- 1Institute of Tropical Medicine, University of Tübingen, Tübingen, Germany
- 2Centre de Recherches Médicales de Lambaréné, Lambaréné, Gabon
- 3German Center for Infection Research (DZIF), partner site Tübingen, Tübingen, Germany
- 4Department of Medical Microbiology, Radboud University Medical Center, Nijmegen, Netherlands
Boromycin is a boron-containing macrolide antibiotic produced by Streptomyces antibioticus with potent activity against certain viruses, Gram-positive bacteria and protozoan parasites. Most antimalarial antibiotics affect plasmodial organelles of prokaryotic origin and have a relatively slow onset of action. They are used for malaria prophylaxis and for the treatment of malaria when combined to a fast-acting drug. Despite the success of artemisinin combination therapies, the current gold standard treatment, new alternatives are constantly needed due to the ability of malaria parasites to become resistant to almost all drugs that are in heavy clinical use. In vitro antiplasmodial activity screens of tetracyclines (omadacycline, sarecycline, methacycline, demeclocycline, lymecycline, meclocycline), macrolides (oleandomycin, boromycin, josamycin, troleandomycin), and control drugs (chloroquine, clindamycin, doxycycline, minocycline, eravacycline) revealed boromycin as highly potent against Plasmodium falciparum and the zoonotic Plasmodium knowlesi. In contrast to tetracyclines, boromycin rapidly killed asexual stages of both Plasmodium species already at low concentrations (~ 1 nM) including multidrug resistant P. falciparum strains (Dd2, K1, 7G8). In addition, boromycin was active against P. falciparum stage V gametocytes at a low nanomolar range (IC50: 8.5 ± 3.6 nM). Assessment of the mode of action excluded the apicoplast as the main target. Although there was an ionophoric activity on potassium channels, the effect was too low to explain the drug´s antiplasmodial activity. Boromycin is a promising antimalarial candidate with activity against multiple life cycle stages of the parasite.
1 Introduction
Malaria remains one of the world’s most serious public health problems, especially in tropical and subtropical areas, with approximately 241 million cases and 627,000 deaths in 2020 (World Health Organization, 2021). Intracellular parasites of the genus Plasmodium cause the disease, and among the six species that can infect humans P. falciparum, P. vivax, P. malariae, P. ovale wallikeri, P. ovale curtisi, and the zoonotic species P. knowlesi (Rutledge et al., 2017; Grignard et al., 2019), P. falciparum is the most virulent (World Health Organization, 2021). P. knowlesi, described originally as a simian parasite, was recognized in 2000 as a zoonotic species that can infect humans (White, 2008). Although P. knowlesi occurs mainly in Malaysia, counting 13,612 malaria cases between 2017 to 2020 (Hu et al., 2021), it can cause severe malaria in humans (Amir et al., 2018) and is phylogenetically closer to other human pathogenic Plasmodium species (P. malariae, P. vivax, and P. ovale species) than P. falciparum (Rutledge et al., 2017; van Schalkwyk et al., 2019).
Plasmodium parasites have a complex life cycle that includes mosquito and vertebrate hosts (Siciliano and Alano, 2015). Clinical symptoms of malaria appear during the replication of parasites in the erythrocytic cycle (lasting approximately 24 h for P. knowlesi and 48 h for P. falciparum) in the human host (van Biljon et al., 2018). These symptoms can develop into life-threatening complications such as severe anemia, liver and kidney failure and cerebral malaria if not treated properly (Milner et al., 2014). During each replication cycle, a small number of asexual parasites starts a different genetic program and develops into the transmissible sexual form, named gametocytes (Portugaliza et al., 2020). Gametocytes are non-dividing parasite stages with low metabolic activity whose immature stages are hidden in the bone marrow while the mature stage (stage V) circulates in the blood stream (Gardiner and Trenholme, 2015)
Currently, the mainstay P. falciparum malaria treatments are artemisinin-based combination therapies (ACT) (World Health Organization, 2021). Artemisinin and its derivatives are fast-acting and rapidly reduce the asexual parasite load but have a short half-life (2-4 h for artemisinin and <1 h for artesunate) (Benakis et al., 1997). They are combined to a slow-acting partner drug with longer half-life to clear remaining parasites, and to protect artemisinin against resistances. Nonetheless, reports of P. falciparum with delayed clearance after artesunate or ACT treatment are accumulating, especially from the Greater Mekong Region where resistances to the partner drugs are also present (Pluijm et al., 2019; WWARN K13 Genotype-Phenotype Study Group, 2019). More recently first reports of kelch13 mutated parasites with a slow clearance have also appeared from Africa (Balikagala et al., 2021). This poses a threat to the existing malaria treatment options (Straimer et al., 2015; Nsanzabana, 2019). In addition, the current ACT drugs are inactive against mature P. falciparum gametocytes (stage IV and V). In low transmission areas is recommended to give additionally a single dose of primaquine, except in children younger than six months, pregnant and breastfeeding women with infants younger than six months, to prevent transmission of the parasite, but these recommendations are so far not strictly followed in all areas (World Health Organization, 2021).
The interest in using antibiotics for malaria treatment appeared due to the emergence of resistant parasites to the former mainstay drug chloroquine. Tetracyclines were the first antibiotics used to treat uncomplicated malaria, dating back to the 1950s (Imboden et al., 1950; Grande et al., 1956). Currently, doxycycline and the combination of sulfadoxine-pyrimethamine are used for malaria prevention of travelers and risk groups in malaria-endemic regions, respectively. In addition, clindamycin combined with quinine is used to treat pregnant women during the first trimester or combined with artesunate or quinine to treat uncomplicated malaria when an ACT is unavailable (World Health Organization, 2021).
Tetracyclines and macrolides, both naturally produced by Streptomyces, have shown promising antiplasmodial activities as reviewed before (Gaillard et al., 2015; Gaillard et al., 2016; de Carvalho et al., 2021). Most antibiotics with antiplasmodial activity target the apicoplast; a plastid-like organelle derived from endosymbiotic bacteria responsible for the biosynthesis of isoprenoid precursors (Yeh and DeRisi, 2011). Antibiotics can be fast or slow-acting drugs, depending on whether they target the treated parasites or act on their progeny, causing the so-called delayed death effect (Dahl and Rosenthal, 2007). Commonly, tetracyclines belong to the latter group (Gaillard et al., 2015; de Carvalho et al., 2021), and novel tetracyclines have shown superior antiplasmodial activity (e.g. eravacycline and tigecycline IC50s at 14 and 38 nM, respectively (Held et al., 2010; Koehne et al., 2021) versus doxycycline and tetracycline IC50s at 241 and 340 nM, respectively) (Koehne et al., 2021). Some macrolides such as ivermectin (Mendes et al., 2017; de Carvalho et al., 2019), borrelidin (Otoguro et al., 2003; Ishiyama et al., 2011), and kitasamycin (also called leucomycin) (Ekland et al., 2011) had a fast/first cycle in vitro growth inhibitory activity against P. falciparum (IC50 values of ~ 100 nM, 1.8 nM and 50 nM, respectively). However, the molecular targets of these agents in Plasmodium species are not known.
This study aimed to identify antibiotics that are potent and fast acting against P. falciparum. Therefore, we evaluated the antiplasmodial effect of two recently marketed tetracyclines, named sarecycline, and omadacycline and some “old” tetracyclines in clinical use named demeclocycline, meclocycline, lymecycline, chlortetracycline and methacycline (Figure 1). In addition, the macrolides oleandomycin, troleandomycin, josamycin, and boromycin were evaluated (Figure 2). Tetracyclines with known antiplasmodial activity were used as controls. We identified boromycin to be a highly active and fast acting antibiotic. Boromycin is a polyether-macrolide antibiotic produced by Streptomyces antibioticus and was the first boron-containing compound found in nature (Dunitz et al., 1971). Previous studies showed a potent effect of boromycin against Gram-positive bacteria, HIV virus and protozoan parasites (Kohno et al., 1996; Moreira et al., 2016; Abenoja et al., 2021). Due to its low nanomolar activity in our screening, we further assessed its antiplasmodial profile also including transmission stages and investigated whether its potential mode of action involves the apicoplast as described for antibiotics in general (Dahl and Rosenthal, 2007) or ionophoric activity on potassium channels as shown before for boromycin (Moreira et al., 2016). In addition, we evaluated its in vitro activity against another Plasmodium species (P. knowlesi).
2 Materials and Methods
2.1 Chemicals
All tested drugs were first dissolved in their respective solvents and subsequently further diluted in complete culture medium to have the desired final concentration (Supplementary Table 1 and Supplementary Information Figures 1, 2).
2.2 Parasite In Vitro Culture
2.2.1 Plasmodium Asexual Stages
P. falciparum laboratory strains 3D7 (chloroquine-sensitive, provided by BEI resources, MRA-102), Dd2 (multidrug-resistant, provided by BEI resources, MRA-150), K1 (multidrug-resistant, provided by BEI resources, MRA-159), 7G8 (chloroquine resistant, provided by BEI resources, MRA-154) and NF-54 (chloroquine sensitive, provided by Sanaria, a vigorous gametocyte producer), and P. knowlesi laboratory strain A1-H.1 (kindly provided by Robert Moon of LSHTM, London, UK under a MTA by the Francis Crick institute) were cultivated in vitro as described before (Moon et al., 2013; de Carvalho et al., 2019). Briefly, the parasites were maintained in complete culture medium consisting of RPMI-1640 (Sigma-Aldrich) supplemented with 1M N-2-hydroxyethylpiperazine-N-2-ethane sulfonic acid (HEPES) solution (2.4% v/v) (Sigma-Aldrich), 200 mM L-glutamine (Gibco), 50 µg/ml gentamicin (Gibco), and 10% of AlbuMax II solution containing RPMI, HEPES, NaHCO3, D-Glucose, hypoxanthine and 50 g/l of AlbuMax II (0.5% wt/vol in culture medium) at 2.5% hematocrit. The cultures were maintained at 5% CO2, 5% O2, at 37°C, with a change of medium every two days. P. falciparum parasites were synchronized by magnetic column separation prior to the assays (Ribaut et al., 2008). P. knowlesi was cultivated as described for P. falciparum, with minor modifications. Parasites were cultivated in complete culture medium as detailed above supplemented with 5% human AB serum. Shortly before the growth inhibition assays, P. knowlesi were synchronized by density centrifugation with Nycodenz solution (Progen) to recover mature schizonts as described before (van Schalkwyk et al., 2017). The Nycodenz solution was prepared according to the manufacturer’s instruction and kept protected from the light at 4°C and warmed before using.
The asexual growth inhibition assays were performed with 3D7 and A1-H.1, while NF-54 was used for gametocyte assays.
2.2.2 P. falciparum Sexual Stages
P. falciparum gametocyte culture was performed as described previously (de Carvalho et al., 2019) with minor modifications. The gametocyte culture was initiated with synchronized ring-stage NF54 parasites at 6% hematocrit and 0.3% parasitemia. Complete culture medium (as described above) was supplemented with 5% human serum and changed daily without parasite dilution for 14 days. The medium was doubled when parasitemia reached 5%, and 50-mM N-acetyl-d-glucosamine (MP Biomedicals GmbH) was added between day 11 and day 14 to remove asexual stages. On day 15, mature gametocytes were purified with a Nycodenz and magnetic column separation (MACS) to remove erythrocytes and concentrate the gametocyte population.
2.3 Growth Inhibition Assays With Asexual Parasites
Growth inhibition assays of asexual stages of P. falciparum were performed as described before (Noedl et al., 2005). Briefly, the drugs (Supplementary Table 1) were distributed in a 3-fold serial dilution in 96-well plates. The highest concentration of the solvent (<0.1%) did not interfere with parasite growth. Synchronized ring-stage parasites were diluted to a parasitemia of 0.05% with O Rh+ erythrocytes in complete culture medium and seeded at a hematocrit of 1.5% in a total volume of 225 μl per well. Plates were subsequently incubated at 5% CO2, 5% O2, at 37°C before the plates were frozen and thawed three times. The growth inhibition of P. falciparum was measured through an enzyme-linked immunosorbent assay (ELISA) for histidine-rich protein 2 (HRP2) using a microplate reader (CLARIOstar BMG Labtech) (excitation filter: 450 nm).
Growth inhibition assays with P. knowlesi were performed as described previously with minor modifications (van Schalkwyk et al., 2019). Briefly, boromycin and chloroquine were distributed in a 3-fold serial dilution in 96-well plates. Synchronized ring-stage parasites were diluted to a parasitemia of 0.5% (a higher parasitemia compared to the HRP2 based assay is needed due to the differences in the limit of detection and therefore optimal assay conditions) with O Rh+ erythrocytes and subsequently seeded at a hematocrit of 2% and complete culture medium without human AB serum but double (20%) AlbuMax II solution in a total volume of 225 μl per well. The growth inhibition of P. knowlesi was measured with SYBR Green-I (ThermoFisher) using CLARIOstar (BMG Labtech) (490 nm excitation filter) as HRP2 is not present in this species. For comparison, growth inhibition of boromycin and chloroquine – treated P. falciparum was measured using the same methodology.
P. falciparum was incubated with the drugs for 3 and 6 days to assess the onset of activity. Both time points were assessed because many antibiotics target only the progeny of the treated parasites, causing a delayed death effect that is measured only after the second replication cycle (after 6 days). Chloroquine and clindamycin were used as positive controls for 3- and 6-day assays, respectively.
To observe the parasite morphology, P. falciparum strain 3D7 and Dd2 were incubated with boromycin at 1 nM and for comparison also 3D7 with chloroquine at 8 nM for 6 h for visual observation of drug effect by light microscopy. Thin blood smears were prepared, fixed in 100% methanol for 10 seconds, and stained with Giemsa (Merck) solution (5%) for 20 minutes. The slides were washed and observed under a Leica DMBL microscope, and pictures were taken using a ProgRes C10 camera and software (Jenoptik), at 100X magnification. Three biological replicates were prepared.
2.4 In Vitro Activity Against Mature Gametocytes of P. falciparum
Drug sensitivity assays against mature (stage V) P. falciparum gametocytes were performed as described previously (Lelièvre et al., 2012; de Carvalho et al., 2019). Briefly, the drugs were precoated in a 3-fold dilution in 96-well plates. The concentration of the solvent did not interfere with parasite growth. Epoxomicin and methylene blue (Supplementary Table 1) were used as positive control. Subsequently, the previously purified mature gametocytes were seeded to the plates (50,000 gametocytes/well) and incubated at 37°C in 5% CO2 and 5% O2. After 48 h, the ATP production was measured by the BacTiterGlo assay (Promega), according to the manufacturer’s instructions. Finally, the results were quantified using a luminometer (LUmo; Autobio). In addition, to visually inspect the effect of boromycin on gametocytes thin blood smears of untreated and boromycin (8 nM) and epoxomicin (15 nM) - treated gametocytes (50,000 gametocytes/well), were prepared, fixed in 100% methanol for 10 seconds, and stained with Giemsa (Merck) solution (5%) for 20 minutes. The slides were washed and observed under a Leica DMBL microscope, and pictures were taken using a ProgRes C10 camera and software (Jenoptik), at 100X magnification.
2.5 Cytotoxicity Assay
HepG2 cells (obtained from ATCC; HB-8065), a human hepatocyte carcinoma cell line, were maintained in DMEM medium (Sigma-Aldrich) supplemented with 10% of inactivated foetal bovine serum (Sigma-Aldrich), 200 mM L-glutamine (Gibco), 12 mL of HEPES buffer (Gibco), and 50 µg/ml penicillin/streptomycin solution (Gibco). Trypsin (Gibco) was used to detach the cells when they reached a semi-confluent layer.
Cytotoxicity was evaluated using the neutral red assay as described previously (Mishra et al., 2021). Briefly, 300,000 cells were seeded in supplemented Dulbecco´s Modified Eagle´s Medium (DMEM) medium as described above to 96-well plates. After 24 hours, the cells were incubated with a twofold serial dilution of the respective drug diluted in supplemented DMEM medium for an additional 24 hours. The highest concentration of the solvent (<1%) did not interfere with cell viability. Subsequently, the drug-containing medium was replaced by a supplemented DMEM medium with 1.5% of neutral red, and the cells were incubated for an additional 3 h at 37°C. Cells were then washed with phosphate buffered saline (pH 7.2), and 100 µL of freshly prepared lysing buffer (50% methanol, 49% distilled water, and 1% acetic acid) were added to the plates. Subsequently, the cells were shaken for 10 min and the absorption was measured at a wavelength of 540 nm using CLARIOstar (BMG Labtech).
2.6 Isopentenyl Pyrophosphate Trilithium Salt (IPP) Rescue Assay
Growth inhibition assays were prepared as described in section Parasite In Vitro Culture with modifications to assess the specificity of boromycin for the inhibition of the isoprenoid precursor biosynthetic pathway (MEP/DOXP/non-mevalonate pathway) found in the apicoplast (Uddin et al., 2018). Briefly, the parasites (P. falciparum strain 3D7) were incubated with boromycin and clindamycin (positive control) and supplemented with IPP (Sigma) at 200 µM for 6 days as described before (Uddin et al., 2018). Since biosynthesis of isoprenoid precursors is the only required function of the apicoplast, supplementation with IPP can rescue treated parasites if the apicoplast is the main target of the drug (Uddin et al., 2018). The assay was done with and without IPP supplementation on the same plate and results were directly compared.
2.7 Ionophoric Activity Assay
Previously, boromycin was described to act as a potassium ionophore in Bacillus subtilis and Mycobacterium tuberculosis (Pache and Zaehner, 1969a; Moreira et al., 2016). So herein, growth inhibition assays complemented with KCl or MgCl2 (negative control) were performed to evaluate if this is also the case in P. falciparum. To define a subtoxic concentration of MgCl2 and KCl for P. falciparum a standard growth inhibition assays with serial dilutions of these compounds were performed as described in section Parasite In Vitro Culture. The concentration of 220 µg/mL was chosen as no signs of toxicity were seen and first inhibitory effects were observed with 440 µg/mL (Supplementary Figure 1) Subsequently, a growth inhibition assay was prepared with a 2-fold dilution of boromycin with the addition of the sub-toxic concentration of either MgCl2 (Sigma) or KCl (Sigma) to all wells. The assay was run in parallel to a standard growth inhibition assay of boromycin without supplementation of MgCl2 or KCl as control, and the obtained IC50s were compared.
2.8 Analysis
All assays were performed at least three times in duplicates. Individual IC50 values were determined by nonlinear regression analysis of log concentration-response curves, using the drc v3.0-1 package (Ritz and Streibig, 2005) of R v4.1.2 (R Core Team, 2014). Mean IC50 values and standard deviations (SDs) were calculated for each growth inhibition assay using Excel. The chemical structures of the drugs were drawn using ChemDraw 19.0. The graphical presentations and statistical analyses (unpaired t-test) were done with GraphPad Prism v8.
3 Results
3.1 Activity of Antibiotics Against Asexual Stages of P. falciparum and P. knowlesi
P. falciparum was exposed to 15 antibiotics (tetracyclines, macrolides) and chloroquine (control drug) for 3 and for 6 days. This allowed to assess the drug´s inhibitory effect on the first and second parasite replication cycle, respectively (see results in Table 1), that indicates a fast or slow onset of antiplasmodial activity of the antibiotics.
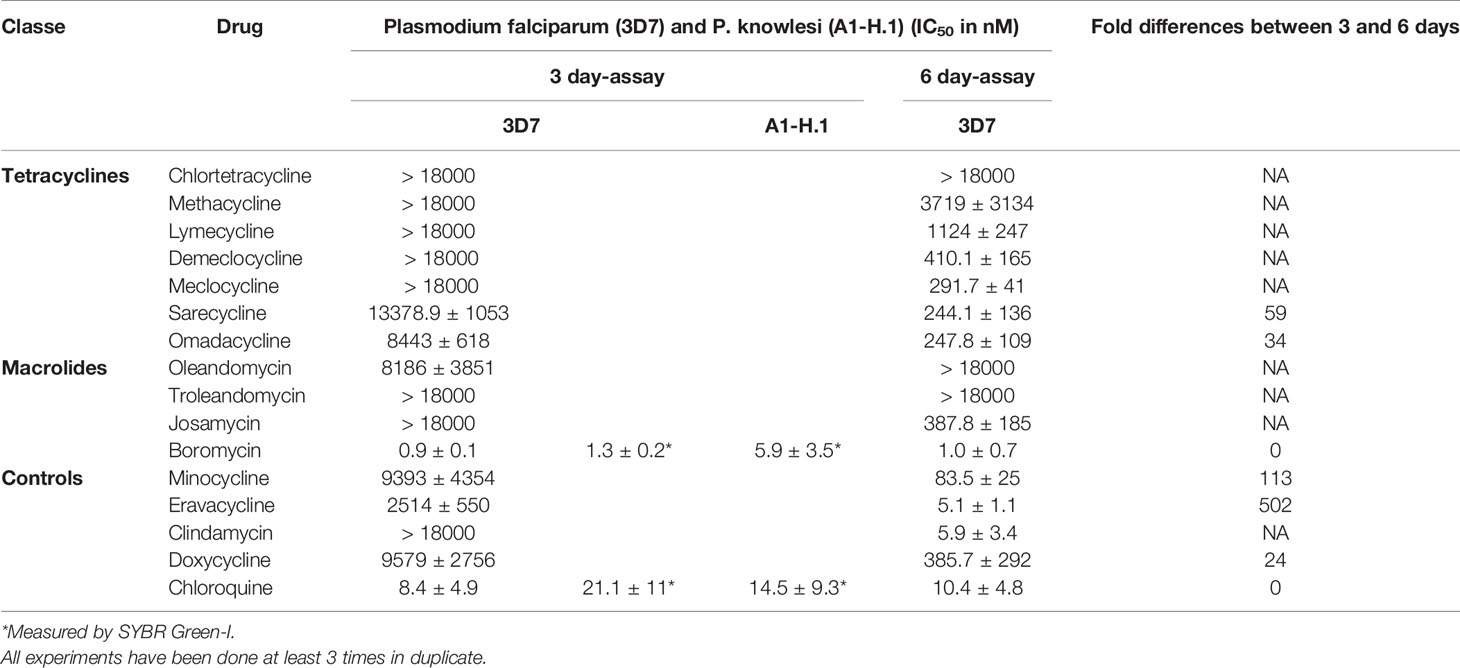
Table 1 Activity of antibiotics against P. falciparum strain 3D7 after 3 and 6 days of incubation and P. knowlesi strain A1-H.1 after 3 days.
None of the tetracyclines evaluated in this study showed a pronounced activity (in the nanomolar range) in the 3-day assay against P. falciparum, but had some delayed activity after 6 days of drug exposure (Table 1). Chlortetracycline, methacycline and lymecycline were still poorly active after 6 days. The first-time tested drugs against P. falciparum demeclocycline, sarecycline, omadacycline and the already previously tested drugs/controls doxycycline, minocycline, and eravacycline were active in the nanomolar range against both species after 6 days (Table 1). Boromycin was highly active against P. falciparum at an IC50 of 1.0 nM after 3 days of incubation (Table 1), To further characterize its antiplasmodial activity, boromycin was also assessed against P. knowlesi and its pronounced activity was confirmed there (Table 1).
3.2 Cytotoxicity of Boromycin Against Human Cells
Boromycin was cytotoxic at millimolar concentrations against human HepG2 cells with a selectivity index (SI) higher than 200,000. (Table 2). Chloroquine was used as comparator and was more cytotoxic than boromycin.

Table 2 Cytotoxicity of boromycin and the comparator chloroquine against HepG2 cells and calculated selectivity index.
3.3 Characterization of the Antiplasmodial Activity of Boromycin
3.3.1 Activity of Boromycin Against Asexual Stages of P. falciparum Chloroquine-Resistant Strains
Different drug resistant strains of P. falciparum (Dd2, K1 and 7G8) were incubated with boromycin for 3 days to assess the potential of cross-resistance. Results were comparable to activities against 3D7 with IC50 values in the picomolar range, and no cross-resistance to boromycin by these parasite lines was seen (Table 3). When investigating the morphological effect of the drug treatment on the parasite, untreated P. falciparum parasites of the strain 3D7 (Figure 3A) showed the usual ring shape, while boromycin-treated ring-stages (Figure 3B), as well as trophozoites and schizonts (– Supplementary Figure 2) showed the same pyknotic appearance after boromycin incubation at 1 nM for 6 h. In addition, the same fast effect and morphological changes were also induced in the chloroquine resistant strain Dd2 (Figure 3C).

Table 3 Antiplasmodial activity of boromycin against different P.falciparum chloroquine-resistant strains in comparison to 3D7 after 3 days of incubation.
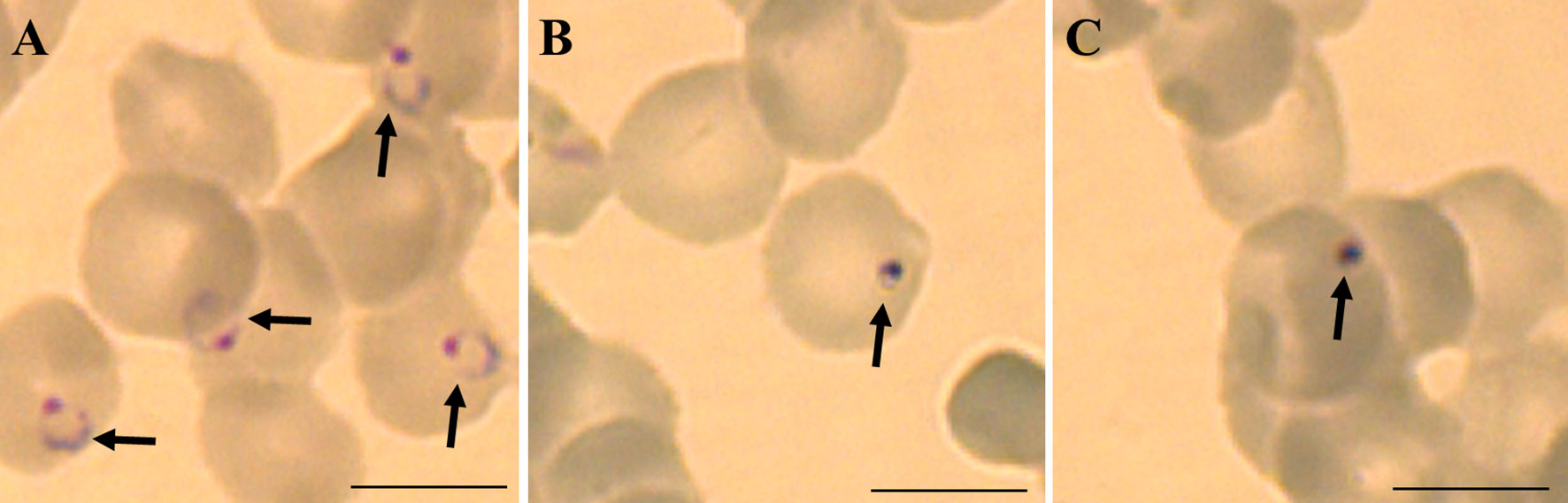
Figure 3 Representative micrographies of P. falciparum infected erythrocytes stained with Giemsa: (A) untreated ring stage parasites after 6 hours, (B) parasites strain 3D7 treated with 1 nM of boromycin for 6 h and (C) parasites strain Dd2 treated with 1 nM of boromycin for 6 h. Arrows: parasites. Scale bar: 10 µm.
3.3.2 Activity of Boromycin Against Mature Gametocytes
Mature P. falciparum gametocytes (stage IV and V) were incubated with a serial dilution of boromycin for 48 h. Afterwards, ATP production was quantified to evaluate the gametocytocidal effect (Figure 4A). The results revealed that boromycin was highly active (IC50 at 8.5 ± 3.6 nM), similarly active as the positive comparator epoxomicin (15.2 ± 3.4 nM) and more active than methylene blue (284 ± 35 nM). When observed microscopically, untreated gametocytes displayed their usual elongated shape with rounded edges (Figure 4A), but both boromycin (Figures 4B, C) and epoxomicin – treated (Figures 4C, D) parasites showed thinner body and edges, and intracellular disorganization. The length was not affected in epoxomicin-treated gametocytes, resulting in a needle-like shape, while boromycin-treated gametocytes presented a smaller appearance.
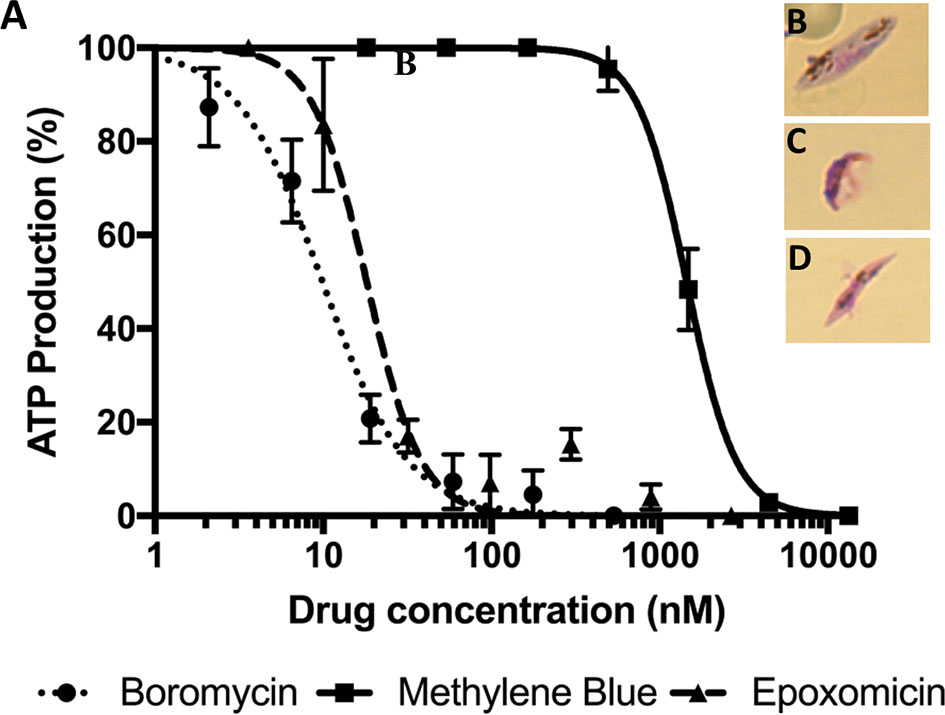
Figure 4 Activity of boromycin, and the positive controls epoxomicin and methylene blue against mature (stage IV-V) P. falciparum gametocytes treated for 48 h. (A) Boromycin showed a more potent activity than methylene blue and similar activity to epoxomicin. (B) Untreated mature gametocytes. (C) Boromycin-treated gametocytes and (D) epoxomicin-treated parasites. All experiments were done at least 3 times in duplicate.
3.3.3 Activity of Boromycin on the Apicoplast
P. falciparum parasites were incubated with boromycin supplemented with IPP for 6 days to assess whether boromycin targets the apicoplast as described for many antibiotics; clindamycin was used as positive control. At the same time a standard growth inhibition assay without IPP was carried out as a comparator. As expected, clindamycin-treated parasites could be rescued by the supplementation with IPP, but the IC50 values for boromycin remained similar at low nanomolar concentrations for both conditions (Table 4), suggesting that this drug effect cannot be rescued by IPP and has a different target from the apicoplast (Figure 5).

Table 4 IC50 values obtained with isopentenyl pyrophosphate trilithium salt (IPP) rescue assay in comparison to standard drug assays against P. falciparum strain 3D7.
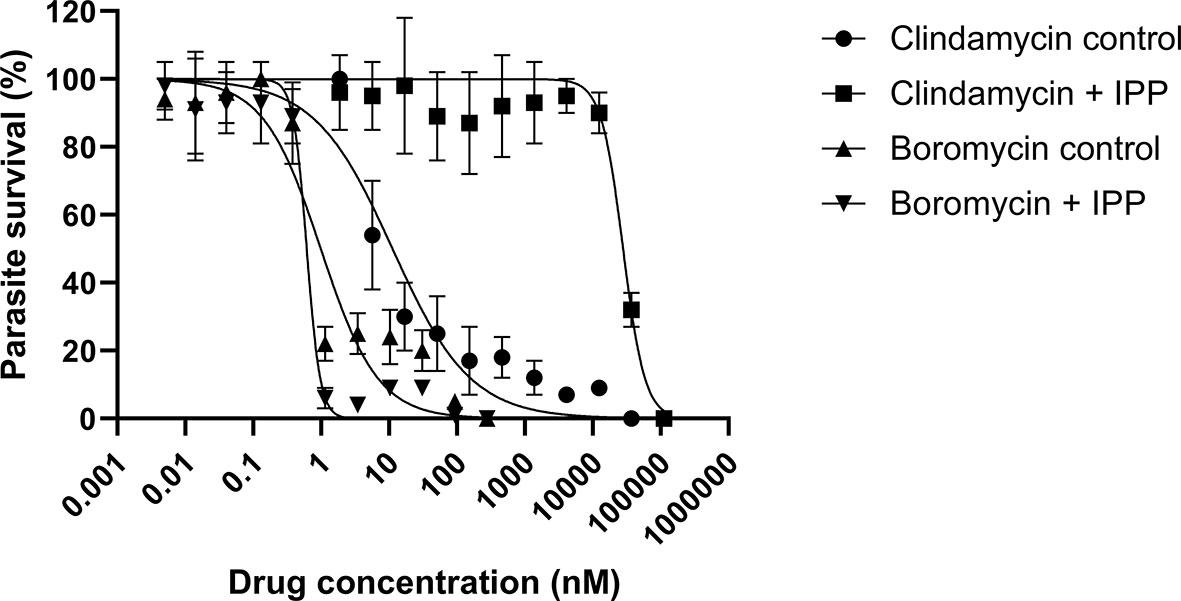
Figure 5 Survival curves of isopentenyl pyrophosphate trilithium salt (IPP) rescue assays. Parasites of the P. falciparum strain 3D7 were treated with boromycin or clindamycin (positive control) with or without IPP supplementation and incubated for 6 days. Boromycin-treated parasites were not rescued by IPP, indicating that the apicoplast is not the main target. Experiments were done at least 3 times in duplicate.
3.3.4 Ionophoric Activity of Boromycin
P. falciparum parasites were incubated with a serial dilution of boromycin, and chloroquine (control) with or without supplementation of 220 µg/mL of MgCl2 or KCl, for 3 days to assess whether boromycin has the ionophoric activity on P. falciparum as described for Mycobacterium and Bacillus subtilis (Abenoja et al., 2021) (Table 5). Boromycin and chloroquine displayed the expected IC50 value, the supplementation of KCl at 220 µg/mL increased IC50 of boromycin by 3-fold, while no difference was observed for chloroquine. On the other hand, the incubation with boromycin or chloroquine in the presence of MgCl2 led to a 3-5-fold decrease in the respective IC50s.

Table 5 Activity of boromycin and the control drug chloroquine against the P. falciparum strain 3D7 in the presence/absence of KCl (220 µg/ml) and MgCl2. (220 µg/ml).
4 Discussion
Antibiotics for malaria treatment have been investigated since the middle of the last century, with tetracyclines playing a central role. In recent years, macrolides have also been particularly studied as antimalarials (Gaillard et al., 2015; Gaillard et al., 2016a; Gaillard et al., 2016b). Although the delayed death effect caused by most antibiotics limits their use as first-line chemotherapy, some antibiotics such as doxycycline, clindamycin, and the combination sulfadoxine-pyrimethamine (that does not display the delayed activity) are recommended as prophylaxis or treatment in certain populations (World Health Organization, 2021). The need for new alternative therapies, along with the limited investments in drug discovery to treat malaria, make drug repurposing a worthy alternative. Herein, the in vitro antiplasmodial activity of tetracyclines and macrolides not previously tested against Plasmodia along with comparator drugs were evaluated against blood-stages of P. falciparum revealing boromycin, as a potent antiplasmodial drug with a fast/first cycle activity that we assessed further.
All tested active tetracyclines showed a delayed death effect against P. falciparum (strain 3D7). The newly licensed tetracyclines omadacycline (licensed for treatment of bacterial pneumonia and acute skin infections) (Nuzyra, 2021) and sarecycline (licensed for treatment of acne) (United States Food and Drug Administration, 2021) showed comparable activity to doxycycline. None of the newly tested tetracyclines achieved the activity of the next generation tetracyclines tigecycline (Held et al., 2010) and eravacycline (Koehne et al., 2021) that suggested that modifications at the C9 position on the D ring that circumvents the ribosomal protection resistance mechanism found in bacteria could be beneficial for the activity against Plasmodia. However, the structurally similar omadacycline did not show this improved activity. In a similar way, differences in activities for these three antibiotics were also seen for rickettsia species (Quade et al., 2021). The delayed death effect was also shown by the macrolide josamycin suggesting that the apicoplast could be the target of this drug. The tetracyclines chlortetracycline, methacycline, lymecycline, and the macrolides troleandomycin and oleandomycin were only poorly active with IC50 in the micromolar range. Whereas the macrolide boromycin showed a fast onset of activity at a single-digit nanomolar concentration against both Plasmodium species.
P. falciparum is undoubtably responsible for most of the malaria burden, especially for most of the malaria deaths, which justifies to mainly tailor treatments to this malaria species. However, there are five additional Plasmodium species responsible for malaria in humans and when elimination of malaria is the aim, all these species must be targeted. Until recently, P. falciparum was the only option for drug screening in vitro because the other human malaria parasites could not be kept in continuous in vitro culture (Mehlotra et al., 2017; van Schalkwyk et al., 2019). However, now P. knowlesi has been adapted for in vitro culture and its inclusion in drug screenings could give additional information on antiplasmodial activities of compounds as it is phylogenetically closer to the other human Plasmodium species (Rutledge et al., 2017; van Schalkwyk et al., 2019). Even though previous studies have claimed that most antiplasmodial compounds are equipotent against P. falciparum and P. knowlesi (Moon et al., 2013; Fatih et al., 2013), recent studies have reported different susceptibility profiles (van Schalkwyk et al., 2021) especially for some novel drugs in development. P. knowlesi was described as less susceptible to inhibitors of the dihydrofolate reductase, dihydroorotate dehydrogenase (van Schalkwyk et al., 2017), and ATP4 (van Schalkwyk et al., 2019). Herein, we also observed that boromycin was very potent against both species, P. knowlesi was slightly less vulnerable than P. falciparum, with a 4.5-fold higher IC50.
The most promising antibiotic from our screening was the macrolide boromycin. Boromycin was isolated from a Streptomyces antibioticus found in a soil sample from Ivory coast and was first described in 1967 (Hütter et al., 1967; Pache and Zaehner, 1969b). It has a potent effect against Gram-positive bacteria in vitro (Moreira et al., 2016), and it was shown to be active against HIV (Kohno et al., 1996), Babesia (Hütter et al., 1967) and some other pathogens (de Carvalho et al., 2019). Its antiplasmodial activity was already once described in a patent filed in the US in 1973 where its activity in the malaria models of P. berghei in mice (ED50: 2.2 mg/kg) and P. gallinaceum in birds (ED50: ~ 7 mg/kg) is given (Prelog et al., 1973). Additionally, its in vitro activity against P. falciparum strain K1 (chloroquine – resistant) and FCR3 (chloroquine – sensitive) was described to be 39 and 40 nM, respectively (Tsutsui et al., 2010). In the same study, only a low selectivity index against MRC-5 cells (~12) was described, but a 97.9% parasite reduction was observed when P. berghei – infected mice were treated p.o. at 10 mg/kg according to Peter´s test. However, no description of the evaluation of its gametocytocidal activity and stage specific effect could be identified by us.
In our investigation, boromycin showed a high activity and a fast onset of action, a desired property of antimalarials to quickly reduce parasite load and avoid the disease progression to the severe form. It also showed an excellent selectivity index against HepG2 cells. This favorable toxicity profile was also confirmed previously (Moreira et al., 2016), and the LD50 in mice is given as 180 mg/kg-1 (Klingel, 1980). These results encouraged a more detailed investigation of the antiplasmodial activity of boromycin.
Boromycin’s in vitro activity against laboratory strains showed potent activity as it was active at low nanomolar and even at picomolar concentrations. No cross-resistance with the tested chloroquine/multi-resistant strains (Dd2, 7G8, and K1) was seen as the IC50 values were in same range as for the drug sensitive strain 3D7, suggesting that is not affected by the common resistance mechanisms (Ding et al., 2012). Morphologically, ring stage parasites showed a pyknotic appearance after 6 h treatment with boromycin at 1 nM, suggesting that it leads to a rapid death. We additionally evaluated the activity of boromycin against mature gametocytes and could see a very potent activity superior to the positive control methylene blue, similarly active as the proteasome inhibitor epoxomicin. Drugs with transmission-blocking activity are of special interest and would be a great asset for controlling and eliminating malaria (Sinden, 2017; Wadi et al., 2019). Currently, primaquine is the only approved drug active against gametocytes (Lin et al., 2017) but its use is restricted mainly due to its haemolysis-inducing effect in patients with glucose-6-phosphate dehydrogenase deficiency (Recht et al., 2018), and additionally it has to be metabolized by a liver enzyme (CYP2D6) to be active. Drugs with multi-stage activities and novel modes of action are especially desired for the next generation of antimalarials (Ding et al., 2012).
The apicoplast is described as the main target of antibiotics that exert the delayed death effect (Dahl and Rosenthal, 2007; Ekland et al., 2011) and the fast-acting antibiotic fosmidomycin (Uddin et al., 2018). Apicoplast-free parasites can survive and proliferate when the medium is supplemented with IPP (Yeh and DeRisi, 2011), but this did not rescue the boromycin-treated parasites in our assay; therefore, we could rule out the apicoplast as a main target.
The ionophoric activity of boromycin, especially for K+ channels, was firstly described in Bacillus subtilis in addition to the impairment of protein, RNA, and DNA synthesis (Pache and Zaehner, 1969a). This ionophoric activity was later confirmed in Mycobacterium bovis, where a rapid loss of membrane potential, reduction of intracellular ATP, and leakage of cytoplasmic proteins were induced (Moreira et al., 2016) and the external addition of a high concentration of KCl (20 mg/mL) decreased boromycin activity from 50% to 10%. To assess whether boromycin acts as a potassium ionophore also in P. falciparum, parasites were simultaneously incubated with boromycin together with the highest non-toxic concentration (220 µg/mL) of KCl or MgCl2 (negative control). We could not incubate Plasmodium parasites at a similar high ion concentration as in the Mycobaterium experiments, as this concentration was toxic to the parasites. We identified a three-fold IC50 increase with the addition of KCl. However, it is most probably not the primary mode of action, as the activity remained in the low nanomolar range. On the other hand, the boromycin IC50 decreased with addition of MgCl2, this was also seen for chloroquine, an effect that was described before (Hess et al., 1995). Recently, boromycin was shown to decrease intracellular multiplication of both Toxoplasma gondii and Cryptosporidium parvum (Abenoja et al., 2021), but the potential target in these parasites was not further investigated.
In conclusion, we presented the antiplasmodial activity of different tetracycline and macrolide antibiotics against P. falciparum. The tetracyclines tested here did not show higher in vitro activities than previously tested tetracyclines and all displayed a slow/second cycle onset of action. The macrolide boromycin showed the most noticeable results as a fast-acting drug against both Plasmodium species and P. falciparum mature gametocytes. These results, along with the historical antiparasitic effects of boromycin, suggest that boromycin deserves a deeper investigation as an alternative therapy for diseases caused by apicomplexan parasites, including Plasmodia.
Data Availability Statement
The original contributions presented in the study are included in the article/Supplementary Material. Further inquiries can be directed to the corresponding author.
Author Contributions
JH and LPC designed the study, experiments were performed by SG-O. JH, LPC, and SG-O analyzed the data, all authors contributed to interpretation of the data. The first draft of the manuscript was written by LPC. All authors commented and contributed to the article and approved the final version of the manuscript.
Conflict of Interest
The authors declare that the research was conducted in the absence of any commercial or financial relationships that could be construed as a potential conflict of interest.
Publisher’s Note
All claims expressed in this article are solely those of the authors and do not necessarily represent those of their affiliated organizations, or those of the publisher, the editors and the reviewers. Any product that may be evaluated in this article, or claim that may be made by its manufacturer, is not guaranteed or endorsed by the publisher.
Acknowledgments
We acknowledge support by Open Access Publishing Fund of University of Tübingen.
Supplementary Material
The Supplementary Material for this article can be found online at: https://www.frontiersin.org/articles/10.3389/fcimb.2021.802294/full#supplementary-material
References
Abenoja, J., Cotto-Rosario, A., O’Connor, R. (2021). Boromycin has Potent Anti-Toxoplasma and Anti-Cryptosporidium Activity. Antimicrob. Agents Chemother. 65, e01278–e01220. doi: 10.1128/AAC.01278-20
Amir, A., Cheong, F. W., de Silva, J. R., Liew, J. W. K., Lau, Y. L. (2018). Plasmodium Knowlesi Malaria: Current Research Perspectives. Infect. Drug Resist. 11, 1145–1155. doi: 10.2147/IDR.S148664
Balikagala, B., Fukuda, N., Ikeda, M., Katuro, O. T., Tachibana, S.-I., Yamauchi, M., et al. (2021). Evidence of Artemisinin-Resistance Malaria in Africa. NEJM 385, 1163–1171. doi: 10.1056/NEJMoa2101746
Benakis, A., Paris, M., Loutan, L., Plessas, C. T., Plessas, S. T. (1997). Pharmacokinetics of Artemisinin and Artesunate After Oral Administration in Healthy Volunteers. Am. J. Trop. Med. Hyg. 56, 17–23. doi: 10.4269/ajtmh.1997.56.17
Dahl, E. L., Rosenthal, P. J. (2007). Multiple Antibiotics Exert Delayed Effects Against the Plasmodium Falciparum Apicoplast. Antimicrob. Agents Chemother. 51, 3485–3490. doi: 10.1128/AAC.00527-07
de Carvalho, L. P., Kreidenweiss, A., Held, J. (2021). Drug Repurposing: A Review of Old and New Antibiotics for the Treatment of Malaria: Identifying Antibiotics With a Fast Onset of Antiplasmodial Action. Molecules 26, 2304. doi: 10.3390/molecules26082304
de Carvalho, L. P., Sandri, T. L., de Melo, E. J. T., Fendel, R., Kremsner, P. G., Mordmüller, B., et al. (2019). Ivermectin Impairs the Development of Sexual and Asexual Stages of Plasmodium Falciparum In Vitro. Antimicrob. Agents Chemother. 63, e00085–e00019. doi: 10.1128/AAC.00085-19
Ding, X. C., Ubben, D., Wells, T. N. C. (2012). A Framework for Assessing the Risk of Resistance for Anti-Malarials in Development. Malar. J. 11, 292–303. doi: 10.1186/1475-2875-11-292
Dunitz, J. D., Hawley, D. M., Miklol, D., White, D. N. J., Berlin, Y., Marusic, R., et al. (1971). Structure of Boromycin. Helv. Chim. Acta 54, 1709–1713. doi: 10.1002/hlca.19710540624
Ekland, E. H., Schneider, J., Fidock, D. A. (2011). Identifying Apicoplast-Targeting Antimalarials Using High-Throughput Compatible Approaches. FASEB J. 25, 3583–3593. doi: 10.1096/fj.11-187401
Fatih, F. A., Staines, H. M., Siner, A., Ahmed, M. A., Woon, L. C., Pasini, E. M., et al. (2013). Susceptibility of Human Plasmodium Knowlesi Infections to Anti-Malarials. Malar. J. 12, 1–7. doi: 10.1186/1475-2875-12-425
Gaillard, T., Dormoi, J., Madamet, M., Pradines, B. (2016a). Macrolides and Associated Antibiotics Based on Similar Mechanism of Action Like Lincosamides in Malaria. Malar. J. 15, 1–11. doi: 10.1186/s12936-016-1114-z
Gaillard, T., Madamet, M., Pradines, B. (2015). Tetracyclines in Malaria. Malar. J. 14, 445. doi: 10.1186/s12936-015-0980-0
Gaillard, T., Madamet, M., Tsombeng, F. F., Dormoi, J., Pradines, B. (2016b). Antibiotics in Malaria Therapy: Which Antibiotics Except Tetracyclines and Macrolides may be Used Against Malaria? Malar. J. 556, 1–10. doi: 10.1186/s12936-016-1613-y
Gardiner, D. L., Trenholme, K. R. (2015). Plasmodium Falciparum Gametocytes: Playing Hide and Seek. Ann. Transl. Med. 3, 45. doi: 10.3978/j.issn.2305-5839.2015.01.23
Grande, E. N., Sanchez, A. R., Sanchez, F. R. (1956). The Treatment of Malaria With Tetracycline. Antibiot. Med. Clin. Ther. 3, 193–196.
Grignard, L., Shah, S., Chua, T. H., William, T., Drakeley, C. J., Fornace, K. M. (2019). Natural Human Infections With Plasmodium Cynomolgi and Other Malaria Species in an Elimination Setting in Sabah, Malaysia. J. Inf. Dis. 220, 1946–1949. doi: 10.1093/infdis/jiz397
Held, J., Zanger, P., Issifou, S., Kremsner, P. G., Mordmüller, B. (2010). In Vitro Activity of Tigecycline in Plasmodium Falciparum Culture-Adapted Strains and Clinical Isolates From Gabon. Int. J. Antimicrob. Agents 35, 587–589. doi: 10.1016/j.ijantimicag.2010.02.003
Hess, F. I., Kilian, A., Söllner, W., Nothdurft, H. D., Pröll, S., Löscher, T. (1995). Plasmodium Falciparum and Plasmodium Berghei: Effect of Magnesium on the Development of Parasitemia. Exp. Parasitol. 80, 186–193. doi: 10.1006/expr.1995.1023
Hu, T. H., Rosli, N., Mohamad, D. S. A., Kadir, K. A., Ching, Z. H., Chai, Y. H., et al. (2021). A Comparison of the Clinical, Laboratory and Epidemiological Features of Two Divergent Subpopulations of Plasmodium Knowlesi. Sci. Rep. 11, 20117. doi: 10.1038/s41598-021-99644-8
Hütter, R., Keller-Schien, W., Knüsel, F., Prelog, V., Rodgers, G. C., Jr., Suter, P., et al. (1967). Stoffwechselprodukte Von Mikroorganismen. 57. Mitteilung. Boromycin. Helv. Chim. Acta 50, 1533–1539. doi: 10.1002/hlca.19670500612
Imboden, C. A., Cooper, W. C., Coatney, G. R., Jeffrey, G. M. (1950). Studies in Human Malaria. XXIX. Trials of Aureomycin, Chloramphenicol, Penicillin, and Dihydrostreptomycin Against the Chesson Strain of Plasmodium Vivax. J. Natl. Malar. Soc. 9, 377–380.
Ishiyama, A., Iwatsuki, M., Namatame, M., Nishihara-Tsukashima, A., Sunazuka, T., Takahashi, Y., et al. (2011). Borrelidin, a Potent Antimalarial: Stage-Specific Inhibition Profile of Synchronized Cultures of Plasmodium Falciparum. J. Antibiot. 64, 381–384. doi: 10.1038/ja.2011.6
Klingel, W. (1980). Bor in Biologie, Medizin Und Pharmazie: Physiologische Wirkungen Und Anwendung Von Borverbindungen (Braunschweig: Springer-Verlag), 902.
Koehne, E., Kreidenweiss, A., Adegbite, B. R., Manego, R. Z., McCall, M. B. B., Mombo-Ngoma, G., et al. (2021). In Vitro Activity of Eravacycline, a Novel Synthetic Halogenated Tetracycline, Against the Malaria Parasite Plasmodium Falciparum. J. Glob. Antimicrob. Resist. 24, 93–97. doi: 10.1016/j.jgar.2020.11.024
Kohno, J., Kawahata, T., Otake, T., Morimoto, M., Mori, H., Ueba, N., et al. (1996). Boromycin, an Anti-HIV Antibiotic. Biosci. Biotechnol. Biochem. 60, 1036–1037. doi: 10.1271/bbb.60.1036
Lelièvre, J., Almela, M. J., Lozano, S., Miguel, C., Franco, V., Leroy, D., et al. (2012). Activity of Clinically Relevant Antimalarial Drugs on Plasmodium Falciparum Mature Gametocytes in an ATP Bioluminescence “Transmission Blocking” Assay. PloS One 7, 1–8. doi: 10.1371/journal.pone.0035019
Lin, J. T., Lon, C., Spring, M. D., Sok, S., Chann, S., Ittiverakul, M., et al. (2017). Single Dose Primaquine to Reduce Gametocyte Carriage and Plasmodium Falciparum Transmission in Cambodia: An Open-Label Randomized Trial. PloS One 12, e0168702. doi: 10.1371/journal.pone.0168702
Mehlotra, R. K., Blankenship, D., Howes, R. E., Rakotomanga, T. A., Ramiranirina, B., Ramboarina, S., et al. (2017). Long-Term In Vitro Culture of Plasmodium Vivax Isolates From Madagascar Maintained in Saimiri Boliviensis Blood. Malar. J. 16, 1–13. doi: 10.1186/s12936-017-2090-7
Mendes, A. M., Albuquerque, I. S., Machado, M., Pissarra, J., Meireles, P., Prudêncio, M. (2017). Inhibition of Plasmodium Liver Infection by Ivermectin. Antimicrob. Agents Chemother. 61, 1–8. doi: 10.1128/AAC.02005-16
Milner, D. A., Jr., Whitten, R. O., Kamiza, S., Carr, R., Liomba, G., Dzamalala, C., et al. (2014). The Systemic Pathology of Cerebral Malaria in African Children. Front. Cell Infect. Microbiol. 4, 1–13. doi: 10.3389/fcimb.2014.00104
Mishra, S. J., Liu, W., Beebe, K., Banerjee, M., Kent, C. N., Munthali, V., et al. (2021). The Development of Hsp90β-Selective Inhibitors to Overcome Detriments Associated With Pan-Hsp90 Inhibition. J. Med. Chem. 11, 1545–1557. doi: 10.1021/acs.jmedchem.0c01700
Moon, R. W., Hall, J., Rangkuti, F., Ho, Y. S., Almond, N., Mitchell, G. H., et al. (2013). Adaptation of the Genetically Tractable Malaria Pathogen Plasmodium Knowlesi to Continuous Culture in Human Erythrocytes. Proc. Natl. Acad. Sci. U. S. A. 110, 531–536. doi: 10.1073/pnas.1216457110
Moreira, W., Aziz, D. B., Dick, T. (2016). Boromycin Kills Mycobacterial Persisters Without Detectable Resistance. Front. Microbiol. 7, 199. doi: 10.3389/fmicb.2016.00199
Noedl, H., Bronnert, J., Yingyuen, K., Attlmayr, B., Kollaritsch, H., Fukuda, M. (2005). Simple Histidine-Rich Protein 2 Double-Site Sandwich Enzyme-Linked Immunosorbent Assay for Use in Malaria Drug Sensitivity Testing. Antimicrob. Agents Chemother. 49, 5–8. doi: 10.1128/AAC.49.8.3575-3577.2005
Nsanzabana, C. (2019). Resistance to Artemisinin Combination Therapies (ACTs): Do Not Forget the Partner Drug! Trop. Med. Infect. Dis. 4, 26. doi: 10.3390/tropicalmed4010026
Nuzyra. Available at: https://www.nuzyra.com/hcp/ (Accessed October 15, 2021).
Otoguro, K., Ui, H., Ishiyama, A., Kobayashi, M., Togashi, H., Takahashi, Y., et al. (2003). In Vitro and In Vivo Antimalarial Activities of a Non-Glycosidic 18-Membered Macrolide Antibiotic, Borrelidin, Against Drug-Resistant Strains of Plasmodia. J. Antibiot. 56, 727–729. doi: 10.7164/antibiotics.56.727
Pache, W., Zaehner, H. (1969a). Metabolic Products of Microorganisms. Arch. für. Mikrobiol. 67, 156–165. doi: 10.1007/BF00409681
Pache, W., Zaehner, H. (1969b). Stoffwechselprodukte Von Mikroorganismen. Arch. Mikrobiol. 288, 281–288. doi: 10.1007/BF00412060
Pluijm, R. W., Imwong, M., Chau, N. H., Hoa, N. T., Thuy-Nhien, N. T., Thanh, N. V., et al. (2019). Determinants of Dihydroartemisinin-Piperaquine Treatment Failure in Plasmodium Falciparum Malaria in Cambodia, Thailand, and Vietnam: A Prospective Clinical, Pharmacological, and Genetic Study. Lancet Inf. Dis. 19, 952–961. doi: 10.1016/S1473-3099(19)30391-3
Portugaliza, H. P., Miyazaki, S., Geurten, F. J. A., Pell, C., Rosanas-Urgell, A., Janse, C. J., et al. (2020). Artemisinin Exposure at the Ring or Trophozoite Stage Impacts Plasmodium Falciparum Sexual Conversion Differently. Elife 9, e60058. doi: 10.7554/eLife.60058
Prelog, V., Zaehner, H., Bickel, H. (1973). U.S. Patent No 584,215 (Ardsley, New York: Ciba-Geigy Corporation).
Quade, B. R., Ramírez-Hernández, A., Blanton, L. S. (2021). In Vitro Susceptibility of Rickettsia Species to Eravacycline, Omadacycline, and Tigecycline. Antimicrob. Agents Chemother. 65, e0066521. doi: 10.1128/AAC.00665-21
R Core Team. (2014). R: A Language and Environment for Statistical Computing (Vienna, Austria: R Foundation for Statistical Computing). Available at: http://www.R-project.org.
Recht, J., Ashley, E. A., White, N. J. (2018). Use of Primaquine and Glucose-6-Phosphate Dehydrogenase Deficiency Testing: Divergent Policies and Practices in Malaria Endemic Countries. PloS Negl. Trop. Dis. 12, e0006230. doi: 10.1371/journal.pntd.0006230
Ribaut, C., Berry, A., Chevalley, S., Reybier, K., Morlais, I., Parzy, D., et al. (2008). Concentration and Purification by Magnetic Separation of the Erythrocytic Stages of All Human Plasmodium Species. Malar. J. 7, 1–5. doi: 10.1186/1475-2875-7-45
Ritz, C., Streibig, J. C. (2005). Bioassay Analysis Using R. J. Stat. Softw. 12, 1–22. doi: 10.18637/jss.v012.i05
Rutledge, G. G., Böhme, U., Sanders, M., Reid, A. J., Cotton, J. A., Maiga-Ascofare, O., et al. (2017). Plasmodium Malariae and P. Ovale Genomes Provide Insights Into Malaria Parasite Evolution. Nature 542, 101–104. doi: 10.1038/nature21038
Siciliano, G., Alano, P. (2015). Enlightening the Malaria Parasite Life Cycle: Bioluminescent Plasmodium in Fundamental and Applied Research. Front. Microbiol. 6, 1–8. doi: 10.3389/fmicb.2015.00391
Sinden, R. E. (2017). Developing Transmission-Blocking Strategies for Malaria Control. PloS Pathog. 13, 1–12. doi: 10.1371/journal.ppat.1006336
Straimer, J., Gnädig, N. F., Witkowski, B., Amaratunga, C., Duru, V., Ramadani, A. P., et al. (2015). K13-Propeller Mutations Confer Artemisinin Resistance in Plasmodium Falciparum Clinical Isolates. Science 347, 428–431. doi: 10.1126/science.1260867
Tsutsui, A., Furuya, Y., Hirose, T., Kim, R., Masuma, R., Matsumoto, A., et al. (2010). Boromycin Derivatives: Synthesis and Antimalarial Activity In Vitro and In Vivo. Heterocycles 82, 289–295. doi: 10.3987/COM-10-S(E)55
Uddin, T., Mcfadden, G. I., Goodman, C. D. (2018). Validation of Putative Apicoplast-Targeting Drugs Using a Chemical Supplementation Assay in Cultured Human Malaria. Antimicrob. Agents Chemother. 62, e01161–e01117. doi: 10.1128/AAC.01161-17
United States Food and Drug Administration Drug Trials Snapshot: Seysara. Available at: https://www.fda.gov/drugs/drug-approvals-and-datab (Accessed October 15, 2021).
van Biljon, R., Niemand, J., van Wyk, R., Clark, K., Verlinden, B., Abrie, C., et al. (2018). Inducing Controlled Cell Cycle Arrest and Re-Entry During Asexual Proliferation of Plasmodium Falciparum Malaria Parasites. Sci. Rep. 8, 1–14. doi: 10.1038/s41598-018-34964-w
van Schalkwyk, D. A., Blasco, B., Davina, N. R., Liew, J. W. K., Amir, A., Lau, Y. L., et al. (2019). Plasmodium Knowlesi Exhibits Distinct In Vitro Drug Susceptibility Profiles From Those of Plasmodium Falciparum. Int. J. Parasitol. Drugs Drug Resist. 9, 93–99. doi: 10.1016/j.ijpddr.2019.02.004
van Schalkwyk, D. A., Moon, R. W., Blasco, B., Sutherland, C. J. (2017). Comparison of the Susceptibility of Plasmodium Knowlesi and Plasmodium Falciparum to Antimalarial Agents. J. Antimicrob. Chemother. 72, 3051–3058. doi: 10.1093/jac/dkx279
van Schalkwyk, D. A., Moon, R. W., Duffey, M., Leroy, D., Sutherland, C. J. (2021). Ex Vivo Susceptibility to New Antimalarial Agents Differs Among Human-Infecting Plasmodium Species. Int. J. Parasitol. Drugs Drug Resist. 17, 5–11. doi: 10.1016/j.ijpddr.2021.07.002
Wadi, I., Nath, M., Anvikar, A. R., Singh, P., Sinha, A. (2019). Recent Advances in Transmission-Blocking Drugs for Malaria Elimination. Future Med. Chem. 11, 3047–3088. doi: 10.4155/fmc-2019-0225
White, N. J. (2008). Plasmodium Knowlesi: The Fifth Human Malaria Parasite. Clin. Infect. Dis. 15, 172–173. doi: 10.1086/524889
World Health Organization. Guidelines for Malaria. Available at: https://www.who.int/publications/i/item/guidelines-for-malaria (Accessed Acessed December 10, 2021).
World Health Organization. (2021). World Malaria Report. Available at: https://www.who.int/teams/global-malaria-programme/reports/world-malaria-report-2021 (Accessed December 22, 2021).
WWARN K13 Genotype-Phenotype Study Group. (2019). Association of Mutation in the Plasmodium Falciparum Kelch13 Gene (Pf3D7_1343700) With Parasite Clearance Rates After Artemisinin-Based Treatments – A WWARN Individual Patient Data Meta-Analysis. BMC Med. 17, 1. doi: 10.1186/s12916-018-1207-3
Keywords: antibiotics, Plasmodium falciparum, Plasmodium knowlesi, tetracyclines, delayed death effect, boromycin, macrolides
Citation: de Carvalho LP, Groeger-Otero S, Kreidenweiss A, Kremsner PG, Mordmüller B and Held J (2022) Boromycin has Rapid-Onset Antibiotic Activity Against Asexual and Sexual Blood Stages of Plasmodium falciparum. Front. Cell. Infect. Microbiol. 11:802294. doi: 10.3389/fcimb.2021.802294
Received: 26 October 2021; Accepted: 27 December 2021;
Published: 14 January 2022.
Edited by:
Vipan Kumar, Guru Nanak Dev University, IndiaCopyright © 2022 de Carvalho, Groeger-Otero, Kreidenweiss, Kremsner, Mordmüller and Held. This is an open-access article distributed under the terms of the Creative Commons Attribution License (CC BY). The use, distribution or reproduction in other forums is permitted, provided the original author(s) and the copyright owner(s) are credited and that the original publication in this journal is cited, in accordance with accepted academic practice. No use, distribution or reproduction is permitted which does not comply with these terms.
*Correspondence: Jana Held, amFuYWhlbGRAaG90bWFpbC5kZQ==; amFuYS5oZWxkQHVuaS10dWViaW5nZW4uZGU=