare that the research was conducted in the absence of any commercial or financial relationships that could be construed as a potential conflict of interest.
Publisher’s Note
All claims expressed in this article are solely those of the authors and do not necessarily represent those of their affiliated organizations, or those of the publisher, the editors and the reviewers. Any product that may be evaluated in this article, or claim that may be made by its manufacturer, is not guaranteed or endorsed by the publisher.
Supplementary Material
The Supplementary Material for this article can be found online at: https://www.frontiersin.org/articles/10.3389/fcimb.2021.819328/full#supplementary-material
References
Allegra, A., Musolino, C., Tonacci, A., Pioggia, G., Gangemi, S. (2020). Interactions Between the MicroRNAs and Microbiota in Cancer Development: Roles and Therapeutic Opportunities. Cancers 12, 805. doi: 10.3390/cancers12040805
Arvola, T., Laiho, K., Torkkeli, S., Mykkänen, H., Salminen, S., Maunula, L., et al. (1999). Prophylactic Lactobacillus GGreduces Antibiotic-Associated Diarrhea in Children With Respiratory Infections: A Randomized Study. Pediatrics 104, e64. doi: 10.1542/peds.104.5.e64
Balla, K. M., Troemel, E. R. (2013). Caenorhabditis elegans as a Model for Intracellular Pathogen Infection. Cell. Microbiol. 15, 1313–1322. doi: 10.1111/cmi.12152
Basu, S., Paul, D. K., Ganguly, S., Chatterjee, M., Chandra, P. K. (2009). Efficacy of High-Dose Lactobacillus rhamnosus GG in Controlling Acute Watery Diarrhea in Indian Children: A Randomized Controlled Trial. J. Clin. Gastroenterol. 43, 208–213. doi: 10.1097/MCG.0b013e31815a5780
Behrouzi, A., Ashrafian, F., Mazaheri, H., Lari, A., Nouri, M., Rad, F. R., et al. (2020). The Importance of Interaction Between MicroRNAs and Gut Microbiota in Several Pathways. Microb. Pathogen. 144, 104200. doi: 10.1016/j.micpath.2020.104200
Bushati, N., Cohen, S. M. (2007). microRNA Functions. Annu. Rev. Cell Dev. Biol. 23, 175–205. doi: 10.1146/annurev.cellbio.23.090506.123406
Cabreiro, F., Gems, D. (2013). Worms Need Microbes Too: Microbiota, Health and Aging in Caenorhabditis Elegans. EMBO Mol. Med. 5, 1300–1310. doi: 10.1002/emmm.201100972
Capurso, L. (2019). Thirty Years of Lactobacillus rhamnosus GG: A Review. J. Clin. Gastroenterol. 53, S1–S41. doi: 10.1097/MCG.0000000000001170
Chen, Q., Tong, C., Ma, S., Zhou, L., Zhao, L., Zhao, X. (2017). Involvement of microRNAs in Probiotics-Induced Reduction of the Cecal Inflammation by Salmonella Typhimurium. Front. Immunol. 8, 704. doi: 10.3389/fimmu.2017.00704
Dai, L. L., Gao, J. X., Zou, C. G., Ma, Y. C., Zhang, K. Q. (2015). Mir-233 Modulates the Unfolded Protein Response in C. elegans During Pseudomonas Aeruginosa Infection. PLoS Pathog. 11, e1004606. doi: 10.1371/journal.ppat.1004606
Davidson, L. E., Fiorino, A.-M., Snydman, D. R., Hibberd, P. L. (2011). Lactobacillus GG as an Immune Adjuvant for Live-Attenuated Influenza Vaccine in Healthy Adults: A Randomized Double-Blind Placebo-Controlled Trial. Eur. J. Clin. Nutr. 65, 501–507. doi: 10.1038/ejcn.2010.289
De Lencastre, A., Pincus, Z., Zhou, K., Kato, M., Lee, S. S., Slack, F. J. (2010). MicroRNAs Both Promote and Antagonize Longevity in C. elegans. Curr. Biol. 20, 2159–2168. doi: 10.1016/j.cub.2010.11.015
Desvignes, T., Batzel, P., Sydes, J., Eames, B. F., Postlethwait, J. H. (2019). miRNA Analysis With Prost! Reveals Evolutionary Conservation of Organ-Enriched Expression and Post-Transcriptional Modifications in Three-Spined Stickleback and Zebrafish. Sci. Rep. 9, 1–15. doi: 10.1038/s41598-019-40361-8
Doron, S., Snydman, D. R., Gorbach, S. L. (2005). Lactobacillus GG: Bacteriology and Clinical Applications. Gastroenterol. Clin. North Am. 34, 483–498. doi: 10.1016/j.gtc.2005.05.011
Durack, J., Kimes, N. E., Lin, D., Mckean, M., Rauch, M., Cabana, M. D., et al. (2017). Early-Life Lactobacillus rhamnosus GG Supplementation of High-Risk for Asthma Infants Reprograms Gut Microbiota Development and Promotes Regulatory T-Cells. J. Allergy Clin. Immunol. 139, AB15. doi: 10.1016/j.jaci.2016.12.103
Gamallat, Y., Ren, X., Walana, W., Meyiah, A., Xinxiu, R., Zhu, Y., et al. (2019). Probiotic Lactobacillus Rhamnosus Modulates the Gut Microbiome Composition Attenuates Preneoplastic Colorectal Aberrant Crypt Foci. J. Funct. Foods 53, 146–156. doi: 10.1016/j.jff.2018.12.018
Garo, L. P., Murugaiyan, G. (2016). Contribution of MicroRNAs to Autoimmune Diseases. Cell. Mol. Life Sci. 73, 2041–2051. doi: 10.1007/s00018-016-2167-4
Groele, L., Szajewska, H., Szypowska, A. (2017). Effects of Lactobacillus rhamnosus GG and Bifidobacterium lactis Bb12 on Beta-Cell Function in Children With Newly Diagnosed Type 1 Diabetes: Protocol of a Randomised Controlled Trial. BMJ Open 7, e017178. doi: 10.1136/bmjopen-2017-017178
Guerin, J., Bacharouche, J., Burgain, J., Lebeer, S., Francius, G., Borges, F., et al. (2016). Pili of Lactobacillus rhamnosus GG Mediate Interaction With β-Lactoglobulin. Food Hydrocoll. 58, 35–41. doi: 10.1016/j.foodhyd.2016.02.016
Han, X., Lee, A., Huang, S., Gao, J., Spence, J. R., Owyang, C. (2019). Lactobacillus rhamnosus GG Prevents Epithelial Barrier Dysfunction Induced by Interferon-Gamma and Fecal Supernatants From Irritable Bowel Syndrome Patients in Human Intestinal Enteroids and Colonoids. Gut Microbes 10, 59–76. doi: 10.1080/19490976.2018.1479625
Harvald, E. B., Sprenger, R. R., Dall, K. B., Ejsing, C. S., Nielsen, R., Mandrup, S., et al. (2017). Multi-Omics Analyses of Starvation Responses Reveal a Central Role for Lipoprotein Metabolism in Acute Starvation Survival in C. elegans. Cell Syst. 538-52, e34. doi: 10.1016/j.cels.2017.06.004
Heydari, Z., Rahaie, M., Alizadeh, A. M., Agah, S., Khalighfard, S., Bahmani, S. (2019). Effects of Lactobacillus Acidophilus and Bifidobacterium Bifidum Probiotics on the Expression of microRNAs 135b, 26b, 18a and 155, and Their Involving Genes in Mice Colon Cancer. Probiotics Antimicrob. Proteins 11, 1155–1162. doi: 10.1007/s12602-018-9478-8
Hsu, A.-L., Murphy, C. T., Kenyon, C. (2003). Regulation of Aging and Age-Related Disease by DAF-16 and Heat-Shock Factor. Science 300, 1142–1145. doi: 10.1126/science.1083701
Ikeda, T., Yasui, C., Hoshino, K., Arikawa, K., Nishikawa, Y. (2007). Influence of Lactic Acid Bacteria on Longevity of Caenorhabditis elegans and Host Defense Against Salmonella enterica Serovar Enteritidis. Appl. Environ. Microbiol. 73, 6404–6409. doi: 10.1128/AEM.00704-07
Irazoqui, J. E., Ng, A., Xavier, R. J., Ausubel, F. M. (2008). Role for β-Catenin and HOX Transcription Factors in Caenorhabditis elegans and Mammalian Host Epithelial-Pathogen Interactions. Proc. Natl. Acad. Sci. U. S. A. 105, 17469–17474. doi: 10.1073/pnas.0809527105
Irazoqui, J. E., Urbach, J. M., Ausubel, F. M. (2010). Evolution of Host Innate Defence: Insights From Caenorhabditis elegans and Primitive Invertebrates. Nat. Rev. Immunol. 10, 47–58. doi: 10.1038/nri2689
Isailovic, N., Daigo, K., Mantovani, A., Selmi, C. (2015). Interleukin-17 and Innate Immunity in Infections and Chronic Inflammation. J. Autoimmun. 60, 1–11. doi: 10.1016/j.jaut.2015.04.006
Isik, M., Blackwell, T. K., Berezikov, E. (2016). MicroRNA Mir-34 Provides Robustness to Environmental Stress Response via the DAF-16 Network in C. elegans. Sci. Rep. 6, 36766. doi: 10.1038/srep36766
Jeong, D. E., Lee, Y., Lee, S. V. (2018). Western Blot Analysis of C. Elegans Proteins. Methods Mol. Biol. 1742, 213–225. doi: 10.1007/978-1-4939-7665-2_19
Kalliomäki, M., Salminen, S., Poussa, T., Arvilommi, H., Isolauri, E. (2003). Probiotics and Prevention of Atopic Disease: 4-Year Follow-Up of a Randomised Placebo-Controlled Trial. Lancet 361, 1869–1871. doi: 10.1016/S0140-6736(03)13490-3
Kim, W., Kim, M., Jho, E.-H. (2013). Wnt/β-Catenin Signalling: From Plasma Membrane to Nucleus. Biochem. J. 450, 9–21. doi: 10.1042/BJ20121284
Kim, Y., Mylonakis, E. (2012). Caenorhabditis elegans Immune Conditioning With the Probiotic Bacterium Lactobacillus acidophilus Strain NCFM Enhances Gram-Positive Immune Responses. Infect. Immun. 80, 2500–2508. doi: 10.1128/IAI.06350-11
Komura, T., Ikeda, T., Yasui, C., Saeki, S., Nishikawa, Y. (2013). Mechanism Underlying Prolongevity Induced by Bifidobacteria in Caenorhabditis elegans. Biogerontology 14, 73–87. doi: 10.1007/s10522-012-9411-6
Krek, A., Grün, D., Poy, M. N., Wolf, R., Rosenberg, L., Epstein, E. J., et al. (2005). Combinatorial microRNA Target Predictions. Nat. Genet. 37, 495–500. doi: 10.1038/ng1536
Lindsay, M. A. (2008). microRNAs and the Immune Response. Trends Immunol. 29, 343–351. doi: 10.1016/j.it.2008.04.004
Lin, R., Sun, Y., Mu, P., Zheng, T., Mu, H., Deng, F., et al. (2020). Lactobacillus Rhamnosus GG Supplementation Modulates the Gut Microbiota to Promote Butyrate Production, Protecting Against Deoxynivalenol Exposure in Nude Mice. Biochem. Pharmacol. 175, 113868. doi: 10.1016/j.bcp.2020.113868
Liu, N., Landreh, M., Cao, K., Abe, M., Hendriks, G.-J., Kennerdell, J. R., et al. (2012). The microRNA miR-34 Modulates Ageing and Neurodegeneration in. Drosophila Nat. 482, 519–523. doi: 10.1038/nature10810
Livak, K. J., Schmittgen, T. D. (2001). Analysis of Relative Gene Expression Data Using Real-Time Quantitative PCR and the 2– ΔΔCT Method. Methods 25, 402–408. doi: 10.1006/meth.2001.1262
Li, D., Yuan, Y., Wang, D. (2020). Regulation of Response to Nanopolystyrene by Intestinal microRNA Mir-35 in Nematode Caenorhabditis Elegans. Sci. Total Environ. 139677. doi: 10.1016/j.scitotenv.2020.139677
Luoto, R., Kalliomäki, M., Laitinen, K., Isolauri, E. (2010). The Impact of Perinatal Probiotic Intervention on the Development of Overweight and Obesity: Follow-Up Study From Birth to 10 Years. Int. J. Obes. 34, 1531–1537. doi: 10.1038/ijo.2010.50
Martorell, P., Llopis, S., Gonzalez, N., Ramón, D., Serrano, G., Torrens, A., et al. (2017). A Nutritional Supplement Containing Lactoferrin Stimulates the Immune System, Extends Lifespan, and Reduces Amyloid β Peptide Toxicity in Caenorhabditis Elegans. Food Sci. Nutr. 5, 255–265. doi: 10.1002/fsn3.388
Mayer, S., Raulf, M.-K., Lepenies, B. (2017). C-Type Lectins: Their Network and Roles in Pathogen Recognition and Immunity. Histochem. Cell Biol. 147, 223–237. doi: 10.1007/s00418-016-1523-7
Murphy, C. T., Mccarroll, S. A., Bargmann, C. I., Fraser, A., Kamath, R. S., Ahringer, J., et al. (2003). Genes That Act Downstream of DAF-16 to Influence the Lifespan of Caenorhabditis elegans. Nature 424, 277–283. doi: 10.1038/nature01789
Mylonakis, E., Aballay, A. (2005). Worms and Flies as Genetically Tractable Animal Models to Study Host-Pathogen Interactions. Infect. Immun. 73, 3833–3841. doi: 10.1128/IAI.73.7.3833-3841.2005
Nicholas, H. R., Hodgkin, J. (2004). Responses to Infection and Possible Recognition Strategies in the Innate Immune System of Caenorhabditis Elegans. Mol. Immunol. 41, 479–493. doi: 10.1016/j.molimm.2004.03.037
Oh, A., Daliri, E. B.-M., Oh, D. H. (2018). Screening for Potential Probiotic Bacteria From Korean Fermented Soybean Paste: In Vitro and Caenorhabditis elegans Model Testing. LWT 88, 132–138. doi: 10.1016/j.lwt.2017.10.007
Park, M. R., Oh, S., Son, S. J., Park, D.-J., Oh, S., Kim, S. H., et al. (2015). Bacillus licheniformis Isolated From Traditional Korean Food Resources Enhances the Longevity of Caenorhabditis Elegans Through Serotonin Signaling. J. Agr. Food Chem. 63, 10227–10233. doi: 10.1021/acs.jafc.5b03730
Park, M. R., Ryu, S., Maburutse, B. E., Oh, N. S., Kim, S. H., Oh, S., et al. (2018). Probiotic Lactobacillus fermentum Strain JDFM216 Stimulates the Longevity and Immune Response of Caenorhabditis elegans Through a Nuclear Hormone Receptor. Sci. Rep. 8, 7441. doi: 10.1038/s41598-018-25333-8
Park, M., Yun, H., Son, S., Oh, S., Kim, Y. (2014). Development of a Direct In Vivo Screening Model to Identify Potential Probiotic Bacteria Using Caenorhabditis elegans. J. Dairy Sci. 97, 6828–6834. doi: 10.3168/jds.2014-8561
Patterson, G. I., Padgett, R. W. (2000). Tgfβ-Related Pathways: Roles in Caenorhabditis Elegans Development. Trends Genet. 16, 27–33. doi: 10.1016/S0168-9525(99)01916-2
Pinto, M. G. V., Gómez, M. R., Seifert, S., Watzl, B., Holzapfel, W. H., Franz, C. M. (2009). Lactobacilli Stimulate the Innate Immune Response and Modulate the TLR Expression of HT29 Intestinal Epithelial Cells In Vitro. Int. J. Food Microbiol. 133, 86–93. doi: 10.1016/j.ijfoodmicro.2009.05.013
Pinto, S., Sato, V. N., De-Souza, E. A., Ferraz, R. C., Camara, H., Pinca, A. P. F., et al. (2018). Enoxacin Extends Lifespan of C. elegans by Inhibiting miR-34-5p and Promoting Mitohormesis. Redox Biol. 18, 84–92. doi: 10.1016/j.redox.2018.06.006
Poupet, C., Chassard, C., Nivoliez, A., Bornes, S. (2020). Caenorhabditis elegans, a Host to Investigate the Probiotic Properties of Beneficial Microorganisms. Front. Nutr. 7. doi: 10.3389/fnut.2020.00135
Pukkila-Worley, R., Ausubel, F. M. (2012). Immune Defense Mechanisms in theCaenorhabditis elegans Intestinal Epithelium. Curr. Opin. Immunol. 24, 3–9. doi: 10.1016/j.coi.2011.10.004
Rosso, S. B., Inestrosa, N. C. (2013). WNT Signaling in Neuronal Maturation and Synaptogenesis. Front. Cell. Neurosci. 7, 103. doi: 10.3389/fncel.2013.00103
Segers, M. E., Lebeer, S. (2014). Towards a Better Understanding of Lactobacillus rhamnosus GG - Host Interactions. Microb. Cell Fact. 13, S7. doi: 10.1186/1475-2859-13-S1-S7
Shimizu, T., Pastuhov, S. I., Hanafusa, H., Sakai, Y., Todoroki, Y., Hisamoto, N., et al. (2021). Caenorhabditis Elegans F-Box Protein Promotes Axon Regeneration by Inducing Degradation of the Mad Transcription Factor. J. Neurosci. 41, 2373–2381. doi: 10.1523/JNEUROSCI.1024-20.2021
Simonsen, K. T., Gallego, S. F., Færgeman, N. J., Kallipolitis, B. H. (2012). Strength in Numbers: “Omics” Studies of C. elegans Innate Immunity. Virulence 3, 477–484. doi: 10.4161/viru.21906
Sun, L., Zhi, L., Shakoor, S., Liao, K., Wang, D. (2016). microRNAs Involved in the Control of Innate Immunity in Candida Infected Caenorhabditis Elegans. Sci. Rep. 6, 36036. doi: 10.1038/srep36036
Tan, K., Deng, D., Ma, X., Cui, Y., Tian, Z. (2020). Pediococcus Acidilactici P25 Protected Caenorhabditis Elegans Against Enterotoxigenic Escherichia Coli K88 Infection and Transcriptomic Analysis of Its Potential Mechanisms. BioMed. Res. Int. 2020, 7340312. doi: 10.1155/2020/7340312
Thomas, J. H. (2006). Adaptive Evolution in Two Large Families of Ubiquitin-Ligase Adapters in Nematodes and Plants. Genome Res. 16, 1017–1030. doi: 10.1101/gr.5089806
Wang, X., Wang, X., Li, L., Wang, D. (2010). Lifespan Extension in Caenorhabditis Elegans by DMSO is Dependent on Sir-2.1 and Daf-16. Biochem. Biophys. Res. Commun. 400, 613–618. doi: 10.1016/j.bbrc.2010.08.113
Xiong, X.-P., Kurthkoti, K., Chang, K.-Y., Li, J.-L., Ren, X., Ni, J.-Q., et al. (2016). miR-34 Modulates Innate Immunity and Ecdysone Signaling in Drosophila. PloS Pathog. 12, e1006034. doi: 10.1371/journal.ppat.1006034
Yan, F., Cao, H., Cover, T. L., Washington, M. K., Shi, Y., Liu, L., et al. (2011). Colon-Specific Delivery of a Probiotic-Derived Soluble Protein Ameliorates Intestinal Inflammation in Mice Through an EGFR-Dependent Mechanism. J. Clin. Invest. 121, 2242–2253. doi: 10.1172/JCI44031
Zanni, E., Laudenzi, C., Schifano, E., Palleschi, C., Perozzi, G., Uccelletti, D., et al. (2015). Impact of a Complex Food Microbiota on Energy Metabolism in the Model Organism Caenorhabditis elegans. BioMed. Res. Int. 2015, 621709. doi: 10.1155/2015/621709
Zhang, W., Zhu, Y.-H., Yang, G.-Y., Liu, X., Xia, B., Hu, X., et al. (2018). Lactobacillus Rhamnosus GG Affects Microbiota and Suppresses Autophagy in the Intestines of Pigs Challenged With Salmonella infantis. Front. Microbiol. 8, 2705. doi: 10.3389/fmicb.2017.02705
Zhou, M., Liu, X., Yu, H., Yin, X., Nie, S.-P., Xie, M.-Y., et al. (2018). Cell Signaling of Caenorhabditis Elegans in Response to Enterotoxigenic Escherichia Coli Infection and Lactobacillus Zeae Protection. Front. Immunol. 9, 1745. doi: 10.3389/fimmu.2018.01745
Zhou, Y., Wang, X., Song, M., He, Z., Cui, G., Peng, G., et al. (2019). A Secreted microRNA Disrupts Autophagy in Distinct Tissues of Caenorhabditis Elegans Upon Ageing. Nat. Commun. 10, 1–14. doi: 10.1038/s41467-019-12821-2
Zhou, M., Zhu, J., Yu, H., Yin, X., Sabour, P., Zhao, L., et al. (2014). Investigation Into In Vitro and In Vivo Models Using Intestinal Epithelial IPEC-J2 Cells and Caenorhabditis Elegans for Selecting Probiotic Candidates to Control Porcine Enterotoxigenic Escherichia coli. J. Appl. Microbiol. 117, 217–226. doi: 10.1111/jam.12505
Keywords: C. elegans, probiotics, L. rhamnosus GG, microRNA, immunity
Citation: Yun B, Ryu S, Kang M, Lee J, Yoo J, Kim Y and Oh S (2022) Probiotic Lacticaseibacillus rhamnosus GG Increased Longevity and Resistance Against Foodborne Pathogens in Caenorhabditis elegans by Regulating MicroRNA miR-34. Front. Cell. Infect. Microbiol. 11:819328. doi: 10.3389/fcimb.2021.819328
Received: 21 November 2021; Accepted: 20 December 2021;
Published: 19 January 2022.
Edited by:
Hyeun Bum Kim, Dankook University, South KoreaReviewed by:
Soo Rin Kim, Kyungpook National University, South KoreaSooyeon Song, Jeonbuk National University, South Korea
Copyright © 2022 Yun, Ryu, Kang, Lee, Yoo, Kim and Oh. This is an open-access article distributed under the terms of the Creative Commons Attribution License (CC BY). The use, distribution or reproduction in other forums is permitted, provided the original author(s) and the copyright owner(s) are credited and that the original publication in this journal is cited, in accordance with accepted academic practice. No use, distribution or reproduction is permitted which does not comply with these terms.
*Correspondence: Younghoon Kim, eWtleXMyNTg0QHNudS5hYy5rcg==; Sangnam Oh, b3NhbmduYW1AamouYWMua3I=