- 1Laboratory of Sustainable Animal Environment, Graduate School of Agricultural Science, Tohoku University, Osaki, Japan
- 2Department of Biochemistry, Faculty of Science, Federal University Oye-Ekiti, Oye-Ekiti, Ekiti State, Nigeria
- 3Department of Biochemistry, School of Science, Federal University of Technology, Akure, Nigeria
Toxoplasma gondii chronically infects the brain as latent cysts containing bradyzoites and causes various effects in the host. Recently, the molecular mechanisms of cyst formation in the mouse brain have been elucidated, but those in the human brain remain largely unknown. Here, we show that abnormal glutamine metabolism caused by both interferon-γ (IFN-γ) stimulation and T. gondii infection induce cyst formation in human neuroblastoma cells regardless of the anti-T. gondii host factor nitric oxide (NO) level or Indoleamine 2,3-dioxygenase-1 (IDO1) expression. IFN-γ stimulation promoted intracellular glutamine degradation in human neuronal cells. Additionally, T. gondii infection inhibited the mRNA expression of the host glutamine transporters SLC38A1 and SLC38A2. These dual effects led to glutamine starvation and triggered T. gondii stage conversion in human neuronal cells. Furthermore, these mechanisms are conserved in human iPSC-derived glutamatergic neurons. Taken together, our data suggest that glutamine starvation in host cells is an important trigger of T. gondii stage conversion in human neurons.
Introduction
Toxoplasma gondii is an obligate intracellular protozoan parasite that can infect the nucleated cells of all warm-blooded animals (Boothroyd, 2009; Dubey, 2010); it is estimated that one-third of the world’s population has already been infected (El-On and Peiser, 2003). Once T. gondii parasites enter the host, they spread the infection throughout the entire body through the bloodstream by hijacking host immune cells such as macrophages (intracellular parasites) (Bierly et al., 2008; Coombes et al., 2013) or free tachyzoites (extracellular parasites) (Unno et al., 2008). T. gondii in many types of tissues grow as tachyzoites, which is the rapidly multiplying form in the acute phase of infection (Dubey, 2009); however, parasite infection in specific organs such as the brain or muscle tissues leads to parasite stage conversion into bradyzoites, which is the slowly multiplying form in the chronic phase of infection that remains throughout the host’s life (Robert-Gangneux and Darde, 2012; Watts et al., 2015). Although anti-T. gondii drugs such as pyrimethamine, sulfadiazine, and atovaquone effectively inhibit the number of tachyzoites, they carry the potential risk of inducing parasite stage conversion to the chronic phase of infection (Ferguson et al., 1994; Gormley et al., 1998; Alday and Doggett, 2017). Additionally, there are no curative drugs that can eliminate bradyzoites. Infected immunocompromised people and fetuses are particularly susceptible to serious symptoms, such as toxoplasmosis, hydrocephalus, and chorioretinitis (Frenkel and Remington, 1980; Montoya and Remington, 2008). T. gondii-infected healthy people are generally asymptomatic; however, recently it has been revealed that chronic infection with T. gondii is a potential cause of various diseases such as depression (Sutterland et al., 2015; Cheng et al., 2020). Therefore, it is important to gain an understanding of the mechanism of T. gondii stage conversion to chronic infection.
T. gondii bradyzoite differentiation or cyst formation induction models using artificial stress stimulation such as high or low pH and heat shock have been established (Weiss et al., 1995; Lyons et al., 2002), and many kinds of parasite genes related to stage conversion and the molecular mechanisms involved have been clarified (Radke et al., 2013; Waldman et al., 2020). For example, T. gondii surface antigen 1 (SAG1), a major surface protein of tachyzoites, is involved in cell adhesion and invasion. SAG1 is a tachyzoite-specific marker because its expression is not detected in bradyzoites (Kasper, 1989). T. gondii bradyzoite antigen 1 (BAG1) is a bradyzoite-specific marker (Tomavo et al., 1991), and cyst wall markers [e.g., bradyzoite-specific cyst-wall protein 1 (CST1)], which are markers of bradyzoite differentiation, have also been identified (Zhang and Smith, 1995). Thus, various stage-specific markers have been revealed, and used to confirm parasite differentiation (Mayoral et al., 2020).
Because T. gondii stage conversion is a cell-type-specific event, it has been suggested that some host factors play an important role in its induction (Lüder and Rahman, 2017). Mice have been used to reveal the physiological stimulations that play an important role in the induction of bradyzoite differentiation or cyst formation; these studies identified some key host factors that induce parasite stage conversion. For example, treatment with mitochondrial inhibitors such as oligomycin and antimycin A induced bradyzoite differentiation in murine bone marrow-derived macrophages (BMDMs) (Bohne et al., 1994). Cell division autoantigen 1 (CDA-1), which is involved in cell cycle progression, has been shown to trigger bradyzoite differentiation and cyst formation in murine skeletal muscle tissue and human fibroblasts (Radke et al., 2006; Swierzy and Lüder, 2015). Inflammatory cytokines, such as interferon-γ (IFN-γ) and tumor necrosis factor-α (TNF-α), have been shown to be important for the induction of parasite stage conversion in mice (Yap et al., 1998; Tobin et al., 2010). For instance, IFN-γ-dependent nitric oxide (NO) production led to stress, including arginine starvation, which induced parasite stage conversion (Bohne et al., 1994; Fox et al., 2004). However, these mechanisms are not common to all cell types or host types; for example, IFN-γ-dependent induction of bradyzoite formation in human monocytes and human foreskin fibroblasts (HFFs) has not been observed (Bohne et al., 1993; Soête et al., 1994). Thus, the key host factors involved in inducing bradyzoite formation in human cells remain largely unknown.
In the present study, we found that IFN-γ stimulation induced bradyzoite formation in human neuronal cells. We further found that the effects of both IFN-γ-dependent intracellular glutamine degradation by glutaminase and T. gondii infection-dependent inhibition of glutamine transporter activation led to glutamine starvation in IFN-γ-stimulated, T. gondii-infected human neuroblastoma cells and human iPSC-derived glutamatergic neurons. Taken together, our data demonstrate that glutamine starvation is a key host factor for inducing T. gondii stage conversion in human neuronal cells.
Materials and Methods
Cell Lines and Parasites
T. gondii strains ME49 and Prugniaud were maintained in Vero cells in RPMI (Nacalai Tesque) supplemented with 2% heat-inactivated fetal bovine serum (FBS; JRH Bioscience), 100 U/mL penicillin, and 0.1 mg/mL streptomycin (Nacalai Tesque), as previously described (Ma et al., 2014). HFFs were maintained in RPMI (Nacalai Tesque) supplemented with 2% heat-inactivated FBS (JRH Bioscience), 100 U/mL penicillin, and 0.1 mg/mL streptomycin (Nacalai Tesque). IMR-32 cells were maintained in MEM (Nacalai Tesque) containing 10% heat-inactivated FBS, 1% non-essential amino acids (Nacalai Tesque), 100 U/mL penicillin, and 0.1 mg/mL streptomycin. A172 cells were maintained in DMEM (Nacalai Tesque) containing 10% heat-inactivated FBS, 100 U/mL penicillin, and 0.1 mg/mL streptomycin. U251-MG cells were maintained in EMEM (Nacalai Tesque) containing 10% heat-inactivated FBS, 100 U/mL penicillin, and 0.1 mg/mL streptomycin.
iPSC-Derived Glutamatergic Neurons
To prepare iPSC-derived glutamatergic neurons, ioGlutamatergic neurons were obtained from Abcam (ab259259). ioGlutamatergic neurons (5.7 × 104) were plated in a 24-well plate containing PDL-Geltrex-coated glass coverslips. On days 0–4, the neurons were cultured in complete glutamatergic neuron medium (CGNM) containing 1 μg/mL doxycycline (Sigma–Aldrich). The CGNM comprised 200 mL of Neurobasal medium (Thermo Fisher), 2 mL of GlutaMAX (100×) (Thermo Fisher), 25 μM 2-mercaptoethanol (Thermo Fisher), 4 mL of B27 (Thermo Fisher), 10 ng/mL NT3 (R&D), and 5 ng/mL BDNF (R&D). On days 5–14 days, the neurons were cultured in CGNM without doxycycline. The medium was changed every 48 h. After 12 days in culture, differentiation of iPSC-derived glutamatergic neurons was confirmed by morphology and gene expression of neuron or glutamatergic neuron-specific markers by use of microscopy and an immunofluorescence assay, respectively.
Reagents
Antibodies against TUBB3 (66375-1-lg), KGA (20170-1-AP), and VGLUT1 (55491-1-AP) were obtained from Proteintech. Salmon E monoclonal antibody for CST1 staining has been described previously (Tomita et al., 2013). The anti-GAP45 antibody was kindly gifted by Dr. Dominique Soldati-Favre (University of Geneva, Switzerland). Recombinant human IFN-γ (300-02) was obtained from Peprotech. Aminoguanidine hydrochloride (396494) was obtained from Sigma–Aldrich. 1-Methyl-DL-tryptophan (sc-224746) and KGA siRNA (sc-105592) were obtained from Santa Cruz Biotechnology, Inc. CB-839 (Cay-22038) was obtained from Cayman Chemical.
Bradyzoite Differentiation
Bradyzoite differentiation was confirmed by the gene expression pattern of SAG1 and BAG1, and by staining of the cyst wall protein CST1. HFFs, IMR-32, A172, U-251 MG cells, or iPSC-derived glutamatergic neurons were cultured and infected with T. gondii [multiplicity of infection (MOI) = 0.5]. For alkaline induction, the culture medium was changed to non-induction medium (pH 7.2) or induction medium (pH 8.2) at 2 h post-infection. For IFN-γ induction, the cells were stimulated with or without IFN-γ (10 ng/ml) at 2 h post-infection. Infected host cells were incubated for 24–72 h under low CO2 conditions. The cyst wall was stained by using an anti-CST1 antibody, and CST1-positive vacuoles were defined as bradyzoite differentiated parasitophorous vacuoles. Quantitative measurements of the cyst wall-positive vacuole rates were performed by counting at least 100 vacuoles per sample.
Quantitative RT-PCR
Total RNA from cells or parasites was extracted by using an RNA basic kit (Nippon genetics), and cDNA was synthesized by using Verso Reverse transcription (Thermo Fisher). Quantitative RT-PCR was performed with a Thermal Cycler Dice Real Time PCR System (Takara) using the Go-Taq Real-Time PCR system (Promega). The values were normalized to glyceraldehyde 3-phosphate dehydrogenase (GAPDH) for human cells or Tubulin or Actin for T. gondii in each sample. The primer sequences are listed in S1 Table.
Ion Chromatography (IC)
IMR-32 cells or A172 cells were plated in a 6-well plate, and then infected or non-infected cells were incubated for 48 h. The culture supernatants were then collected and filtrated through a 0.4-μm filter membrane before being analyzed. The concentrations of 11 major ions were measured by using the HIC-20A SUPER ion chromatography system (Shimazu).
Measurement of Kynurenine
The kynurenine concentration in the culture medium was measured by using the Ehrlich reagent method (Braun et al., 2005). Briefly, 70 μL of culture supernatant was mixed with 35 μL of 30% trichloroacetic acid, and centrifuged at 8,000 × g for 5 min. Then, 75 μL of the supernatant was added to an equal volume of Ehrlich reagent (0.8% p-dimethylaminobenzaldehyde in acetic acid) in a 96-well plate, and the absorbance was read at 490 nm. The values were determined by using a standard curve with defined concentrations of kynurenine (Sigma–Aldrich).
Measurement of Glutamine
IMR-32 cells or iPSC-derived glutamatergic neurons were plated in a 96-well plate, and then infected and non-infected cells were incubated for 24 or 48 h. The concentration of extracellular and intracellular glutamine was measured by using the Glutamine/Glutamate-Glo™ Assay (Promega) and a GloMax Navigator Microplate Luminometer (Promega) according to the manufacturer’s instructions.
Immunofluorescence Assays
HFFs, IMR-32, A172, and U-251 MG cells, or iPSC-derived glutamatergic neurons were cultured on glass coverslips and infected with T. gondii (MOI = 0.5) for the indicated time. The cells were then fixed in PBS containing 4% paraformaldehyde for 15 min at room temperature. Cells were permeabilized with PBS containing 0.1% Triton X-100 for 5 min, and then blocked with 2% FBS in PBS for 1 h at room temperature. Next, the cells were incubated with the indicated primary antibodies for 1 h at 37°C, followed by incubation with Alexa 488- or Alexa 594-conjugated secondary antibodies (Molecular Probes) and DAPI for 1 h at 37°C in the dark. Finally, coverslips were mounted onto glass slides with ProLong Glass Antifade Mountant (Invitrogen) and analyzed by using a BZ-X810 All-in-one Fluorescence Microscope (Keyence).
Inhibitor Treatment
IMR-32 cells were pre-treated with Nω-Propyl-L-Arginine hydrochloride (2 μM) or 1-methyl-DL-tryptophan (1 mM) for 3 h, and then infected with the parasite. The culture medium was changed 2 h post-infection, and fresh medium containing 50 ng/mL IFN-γ and Nω-Propyl-L-Arginine hydrochloride (2 μM) or 1-Methyl-DL-tryptophan (1 mM) was added for 24–72 h.
Plaque Assay
HFF cells were pre-treated with CB-839 (2 μM) for 3 h, and then infected with the parasite. The culture medium was changed 2 h post-infection, and fresh medium containing 50 ng/mL IFN-γ with or without CB-839 (2 μM) was added for 5 days. Cells were fixed with 4% paraformaldehyde for 30 min at room temperature, then washed with PBS and stained with 0.1% crystal violet (CV) for 10 min. Images were analyzed by using Image J.
Western Blot Analyses
Cells were lysed in a lysis buffer (0.5% Nonidet P-40, 150 mM NaCl, and 20 mM Tris-HCl, pH 7.5) containing a protease inhibitor cocktail (Roche). The cell lysates were separated by SDS-PAGE and transferred to polyvinylidene difluoride membranes (Immobilon-P, Millipore) and subjected to Western blot analyses as described previously (Bando et al., 2019).
siRNA Transfection and Parasite Infection
ioGlutamatergic neurons (5.7 × 104) were plated in a 24-well plate and developed to iPSC-derived glutamatergic neurons. Ten days after development, KGA siRNA was transfected by using Lipofectamine 2000 (Invitrogen, Carlsbad, CA) according to the manufacturer’s instructions. At 24 h post-lipofection, the wells were washed and then incubated for an additional 24 h. KGA siRNA-transfected, iPSC-derived glutamatergic neurons were infected with T. gondii (MOI = 0.5) for 2 h, and then stimulated with or without IFN-γ (10 ng/ml). Infected host cells were incubated for 24–72 h under low CO2 conditions.
Statistical Analyses
All statistical analyses were performed by using Excel (Microsoft) or Prism 8 (GraphPad). All experimental points and n values represent an average of three biological replicates (three independent experiments). The statistical significance of differences in mean values was analyzed by using an unpaired two-tailed Student’s t-test. p < 0.05 was considered statistically significant.
Results
IFN-γ Stimulation Induces Stage Conversion in Type II Strains of T. gondii in Human Neuroblastoma Cells
The physiological conditions that induce T. gondii stage conversion in human brain cells are unclear. Therefore, we tested the effect of IFN-γ on the expression of the bradyzoite-specific gene BAG1 or the tachyzoite-specific gene SAG1 by using the T. gondii Type II ME49 strain in the following human brain cell lines: astrocytoma cell line (A172), glioblastoma cell line (U-251 MG), and neuroblastoma cell line (IMR-32) (Figures 1A, B). Although alkaline stress (pH 8.2) induced BAG1 gene expression in all cell lines tested (Figure S1A), IFN-γ-dependent upregulation of BAG1 gene expression was observed in IMR-32 cells, but not in A172 or U-251 MG cells (Figure 1A). In addition, we found that SAG1 gene expression was downregulated in IMR-32 cells (Figure 1B). T. gondii cyst wall CST1 formation around bradyzoites in IMR-32 cells was found only in IFN-γ-stimulated cells (Figures 1C, D). These phenomena were also observed with the Type II T. gondii Prugniaud strain (Figures S1B, C). These results indicate that Type II T. gondii differentiate into bradyzoites and form a cyst wall in response to IFN-γ stimulation in human neuroblastoma cells.
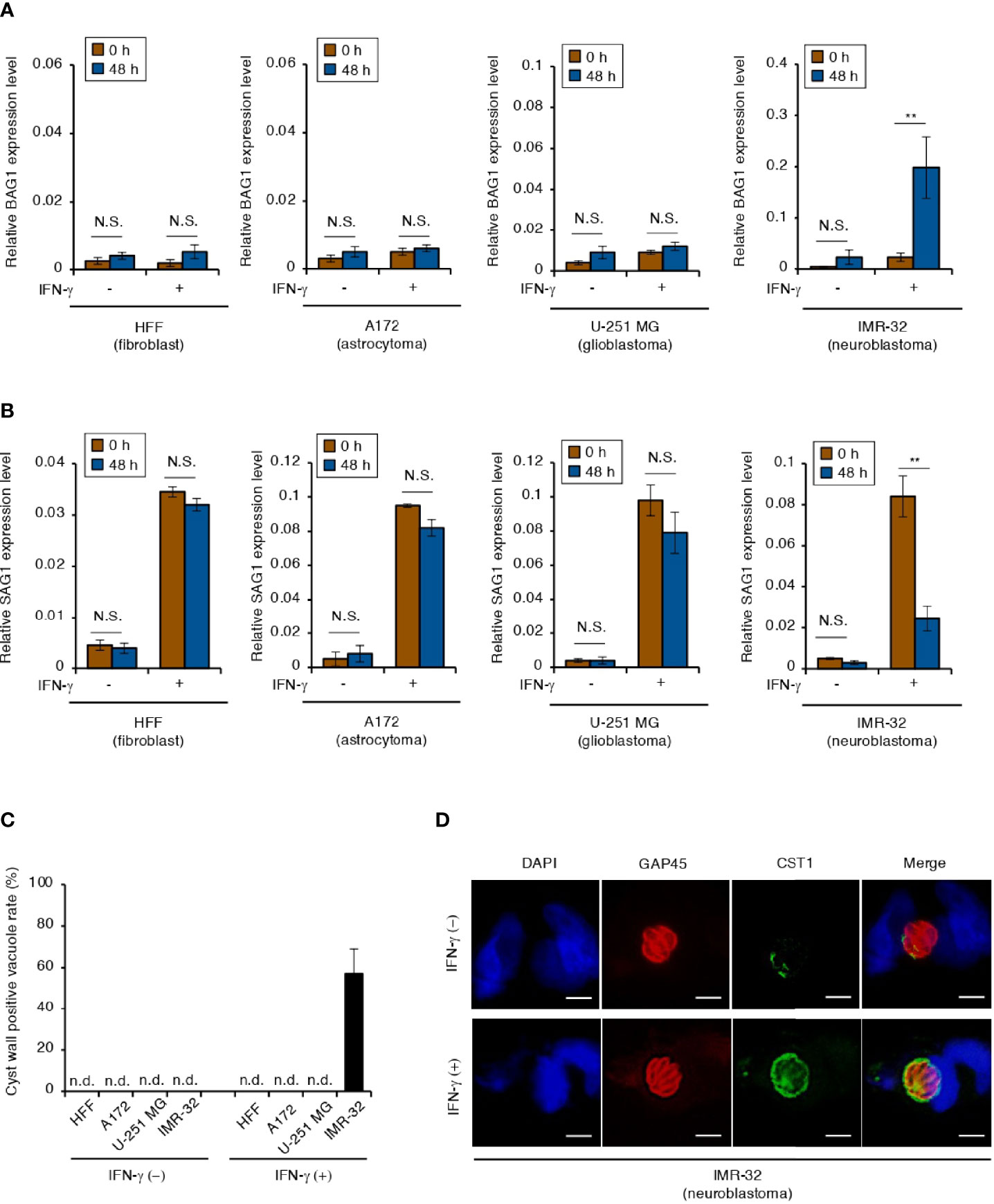
Figure 1 IFN-γ stimulation induces T. gondii stage conversion in human neuroblastoma cells. (A, B) HFFs, A172, U-251 MG, and IMR-32 cells infected with T. gondii ME49 were untreated or treated with IFN-γ and incubated for 0 or 48 h. Then, the BAG1 (A) or SAG1 (B) mRNA level was analyzed by use of quantitative RT-PCR. (C, D) IMR-32 cells infected with T. gondii strain ME49 were untreated or treated with IFN-γ. Cyst wall formation was assessed by IFA at 72 hours post-infection. (C) The percentage of CST1-positive vacuoles was determined. (D) Representative IFA images of T. gondii GAP45 (red) and CST1 (green); nuclei were stained with DAPI (blue). Scale bars correspond to 5 μm. Data are representative of three independent experiments. Indicated values are means ± SD (three biological replicates per group from three independent experiments) (A–C). **p < 0.01; N.S., not significant; n.d., not detected; Student’s t-test.
IFN-γ-Dependent Bradyzoite Differentiation in Human Neuroblastoma Cells Does Not Rely on NO Production
IFN-γ-induced bradyzoite differentiation in murine BMDMs depends on NO production (Bohne et al., 1994). In our study, an IFN-γ-dependent increase in NO production was detected in IMR-32 cells, but not in A172 or U251-MG cells (Figure 2A). Humans have three isoforms of nitric oxide synthase (NOS): inducible NOS (iNOS), epithelial NOS (eNOS), and neuronal NOS (nNOS) (Umar and van der Laarse, 2010). We therefore tested which NOS isoform is important for NO production in IFN-γ-stimulated IMR-32 cells (Figure 2B). We detected nNOS gene expression in IMR-32 cells but not in A172 cells in an IFN-γ-dependent manner (Figure 2B and Figure S2A). Then, we tested the effect of IFN-γ-dependent NO production on bradyzoite differentiation in IMR-32 cells by using Nω-propyl-L-arginine (L-NPA), a highly selective nNOS inhibitor (Figures 2C, D). Although L-NPA inhibited NO production in IFN-γ-stimulated IMR-32 cells (Figure 2C), there was no difference in BAG1 or SAG1 gene expression in the presence or absence of L-NPA (Figure 2D). These results suggest that NO production does not play a key role in the induction of bradyzoite differentiation in IFN-γ-stimulated human neuroblastoma cells.
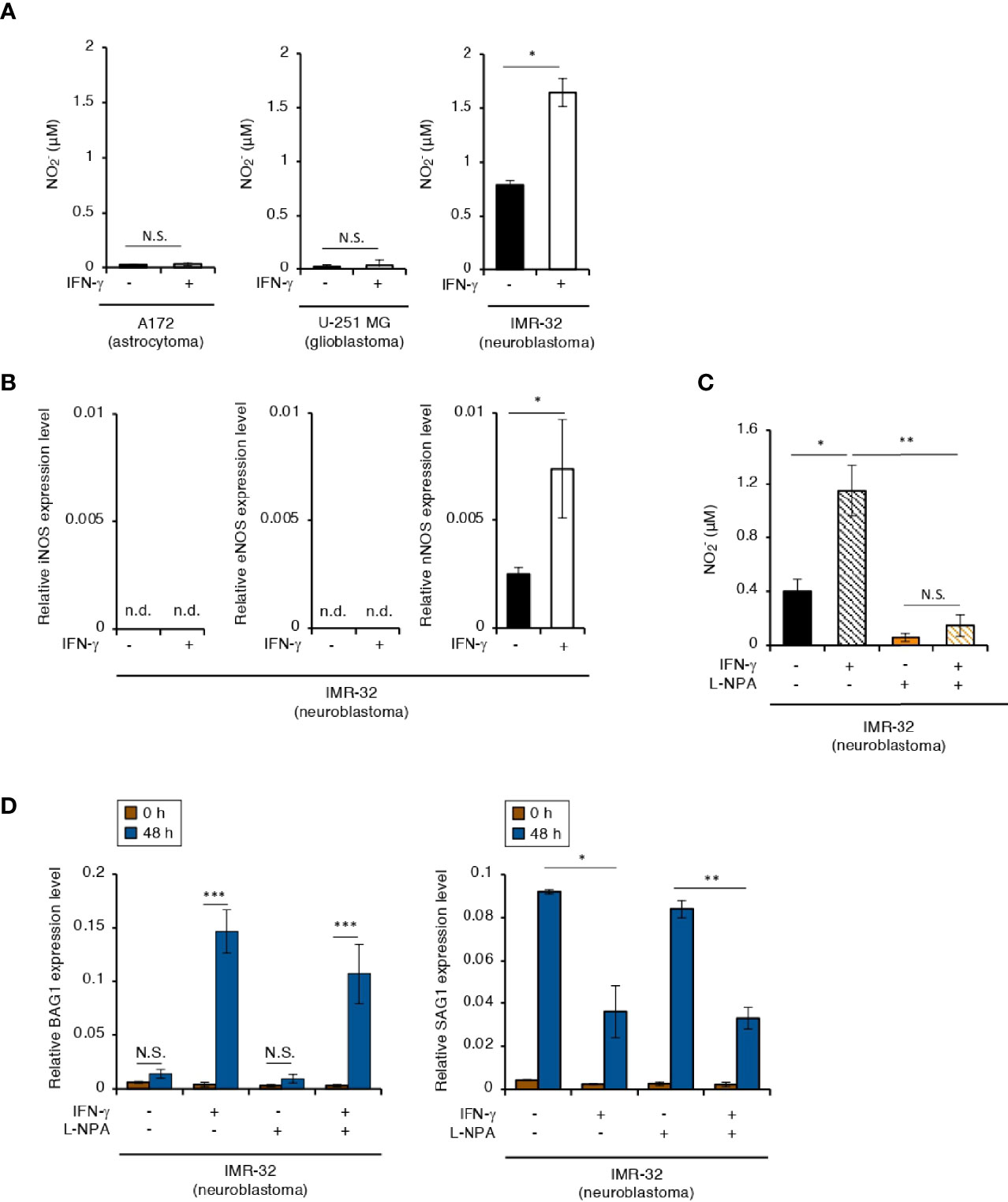
Figure 2 NO concentration plays no role in the IFN-γ-dependent T. gondii stage conversion in human neuroblastoma cells. (A) A172, U-251 MG, and IMR-32 cells were untreated or treated with IFN-γ and incubated for 0 or 48 h. The level of NO2 released into the culture supernatant was measured by IC. (B) IMR-32 cells were untreated or treated with IFN-γ and incubated for 24 h. The iNOS, eNOS, and nNOS mRNA levels were analyzed by use of quantitative RT-PCR. (C) IMR-32 cells were untreated or treated with IFN-γ and/or aminoguanidine and incubated for 48 h. The level of NO2 released into the culture supernatant was measured by IC. (D) IMR-32 cells infected with T. gondii ME49 were untreated or treated with IFN-γ and/or aminoguanidine and incubated for 48 h. Then, the BAG1 or SAG1 mRNA level were analyzed by use of quantitative RT-PCR. Values are means ± SD (three biological replicates per group from three independent experiments) (A–D). *p < 0.05, **p < 0.01, ***p < 0.001; N.S., not significant; Student’s t-test. n.d., not detected.
Indoleamine 2,3-Dioxygenase-1 (IDO1)-Dependent Tryptophan Starvation Does Not Influence IFN-γ-Induced Bradyzoite Differentiation in Human Neuroblastoma Cells
IFN-γ stimulation induces IDO1-dependent tryptophan starvation in various human cells (Bando et al., 2018a). Therefore, we tested the effect of IFN-γ stimulation on IDO1 expression in IMR-32 cells (Figures 3A, B). IDO1 gene expression was induced in IFN-γ-stimulated IMR-32 cells (Figure 3A). Furthermore, kynurenine, which is a metabolite in the IDO pathway, was detected in the culture medium of IFN-γ-stimulated IMR-32 cells (Figure 3B). Then, we examined the effect of IDO1 gene expression on bradyzoite differentiation in IMR-32 cells treated with 1-methyl-DL-tryptophan (1-DL-MT), which is an IDO1 inhibitor (Figures 3C, D). Although 1-DL-MT treatment inhibited IDO1 activity (Figure 3C), there was no difference in the BAG1 or SAG1 gene expression pattern (Figure 3D). These results suggest that IDO1-dependent tryptophan starvation stress does not affect bradyzoite differentiation in IFN-γ-stimulated human neuroblastoma cells.
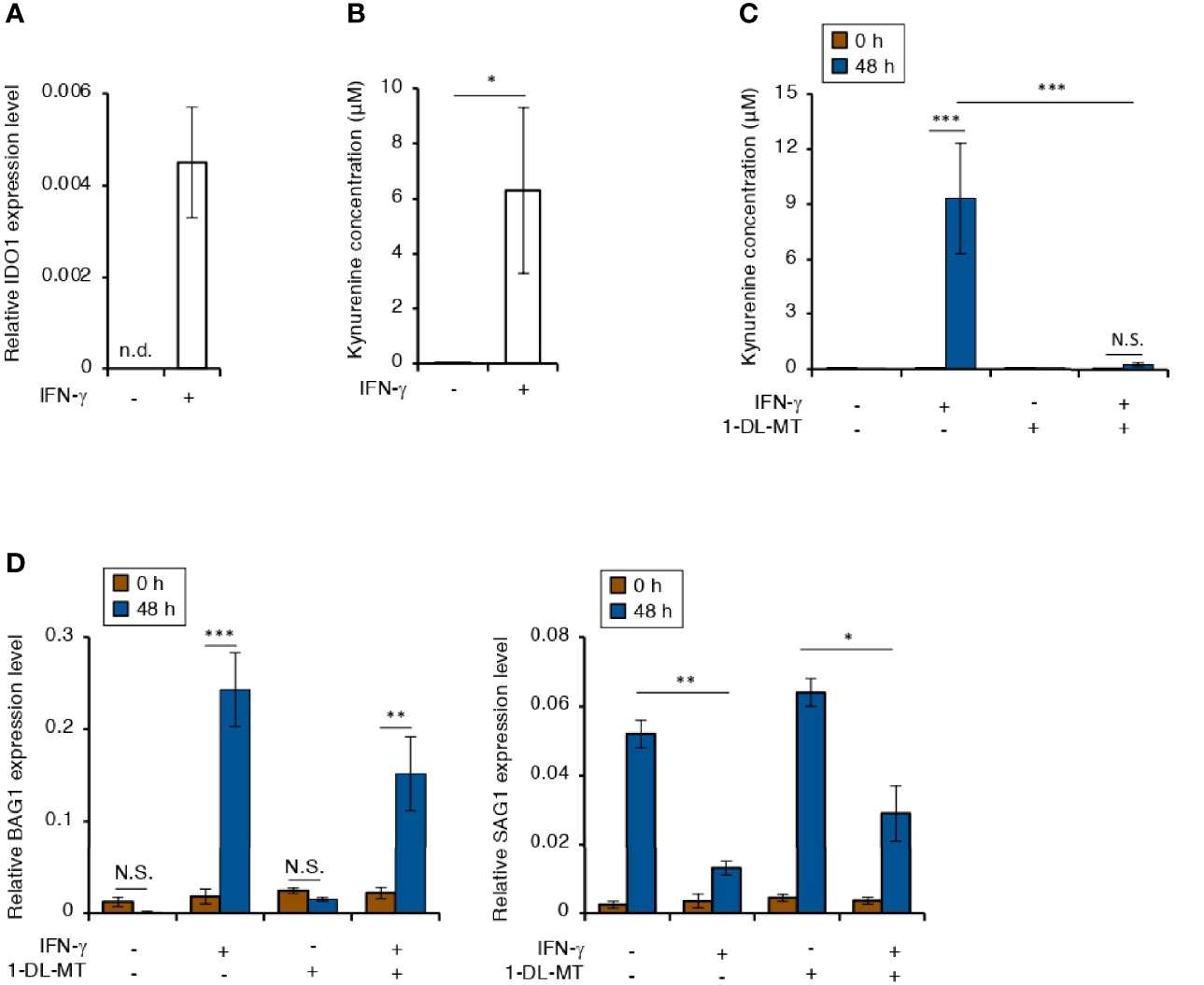
Figure 3 Tryptophan degradation plays no role in the IFN-γ-dependent T. gondii stage conversion in human neuroblastoma cells. (A) IMR-32 cells were untreated or treated with IFN-γ and incubated for 24 h. The IDO1 mRNA level was analyzed by use of quantitative RT-PCR. (B) IMR-32 cells were untreated or treated with IFN-γ and incubated for 48 h. The level of kynurenine released into the culture supernatant was measured by ELISA. (C) IMR-32 cells were untreated or treated with IFN-γ and/or 1-DL-MT and incubated for 48 h. The level of kynurenine released into the culture supernatant was measured by ELISA. (D) IMR-32 cells infected with T. gondii ME49 were untreated or treated with IFN-γ and/or 1-DL-MT and incubated for 0 or 48 h. Then, the BAG1 or SAG1 mRNA level was analyzed by use of quantitative RT-PCR. Values are means ± SD (three biological replicates per group from three independent experiments) (A–D). *p < 0.05, **p < 0.01, ***p < 0.001; N.S., not significant; Student’s t-test.
The Concentration of the Metabolic Product NH4 in the Culture Medium Is Increased by IFN-γ Stimulation in Human Neuroblastoma Cells
IFN-γ stimulation induced bradyzoite differentiation only in neuroblastoma cells (Figure 1A). Therefore, to elucidate the neuronal cell-specific response to IFN-γ stimulation, we compared the IFN-γ-inducible gene expression pattern of predicted-anti-T. gondii response genes in A172 and IMR-32 cells (Figures S2A, B). We did not find an IMR-32 cell-specific gene expression pattern other than that of nNOS (Figures S2A, B). Next, we compared the metabolic profiles by using ion chromatography (Figures S3A, B). We found that and were specifically high in the culture medium of IFN-γ-stimulated IMR-32 cells (Figures S3A, B). This IFN-γ-dependent nNOS gene expression and NO production confirmed our previous results (Figures 2A, B). Therefore, we focused on as a host factor candidate involved in the induction of bradyzoite differentiation and cyst formation.
The Intracellular Glutamine Concentration Is Limited in IFN-γ-Stimulated Human Neuroblastoma Cells by T. gondii Infection
NH4 accumulation is toxic to many cell types and activates several stress responses (Braissant et al., 2013; Wang et al., 2018). Hence, we examined the effect of a high concentration of NH4 in the culture medium on BAG1 or SAG1 gene expression in IMR-32 cells (Figure 4A). There was no difference in either gene expression pattern in IMR-32 cells cultured with or without a high concentration of NH4 (Figure 4A). It has been reported that most of the NH4 produced in the brain is derived from glutamine metabolism, which includes glutamine influx and glutaminase degradation (Bak et al., 2006). Therefore, we next examined the IFN-γ-dependent expression of the main glutamine transporters: solute carrier family 38 members A1 and A2 (SLC38A1 and SLC38A2) (Varoqui et al., 2000; González-González et al., 2005), in IMR-32 cells (Figure 4B). Although IFN-γ induced SLC38A1 and SLC38A2 gene expression in non-infected cells, this expression was inhibited in T. gondii-infected cells (Figure 4B), suggesting that T. gondii infection suppressed IFN-γ-dependent glutamine influx in IMR-32 cells. We next examined the glutamine dynamics in the culture medium (extracellular) (Figures 4C, D) and in the IMR-32 cells (intracellular) (Figure 4E). The extracellular glutamine concentration in the culture medium of IFN-γ-stimulated, T. gondii-infected IMR-32 cells was higher than that of IFN-γ-stimulated, non-infected IMR-32 cells (Figure 4C). Furthermore, the IFN-γ-induced NH4 increase in the culture medium was inhibited by T. gondii infection (Figure 4D). In contrast, the intracellular glutamine concentration in IFN-γ-stimulated, T. gondii-infected IMR-32 cells was significantly reduced compared with that in IFN-γ-stimulated, non-infected IMR-32 cells (Figure 4E). These results suggest that T. gondii infection leads to intracellular glutamine starvation via suppression of glutamine transporter activity in human neuroblastoma cells.
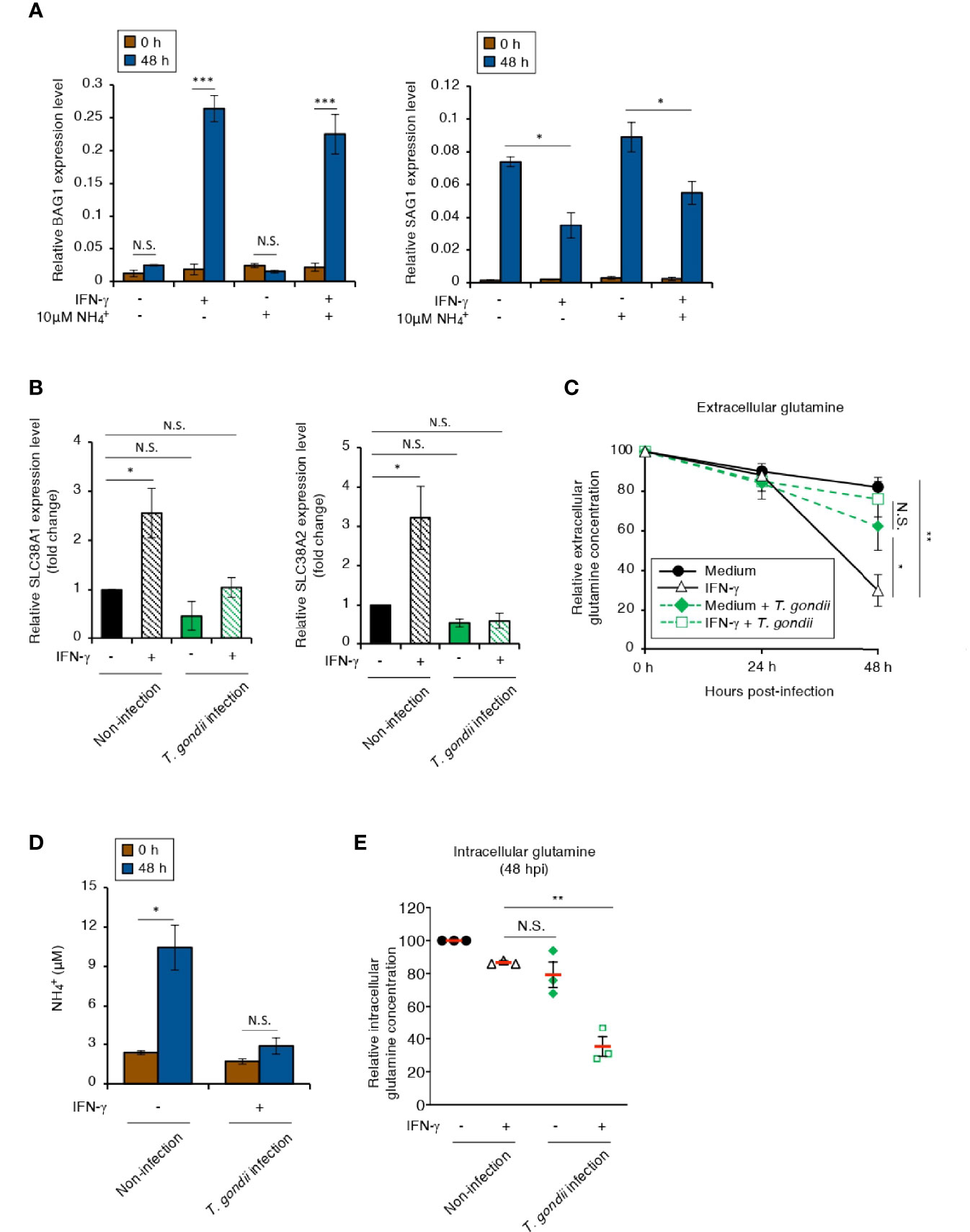
Figure 4 The intracellular glutamine concentration decreases in an IFN-γ-stimulated, T. gondii-infected human neuroblastoma cells. (A) IMR-32 cells infected with T. gondii ME49 were untreated or treated with IFN-γ and/or and incubated for 0 or 48 h. The BAG1 or SAG1 mRNA level was analyzed by use of quantitative RT-PCR. (B) IMR-32 cells uninfected or infected with T. gondii ME49 were untreated or treated with IFN-γ and incubated for 0 or 48 h. The SLC38A1 and SLC38A2 mRNA levels were analyzed by use of quantitative RT-PCR. (C) IMR-32 cells uninfected or infected with T. gondii ME49 were untreated or treated with IFN-γ. The glutamine level in the culture supernatant at 0, 24, and 48 h after parasite infection was measured by ELISA. (D) IMR-32 cells uninfected or infected with T. gondii ME49 were untreated or treated with IFN-γ and incubated for 0 or 48 h. The amount of released into the culture supernatant was measured by IC. (E) IMR-32 cells uninfected or infected with T. gondii ME49 were untreated or treated with IFN-γ. The level of intracellular glutamine at 48 h after parasite infection was measured by ELISA. Values are means ± SD (three biological replicates per group from three independent experiments) (A–E). *p < 0.05, **p < 0.01, ***p < 0.001; N.S., not significant; Student’s t-test.
Glutaminase Activity Directly Affects IFN-γ-Dependent T. gondii Stage Conversion in Human Neuroblastoma Cells
Glutamine serves as a bioenergetic substrate for T. gondii growth (Blume et al., 2009), suggesting the possibility that glutamine starvation triggers T. gondii stage conversion. We showed that bradyzoite differentiation is not induced in unstimulated IMR-32 cells (Figure 1); therefore, we hypothesized that pharmacological inhibition of IFN-γ-dependent glutamine degradation may prevent glutamine starvation and thereby suppress IFN-γ-dependent T. gondii stage conversion in IMR-32 cells. To test this hypothesis, we examined the effect of CB-839, a selective glutaminase inhibitor (Xu et al., 2019), on the intracellular glutamine concentration (Figure 5A). We found that CB-839 treatment restored the intracellular glutamine concentration in IFN-γ-stimulated, T. gondii-infected IMR-32 cells (Figure 5A). Next, we examined the effect of CB-839 treatment on the parasite by using a plaque assay, and found that CB-839 treatment had no effect on the parasite plaque size or number (Figures 5B, C). Then, we examined the effect of CB-839 treatment on IFN-γ-dependent bradyzoite differentiation (Figures 5D, E). We found that IFN-γ-induced BAG1 gene expression was suppressed in IFN-γ-stimulated IMR-32 cells treated with CB-839 (Figure 5D). Importantly, pretreating T. gondii with CB-839 did not affect the IFN-γ-induced BAG1 gene expression (Figure 5E), suggesting that the effect of CB-839 treatment was the result of the inhibition of the host glutaminase activity. Furthermore, we examined the effect of CB-839 on T. gondii cyst wall CST1 formation (Figures 5F, G). We found that CB-839 treatment inhibited IFN-γ-induced cyst wall CST1 formation (Figures 5F, G). These results suggest that IFN-γ-induced intracellular glutamine starvation triggers T. gondii stage conversion in human neuroblastoma cells.
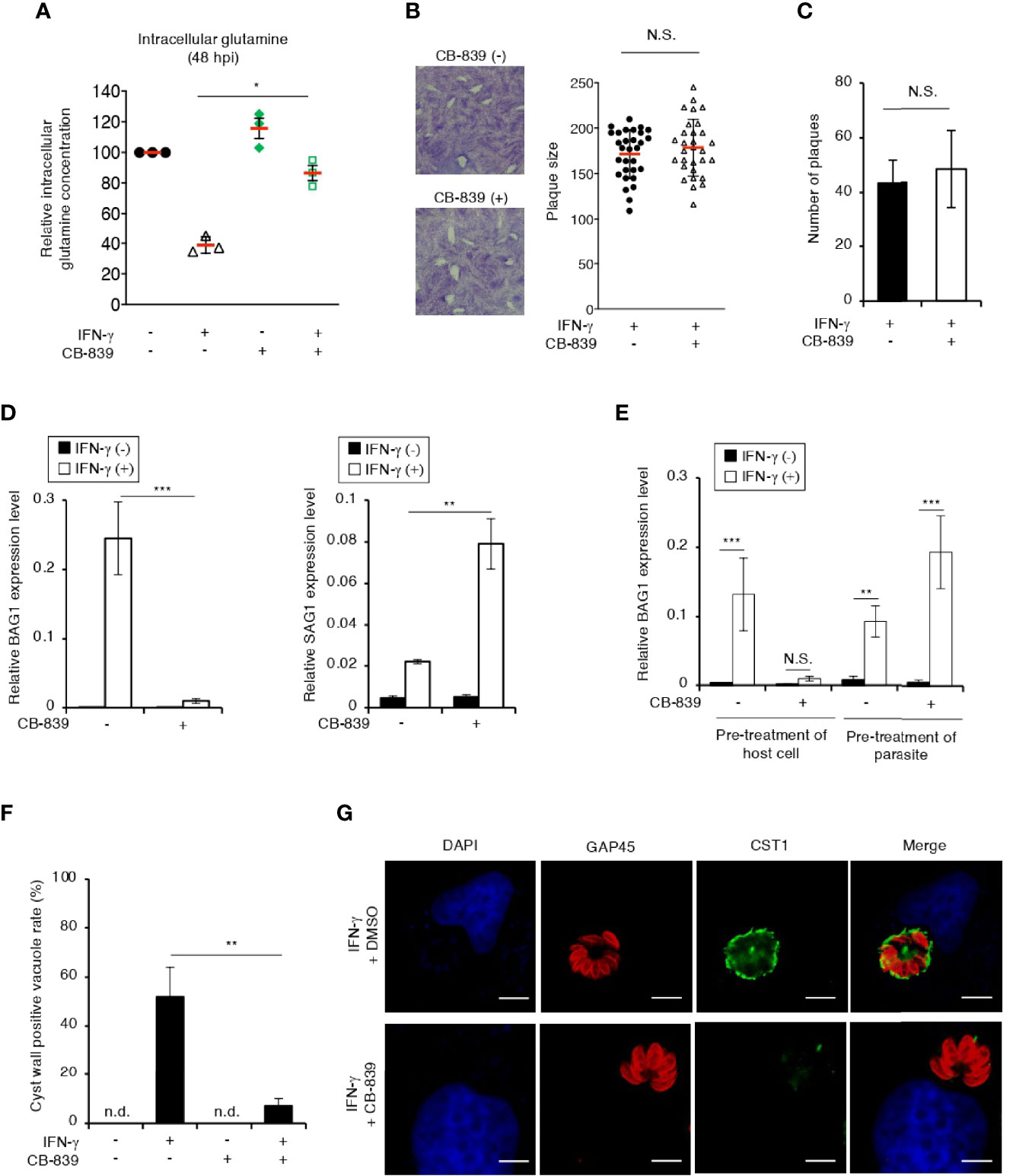
Figure 5 IFN-γ-dependent T. gondii stage conversion is suppressed by treatment with a glutaminase inhibitor in human neuroblastoma cells. (A) IMR-32 cells infected with T. gondii ME49 were untreated or treated with IFN-γ and/or CB-839. The level of intracellular glutamine at 48 h after parasite infection was measured by ELISA. (B, C) HFF cells infected with T. gondii ME49 were treated with IFN-γ and/or CB-839. Plaques were stained at 4 days post-infection. (B) Plaque sizes were measured by using the Image J software. (C) Plaque numbers of individual strains were counted. (D) IMR-32 cells infected with T. gondii ME49 were untreated or treated with IFN-γ and/or CB-839 and incubated for 0 or 48 h. The BAG1 or SAG1 mRNA level at 48 h after parasite infection was analyzed by use of quantitative RT-PCR. (E) IMR-32 cells or T. gondii ME49 were pretreated with CB-839 for 3 h, and then washed before infection. CB-839-pretreated or untreated IMR-32 cells were infected with pre-treated or untreated T. gondii ME49. The BAG1 mRNA levels at 48 h after parasite infection were analyzed by use of quantitative RT-PCR. (F, G) IMR-32 cells infected with T. gondii ME49 were treated with IFN-γ and/or CB-839. Cyst wall formation was assessed by IFA at 72 h post-infection. (F) The percentage of CST1-positive vacuoles was determined. (G) Representative IFA images of T. gondii GAP45 (red) and CST1 (green); nuclei were stained with DAPI (blue). Scale bars correspond to 5 μm. Data are representative of three independent experiments. Values are means ± SD (three biological replicates per group from three independent experiments) (A–F). *p < 0.01, **p < 0.01, ***p < 0.001; N.S., not significant; n.d., not detected; Student’s t-test.
T. gondii Stage Conversion Is Induced in Human iPSC-Derived Glutamatergic Neurons by IFN-γ-Dependent Intracellular Glutamine Starvation
Glutamatergic neurons produce glutamate, which is one of the most common excitatory neurotransmitters in the CNS (Zeng and Sanes, 2017). To confirm the glutamine starvation-induced T. gondii stage conversion in glutamatergic neurons, we differentiated glutamatergic neurons from human induced pluripotent stem cells (iPSCs) (Figures S4A, B). Axon elongation was evident in a healthy neuronal culture of iPSC-derived neurons 12 days after differentiation induction (Figure S4A). In addition, the neuronal marker tubulin beta 3 class III (TUBB3) (Ferreira and Caceres, 1992) and the specific biochemical marker of glutamatergic neurons and glutamatergic synapses vesicular glutamate transporter 1 (VGLUT1) (El Mestikawy et al., 2011) were detected in the iPSC-derived neurons (Figure S4B), suggesting that most of the iPSC-derived neurons differentiated into glutamatergic neurons. To confirm the effect of IFN-γ stimulation on T. gondii stage conversion, we examined the mRNA expression of the glutamine transporters SLC38A1 and SLC38A2 in iPSC-derived glutamatergic neurons (Figure 6A). The expression levels of SLC38A1 and SLC38A2 were upregulated by IFN-γ stimulation; however, they were inhibited by T. gondii infection in iPSC-derived glutamatergic neurons (Figure 6A). We next examined the effect of parasite infection on intracellular glutamine concentration in iPSC-derived glutamatergic neurons (Figure 6B). The intracellular glutamine concentration in IFN-γ-stimulated, T. gondii-infected iPSC-derived glutamatergic neurons was significantly reduced compared with that in IFN-γ-stimulated, non-infected iPSC-derived glutamatergic neurons (Figure 6B). Then, we examined the effect of CB-839 on the intracellular glutamine concentration and BAG1 and SAG1 gene expression in iPSC-derived glutamatergic neurons (Figures 6C, D). We found that CB-839 treatment restored the intracellular glutamine concentration in IFN-γ-stimulated, T. gondii-infected iPSC-derived glutamatergic neurons (Figure 6C). In addition, downregulation of BAG1 or upregulation of SAG1 gene expression were observed in response to CB-839 treatment compared to non-treatment in IFN-γ-stimulated, T. gondii-infected iPSC-derived glutamatergic neurons (Figure 6D). Finally, we examined the effect of CB-839 on T. gondii cyst wall CST1 formation in iPSC-derived glutamatergic neurons (Figures 6E, F). We found that CB-839 treatment inhibited IFN-γ-induced cyst wall CST1 formation (Figures 6E, F). Furthermore, inhibition of IFN-γ-induced T. gondii stage conversion was also observed in glutaminase knockdown iPSC-derived glutamatergic neurons (Figure S5). These results indicate that disruption of glutamine metabolism by both T. gondii infection and IFN-γ stimulation lead to glutamine starvation, triggering T. gondii stage conversion in human neuronal cells, including glutamatergic neurons (Figure 7).
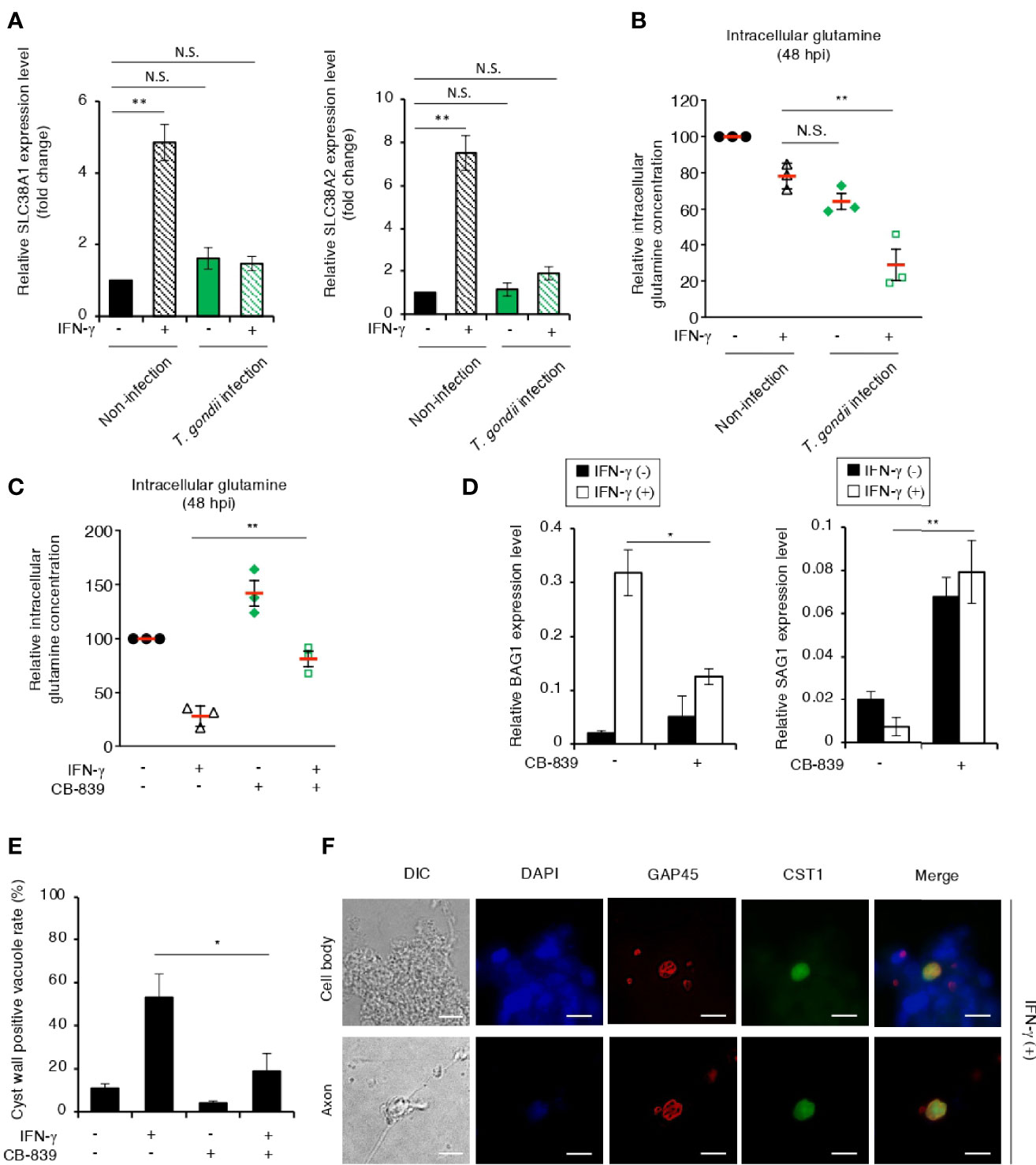
Figure 6 T. gondii stage conversion is accelerated by IFN-γ-dependent glutamine starvation in human iPSC-derived glutamatergic neurons. (A) Human iPSC-derived glutamatergic neurons uninfected or infected with T. gondii ME49 were untreated or treated with IFN-γ and incubated for 24 h. The SLC38A1 or SLC38A2 mRNA level was analyzed by use of quantitative RT-PCR. (B) Human iPSC-derived glutamatergic neurons uninfected or infected with T. gondii ME49 were untreated or treated with IFN-γ. The level of intracellular glutamine at 48 h after parasite infection was measured by ELISA. (C) Human iPSC-derived glutamatergic neurons infected with T. gondii ME49 were untreated or treated with IFN-γ and/or CB-839. The level of intracellular glutamine at 48 h after parasite infection was measured by ELISA. (D) Human iPSC-derived glutamatergic neurons infected with T. gondii ME49 were untreated or treated with IFN-γ and/or CB-839. The BAG1 or SAG1 mRNA level at 48 h after parasite infection was analyzed by use of quantitative RT-PCR. (E, F) Human iPSC-derived glutamatergic neurons infected with T. gondii ME49 were treated with IFN-γ. Cyst wall formation in the cell bodies or axons was assessed by IFA at 72 h post-infection. (E) The percentage of CST1-positive vacuoles was determined. (F) Representative IFA images of T. gondii GAP45 (red) and CST1 (green); the nuclei were stained with DAPI (blue). Scale bars correspond to 10 μm. Data are representative of three independent experiments. Values are means ± SD (three biological replicates per group from three independent experiments) (A–E). *p < 0.05, **p < 0.01, N.S., not significant; Student’s t-test.
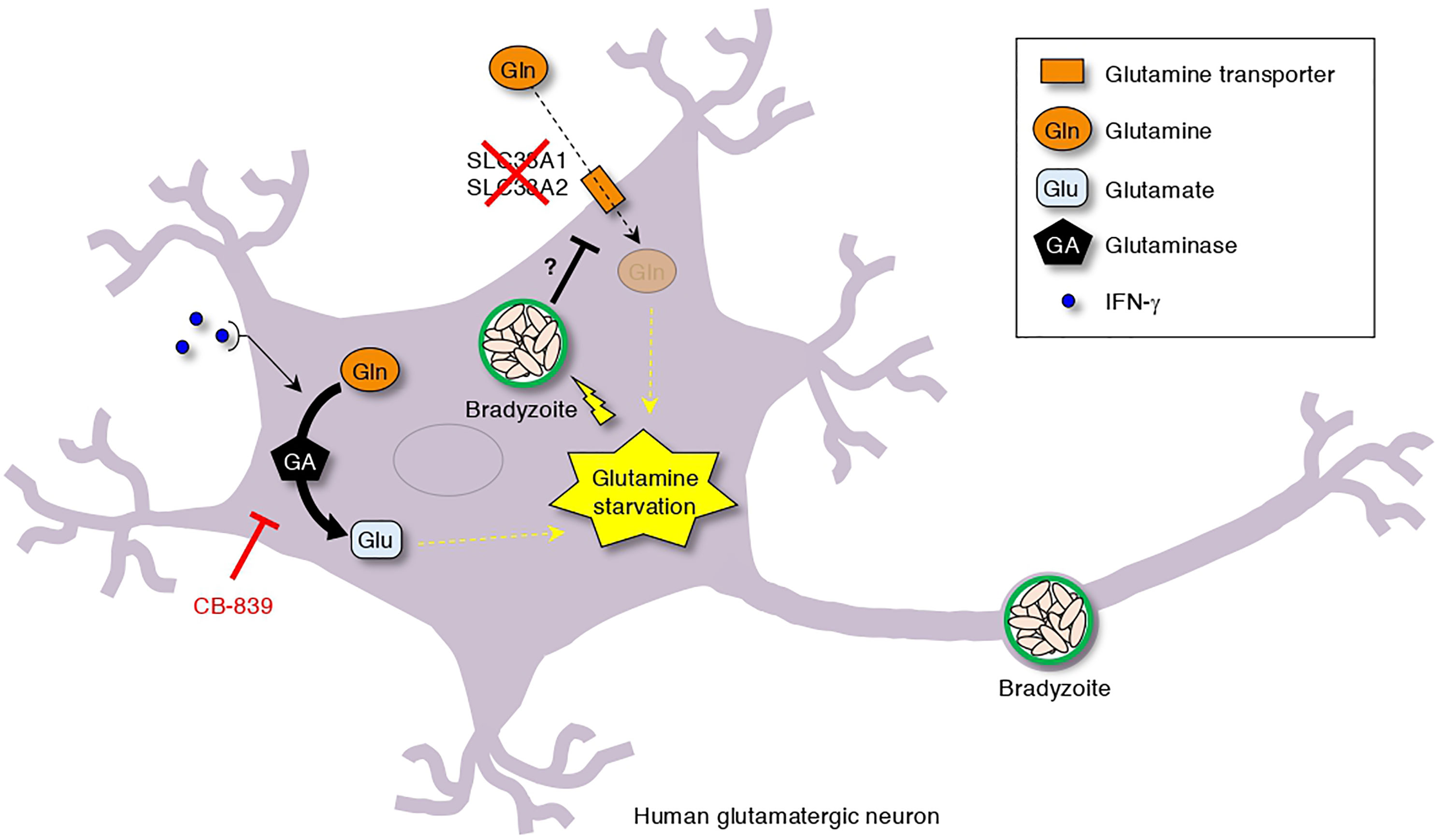
Figure 7 Simplified scheme of T. gondii stage conversion in human neuronal cells. The dual effect of T. gondii infection-dependent inhibition of glutamine transporter activation and IFN-γ-dependent glutamine degradation cause glutamine starvation, which triggers IFN-γ-dependent T. gondii stage conversion in human neuronal cells. As shown, CB-839 treatment can prevent IFN-γ-dependent T. gondii stage conversion.
Discussion
In the present study, we demonstrated that IFN-γ induces T. gondii stage conversion in human neuronal cells and that the mechanism involves glutamine starvation caused by IFN-γ-dependent activation of glutamine metabolism and T. gondii infection-induced inhibition of glutamine transporter activation in host cells.
Although IFN-γ is essential for the anti-T. gondii host immune response, its molecular mechanism is somewhat different in mice and humans (Ohshima et al., 2014; Bando et al., 2018a). For example, IFN-γ-inducible GTPase-dependent destruction of parasitophorous vacuoles plays an important role in the IFN-γ-dependent anti-T. gondii activity in mice (Yamamoto et al., 2012). However, IFN-γ-induced, IDO1-dependent tryptophan degradation has been shown to be important in the anti-T. gondii response in humans (Pfefferkorn et al., 1986; Bando et al., 2018a). These findings suggest that stress responses induced by parasites and caused by IFN-γ also differ between mice and humans. Previous reports have shown that the differences in T. gondii stress responses are connected to stage conversion induced by IFN-γ stimulation (Bohne et al., 1994; Tobin et al., 2010); however, the role of IFN-γ in T. gondii stage conversion in humans is unknown. In this study, we found that IFN-γ-dependent induction of stage conversion of Type II T. gondii occurred in neuroblastoma cells and human iPSC-derived glutamatergic neurons. Although previous studies report spontaneous cyst formation of T. gondii in human primary neurons and murine skeletal muscle cells (Halonen et al., 1996; Ferreira-Da-Silva Mda et al., 2009), we found that spontaneous stage conversion occurred in iPSC-derived glutamatergic neurons but not in IMR-32 cells, which is consistent with previous studies. However, the stage conversion rate was enhanced by IFN-γ stimulation in iPSC-derived glutamatergic neurons, suggesting that IFN-γ has a role in accelerating stage conversion in iPSC-derived glutamatergic neurons. Investigating the differences between iPSC-derived glutamatergic neurons and IMR-32 cells may be important for determining the cell-type specificity of T. gondii stage conversion or the mechanisms of spontaneous stage conversion.
In previous reports, NO-dependent arginine starvation was shown to be important for bradyzoite formation in mouse BMDMs (Fox et al., 2004). In contrast, in the present study, we found that IFN-γ-dependent T. gondii stage conversion in neuronal cells was independent of NO production. The reason for this contradiction may be that the IFN-γ-dependent NO production levels are different between mouse cells and human cells. The NO concentration in response to IFN-γ stimulation of mouse cells has been reported to reach 100 μM (Gomez-Marin, 2000), whereas that in human cells is less than 10 μM according to a previous study (Bando et al., 2018b) and this study. Therefore, it is likely that NO is produced in human neuronal cells upon IFN-γ stimulation, but not to a sufficient extent to induce T. gondii stage conversion. We also found that IDO1-dependent tryptophan degradation was not associated with the induction of IFN-γ-dependent T. gondii stage conversion in human neuronal cells. Although we showed that tryptophan degradation by IDO1 occurs in neuronal cells upon IFN-γ stimulation before T. gondii infection (pre-treatment conditions), we have previously shown that T. gondii inhibits IDO1 activity via the parasite virulence factor TgIST upon IFN-γ stimulation after T. gondii infection (post-treatment conditions) (Bando et al., 2018a). T. gondii must inhibit IFN-γ-dependent anti-T. gondii immune responses to survival, but it also must activate the IFN-γ-dependent host metabolism to change its life-stage. Therefore, the counterbalance of TgIST-dependent inhibition of IFN-γ responses might have importance for stage conversion in neuronal cells. In the future, we plan to examine the relationship between TgIST and stage conversion in neuronal cells.
Glutamate is a major neurotransmitter; hence, brain neurons have unique glutamine metabolism (Erecińska and Silver, 1990). Glutamate and are frequently produced by glutamine degradation in neurons. These metabolites are transported into astrocytes for glutamine synthesis by glutamine synthetase, to resupply neurons with glutamine through glutamine transporters. This process is called the glutamine-glutamate cycle (Bak et al., 2006). Previous reports have suggested a relationship between the disruption of the glutamine-glutamate cycle and T. gondii stage conversion. Indeed, it has been reported that the glutamine transporter function in astrocytes is inhibited by T. gondii infection (Lee et al., 2014), and that glutamate levels are elevated in the brain of mice with T. gondii chronic infection (David et al., 2016). However, the effects of T. gondii infection on neurons and glutamine metabolism are not well understood. In the present study, we showed that T. gondii infection impairs the intracellular glutamine concentration in neurons by suppressing the activation of glutamine transporters. Further investigations are needed to identify the exact virulence molecules and mechanisms involved in this phenomenon.
There are various types of neurons, including glutamatergic, GABAergic, and dopaminergic neurons; glutamatergic neurons are one of the major neurons responsible for fast excitatory transmission in the CNS (Baude et al., 2009; Langel et al., 2018). Previous studies have reported a relationship between GABAergic neurons and T. gondii infection (Brooks et al., 2015; Li et al., 2019), and have also reported the interaction between human neuronal-like cells and T. gondii bradyzoite formations (Tanaka et al., 2016; Passeri et al., 2016); however, few studies have focused on glutamatergic neurons. In the present study, we showed that T. gondii stage conversion is efficiently induced by IFN-γ stimulation in human iPSC-derived glutamatergic neurons, and that the mechanism involves glutamine starvation caused by glutaminase activation. Because iPSC-derived glutamatergic neurons have similar characteristics to their in vivo counterparts, our results suggest that glutamine starvation-induced T. gondii stage conversion occurs in vivo in humans. A previous report found that T. gondii mostly infects neurons and not astrocytes throughout the acute and chronic in vivo infection of mouse brain, and that cyst formation occurs not only in cell bodies but also in axons (Cabral et al., 2016). Our results are consistent with this previous report using mice. Together, these findings may suggest that the infection and pathology mechanisms in the brain are conserved between mice and humans even though the induction mechanisms of T. gondii stage conversion are different. The findings also demonstrate the importance of using model organisms and target hosts. In addition, we found that treatment with the glutaminase inhibitor CB-839 or glutaminase knockdown led to the inhibition of IFN-γ-dependent T. gondii stage conversion. These results support the idea that IFN-γ-dependent glutamine starvation caused by glutaminase-dependent glutamine degradation is essential for the induction of T. gondii stage conversion in human neuronal cells.
In summary, here we found a novel T. gondii stage conversion mechanism that involves IFN-γ-induced glutamine-dependent bradyzoite differentiation and cyst formation in human neuronal cells. We revealed that these effects were suppressed by treatment with a glutaminase inhibitor. Further elucidation of these effects may contribute to the development of advanced therapeutic strategies for the prevention of chronic infection of the human brain.
Data Availability Statement
The original contributions presented in the study are included in the article/Supplementary Material. Further inquiries can be directed to the corresponding author.
Author Contributions
HB and KK designed the research. HB and JO prepared the materials. HB, NW, and JO performed experiments using human cells. HB and YF set up the administrative experimental protocols for all experiments in this study. HB prepared T. gondii and performed experiments. HB and KK wrote the manuscript. KK supervised all experiments. All authors contributed to the article and approved the submitted version.
Funding
This study was funded by grants-in-aid for Scientific Research (B:17H03913) Young Scientists (19K16628), and Young Scientists (B) (17K15677) from the Ministry of Education, Culture, Science, Sports, and Technology (MEXT) of Japan, and by a Livestock Promotional Subsidy from the Japan Racing Association, the Uehara Memorial Foundation (J200002710), and The Morinaga Foundation for Health & Nutrition (AV602061).
Conflict of Interest
The authors declare that the research was conducted in the absence of any commercial or financial relationships that could be construed as a potential conflict of interest.
Publisher’s Note
All claims expressed in this article are solely those of the authors and do not necessarily represent those of their affiliated organizations, or those of the publisher, the editors and the reviewers. Any product that may be evaluated in this article, or claim that may be made by its manufacturer, is not guaranteed or endorsed by the publisher.
Acknowledgments
We thank Y. Yokoyama (Tohoku University) for technical assistance.
Supplementary Material
The Supplementary Material for this article can be found online at: https://www.frontiersin.org/articles/10.3389/fcimb.2021.788303/full#supplementary-material
Supplementary Figure 1 | Induction of T. gondii stage conversion under various experimental conditions. (A) HFFs, A172, U-251 MG, and IMR-32 cells infected with T. gondii ME49 were cultured in medium at a pH of 7.2 or 8.2. The BAG1 mRNA level at 48 h after parasite infection was analyzed by use of quantitative RT-PCR. (B, C) IMR-32 cells infected with T. gondii strain Prugniaud were untreated or treated with IFN-γ. Cyst wall formation was assessed by IFA at 72 hours post-infection. (B) The percentage of CST1-positive vacuoles was determined. (C) Representative IFA images of T. gondii GAP45 (red) and CST1 (green); nuclei were stained with DAPI (blue). Scale bars correspond to 5 μm. Data are representative of three independent experiments. Indicated values are means ± SD (three biological replicates per group from three independent experiments) (A, B). **p < 0.01; ***p < 0.001; N.S., not significant; n.d., not detected; Student’s t-test.
Supplementary Figure 2 | IFN-γ-induced gene expression pattern of predicted anti-T. gondii immune responses related to host factors in A172 and IMR-32 cells. (A, B) A172 and IMR-32 cells were untreated or treated with IFN-γ and incubated for 24 h. (A) Quantitative RT-PCR analysis of the indicated mRNA level was performed. (B) Heatmap of log-fold change (LFC) comparisons of differential gene expression in untreated and IFN-γ-treated A172 and IMR-32 cells. A relative decrease in LFC is indicated in blue and a relative increase is indicated in red. Values are means ± SD (three biological replicates per group from three independent experiments) (A). *p < 0.05, ***p < 0.001; N.S., not significant; n.d., not detected; Student’s t-test.
Supplementary Figure 3 | IFN-γ-induced metabolic profiles of T. gondii-infected A172 and IMR-32 cells. (A, B) A172 and IMR-32 cells were untreated or treated with IFN-γ and incubated for 48 h. (A) The levels of the indicated ions in the culture supernatant were measured by IC. (B) Heatmap of log-fold change (LFC) comparisons of differential ion concentrations in untreated and IFN-γ-treated A172 and IMR-32 cells. A relative decrease in LFC is indicated in blue, and an increase is indicated in red. Values are means ± SD (three biological replicates per group from three independent experiments) (A). *p < 0.05, **p < 0.01; N.S., not significant; Student’s t-test.
Supplementary Figure 4 | Confirmation of differentiation of human iPSC-derived glutamatergic neurons. (A, B) iPSCs were differentiated for 12 days. (A) Morphology of neurons and complex neural networks via axons. Scale bars, 100 μm. (B) The expression of the neuronal marker TUBB3 and the glutamatergic neuron marker VGLUT1 were assessed. Representative IFA images of TUBB3 (red) or VGLUT1 (green) in iPSC-derived glutamatergic neurons; nuclei were stained with DAPI (blue). Scale bars correspond to 50 μm.
Supplementary Figure 5 | The effect of glutaminase knockdown on IFN-γ-induced T. gondii stage conversion in human iPSC-derived glutamatergic neurons. (A) KGA siRNA was transfected into human iPSC-derived glutamatergic neurons. After 48 hours, the expression of KGA in human iPSC-derived glutamatergic neuron lysates was detected by Western blotting. (B, C) KGA siRNA was transfected into human iPSC-derived glutamatergic neurons, which were then infected with T. gondii ME49, and then left untreated or treated with IFN-γ. (B) The BAG1 or SAG1 mRNA level at 48 h after parasite infection was analyzed by use of quantitative RT-PCR. (C) The percentage of CST1-positive vacuoles in the cell bodies or axons was determined by IFA at 72 h post-infection. Values are means ± SD (three biological replicates per group from three independent experiments) (B, C). *p < 0.05, ***p < 0.001; N.S., not significant; Student’s t-test.
References
Alday, P. H., Doggett, J. S. (2017). Drugs in Development for Toxoplasmosis: Advances, Challenges, and Current Status. Drug Des. Devel. Ther. 11, 273–293. doi: 10.2147/dddt.S60973
Bak, L. K., Schousboe, A., Waagepetersen, H. S. (2006). The Glutamate/GABA-Glutamine Cycle: Aspects of Transport, Neurotransmitter Homeostasis and Ammonia Transfer. J. Neurochem. 98, 641–653. doi: 10.1111/j.1471-4159.2006.03913.x
Bando, H., Lee, Y., Sakaguchi, N., Pradipta, A., Ma, J. S., Tanaka, S., et al. (2018b). Inducible Nitric Oxide Synthase Is a Key Host Factor for Toxoplasma GRA15-Dependent Disruption of the Gamma Interferon-Induced Antiparasitic Human Response. MBio 9, e01738–18. doi: 10.1128/mBio.01738-18
Bando, H., Lee, Y., Sakaguchi, N., Pradipta, A., Sakamoto, R., Tanaka, S., et al. (2019). Toxoplasma Effector GRA15-Dependent Suppression of IFN-γ-Induced Antiparasitic Response in Human Neurons. Front. Cell Infect. Microbiol. 9, 140. doi: 10.3389/fcimb.2019.00140
Bando, H., Sakaguchi, N., Lee, Y., Pradipta, A., Ma, J. S., Tanaka, S., et al. (2018a). Toxoplasma Effector TgIST Targets Host IDO1 to Antagonize the IFN-Gamma-Induced Anti-Parasitic Response in Human Cells. Front. Immunol. 9, 2073. doi: 10.3389/fimmu.2018.02073
Baude, A., Strube, C., Tell, F., Kessler, J. P. (2009). Glutamatergic Neurotransmission in the Nucleus Tractus Solitarii: Structural and Functional Characteristics. J. Chem. Neuroanat. 38, 145–153. doi: 10.1016/j.jchemneu.2009.03.004
Bierly, A. L., Shufesky, W. J., Sukhumavasi, W., Morelli, A. E., Denkers, E. Y. (2008). Dendritic Cells Expressing Plasmacytoid Marker PDCA-1 are Trojan Horses During Toxoplasma Gondii Infection. J. Immunol. 181, 8485–8491. doi: 10.4049/jimmunol.181.12.8485
Blume, M., Rodriguez-Contreras, D., Landfear, S., Fleige, T., Soldati-Favre, D., Lucius, R., et al. (2009). Host-Derived Glucose and its Transporter in the Obligate Intracellular Pathogen Toxoplasma Gondii are Dispensable by Glutaminolysis. Proc. Natl. Acad. Sci. U. S. A. 106, 12998–13003. doi: 10.1073/pnas.0903831106
Bohne, W., Heesemann, J., Gross, U. (1993). Induction of Bradyzoite-Specific Toxoplasma Gondii Antigens in Gamma Interferon-Treated Mouse Macrophages. Infect. Immun. 61, 1141–1145. doi: 10.1128/iai.61.3.1141-1145.1993
Bohne, W., Heesemann, J., Gross, U. (1994). Reduced Replication of Toxoplasma Gondii is Necessary for Induction of Bradyzoite-Specific Antigens: A Possible Role for Nitric Oxide in Triggering Stage Conversion. Infect. Immun. 62, 1761–1767. doi: 10.1128/iai.62.5.1761-1767.1994
Boothroyd, J. C. (2009). Toxoplasma Gondii: 25 Years and 25 Major Advances for the Field. Int. J. Parasitol. 39, 935–946. doi: 10.1016/j.ijpara.2009.02.003
Braissant, O., Mclin, V. A., Cudalbu, C. (2013). Ammonia Toxicity to the Brain. J. Inherit. Metab. Dis. 36, 595–612. doi: 10.1007/s10545-012-9546-2
Braun, D., Longman, R. S., Albert, M. L. (2005). A Two-Step Induction of Indoleamine 2,3 Dioxygenase (IDO) Activity During Dendritic-Cell Maturation. Blood 106, 2375–2381. doi: 10.1182/blood-2005-03-0979
Brooks, J. M., Carrillo, G. L., Su, J., Lindsay, D. S., Fox, M. A., Blader, I. J. (2015). Toxoplasma Gondii Infections Alter GABAergic Synapses and Signaling in the Central Nervous System. mBio 6, e01428–e01415. doi: 10.1128/mBio.01428-15
Cabral, C. M., Tuladhar, S., Dietrich, H. K., Nguyen, E., Macdonald, W. R., Trivedi, T., et al. (2016). Neurons are the Primary Target Cell for the Brain-Tropic Intracellular Parasite Toxoplasma Gondii. PloS Pathog. 12, e1005447. doi: 10.1371/journal.ppat.1005447
Cheng, J. H., Xu, X., Li, Y. B., Zhao, X. D., Aosai, F., Shi, S. Y., et al. (2020). Arctigenin Ameliorates Depression-Like Behaviors in Toxoplasma Gondii-Infected Intermediate Hosts via the TLR4/NF-κB and TNFR1/NF-κB Signaling Pathways. Int. Immunopharmacol. 82, 106302. doi: 10.1016/j.intimp.2020.106302
Coombes, J. L., Charsar, B. A., Han, S. J., Halkias, J., Chan, S. W., Koshy, A. A., et al. (2013). Motile Invaded Neutrophils in the Small Intestine of Toxoplasma Gondii-Infected Mice Reveal a Potential Mechanism for Parasite Spread. Proc. Natl. Acad. Sci. U.S.A. 110, E1913–E1922. doi: 10.1073/pnas.1220272110
David, C. N., Frias, E. S., Szu, J. I., Vieira, P. A., Hubbard, J. A., Lovelace, J., et al. (2016). GLT-1-Dependent Disruption of CNS Glutamate Homeostasis and Neuronal Function by the Protozoan Parasite Toxoplasma Gondii. PloS Pathog. 12, e1005643. doi: 10.1371/journal.ppat.1005643
Dubey, J. P. (2009). History of the Discovery of the Life Cycle of Toxoplasma Gondii. Boca Raton, FL: CRC Press. Int. J. Parasitol. 39, 877–882. doi: 10.1016/j.ijpara.2009.01.005
El Mestikawy, S., Wallén-Mackenzie, A., Fortin, G. M., Descarries, L., Trudeau, L. E. (2011). From Glutamate Co-Release to Vesicular Synergy: Vesicular Glutamate Transporters. Nat. Rev. Neurosci. 12, 204–216. doi: 10.1038/nrn2969
Erecińska, M., Silver, I. A. (1990). Metabolism and Role of Glutamate in Mammalian Brain. Prog. Neurobiol. 35, 245–296. doi: 10.1016/0301-0082(90)90013-7
Ferguson, D. J., Huskinson-Mark, J., Araujo, F. G., Remington, J. S. (1994). An Ultrastructural Study of the Effect of Treatment With Atovaquone in Brains of Mice Chronically Infected With the ME49 Strain of Toxoplasma Gondii. Int. J. Exp. Pathol. 75, 111–116.
Ferreira, A., Caceres, A. (1992). Expression of the Class III Beta-Tubulin Isotype in Developing Neurons in Culture. J. Neurosci. Res. 32, 516–529. doi: 10.1002/jnr.490320407
Ferreira-Da-Silva Mda, F., Takács, A. C., Barbosa, H. S., Gross, U., Lüder, C. G. (2009). Primary Skeletal Muscle Cells Trigger Spontaneous Toxoplasma Gondii Tachyzoite-to-Bradyzoite Conversion at Higher Rates Than Fibroblasts. Int. J. Med. Microbiol. 299, 381–388. doi: 10.1016/j.ijmm.2008.10.002
Fox, B. A., Gigley, J. P., Bzik, D. J. (2004). Toxoplasma Gondii Lacks the Enzymes Required for De Novo Arginine Biosynthesis and Arginine Starvation Triggers Cyst Formation. Int. J. Parasitol. 34, 323–331. doi: 10.1016/j.ijpara.2003.12.001
Frenkel, J. K., Remington, J. S. (1980). Hepatitis in Toxoplasmosis. N. Engl. J. Med. 302, 178–179. doi: 10.1056/NEJM198001173020316
Gomez-Marin, J. E. (2000). No NO Production During Human Toxoplasma Infection. Parasitol. Today 16, 131. doi: 10.1016/S0169-4758(99)01614-2
González-González, I. M., Cubelos, B., Giménez, C., Zafra, F. (2005). Immunohistochemical Localization of the Amino Acid Transporter SNAT2 in the Rat Brain. Neuroscience 130, 61–73. doi: 10.1016/j.neuroscience.2004.09.023
Gormley, P. D., Pavesio, C. E., Minnasian, D., Lightman, S. (1998). Effects of Drug Therapy on Toxoplasma Cysts in an Animal Model of Acute and Chronic Disease. Invest. Ophthalmol. Vis. Sci. 39, 1171–1175.
Halonen, S. K., Lyman, W. D., Chiu, F. C. (1996). Growth and Development of Toxoplasma Gondii in Human Neurons and Astrocytes. J. Neuropathol. Exp. Neurol. 55, 1150–1156. doi: 10.1097/00005072-199611000-00006
Kasper, L. H. (1989). Identification of Stage-Specific Antigens of Toxoplasma Gondii. Infect. Immun. 57, 668–672. doi: 10.1128/iai.57.3.668-672.1989
Langel, J., Ikeno, T., Yan, L., Nunez, A. A., Smale, L. (2018). Distributions of GABAergic and Glutamatergic Neurons in the Brains of a Diurnal and Nocturnal Rodent. Brain Res. 1700, 152–159. doi: 10.1016/j.brainres.2018.08.019
Lee, I. P., Evans, A. K., Yang, C., Works, M. G., Kumar, V., De Miguel, Z., et al. (2014). Toxoplasma Gondii is Dependent on Glutamine and Alters Migratory Profile of Infected Host Bone Marrow Derived Immune Cells Through SNAT2 and CXCR4 Pathways. PloS One 9, e109803. doi: 10.1371/journal.pone.0109803
Li, Y., Severance, E. G., Viscidi, R. P., Yolken, R. H., Xiao, J. (2019). Persistent Toxoplasma Infection of the Brain Induced Neurodegeneration Associated With Activation of Complement and Microglia. Infect. Immun. 87, e00139–19. doi: 10.1128/iai.00139-19
Lüder, C. G. K., Rahman, T. (2017). Impact of the Host on Toxoplasma Stage Differentiation. Microb. Cell 4, 203–211. doi: 10.15698/mic2017.07.579
Lyons, R. E., Mcleod, R., Roberts, C. W. (2002). Toxoplasma Gondii Tachyzoite-Bradyzoite Interconversion. Trends Parasitol. 18, 198–201. doi: 10.1016/s1471-4922(02)02248-1
Ma, J. S., Sasai, M., Ohshima, J., Lee, Y., Bando, H., Takeda, K., et al. (2014). Selective and Strain-Specific NFAT4 Activation by the Toxoplasma Gondii Polymorphic Dense Granule Protein GRA6. J. Exp. Med. 211, 2013–2032. doi: 10.1084/jem.20131272
Mayoral, J., Di Cristina, M., Carruthers, V. B., Weiss, L. M. (2020). Toxoplasma Gondii: Bradyzoite Differentiation In Vitro and In Vivo. Methods Mol. Biol. 2071, 269–282. doi: 10.1007/978-1-4939-9857-9_15
Montoya, J. G., Remington, J. S. (2008). Management of Toxoplasma Gondii Infection During Pregnancy. Clin. Infect. Dis. 47, 554–566. doi: 10.1086/590149
Ohshima, J., Lee, Y., Sasai, M., Saitoh, T., Su Ma, J., Kamiyama, N., et al. (2014). Role of Mouse and Human Autophagy Proteins in IFN-Gamma-Induced Cell-Autonomous Responses Against Toxoplasma Gondii. J. Immunol. 192, 3328–3335. doi: 10.4049/jimmunol.1302822
Passeri, E., Jones-Brando, L., Bordón, C., Sengupta, S., Wilson, A. M., Primerano, A., et al. (2016). Infection and Characterization of Toxoplasma Gondii in Human Induced Neurons From Patients With Brain Disorders and Healthy Controls. Microbes Infect. 18, 153–158. doi: 10.1016/j.micinf.2015.09.023
Pfefferkorn, E. R., Rebhun, S., Eckel, M. (1986). Characterization of an Indoleamine 2,3-Dioxygenase Induced by Gamma-Interferon in Cultured Human Fibroblasts. J. Interferon Res. 6, 267–279. doi: 10.1089/jir.1986.6.267
Radke, J. R., Donald, R. G., Eibs, A., Jerome, M. E., Behnke, M. S., Liberator, P., et al. (2006). Changes in the Expression of Human Cell Division Autoantigen-1 Influence Toxoplasma Gondii Growth and Development. PloS Pathog. 2, e105. doi: 10.1371/journal.ppat.0020105
Radke, J. B., Lucas, O., De Silva, E. K., Ma, Y., Sullivan, W. J., Jr., Weiss, L. M., et al. (2013). ApiAP2 Transcription Factor Restricts Development of the Toxoplasma Tissue Cyst. Proc. Natl. Acad. Sci. U. S. A. 110, 6871–6876. doi: 10.1073/pnas.1300059110
Robert-Gangneux, F., Darde, M. L. (2012). Epidemiology of and Diagnostic Strategies for Toxoplasmosis. Clin. Microbiol. Rev. 25, 264–296. doi: 10.1128/cmr.05013-11
Soête, M., Camus, D., Dubremetz, J. F. (1994). Experimental Induction of Bradyzoite-Specific Antigen Expression and Cyst Formation by the RH Strain of Toxoplasma Gondii In Vitro. Exp. Parasitol. 78, 361–370. doi: 10.1006/expr.1994.1039
Sutterland, A. L., Fond, G., Kuin, A., Koeter, M. W., Lutter, R., Van Gool, T., et al. (2015). Beyond the Association. Toxoplasma Gondii in Schizophrenia, Bipolar Disorder, and Addiction: Systematic Review and Meta-Analysis. Acta Psychiatr. Scand. 132, 161–179. doi: 10.1111/acps.12423
Swierzy, I. J., Lüder, C. G. (2015). Withdrawal of Skeletal Muscle Cells From Cell Cycle Progression Triggers Differentiation of Toxoplasma Gondii Towards the Bradyzoite Stage. Cell Microbiol. 17, 2–17. doi: 10.1111/cmi.12342
Tanaka, N., Ashour, D., Dratz, E., Halonen, S. (2016). Use of Human Induced Pluripotent Stem Cell-Derived Neurons as a Model for Cerebral Toxoplasmosis. Microbes Infect. 18, 496–504. doi: 10.1016/j.micinf.2016.03.012
Tobin, C., Pollard, A., Knoll, L. (2010). Toxoplasma Gondii Cyst Wall Formation in Activated Bone Marrow-Derived Macrophages and Bradyzoite Conditions. J. Vis. Exp. 42, 2091. doi: 10.3791/2091
Tomavo, S., Fortier, B., Soete, M., Ansel, C., Camus, D., Dubremetz, J. F. (1991). Characterization of Bradyzoite-Specific Antigens of Toxoplasma Gondii. Infect. Immun. 59, 3750–3753. doi: 10.1128/iai.59.10.3750-3753.1991
Tomita, T., Bzik, D. J., Ma, Y. F., Fox, B. A., Markillie, L. M., Taylor, R. C., et al. (2013). The Toxoplasma Gondii Cyst Wall Protein CST1 is Critical for Cyst Wall Integrity and Promotes Bradyzoite Persistence. PloS Pathog. 9, e1003823. doi: 10.1371/journal.ppat.1003823
Umar, S., van der Laarse, A. (2010). Nitric Oxide and Nitric Oxide Synthase Isoforms in the Normal, Hypertrophic, and Failing Heart. Mol. Cell Biochem. 333, 191–201. doi: 10.1007/s11010-009-0219-x
Unno, A., Suzuki, K., Xuan, X., Nishikawa, Y., Kitoh, K., Takashima, Y. (2008). Dissemination of Extracellular and Intracellular Toxoplasma Gondii Tachyzoites in the Blood Flow. Parasitol. Int. 57, 515–518. doi: 10.1016/j.parint.2008.06.004
Varoqui, H., Zhu, H., Yao, D., Ming, H., Erickson, J. D. (2000). Cloning and Functional Identification of a Neuronal Glutamine Transporter. J. Biol. Chem. 275, 4049–4054. doi: 10.1074/jbc.275.6.4049
Waldman, B. S., Schwarz, D., Wadsworth, M. H., 2nd, Saeij, J. P., Shalek, A. K., Lourido, S. (2020). Identification of a Master Regulator of Differentiation in Toxoplasma. Cell 180, 359–372.e316. doi: 10.1016/j.cell.2019.12.013
Wang, F., Chen, S., Jiang, Y., Zhao, Y., Sun, L., Zheng, B., et al. (2018). Effects of Ammonia on Apoptosis and Oxidative Stress in Bovine Mammary Epithelial Cells. Mutagenesis 33, 291–299. doi: 10.1093/mutage/gey023
Watts, E., Zhao, Y., Dhara, A., Eller, B., Patwardhan, A., Sinai, A. P. (2015). Novel Approaches Reveal That Toxoplasma Gondii Bradyzoites Within Tissue Cysts Are Dynamic and Replicating Entities In Vivo. mBio 6, e01155–e01115. doi: 10.1128/mBio.01155-15
Weiss, L. M., Laplace, D., Takvorian, P. M., Tanowitz, H. B., Cali, A., Wittner, M. (1995). A Cell Culture System for Study of the Development of Toxoplasma Gondii Bradyzoites. J. Eukaryot. Microbiol. 42, 150–157. doi: 10.1111/j.1550-7408.1995.tb01556.x
Xu, X., Meng, Y., Li, L., Xu, P., Wang, J., Li, Z., et al. (2019). Overview of the Development of Glutaminase Inhibitors: Achievements and Future Directions. J. Med. Chem. 62, 1096–1115. doi: 10.1021/acs.jmedchem.8b00961
Yamamoto, M., Okuyama, M., Ma, J. S., Kimura, T., Kamiyama, N., Saiga, H., et al. (2012). A Cluster of Interferon-Gamma-Inducible P65 GTPases Plays a Critical Role in Host Defense Against Toxoplasma Gondii. Immunity 37, 302–313. doi: 10.1016/j.immuni.2012.06.009
Yap, G. S., Scharton-Kersten, T., Charest, H., Sher, ,. A. (1998). Decreased Resistance of TNF Receptor P55- and P75-Deficient Mice to Chronic Toxoplasmosis Despite Normal Activation of Inducible Nitric Oxide Synthase In Vivo. J. Immunol. 160, 1340–1345.
Zeng, H., Sanes, J. R. (2017). Neuronal Cell-Type Classification: Challenges, Opportunities and the Path Forward. Nat. Rev. Neurosci. 18, 530–546. doi: 10.1038/nrn.2017.85
Keywords: bradyzoite, IFN-γ, iPSC-derived glutamatergic neurons, Toxoplasma gondii, human
Citation: Bando H, Fukuda Y, Watanabe N, Olawale JT and Kato K (2022) Depletion of Intracellular Glutamine Pools Triggers Toxoplasma gondii Stage Conversion in Human Glutamatergic Neurons. Front. Cell. Infect. Microbiol. 11:788303. doi: 10.3389/fcimb.2021.788303
Received: 02 October 2021; Accepted: 16 December 2021;
Published: 13 January 2022.
Edited by:
Moritz Treeck, Francis Crick Institute, United KingdomReviewed by:
Martin Blume, Robert Koch Institute (RKI), GermanyCarsten Lüder, University Medical Center Göttingen, Germany
Emma Harriet Wilson, University of California, Riverside, United States
Copyright © 2022 Bando, Fukuda, Watanabe, Olawale and Kato. This is an open-access article distributed under the terms of the Creative Commons Attribution License (CC BY). The use, distribution or reproduction in other forums is permitted, provided the original author(s) and the copyright owner(s) are credited and that the original publication in this journal is cited, in accordance with accepted academic practice. No use, distribution or reproduction is permitted which does not comply with these terms.
*Correspondence: Kentaro Kato, a2VudGFyby5rYXRvLmM3QHRvaG9rdS5hYy5qcA==